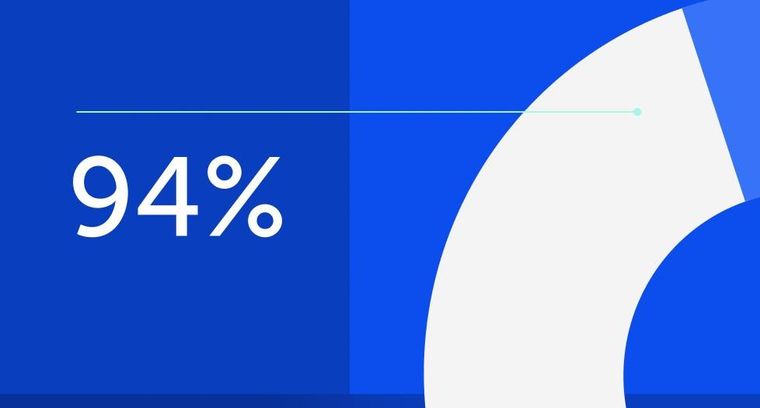
94% of researchers rate our articles as excellent or good
Learn more about the work of our research integrity team to safeguard the quality of each article we publish.
Find out more
ORIGINAL RESEARCH article
Front. Mar. Sci., 26 August 2022
Sec. Marine Biogeochemistry
Volume 9 - 2022 | https://doi.org/10.3389/fmars.2022.920483
This article is part of the Research TopicZooplankton and Nekton: Gatekeepers of the Biological Pump - Volume IIView all 5 articles
Life strategies, ecophysiological performances and diel vertical migration (DVM) of zooplankton key species affect the efficiency and strength of the biological carbon pump (BCP). However, it is unclear to what extent different functional groups affect the BCP. Depth-stratified day and night samples (0-800 m) from the subtropical South Atlantic were analyzed focusing on the calanoid copepod community. Calanoid abundance, biomass distribution and species-specific impact on the passive (fecal pellets) and active (via DVM) vertical flux of carbon were determined. Species were assigned to different migrant groups where, their contributions were estimated by using the proportion of the migratory community instead of simple day-night differences in biomass. This novel approach leads to more robust flux estimates, particularly for small sample sizes. According to migration ranges and day/night residence depth, functional groups were characterized, i.e. small- and large-scale epipelagic and mesopelagic migrants. Epipelagic small-scale migrants transported respiratory (1.5 mg C m-2 d-1) and fecal pellet (1.1 mg C m-2 d-1) carbon from the upper to the lower epipelagic zone, where the latter can fuel the microbial loop, and thus deep chlorophyll maxima, or be ingested by other zooplankton. Large-scale migrants actively transported up to 10.5 mg C m-2 d-1 of respiratory carbon from the epipelagic layer into the twilight zone. The majority was transported by Pleuromamma borealis (5.7 mg C m-2 d-1) into the upper mesopelagic. In addition, up to 8.0 mg C m-2 d-1 was potentially egested as fecal material by large-scale zone shifters. Mesopelagic migrants transported respiratory (0.2 mg C m-2 d-1) and fecal pellet carbon (0.1 mg C m-2 d-1) even deeper into the ocean. Community consumption of migrants in the epipelagic layer during the night was 98 mg C m-2 d-1, while non-migrants consumed 98-208 mg C m-2 d-1 in the epipelagic zone, with a potential subsequent egestion of 29-62 mg C m-2 d-1. This carbon may fuel omnivorous-detritivorous feeding, the microbial loop and/or may sink as fecal pellets. This case study shows how calanoid functional groups mediate carbon fluxes in the subtropical South Atlantic Ocean and demonstrates how detailed community analyses can elucidate the complexity of pelagic carbon budgets.
The biological carbon pump (BCP) is a key mechanism within the global carbon cycle, and the transfer of organic carbon derived from primary production into the deep sea is strongly mediated by the zooplankton link. The BCP removes 5-16 Gt C y-1 from surface waters into the oceans’ interior, ultimately reducing atmospheric CO2 levels. Approximately 1% of this organic carbon reaches the deep-sea sediments (Falkowski et al., 1998; Henson et al., 2011; Sigman and Hain, 2012; Archibald et al., 2019b). Marine food webs play an essential role in mediating the CO2 exchange and transformation between the atmosphere and the oceans (Steinberg and Landry, 2017), with 21-65% of marine primary production (PP) channeled through mesozooplankton to higher trophic levels (Hernández-León et al., 2001; Hernández-León and Ikeda, 2005; Bode et al., 2018b).
Zooplankton modifies organic carbon via ingestion, sloppy feeding, incorporation into body tissue (growth, reproduction), egestion, i.e. repackaging into fecal pellets, excretion and respiration (Iversen et al., 2010; Steinberg and Landry, 2017; Maas et al., 2020; Pauli et al., 2021). In terms of passive carbon fluxes, these processes affect aggregate size spectra, biochemical composition, and consequently, sinking speed of particulate organic carbon on its way to the deep sea (Kiørboe, 2000; Iversen and Ploug, 2010). Fecal pellets can be degraded by the microbial loop, re-ingested by zooplankton and/or they may sink further to deeper layers forming larger, rapidly sinking aggregates (Stamieszkin et al., 2015; Steinberg and Landry, 2017; Pauli et al., 2021). Fecal pellet flux usually accounts for ≤40% of total passive POC flux. However, it is highly variable and strongly influenced by sporadic events, e.g. salp “blooms” (Bruland and Silver, 1981; Perissinotto and Pakhomov, 1998). In a recent global model, it was estimated that the passive carbon flux (= gravitational pump) is responsible for 70% of total global carbon export, of which 85% were zooplankton pellets, 15% sinking phytoplankton aggregates, while migrating zooplankton accounted for ~10% of total export (Nowicki et al., 2022). Pellet size, density and the abundance of key zooplankton taxa are critical determinants of passive POC fluxes. Pellets of copepods and euphausiids are able to sink tens to hundreds of meters per day, while larger and denser salp fecal pellets cover a daily distance of more than one thousand meters (Thor et al., 2003; Turner, 2015; Steinberg and Landry, 2017; Karakuş et al., 2021; Pauli et al., 2021). In the Southern Ocean, for instance, feeding behavior and vertical distribution of the zooplankton community directly impacted the amount of carbon exported to the deep ocean (Manno et al., 2015). Therefore, the strength (surface processes of carbon uptake) and efficiency (subsurface processes of carbon transport to the deep sea) of the BCP depend heavily on biological components, e.g. microbial activity, zooplankton composition, grazing and particle aggregation, which may change regionally and/or seasonally (De La Rocha and Passow, 2007; Buesseler and Boyd, 2009; Turner, 2015; Armengol et al., 2019).
Active vertical carbon transport by diel migrants can represent a considerable portion of total carbon export (Al-Mutairi and Landry, 2001; Kobari et al., 2013; Stukel et al., 2013; Kwong et al., 2020), depending on the size, taxonomic structure of the associated zooplankton community and also hydrographic conditions (Al-Mutairi and Landry, 2001; Steinberg et al., 2008; Kiko and Hauss, 2019; Tutasi and Escribano, 2020). In the Sargasso Sea, active carbon flux comprised 5-33% of the passive POC flux, and an increase in migrant mesozooplankton biomass caused a significant increase in the mean annual active carbon transport from the epi- to the mesopelagic zone (Steinberg et al., 2012). Shifts in the zooplankton community composition induced by climate change directly affect the global carbon cycle by impacting the transport of organic material from the surface ocean to great depths (Brun et al., 2019). Via vertical migration, organisms can transport biomass to deeper layers, where they respire, produce fecal pellets and/or they can be ingested by deeper-dwelling predators or are exposed to natural mortality. It is recognized that zooplankton body size, feeding strategies and DVM behavior affect the efficiency of the BCP (Steinberg et al., 2000; Al-Mutairi and Landry, 2001; Isla et al., 2015; Cavan et al., 2017; Brun et al., 2019; Hernández-León et al., 2019).
Copepod communities are characterized by high taxonomic and functional diversity (Steinberg and Landry, 2017) and they comprise 50-95% of total mesozooplankton biomass, playing a crucial role in marine food webs, biogeochemical cycles and consequently, in the BCP (Longhurst and Harrison, 1989; Turner, 2004; Brun et al., 2019). DVM is usually characterized by an ascent of certain zooplankton species to surface waters at dusk/night, where they feed, followed by a descent at dawn/day, after which they reside at depth until the next ascent (Pearre, 2003; Isla et al., 2015). In contrast to this regular DVM, other migration patterns have been described, such as inverse DVM (iDVM) with a reversed movement pattern (Ohman et al., 1983) and ontogenetic vertical migration (OVM), where vertical movement is related to developmental stages (Hays, 1995; Holliland et al., 2012). Inverse diel migrants feed in the upper layers during the day and migrate to deeper layers during the night, presumably to maximize feeding while avoiding competition and/or predation by regular migrants (Bosch and Rowland Taylor, 1973; Ohman et al., 1983; Pinti et al., 2019). Timing and distances traveled via DVM vary among species and regions, and the impact of this behavior on the active carbon flux critically depends on the biomass and metabolic demands of migrants and their respective DVM ranges. Grouping zooplankton communities by their key traits such as size, metabolic demand, feeding strategy and DVM behavior will help to understand their role in the BCP (Hays et al., 1994; Cavan et al., 2017; Brun et al., 2019; Teuber et al., 2019; Tutasi and Escribano, 2020).
Data on copepod distribution with high taxonomic resolution and their impact on the BCP in the subtropical South Atlantic are scarce. The present case study investigated vertical migration behavior of calanoid copepods and how they potentially affect the BCP in this area. In particular, we 1) describe the calanoid community structure and composition with a high taxonomic and vertical resolution; 2) identify diel vertical migrants; 3) determine key species involved in the removal of organic matter (ingestion) and active transfer of carbon out of the epipelagic zone and; 4) identify and compare different calanoid groups and elucidate their role in active and passive carbon fluxes via respiration and fecal pellet production (egestion). We discuss how the different calanoid functional groups impact the ocean’s carbon cycle focusing on major traits such as body size, energetic demands, feeding strategies and DVM behavior. Finally, the high taxonomic and vertical resolution allowed us to develop a new method of estimating carbon fluxes by using the proportion of the taxon-specific migratory community instead of simple day-night differences in biomass. This new approach leads to more robust flux estimates, being less prone to errors, particularly for small sample sizes. Ultimately, this new approach provides a better framework to understand the importance of community composition and species-specific traits for the carbon flux mediated by calanoid copepods in the subtropical South Atlantic.
Mesozooplankton samples were collected in the southeastern Atlantic Ocean during the research cruise ANT XXIX/1 with RV Polarstern in November 2012. Stratified vertical hauls were carried out within 24 h with a Hydro-Bios Multinet Maxi (0.5 m2 net opening, 9 nets, 150 µm mesh size) from 800 m depth to the surface (strata: 800-700-600-500-400-300-200-100-50-0 m). The filtered water volume was measured with a flowmeter attached to the net opening. After retrieval, samples were preserved in a 4% borax-buffered formaldehyde in seawater solution. Abundance and community structure of calanoid copepods of one day (stn. 16; bottom depth 5,433 m) and one night station (stn. 15; bottom depth 5,462 m) were analyzed (Figure 1). Simultaneous profiles of temperature, salinity, and dissolved oxygen content were obtained with a conductivity temperature depth sensor (CTD) attached to a rosette water sampler (Fahrbach and Rohardt, 2008). Primary production rates were measured at each station via 13C bicarbonate tracer incubations, for details consult Fonseca-Batista et al. (2017).
Figure 1 Study area and physical parameters. (A) Day (stn. 16, grey dot) and night (stn. 15, black dot) stations located in the southeastern subtropical Atlantic; (B) Temperature (°C, red), salinity (black) and dissolved oxygen (µmol L-1, blue, only stn. 15) for day (left) and night (right) from surface to 700 m depth. Stn. 17 (open circle) was added for primary production reference data.
In the home laboratory, formalin-preserved mesozooplankton samples were split (Hernández-LeónIkeda, 2005) and transferred to a sorting solution (0.5% propylene-phenoxetol, 5% propylene glycol and 94.5% deionized freshwater; Steedman, 1976). Calanoid copepods were sorted according to their developmental stages (copepodids C1-3 and C4/5, adult females and males), counted and identified to genus or, if possible, to species level under a dissecting microscope (Leica MZ12) (Bradford-Grieve et al., 1999; Razouls et al., 2005). Rare species (<100 individuals per sample) were counted from the entire sample. Total length (TL, mm) of up to 100 calanoid individuals per taxonomic category (i.e. family/genus/species) and stage was measured (~6,600 specimens in total). Abundance and size measurements data for all species found here are available from the data publisher PANGAEA via the persistent identifier https://doi.pangaea.de/10.1594/PANGAEA.946061. Dry mass (mg, DM) of calanoids [1] was calculated based on the median TL of each taxonomic category (Chisholm and Roff, 1990; Homma et al., 2011).
Individual respiration rates (µl O2 ind-1 h-1) for slow- [2, Rs] and fast-moving [3, Rf] calanoids were calculated by applying regression models based on ~300 in situ respiration measurements using a 10-Channel Fiber Optic Oxygen Meter (OXY-10, PreSens, Precision Sensing GmbH) during two research cruises in this area (Bode et al., 2013; Bode et al., 2018a). According to Bode et al. (2013) and Teuber et al. (2019), slow-moving copepods belong to the copepod families Eucalanidae, Subeucalanidae and Rhincalanidae, while all other copepods represented fast-moving species. Individual respiration rates were calculated from individual DM (mg) and in situ temperatures (T), i.e. the median temperature (°C) at the respective sampling depth, applying the equations from Bode et al. (2018a):
For non-migrating copepods, daily Rs and Rf were calculated for 24 h at their residence depth. For migrating copepods, a residence time of 12 h each was assumed in the upper and lower layers, respectively, and thus respiration and finally carbon demand were calculated for 12 h each at the two residence depths with the two respective in situ temperatures.
Since respiration rates from laboratory experiments usually reflect the copepods’ routine or standard metabolism, they can be converted to carbon units to calculate the copepods’ minimum energy requirements (Ikeda et al., 2001). For the calculation of respiratory carbon loss, oxygen consumption rates (R, µl O2 ind-1 d-1) were converted to respiratory carbon losses [4] (RC, µg C ind-1 d-1) assuming that 1 ml O2 equals 0.44 mg organic carbon using a respiratory quotient (RQ) of 0.82 for a mixed diet consisting of proteins, carbohydrates and lipids (Winberg, 1956; Gnaiger, 1983; Schukat et al., 2013). MMC is the molar mass of carbon (12 g mol-1) and MV the molar volume of an ideal gas (22.4 L mol-1):
Ingestion rates [5] (I, µg C ind-1 d-1) were calculated after Ikeda and Motoda (1978), using an absorption efficiency (U) of 0.7 and a gross growth efficiency (K1) of 0.3. Egestion rates (E; µg C ind-1 d-1) were assumed to be 30% of ingestion (E = 0.3 x I) (Ikeda and Motoda, 1978).
Total community ingestion and egestion for each taxon and copepodite stage was calculated based on their abundances per m2 in the respective depth interval.
Particulate organic carbon (POC) flux to each underlying depth layer was assessed based on average primary production rates (Cprod, mg C m-2 d-1) in this region as determined by Fonseca-Batista et al. (2017) during the research cruise, and applying the equation after Suess (1980) [6], where Cflux is the POC flux (mg C m-2 d-1) at water depth Z ≥ 50 m.
To assess the potential impact of calanoids on the POC flux, we used a box model after Homma et al. (2011) and modified after Bode et al. (2018a). Model parameters and assumptions were as follows: (i) the amount of organic carbon (OC) that enters the system (POCin) is provided by primary production (PP) for the surface layer (0-50 m) and it can be calculated for the subsequent strata according to [6] depending on water depth Z. (ii) The amount of OC that is lost within a layer can be consumed by calanoids; whatever is lost and is not directly consumed by calanoids, is accessible to other organisms within the same layer or will become available as suspended POC. (iii) The absorption efficiency (U = 0.7) is constant throughout the water column and the egestion of calanoids, i.e. fecal pellet production, contributes to the POC reaching the depth layer below. (iv) Remaining POC that is not ingested or is egested by others in the same layer, directly sinks. Lastly, (v) the RQ is constant throughout the water column and RC is lost as dissolved inorganic carbon (DIC) in the respective depth layer.
Based on differences in vertical distribution, species were assigned to four different types of migrant groups, switching between different depth zones with specific migration ranges, i.e. small-scale epipelagic migrants, large-scale DVM zone shifters, small- or large-scale migrants at the epi- and mesopelagic interface as well as large-scale mesopelagic migrants. To determine DVM distances, weighted mean depths (WMD) of taxa for both day and night stations [7] and migration ranges ΔZ (defined as WMDday minus WMDnight) were calculated after Andersen et al. (2001).
where ni is abundance per m3, zi is the depth range of the respective sampling interval and di is the mid-depth of the sampling interval. Copepod species were identified and classified as non-migrants (ΔZ < 25 m), small-scale migrants (25 m < ΔZ > 100 m), large-scale migrants (ΔZ > 100 m), while inverse migrants were characterized by negative ΔZ.
Migrant biomass (mg DM m-2) was estimated across different reference water depths, i.e. across 50 m depth (within the epipelagic zone), 200 m (across the upper mesopelagic boundary), 300 m (within the upper mesopelagic layer) and 400 m (across the lower mesopelagic boundary). Given the similarities between hydrographic regime and community composition among the sampled stations, we assume the migrants’ habitat distribution did not change substantially. Due to currents and changes in water masses, species composition varies naturally in marine pelagic ecosystems. To compensate for these irregularities in day/night biomass, migrant biomass was calculated based on the migratory portion (MP) of a respective taxon instead of the simple difference in biomass between day and night. To determine the MP crossing a certain depth layer, the MP of biomass was calculated based on the absolute biomass of the night-time station and the relative changes in biomass vertical distribution between day and night. Albeit net avoidance is not a concern for copepods, we propose this method to be applied for zooplankton carbon flux calculations in general. Therefore, to account for bias of net avoidance, the night-time biomass was determined as the final community to calculate the migrant’s population proportion.
The total respiratory carbon flux (RCflux) across the reference depths was calculated based on species-specific migrant biomass and energy requirement [8], indicating the daily downward RCflux (mg C m-2 d-1) calculated for 12 h at in situ temperatures at the respective resident depth:
where MRCdeep is the mass-specific respiration rate converted to carbon (mg C mg DM-1 h-1), respired at depth during day (DVM) or during night (iDVM) calculated at in situ temperatures, Btn is the integrated biomass of the respective migratory taxa at night (mg DM m-2), and MPz is the migrating portion of the population across the reference depth, i.e. the depth above or below which the largest MP was found.
Both stations were located at the margin of the subtropical South Atlantic gyre, under the influence of the Benguela Current (BC) upwelling system. Sea surface temperature (SST) was relatively low at 18-19°C compared to other subtropical open-ocean regions. The mixed layer reached down to approx. 40 m, with another subsurface mixed layer down to approx. 105 m (0-38 m: 18.8°C, 49-105 m: 17.8-17.4°C; Figure 1). Below that, temperature and salinity decreased steadily with depth, reaching 5°C at 700 m. An oxygen minimum zone (<60 µmol O2 L-1) was present at the day station 16 between 320 and 450 m (minimum of 41 µmol O2 L-1 at 386 m). For the night station, no oxygen profile is available due to technical problems. Chlorophyll a concentration was high compared to other oceanic regions in the eastern Atlantic Ocean during the research cruise. Primary production rates averaged 1012 mg C m-2 d-1 in this region during the cruise, which was used for our carbon budget calculations (1429 mg C m-2 d-1 at stn. 15, 676 mg C m-2 d-1 at stn. 16 and 930 mg C m-2 d-1 at stn. 17 in Fonseca-Batista et al. (2017). The hydrography has been described in detail in Bode et al. (2015); Bode et al., (2018a); Bode et al. (2018b) and Fonseca-Batista et al. (2017).
In total, 25 calanoid copepod families and 57 genera were identified, comprising at least 117 species. The mean total length of the calanoids varied widely, ranging from 0.4 mm for copepodids of Disco spp. to 6.7 mm for Eucalanus hyalinus adult females. The calanoid copepod community showed pronounced vertical changes and depth zones could be divided into upper and lower epipelagic (UEL = 0-100 m, LEL = 100-200 m) as well as upper and lower mesopelagic layer (UML = 200-400 m, LML = 400-800 m).
In general, abundance and biomass decreased with depth, with maxima in the surface layer during night and day and a bimodal distribution during the day (Figure 2). Total calanoid abundance (TCA) decreased from max. 1289 ind m-3 during the night and 508 ind m-3 during the day in the surface layer to 203 ind m-3 (night) and 94 ind m-3 (day) in the 50-100 m layer and further to 35 ind m-3 (night) and 24 ind m-3 (day) in the 100-200 m layer. TCA increased again in the 200-300 m layer with 45 ind m-3 during the night and 147 ind m-3 during the day. Minimum TCA occurred in the deepest layer (700-800 m) with 8 ind m-3 at night and 6 ind m-3 during the day (Figure 2). TCA averaged for each the UML and LML yielded higher abundances during the day (UML = 113 ind m-3; LML = 16 ind m-3) as compared to the night (UML = 51 ind m-3; LML = 12 ind m-3).
Figure 2 Vertical distribution of total calanoid copepod abundance (left) and biomass (right) from the surface to 800 m depth during day (grey bars) and night (black bars). The x-axis depicts the relative total calanoid abundance and respective biomass per station; the numbers beside each bar indicate absolute abundance (ind m-3) and biomass (mg DM m-3) in that specific depth layer.
Correspondingly, total calanoid biomass (TCB) was higher during the night with 33 mg DM m-3 in the upper 50 m compared to 14 mg DM m-3 during the day (Figure 2). TCB decreased strikingly with depth and was similar for both day and night in the 50-100 m layer (night-day = 4.4-4.3 mg DM m-3) and in the 100-200 m layer (night = 1.6 mg DM m-3; day = 1.1 mg DM m-3). TCB increased again in the 200-300 m layer with 4.8 mg DM m-3 during the night and 3.1 mg DM m-3 during the day. Minimum TCB occurred in the deepest layer (700-800 m) with 0.2 mg DM m-3 at night and 0.5 mg DM m-3 during the day (Figure 2). In accordance to abundance, TCB averaged for each the UML and LML yielded higher biomass at depth during the day (UML = 3.9 mg DM m-3; LML = 1.5 mg DM m-3) as opposed to the night (UML = 2.5 mg DM m-3; LML = 0.7 mg DM m-3).
Due to different roles in passive and active carbon fluxes, the calanoid community was divided into a non-migrant community at the respective residence depths and a migrant one between different depths, based on their species- and stage-specific weighted mean depths (WMDs) and migration ranges (ΔZ). If developmental stages showed different migration behavior within one species or genus, they were assigned to different groups. Non-migrant species of the families Eucalanidae, Subeucalanidae and Rhincalanidae were assigned to a separate group, the so-called “thrifty floater” community, since they are slow-moving copepods with low metabolic rates and potential bimodal vertical distribution. If ΔZ was >25 m but abundances were very low (≤0.5 ind/m-3), or rare species occurred only during day or night, their migration behavior could not be characterized, taxa were placed in an undetermined group. For details on WMDs see Supplementary Material.
In this study, around 42 calanoid taxa were identified as non-migrants (Supplementary Figure 1), as the difference between their day and night WMDs was <25 m. The non-migrant epipelagic community (0-200 m) consisted mostly of all copepodite and adult stages of Acartia spp., Nannocalanus minor, Scolecithrix danae and Centropages bradyi, and furthermore of Calocalanus C4/5 copepodids, Clausocalanus males and C1-3 stages, females and C4/5 copepodids of Ctenocalanus spp., Neocalanus gracilis C1-5 copepodids as well as females and copepodids C1-5 of Euchirella spp., Lucicutia gaussae and Mecynocera clausi. In addition, adult females and copepodids C1-5 of Paracalanidae (Delibus, Paracalanus) also had an important contribution to the energetic demands in the EL. In the upper and lower mesopelagic layers (200-800 m), the most important non-migrant taxa regarding the metabolic demands of the community were adult females and males of Metridia effusa, and Spinocalanus spp., all copepodite stages of Metridia venusta, Scaphocalanus and Temoropia spp., copepodids C1-5 of Pleuromamma quadrungulata and copepodids C4/C5 of Scolecithricella.
As mentioned above, within the non-migrants, we further characterized certain taxa based on their metabolic strategies. The “thrifty floater” term was coined by Teuber et al. (2019) and here referred to the group that consisted of E. hyalinus, Rhincalanus cornutus, Rhincalanus nasutus, Subeucalanus spp. (mostly Subeucalanus mochachus/pileatus and/or subtenuis) and Pareucalanus spp. (mostly Pareucalanus sewelli and/or langae) (Figure 3). Rather than undergoing DVM, these groups are assumed to have bimodal distribution patterns due to their special life cycle adaptations including opportunistic dormancy stages. Females of E. hyalinus and R. cornutus were dominant in this group from EL to LML, while females of R. nasutus were also dominant at depth. Females of Pareucalanus spp. were less abundant, occurring mostly in the EL. In addition to E. hyalinus and R. cornutus, adult females and copepodids C1-3 and C4/5 of Subeucalanus spp. as well as C1-3 and C4/5 stages of Pareucalanus spp. were also abundant at depths during the day (Figure 3).
Figure 3 “Thrifty floater” community. Relative biomass distribution for day (grey bars) and night (black bars) of main species within the thrifty floater community. Weighted mean depth (m) values for day and night of each group are indicated. Absolute total biomass values (mg DM m-2) are added for each taxon below the bars.
The undetermined group was mainly composed of Metridinidae C1-3. Due to the low taxonomic resolution, their migration behavior could not be resolved. Copepodids C1-3 of Scolecithricella spp., copepodids C4/5 of Pseudoamallothrix spp. and Metridia curticauda/brevicauda contributed to total calanoid ingestion but, their migratory behavior was also unclear (Supplementary Figure 1).
In total, 25 calanoid taxa performed large- (ΔZ >, 100, m) and small-scale (ΔZ between 25 and 100 m) regular DVM and inverse DVM (iDVM). Calanoids were separated into four migrant functional groups containing a regular (r) and inverse (i) DVM subgroup according to their WMDs during night and day, migration behavior (large-/small-scale) and their association with specific depth zones (EL, UML, LML) (see also Tables 1, 2 and Figure 4 for DVM, Figure 5 for iDVM). Metridia lucens was treated separately, as it is a known large-scale migrant. However, it was absent at our night station, but highly abundant at 200-800 m during the day (38 ind m-3). It was classified as a large-scale zone shifter, since copepodids C4/5 had a WMD of 440 m and females of 320 m during the day, assuming that 100% of the population migrated into the 0-50 m layer during night (Table 1).
Table 1 Respiratory carbon (RC flux) and egestive carbon (EC flux) flux of the dominant diel vertical migrating copepods.
Table 2 Percentage contribution of most important (>5% FG flux) regular migrants within respective functional groups to total respiratory carbon (RC) flux across reference depths.
Figure 4 Diel vertical migrants. (A) Biomass distribution of main regular diel vertical migrants (G1r – 2r) and (B) biomass distribution of main regular diel vertical migrants (G2r – 4r). Absolute total biomass values (mg DM m-2) are given for day (grey bars) and night (black bars) for each taxon. Weighted mean depths (m) for day and night for each group are indicated.
Figure 5 Inverse diel vertical migrants. Biomass distribution of main inverse diel vertical migrants (G1i–4i). Absolute total biomass values (mg DM m-2) are given for day (grey bars) and night (black bars) for each taxon. Weighted mean depths (m) for day and night of each group are indicated.
Group 1 - Small-scale epipelagic migrants: Small-scale migrants in the EL, migrating within 0-200 m, never leaving this depth zone.
1r: Copepodids C1-3, adult females of Calocalanus spp. were classified as small-scale migrants within the upper EL with WMDs of 80 and 72 m for the day and 30 and 45 m for the night, respectively. Mesocalanus tenuicornis females migrated within the entire EL, with WMD of 150 m during the day and 92 m at night (Figure 4 and Table 1).
1i: iDVM in the upper 100 m was performed by copepodids C1-3 and C4/5 of M. tenuicornis. WMDs for these stages were 34 and 36 m during the day, while at night WMDs were 63 and 64 m, respectively. N. gracilis females migrated within the entire EL with WMDs of 75 m during day and 156 m during night (Figure 5 and Table 1).
Group 2 - Large-scale DVM zone shifters: Large-scale migrants moving across the EL (200 m) to the UML and/or LML.
2r: Pleuromamma borealis had a large migration distance of >200 m with day WMDs of 247-257 m and night WMDs of 25-33 m for copepodids C1-5 and adult females. Day WMDs of females and C4/5 of Clausocalanus spp. (263 and 302 m, resp.) and Pleuromamma abdominalis (344 and 302 m, resp.) were similar to those of P. borealis. However, these two species were scattered in multiple upper water layers during the night, while a small part of the population seemed to stay at depth. Males of small Pleuromamma spp. (P. borealis/gracilis/piseki) were summarized in one category, as they are morphologically difficult to identify to species level. They had a WMD of 450 m during the day and 101 m at night. Other species were also large-scale zone shifters, however, with much lower abundances: Pleuromamma xiphias females (WMD day: 650 m, WMD night: 174 m), P. quadrungulata males (WMD day: 568 m, WMD night: 176 m), Pleuromamma robusta females (WMD day: 367 m, WMD night: 150 m), Scottocalanus securifrons females (WMD day: 450 m, WMD night: 150 m), Lucicutia c.f. ovalis females, males and C1-5 copepodids (WMDs day: 50-75 m, WMDs night: 750 m) (Figure 4 and Table 1).
2i: Large-scale iDVM was performed by Aetideus armatus (C1-3) and Aetideus giesbrechti females, exhibiting day WMDs of 75 m and 150 m, respectively, and night WMDs of 303-350 m (Figure 5 and Table 1). However, only a small part of the populations seemed to migrate (24% and 10% migratory portion, respectively).
Group 3 - Small- or large-scale DVM at the epi- and mesopelagic interface: Small- or large-scale migrants within the UML; the majority of the populations always remained below 200 m.
3r: Females of P. quadrungulata occurred with a daytime WMD of 350 m and a nighttime WMD of 228 m. Yet, a smaller part of the population also migrated into the LEL connecting this zone to the UML (Figure 4 and Table 1).
3i: Small-scale iDVM was performed by M. effusa (C1-5) and Scolecithricella spp. (males) with similar day and night WMDs of ~265/272 m and 313/354 m, respectively (Figure 5 and Table 1).
Group 4 - Large-scale DVM mesopelagic zone shifters: Large-scale migrants within the mesopelagic zone, switching between UML and LML.
4r: This category comprised typical deep-sea species including copepodids C4/5 of Scottocalanus and adult females of Augaptilus longicaudatus, Haloptilus paralongicirrus, Metridia venusta and Spinocalanus c.f. magnus. Daytime WMDs ranged between 431 to 650 m in this group, while nighttime WMDs were between 250 and 412 m (Figure 4 and Table 1).
4i: Females of Euchirella pulchra and Haloptilus c.f. oxycephalus comprised this group, with daytime WMDs of 485 and 546 m, respectively. At night, WMD was 650 m for both (Figure 5 and Table 1).
Total calanoid community ingestion (TCI) of the non-migrant group including the “thrifty floater” and undetermined groups in the EL (0-200 m) ranged from 98 mg C m-2 d-1 at the day stn. 16 to 208 mg C m-2 d-1 at the night (stn. 15), while migrants (G1r, G2r and M. lucens) consumed 98 mg C m-2 d-1, i.e. only nighttime feeding in the EL (Figure 6). Within the UML, 17 to 24 mg C m-2 d-1 were ingested by the non-migrant group and only 0.9 mg C m-2 d-1 by deeper migrant groups (G3r, G4r). Thus, the non-migrating community had the largest impact on carbon fluxes in the respective depth ranges. Non-migrant calanoids were responsible for the potential consumption of 10-21% of primary production in the EL, whereas another 10% could be attributed to migrant species. In the mesopelagic layers, rather low fractions of incoming POC were potentially consumed by calanoid copepods, with the majority of POCin consumed by non-migrants in the UML (8.3-12.0%) and in the LML (7.1-9.0%) (Figure 6).
Figure 6 Calanoid-mediated processes of removal and transfer of particulate organic carbon (POC). Incoming organic carbon is indicated as primary production (PP) at the surface layers on the left. Incoming (POCin) and outgoing POC (POCout) of 200 m, 400 m and 800 m are given after Suess (1980). The non-migrating groups (non-migrant, “thrifty floater”, undetermined) are summarized on the left giving total community ingestion (I), egestion (E) and respiration (R) for stns. 15/16 in the EL (0-200 m), UML (200-400 m) and LML (400-800 m). Potential impacts of their ingestion on PP or POCin, egestion on POCout and respiration on POClost are given, the latter indicating the fraction of organic carbon “lost” in that specific layer (after Suess, 1980) (POCin - POCout) and respired as inorganic carbon by copepods. On the right, only the regular migrant groups are shown due to the negligible impact of the inverse migrants in this study. Total community I, E and R are given for each migrant group as well as respiratory carbon (RC) and egested carbon (EC) are indicated with arrows down to the weighted mean depths of each group during the day. Active fluxes were determined across 100 m, 200 m and 400 m. Metridia lucens was added as separate migrant (large-scale zone shifter), because it was absent at the night station and the maximum potential I, E and R was calculated assuming a migratory portion of 100% into the 0-50 m layer. The “?” indicates this uncertainty. All values are given as mg C m-2 d-1.
Among the non-migrating taxa, N. minor females and C1-3 (9-35 mg C m-2 d-1), Acartia spp. females and C1-3 (28-36 mg C m-2 d-1) and M. clausi females (2-20 mg C m-2 d-1) contributed most to EL community consumption. Altogether, non-migrants consumed a total of 56-153 mg C m-2 d-1 in the upper 50 m, and the largest fraction was ingested by adult females with 34-75 mg C m-2 d-1. Overall, the undetermined DVM group ingested 5-9 mg C m-2 d-1 in the EL (0-200 m). The “thrifty floater” group ingested a total of 18-21 mg C m-2 d-1 in the EL, with the large E. hyalinus (8-14 mg C m-2 d-1) and R. cornutus (3-6 mg C m-2 d-1) showing highest community consumption rates. Since community ingestion decreased with depth while diversity increased, taxon-specific community ingestion was generally ≤10 mg C m-2 d-1 in the UML and ≤4 mg C m-2 d-1 in the LML.
Among the migrants, highest ingestion rate in the EL was observed for G2r (62 mg C m-2 d-1). Most of this carbon was consumed in the upper 0-50 m by P. borealis (44 mg C m-2 d-1). Within G1r migrants, a total of 7.6 mg C m-2 d-1 was ingested in the EL (Figure 6), with Calocalanus spp. being the main consumer (7.3 mg C m-2 d-1). Young copepodids C1-3 and adult females of small Calocalanus spp. and M. tenuicornis (migrant group G1r) transported up to 1.5 mg C m-2 d-1 as respiratory carbon (RC) and 1.1 mg C m-2 d-1 as egestive carbon (EC) from the 0-50 m into the 50-100 m layer. Migrant group G2r actively transported 7.9 mg RC m-2 d-1 and 6.0 mg EC m-2 d-1 from the EL down into upper mesopelagic layers, with P. borealis (5.7 mg RC m-2 d-1) as main contributor (C1-6 stages, Tables 1, 2). M. lucens was assumed to be a large-scale zone shifter. M. lucens copepodids C4/5 had a WMD of 440 m and females of 320 m during day, but the species was completely absent in the night samples. As known migrants, they potentially transported 2.6 mg RC m-2 d-1 and 2.0 mg EC m-2 d-1 from the surface layers into the UML and even LML. Assuming a migratory portion of 100% into the 0-50 m, M. lucens potentially added a maximum of 28 mg C m-2 d-1 to TCI in the surface layer (Figure 6 and Table 2).
Due to the very low abundances of mesopelagic migrants (G3r and G4r), active transport of carbon within the UML and into the LML was present but low (Table 1). Adult females of P. quadrungulata (G3r) actively transported 0.1 mg C m-2 d-1 as each, RC and EC across 300 m within the UML, while M. effusa (G3i) transported <0.1 mg C m-2 d-1 of RC and EC across that depth (Tables 1, 2). Likewise, low values were observed across 400 m for deeper LML migrants G4r (Table 1 and Figure 2). Inverse migrants made a very small contribution to the community fluxes. G1i and G3i were the most important groups, ingesting around 0.9 mg C m-2 d-1 in the upper 0-100 m and 0.4 mg C m-2 d-1 in the UML, respectively. Active transport by inverse migrants was very low with overall 0.2 mg C m-2 d-1 of both RC and EC (Table 1; G1i – G4i).
Comparing active versus passive carbon fluxes of depth-stratified calanoid copepod communities and identifying the key players involved in each community helps to disentangle carbon pathways and better understand the ocean carbon cycle. We identified different functional groups of calanoid communities with specific roles in export and recycling processes and consequently in the biological carbon pump (BCP) in the subtropical Southeast Atlantic, an area largely understudied.
The limited number of stations analyzed here represent large uncertainties in our flux estimates and functional groupings. Yet, to the best of our knowledge, no other studies within this particular region of the South Atlantic attempted to estimate export fluxes and migration patterns of the calanoid community, let alone in such detail. Despite the lack of replication, our results fit well to previous studies discussed below (see also Table 3). The calanoid community composition in this area corroborates the composition of our functional groups (Bode et al., 2018a; Bode et al., 2018b; Teuber et al., 2019). Mesotrophic conditions with high abundances of small- to medium-sized calanoids have been reported for the eastern subtropical South Atlantic influenced by the Benguela Current, i.e. upwelling filaments can extend up to 600 km offshore (~72-93 species found; Woodd-Walker, 2001; Woodd-Walker et al., 2002; Schnack-Schiel et al., 2010; Bode et al., 2018a; Bode et al., 2018b). Zooplankton biomass values in the upper 200 m have been reported to display rather weak seasonal and interannual signals in open ocean regions (Martin et al., 2015).
Table 3 Literature data of active carbon fluxes (respiratory carbon) from epi- to mesopelagic depths mediated by mesozooplankton in different areas/regions of the world ocean in comparison with our study.
Generally, studies focusing on carbon fluxes are restricted to the epipelagic layer (<200 m), with differences in biomass being only attributed to the presence of diel vertical migrants, disregarding other possible causes (e.g. currents/changes in water masses, species composition, patchiness). However, biomass and abundance mismatches have been observed in studies even when replication was possible. For instance, in the coastal upwelling system off northern Chile, total zooplankton biomass integrated over 600 m was ~1.7 times higher during the night than during the day (Escribano et al., 2009). Additionally, biomass of the copepod Eucalanus inermis integrated for the upper 600 m also varied 1.9-4.3 times between the same stations sampled during day and night off northern Chile (Hidalgo et al., 2005). Schukat et al. (2021) also observed a ~1.2-1.9 times difference in abundance (0-100 m) within the same stations during day and night (two 24 h stations, sampled at 6 h intervals) for the surface-concentrated species, Calanus chilensis, from the northern Humboldt Current off Peru. As such, differences in biomass/abundance between day and night (here, ~1.8 times higher biomass in the night sample) may not necessarily be a consequence of small sample size and/or too dissimilar oceanographic stations but appears to be a natural phenomenon of marine pelagic copepod communities. Consequently, our approach to calculate active flux based on the proportion of the taxon-specific migratory community to a given depth bypasses this issue and should be considered in future studies.
The processes on how migrant copepod communities affect export fluxes in the pelagic realm can vary according to seasonality (high migrant biomass leading to high carbon removal in post-bloom conditions, Isla et al., 2015), environmental parameters and region (Cavan et al., 2017; Archibald et al., 2019b; Kiko and Hauss, 2019), community composition (Yamaguchi et al., 2002; Wassmann et al., 2003; Brun et al., 2019; Soviadan et al., 2021) and size (Iversen et al., 2010; Turner, 2015; Ohman and Romagnan, 2016). The total active respiratory carbon (RC) flux of calanoid copepods across 200 m added up to 10.5 mg C m-2 d-1, which equals 3.8 g C m-2 y-1. This was about twice as high as the annual mean for the Bermuda Atlantic Time-Series study (BATS) area in the oligotrophic subtropical North Atlantic gyre with 1.5 ± 0.5 g C m-2 y-1 actively transported across 150 m (Table 3; Steinberg et al., 2012). Our study area was still affected by the highly productive Benguela Current (Bode et al., 2018a; Bode et al., 2018b), which may have caused higher abundances of migrant species, and as such, a higher RC flux compared to the one in the oligotrophic region from the BATS site. To compare our study with existing literature, we assumed a 12 h residence time at any given depth during the day or night to calculate the RC flux of migrants, which may lead to an overestimation (Dam et al., 1995; Zhang and Dam, 1997; Steinberg et al., 2012; Ariza et al., 2015). Nevertheless, according to the newest quantification of the biological carbon pump, the total carbon export for a respective “tropical” region, where our stations were located, was 30 g C m-2 y-1, of which 11% (equals 3.3 g C m-2 y-1) could be assigned to migrating zooplankton (Nowicki et al., 2022), thus, fitting well to the estimated values in the present study.
Considering that the South Atlantic has an area of ~40 million km2 (https://www.ngdc.noaa.gov), the active carbon flux of calanoid copepods from the epi- to the mesopelagic zone, mostly caused by small- to medium-sized P. borealis and M. lucens, yielded an estimate of 1.5 Mt C y-1 in the present study. This flux was actively transported down as respiratory carbon via DVM and may represent an important source of carbon removal in the oceans. In the Southern Ocean, macrozooplankton was estimated to contribute 0.12 Gt C y-1 to total modeled carbon export across 150 m (Karakuş et al., 2021) and zooplankton-mediated processes accounted for up to 80% of salp fecal pellet flux (Pauli et al., 2021). Altogether, a mean global carbon export of ~10.2 Gt C y-1 was recently estimated, still containing high uncertainties, especially due to high spatial and seasonal variability. This suggests a weakening of the ocean’s carbon storage capacities due to climate-change-induced stratification and expansion of the subtropical gyres (Nowicki et al., 2022). In contrast, in the northern North Atlantic, for instance, carbon export increased due to shifting distributions of abundant, large-sized copepods (Brun et al., 2019).
For macrozooplankton, e.g pelagic decapods, within the northern Benguela upwelling system, the potential RC flux out of the EL was 4.4 mg C m-2 d-1 and 2.6 mg C m-2 d-1 within the UML and LML between 200 and 600 m, respectively (Schukat et al., 2013). Species-specific migration behaviors are evident for the decapods, as also shown for the copepods in our study, emphasizing that migration patterns and distances vary across species. However, highly variable standard deviations for the species-specific WMDs (0 to 124 m) of the decapod species (Schukat et al., 2013) indicate the importance of further research on migration amplitudes for copepods in the Benguela Current and adjacent regions. Patchiness and net avoidance of macrozooplankton and micronekton can have a crucial impact on abundance and thus subsequent estimates such as RC flux, highly depending on the sampling efficiency of the net used (Schukat et al., 2013 and references therein). For copepod sampling, however, net avoidance is not a concerning factor (Pillar, 1984). In this study, biomass of non-migrants within 0-200 m did not show a considerable difference between day and night biomass (430 versus 578 mg C m-2, resp.), also indicating a minor potential effect of the sampling gear.
In the present study, the majority of organic carbon was consumed by the non-migrant groups, while their abundance and biomass decreased with depth, which is the typical pattern seen for pelagic ecosystems with decreasing food availability (Yamaguchi et al., 2002; Kosobokova et al., 2011). Overall, the potential impact of calanoid community ingestion on primary production (PP) in the epipelagic layer (EL) was relatively low (10-21% of PP for non-migrant and 10% of PP for migrant groups). These values are reasonable considering the high primary production due to the influence of the Benguela Current (Bode et al., 2018a) and that, especially in (sub)tropical open-ocean regions, microzooplankton (<200 µm) are major phytoplankton consumers resulting in more complex and potentially less efficient food chains (Dam et al., 1995; Hernández-León et al., 2002; Calbet and Landry, 2004). The importance of smaller-sized calanoids, not only in terms of abundance but also in terms of grazing, and their role in ecosystem functioning has been recognized since the 1990s (Morales et al., 1991; Turner, 2004). However, distribution studies on a high taxonomic level are extremely time-consuming and therefore rare, ultimately limiting our understanding of the carbon pathways and flux dynamics between the epi- and mesopelagic realms, and the role of smaller-sized calanoids in it. It has been estimated that small copepods (<2 mm total length) ingest 1.8-27.2 Gt C y-1 globally, suggesting that this ignored metazoan-copepod link could increase current estimates of biogeochemical fluxes and export to higher trophic levels and cannot be neglected in global models (Roura et al., 2018).
Copepod size has been appointed as a key trait responsible in determining the amount of fecal pellets (FP) that reach the deep sea, with community composition playing a major role in the variability of this flux (Steinberg et al., 2012; Stamieszkin et al., 2015; Steinberg and Landry, 2017). Small FP have reduced aggregation potential and sinking rates, and as such they are more exposed to coprorhexy, coprophagy and/or microbial remineralization processes, reducing sinking FP flux and increasing retention processes (Kiørboe, 2000; Kiørboe, 2001; Iversen and Poulsen, 2007; Steinberg et al., 2012; Stamieszkin et al., 2015). Species >2 mm total length (TL) may already contribute substantially to sinking POC fluxes (Stamieszkin et al., 2015; respective prosome lengths (PL) were converted to TL with a mean copepod PL:TL ratio of 0.8). Hence, copepods of ≤1.3 mm TL mostly contribute to the recycling of carbon and nutrients in their respective depth layer, while fecal pellets of medium-sized species (~1.3-2.0 mm TL) may partly sink out and contribute to export processes. Major consumers in the present study were small species of Paracalanus, Delibus, Ctenocalanus, Mecynocera, Calocalanus (max. mean TL 0.8 mm) and Acartia (max. mean TL 1.2 mm), as well as medium-sized N. minor (max. mean TL 2.1 mm), P. borealis (max. mean TL 2.0 mm) and M. lucens (max. mean TL 2.3 mm). Of these dominant species, the latter three may partly contribute to the vertical POC fluxes in a given layer due to their sizes. P. borealis and M. lucens also transport respiratory and egestive carbon down to mesopelagic layers via DVM, linking the surface layer to the twilight zone (Kelly et al., 2019). Bode et al. 2018a, Bode et al., 2018b described similar results of small copepod species dominating total copepod ingestion in the epipelagic zone in this area.
Epipelagic small-scale regular migrants such as Calocalanus spp. also transport respiratory and egestive carbon from the upper into the lower epipelagic zone, where it can fuel the microbial loop, deep chlorophyll maxima or, in terms of organic carbon, be potentially ingested by other migrant or non-migrant zooplankton. G1i was the group with the largest contribution to the active flux among inverse migrants. Yet, they had a much lower impact on the overall active RC carbon across 50 m (0.2 mg C m-2 d-1) when, compared to regular migrants, with N. gracilis females being the most important taxon. Small (sub)tropical epipelagic calanoids are characterized by constantly high metabolic rates (Kattner and Hagen, 2009; Teuber et al., 2013), thus, they may be continuously feeding, egesting, producing molts etc. High fecal pellet production rates will greatly support the epipelagic and mesopelagic microbial communities (Thor et al., 2003).
“Thrifty floater” taxa such as E. hyalinus, R. cornutus, Pareucalanus spp. (max. mean TL 3.6-6.7 mm) and larger migrants such as P. abdominalis (max. mean TL 3.5 mm) can have a major impact on the export POC fluxes at epi- and mesopelagic depths, as they produce larger, rapidly sinking fecal pellets. The ‘thrifty floater’ species are able to retrieve into oxygen minimum zones (Auel and Verheye, 2007). They may actively reduce their metabolic rates and enter event-driven dormancy at copepodids C5 or adult females, when resource conditions are unfavorable (Teuber et al., 2014; Teuber et al., 2019). These species typically show bimodal vertical distribution patterns, as seen in the present study, and likely contribute to the export via fecal pellet flux throughout the entire water column. In contrast, large DVM species such as P. abdominalis were present only in low abundances in this subtropical oceanic region. Yet, in colder, more productive regions large DVM key species have a high impact on both passive and active carbon fluxes and such play an important role in terms of global biological carbon pump efficiencies (Steinberg and Landry, 2017; Karakuş et al., 2021; Pauli et al., 2021).
In total, the large-scale zone shifters (G2r) alone ingested approximately 6% of surface PP and transferred up to 7.9 mg C m-2 d-1 into the upper mesopelagic zone, representing the most important group regarding the active transfer of carbon into the twilight zone. M. lucens can likely be added to this group (Timonin et al., 1992; Atkinson et al., 1996; Timonin, 1997), but with an even deeper day-time residence depth transporting up to 2.5 mg C m-2 d-1 into the lower mesopelagic zone (Figure 6). Few reports exist of Clausocalanus migrating in large numbers (Roe, 1984; Wishner et al., 2008) and about diel changes of P. borealis (Roe, 1972; Roe, 1984; Andersen et al., 2004). Little is known about the movement of smaller taxa (e.g., Microcalanus) despite, their acknowledged significant ecological and biogeochemical roles (Roura et al., 2018). Besides larger-sized P. abdominalis and P. xiphias, the small congeners of Pleuromamma gracilis undergo similar DVM in the North Pacific subtropical gyre (Ambler and Miller, 1987). Interestingly, active ambush-feeders (carnivores) such as A. armatus and A. giesbrechti were performing iDVM with very small RC flux contributions (<0.01 mg C m-2 d-1). However, reports describing species that display iDVM are scarce (Roe, 1984) and insight into the ecological feedback is still limited (Pinti et al., 2019). Due to the few reports on taxa performing inverse migration and the low biomass found in our study, their contribution to the active transfer of carbon was reasonably small. Avoiding the competition of regular migrants and finding better feeding opportunities appear to be vital drivers of iDVM (Ohman et al., 1983), but this behavior is not yet fully understood. Overall, studies on the species-specific DVM behavior of abundant organisms in the oceans are still lacking and focusing on their traits will have a significant impact on how we model global carbon cycles. New camera systems used to collect distributional data in the field could help to better understand and integrate these different behaviors into Earth’s system models (Giering et al., 2020; Kiko et al., 2020; Maas et al., 2021).
It is not well understood if epi- and mesopelagic smaller-scale migrants are continuously feeding and egesting (Ambler and Miller, 1987). The extent to which migrant copepods defecate their gut contents during non-feeding residence times at depths is still not known. Gut passage times of copepods are highly variable among species and difficult to assess (Morales et al., 1991). For instance, sub-Antarctic M. lucens and Pleuromamma robusta were feeding mainly during the night in upper layers with rather long gut evacuation times leading to potential defecation of some of the food ingested in surface layers at depth (Atkinson et al., 1996). Mean gut passage times of migrating P. xiphias and Euchirella messinensis from the BATS site (Bermuda) were 191 and 114 min., respectively (Schnetzer and Steinberg, 2002). Longer gut evacuation times were observed among large-scale DVM species and are likely due to lower temperatures at depth depressing their metabolism (Atkinson et al., 1996; Schnetzer and Steinberg, 2002). Furthermore, high metabolic rates and continuous food availability may argue for constant feeding of epipelagic active converters (Kattner and Hagen, 2009; Teuber et al., 2019) such as Calocalanus, but for the mesopelagic zone this is still largely unknown. Several species have been observed to adapt their DVM behavior to food availability in highly variable ecosystems such as the southern Benguela upwelling system with calanid copepods remaining in the more productive upper layers for the entire 24 h and stopping DVM, if food availability is low (Huggett et al., 2007). M. tenuicornis, also a calanid copepod, did not perform such small-scale DVM in the oligotrophic North Pacific Central gyre (Ambler and Miller, 1987), exemplifying how much this behavior can vary. Mesopelagic migrants may take advantage of higher food concentrations in the epi- and mesopelagic interface, i.e. increased POC fluxes below the euphotic zone (Buesseler and Boyd, 2009; Sano et al., 2013; Le Mézo and Galbraith, 2021), but may have to feed continuously due to generally lower food availabilities at depth. This may depend on the feeding strategy and trophic preferences of respective species, e.g. predatory cruising vs. detritivorous scavenging.
Both retention and export processes can be greatly enhanced by the activities of diel vertical migrants, essentially “feeding” the mesopelagic realm (Wassmann, 1998; Isla et al., 2015; Gorgues et al., 2019; Stukel et al., 2019; Hernández-León et al, 2020). There are different potential cascading effects depending on how different calanoid species or groups can successively transport carbon even deeper into the ocean’s interior via DVM, following the so-called Vinogradov’s ladder of migration (Vinogradov, 1962; Hernández-León et al, 2020). The different migration depths and occurrence ranges (100-600 m) of decapods (Schukat et al., 2013) and calanoid copepods (this study, Supplementary Figure 2) indicate that Vinogradov’s ladder of migration applies within zooplankton orders. The carbon transport along this ladder has been described for zooplankton biomass in general (Hernández-León et al, 2020), but the processes how carbon is successively passed on are not well understood. In our study, for instance, large non-migrants (e.g. E. hyalinus) produce large fecal pellets in EL which can sink out into and enrich the UML. Here, omnivorous-detritivorous mesopelagic migrants, (e.g. Scottocalanus) may readily feed on this carbon material once ascending to the UML and then returning to the LML for the rest of the day (Sano et al., 2013; Le Mézo and Galbraith, 2021). Small-scale epipelagic inverse and regular migrants of group 1 (G1r: Calocalanus; G1i: M. tenuicornis, N. gracilis) transport carbon ingested at the surface to subsurface layers, where subsurface phytoplankton can be fueled by the CO2 respired or by the nutrients made available via the microbial loop from their fecal pellets (Turner, 2002; Steinberg and Landry, 2017). In G1i, M. tenuicornis (max. mean TL 2.2 mm) and N. gracilis also produce fecal pellets large enough to sink out into the LEL or UML.
In the twilight zone, sinking materials become increasingly important as food source and omnivorous-detritivorous and carnivorous feeding strategies are advantageous (Vinogradov, 1962; Kiørboe, 2000; Kiørboe, 2001; Koppelmann and Frost, 2008; Sano et al., 2013; Bode et al., 2018b; Teuber et al., 2019; Maas et al., 2020). For instance, dissolved organic matter excreted by migrating P. xiphias has been identified to enrich and support growth of the mesopelagic microbial communities (Maas et al., 2020), further creating pathways between the upper and lower layers of the ocean. Although in our study, biomass of iDVM groups was low and therefore, their cascading effects appear weak, inverse mesopelagic migrants (e.g. G3i and G4i) can effectively repackage organic matter from the layer below the epipelagic zone. Much like regular migrants, this can be achieved by transporting carbon further into the ocean’s interior, where it can either be respired, egested (with subsequent recycling or sinking out) or ingested (as prey). They can prey upon regular migrants of G2 calanoids, their fecal pellets or organic matter derived from the microbial loop (Turner, 2002; Iversen and Ploug, 2010; Steedman, 1976). For example, in G3i, medium-sized M. effusa and Scolecithricella spp. (max. mean TL 1.6 mm) produce intermediate-sized fecal pellets, which may partly sink out and partly be immediately remineralized (Thor et al., 2003; Iversen et al., 2010; Steedman, 1976), constituting an important component of the processes maintaining the BCP. In the northern Benguela upwelling system, mesopelagic migrant decapods transported organic carbon ingested in upper mesopelagic layers deeper into lower mesopelagic depths (Schukat et al., 2013). As such, DVM can be a significant gateway for nutrients to reach the upper mesopelagic community, where active input of organic matter can provide enough energy to sustain the deeper-living organisms’ demands (Stukel et al., 2019; Hernández-León et al, 2020).
In the light of current anthropogenic emissions of 36.6 Gt CO2 y-1 (2018; https://de.statista.com), our estimates of copepod active carbon fluxes in the eastern South Atlantic may appear depressingly low. High uncertainties still exist in global carbon export models and their predictions for the future ocean, especially due to high regional and seasonal variability. Our case study in the subtropical South Atlantic emphasizes the complexity of the copepods’ role in the biological carbon pump in subtropical oceanic regions, demonstrating how the identification of different calanoid functional groups may disentangle mechanisms of carbon recycling and export processes. Applying this approach with higher spatial coverage and in regions with higher migrant biomass, will elucidate the different mechanisms, in which carbon is transported along Vinogradov’s ladder of migration into the deep ocean, particularly if considering also other zooplankton key taxa such as salps, euphausiids or decapods. Hence, the pieces of the puzzle still need to be resolved and put together. Identifying key plankton functional types will help us to better quantify and accurately model the mechanisms and pathways involved in the ocean’s carbon cycle.
The datasets presented in this study can be found in online repositories. The names of the repository/repositories and accession number(s) can be found below: https://doi.pangaea.de/10.1594/PANGAEA.946058, PDI-31522.
LDFO sorted and identified the specimens, conducted the morphological and data analyses and wrote the manuscript. MBD supervised the abundance and data analyses and contributed significantly to writing the manuscript. AS supervised the active flux calculations and together with HA and WH contributed to the supervision and finalization of the manuscript. All authors contributed to the article and approved the submitted version.
We would like to thank the captain and crew of RV Polarstern during ANT-XXIX/1 for their skillfull support during the cruise. Thank you to the students of the EUROPA - “European Universities & Research Onboard Polarstern in the Atlantic” training cruise for their support during net sampling.
The authors declare that the research was conducted in the absence of any commercial or financial relationships that could be constructed as a potential conflict of interest.
All claims expressed in this article are solely those of the authors and do not necessarily represent those of their affiliated organizations, or those of the publisher, the editors and the reviewers. Any product that may be evaluated in this article, or claim that may be made by its manufacturer, is not guaranteed or endorsed by the publisher.
The Supplementary Material for this article can be found online at: https://www.frontiersin.org/articles/10.3389/fmars.2022.920483/full#supplementary-material
Suppplementary Figure 1 | Biomass distribution of species with undetermined diel vertical migration behavior. Absolute total biomass values (mg DM m-2) are given for day (grey bars) and night (black bars) for each taxon. Weighted mean depths (m) for day and night of each group are indicated.
Suppplementary Figure 2 | Average day (open circles) and night (closed circles) weighted mean depths of calanoid functional groups. Range of migrations among groupings demonstrate Vinogradov’s ladder of migrations, with overlapping communities transporting and potentially exchanging resources into deeper waters from surface layers.
Al-Mutairi H., Landry M. R. (2001). Active export of carbon and nitrogen at station ALOHA by diel migrant zooplankton. Deep-Sea. Res. II. 48, 2083–2103. doi: 10.1016/S0967-0645(00)00174-0
Ambler J. W., Miller C. B. (1987). Vertical habitat-partitioning by copepodites and adults of subtropical oceanic copepods. Mar. Biol. 94, 561–577. doi: 10.1007/BF00431403
Andersen V., Devey C., Gubanova A., Picheral M., Melnikov V., Tsarin S., et al. (2004). Vertical distributions of zooplankton across the Almeria–Oran frontal zone (Mediterranean Sea). J. Plankton. Res. 26, 275–293. doi: 10.1093/plankt/fbh036
Andersen V., Gubanova A., Nival P., Ruellet T. (2001). Zooplankton community during the transition from spring bloom to oligotrophy in the open NW Mediterranean and effects of wind events. 2. Vertical distributions and migrations. J. Plankton. Res. 23, 243–261. doi: 10.1093/plankt/23.3.243
Archibald K. M., Siegel D. A., Doney S. C. (2019b). Modeling the impact of zooplankton diel vertical migration on the carbon export flux of the biological pump. Glob. Biogeochem. Cycles. 33, 181–199. doi: 10.1029/2018GB005983
Ariza A., Garijo J. C., Landeira J. M., Bordes F., Hernández-León S. (2015). Migrant biomass and respiratory carbon flux by zooplankton and micronekton in the subtropical northeast Atlantic Ocean (Canary Islands). Prog. Oceanogr. 134, 330–342. doi: 10.1016/j.pocean.2015.03.003
Armengol L., Calbet A., Franchy G., Rodríguez-Santos A., Hernández-León S. (2019). Planktonic food web structure and trophic transfer efficiency along a productivity gradient in the tropical and subtropical Atlantic Ocean. Sci. Rep. 9, 1–19. doi: 10.1038/s41598-019-38507-9
Atkinson A., Ward P., Murphy E. J. (1996). Diel periodicity of subantarctic copepods: relationships between vertical migration, gut fullness and gut evacuation rate. J. Plankton. Res. 18, 1387–1405. doi: 10.1093/plankt/18.8.1387
Auel H., Verheye H. M. (2007). Hypoxia tolerance in the copepod Calanoides carinatus and the effect of an intermediate oxygen minimum layer on copepod vertical distribution in the northern Benguela current upwelling system and the Angola–Benguela front. J. Exp. Mar. Biol. Ecol. 352, 234–243. doi: 10.1016/j.jembe.2007.07.020
Bode M., Hagen W., Cornils A., Kaiser P., Auel H. (2018a). Copepod distribution and biodiversity patterns from the surface to the deep sea along a latitudinal transect in the eastern Atlantic Ocean (24°N to 21°S). Prog. Oceanogr. 161, 66–77. doi: 10.1016/j.pocean.2018.01.010
Bode M., Hagen W., Schukat A., Teuber L., Fonseca-Batista D., Dehairs F., et al. (2015). Feeding strategies of tropical and subtropical calanoid copepods throughout the eastern Atlantic Ocean – latitudinal and bathymetric aspects. Prog. Oceanogr. 138, 268–282. doi: 10.1016/j.pocean.2015.10.002
Bode M., Koppelmann R., Teuber L., Hagen W., Auel H. (2018b). Carbon budgets of mesozooplankton copepod communities in the eastern Atlantic Ocean-regional and vertical patterns between 24°N and 21°S. Glob. Biogeochem. Cycles. 32, 840–857. doi: 10.1029/2017GB005807
Bode M., Schukat A., Hagen W., Auel H. (2013). Predicting metabolic rates of calanoid copepods. J. Exp. Mar. Biol. Ecol. 444, 1–7. doi: 10.1016/j.jembe.2013.03.003
Bosch H. F., Rowland Taylor R. (1973). Diurnal vertical migration of an estuarine cladoceran Podon polyphemoides, in the Chesapeake Bay. Mar. Biol. 19, 172–181. doi: 10.1007/BF00353589
Bradford-Grieve J. M., Markhaseva E., Rocha C., Abiahy B., Boltovskoy D. (1999). South Atlantic zooplankton. Blackhuys Publ. Leiden Neth. 1705, 869–1098
Bruland K. W., Silver M. W. (1981). Sinking rates of fecal pellets from gelatinous zooplankton (salps, pteropods, doliolids). Mar. Biol. 63, 295–300. doi: 10.1007/BF00395999
Brun P., Stamieszkin K., Visser A. W., Licandro P., Payne M. R., Kiørboe T. (2019). Climate change has altered zooplankton-fuelled carbon export in the North Atlantic. Nat. Ecol. Evol. 3, 416–423. doi: 10.1038/s41559-018-0780-3
Buesseler K. O., Boyd P. W. (2009). Shedding light on processes that control particle export and flux attenuation in the twilight zone of the open ocean. Limnol. Oceanogr. 54, 1210–1232. doi: 10.4319/lo.2009.54.4.1210
Calbet A., Landry M. R. (2004). Phytoplankton growth, microzooplankton grazing, and carbon cycling in marine systems. Limnol. Oceanogr. 49, 51–57. doi: 10.4319/lo.2004.49.1.0051
Cavan E. L., Henson S. A., Belcher A., Sanders R. (2017). Role of zooplankton in determining the efficiency of the biological carbon pump. Biogeosciences 14, 177–186. doi: 10.5194/bg-14-177-2017
Chisholm L., Roff J. (1990). Abundances, growth rates, and production of tropical neritic copepods off Kingston, Jamaica. Mar. Biol. 106, 79–89. doi: 10.1007/BF02114677
Dam H. G., Roman M. R., Youngbluth M. J. (1995). Downward export of respiratory carbon and dissolved inorganic nitrogen by diel-migrant mesozooplankton at the JGOFS Bermuda time-series station. Deep-Sea Res. I. 42, 1187–1197. doi: 10.1016/0967-0637(95)00048-B
De La Rocha C. L., Passow U. (2007). Factors influencing the sinking of POC and the efficiency of the biological carbon pump. Deep-Sea Res. II. 54, 639–658. doi: 10.1016/j.dsr2.2007.01.004
Escribano R., Hidalgo P., Krautz C. (2009). Zooplankton associated with the oxygen minimum zone system in the northern upwelling region of Chile during March 2000. Deep-Sea Res. Part II. 56 (16), 1083–1094. doi: 10.1016/j.dsr2.2008.09.009
Fahrbach E., Rohardt G. (2008). Physical oceanography during POLARSTERN cruise ANT-XXIV/3. PANGAEA. doi: 10.1594/PANGAEA 733414
Falkowski P. G., Barber R. T., Smetacek V. (1998). Biogeochemical controls and feedbacks on ocean primary production. Science 281, 200–206. doi: 10.1126/science.281.5374.200
Fonseca-Batista D., Dehairs F., Riou V., Fripiat F., Elskens M., Deman F., et al. (2017). Nitrogen fixation in the eastern Atlantic reaches similar levels in the southern and northern hemisphere: N2 fixation in the eastern Atlantic. J. Geophys. Res. Oceans. 122, 587–601. doi: 10.1002/2016JC012335
Giering S. L. C., Cavan E. L., Basedow S. L., Briggs N., Burd A. B., Darroch L. J., et al. (2020). Sinking organic particles in the ocean – flux estimates from in situ optical devices. Front. Mar. Sci. 6. doi: 10.3389/fmars.2019.00834
Gnaiger E. (1983). “Calculation of energetic and biochemical equivalents of respiratory oxygen consumption,” in Polarographic oxygen sensors (Berlin, Heidelberg: Springer), 337–345.
Gorgues T., Aumont O., Memery L. (2019). Simulated changes in the particulate carbon export efficiency due to diel vertical migration of zooplankton in the north Atlantic. Geophys. Res. Lett. 46, 5387–5395. doi: 10.1029/2018GL081748
Hannides C. C., Landry M. R., Benitez-Nelson C. R., Styles R. M., Montoya J. P., Karl D. M. (2009). Export stoichiometry and migrant-mediated flux of phosphorus in the north Pacific subtropical gyre. Deep-Sea Res. I Pap. 56, 73–88. doi: 10.1016/j.dsr.2008.08.003
Hays G. C. (1995). Ontogenetic and seasonal variation in the diel vertical migration of the copepods Metridia lucens and Metridia longa. Limnol. Oceanogr. 40, 1461–1465. doi: 10.4319/lo.1995.40.8.1461
Hays G. C., Proctor C. A., John A. W. G., Warner A. J. (1994). Interspecific differences in the diel vertical migration of marine copepods: The implications of size, color, and morphology. Limnol. Oceanogr. 39, 1621–1629. doi: 10.4319/lo.1994.39.7.1621
Henson S. A., Sanders R., Madsen E., Morris P. J., Le Moigne F., Quartly G. D. (2011). A reduced estimate of the strength of the ocean’s biological carbon pump. Geophys. Res. Lett. 38 (4):L04606. doi: 10.1029/2011GL046735
Hernández-León S., Gómez M., Pagazaurtundua M., Portillo-Hahnefeld A., Montero I., Almeida C. (2001). Vertical distribution of zooplankton in Canary Island waters: Implications for export flux. Deep-Sea Res. I. 48, 1071–1092. doi: 10.1016/S0967-0637(00)00074-1
Hernández-León S., Almeida C., Portillo-Hahnefeld A., Gómez M., Rodriguez J., Arístegui J. (2002). Zooplankton biomass and indices of feeding and metabolism in relation to an upwelling filament off Northwest Africa. J. Mar. Res. 60, 327–346. doi: 10.1357/00222400260497516
Hernández-León S., Ikeda T. (2005). A global assessment of mesozooplankton respiration in the ocean. J. Plankton Res., 27(2), pp.153–158.
Hernández-León S., Olivar M. P., Fernández de Puelles M. L., Bode A., Castellón A., López-Pérez C., et al. (2019). Zooplankton and micronekton active flux across the tropical and subtropical Atlantic Ocean. Front. Mar. Sci. 6. doi: 10.3389/fmars.2019.00535
Hernández-León S., Koppelmann R., Fraile-Nuez E., Bode A., Mompeán C., Irigoien X., et al. (2020). Large deep-sea zooplankton biomass mirrors primary production in the global ocean. Nat. Commun. 11 (1), 1–8. doi: 10.1038/s41467-020-19875-7
Hidaka K., Kawaguchi K., Murakami M., Takahashi M. (2001). Downward transport of organic carbon by diel migratory micronekton in the western equatorial Pacific: Its quantitative and qualitative importance. Deep-Sea Res. I. 48, 1923–1939. doi: 10.1016/S0967-0637(01)00003-6
Hidalgo P., Escribano R., Morales C. E. (2005). Ontogenetic vertical distribution and diel migration of the copepod Eucalanus inermis in the oxygen minimum zone off northern Chile (20–21 s). J. Plankton Res. 27 (6), 519–529. doi: 10.1093/plankt/fbi025
Holliland P. B., Ahlbeck I., Westlund E., Hansson S. (2012). Ontogenetic and seasonal changes in diel vertical migration amplitude of the calanoid copepods Eurytemora affinis and Acartia spp. in a coastal area of the northern Baltic proper. J. Plankton Res. 34, 298–307. doi: 10.1093/plankt/fbs001
Homma T., Yamaguchi A., Bower J. R., Imai I. (2011). Vertical changes in abundance, biomass, and community structure of copepods in the northern north Pacific and Bering Sea at 0-3,000m depth, and their role on the vertical flux of surface-produced organic material. Bull. Fish. Sci. Hokkaido. 61, 29–47. http://hdl.handle.net/2115/48638.
Huggett J. A., Richardson A. J., Field J. G. (2007). Comparative ecology of the copepods Calanoides carinatus and Calanus agulhensis, – the influence of temperature and food. Afr. J. Mar. Sci. 29, 473–490. doi: 10.2989/AJMS.2007.29.3.14.344
Ikeda T., Kanno Y., Ozaki K., Shinada A. (2001). Metabolic rates of epipelagic marine copepods as a function of body mass and temperature. Mar. Biol. 139, 587–596. doi: 10.1007/s002270100608
Ikeda T., Motoda S. (1978). Estimated zooplankton production and their ammonia excretion in the Kuroshio and adjacent seas. Fish. Bull. 76, 357–367.
Isla A., Scharek R., Latasa M. (2015). Zooplankton diel vertical migration and contribution to deep active carbon flux in the NW Mediterranean. J. Mar. Syst. 143, 86–97. doi: 10.1016/j.jmarsys.2014.10.017
Iversen M. H., Nowald N., Ploug H., Jackson G. A., Fischer G. (2010). High resolution profiles of vertical particulate organic matter export off Cape Blanc, Mauritania: Degradation processes and ballasting effects. Deep-Sea Res. I. Pap. 57, 771–784. doi: 10.1016/j.dsr.2010.03.007
Iversen M. H., Ploug H. (2010). Ballast minerals and the sinking carbon flux in the ocean: Carbon-specific respiration rates and sinking velocity of marine snow aggregates. Biogeosciences 7, 2613–2624. doi: 10.5194/bg-7-2613-2010
Iversen M. H., Poulsen L. K. (2007). Coprorhexy, coprophagy, and coprochaly in the copepods Calanus helgolandicus, Pseudocalanus elongatus, and Oithona similis. Mar. Ecol. Prog. Ser. 350, 79–89. doi: 10.3354/meps07095
Karakuş O., Völker C., Iversen M., Hagen W., Wolf-Gladrow D., Fach B., et al. (2021). Modeling the impact of macrozooplankton on carbon export production in the Southern Ocean. J. Geophys. Res. Oceans. 126, e2021JC017315. doi: 10.1029/2021JC017315
Kattner G., Hagen W. (2009). “Lipids in marine copepods: latitudinal characteristics and perspective to global warming,” in Lipids in aquatic ecosystems. Eds. Kainz M., Brett M. T., Arts M. T. (New York, NY: Springer), 257–280. doi: 10.1007/978-0-387-89366-2_11
Kelly T. B., Davison P. C., Goericke R., Landry M. R., Ohman M. D., Stukel M. R. (2019). The importance of mesozooplankton diel vertical migration for sustaining a mesopelagic food web. Front. Mar. Sci. 6. doi: 10.3389/fmars.2019.00508
Kiørboe T. (2000). Colonization of marine snow aggregates by invertebrate zooplankton: abundance, scaling, and possible role. Limnol. Oceanogr. 45, 479–484. doi: 10.4319/lo.2000.45.2.0479
Kiørboe T. (2001). Formation and fate of marine snow: small-scale processes with large- scale implications. Sci. Mar. 65, 57–71. doi: 10.3989/scimar.2001.65s257
Kiko R., Brandt P., Christiansen S., Faustmann J., Kriest I., Rodrigues E., et al. (2020). Zooplankton-mediated fluxes in the eastern tropical North Atlantic. Front. Mar. Sci. 7. doi: 10.3389/fmars.2020.00358
Kiko R., Hauss H. (2019). On the estimation of zooplankton-mediated active fluxes in oxygen minimum zone regions. Front. Mar. Sci. 6. doi: 10.3389/fmars.2019.00741
Kobari T., Kitamura M., Minowa M., Isami H., Akamatsu H., Kawakami H., et al. (2013). Impacts of the wintertime mesozooplankton community to downward carbon flux in the subarctic and subtropical Pacific Oceans. Deep-Sea Res. I. 81, 78–88. doi: 10.1016/j.dsr.2013.07.003
Koppelmann R., Frost J. (2008). The ecological role of zooplankton in the twilight and dark zones of the ocean. Biol. Oceanogr. Res. Trends 67–130.
Kosobokova K. N., Hopcroft R. R., Hirche H.-J. (2011). Patterns of zooplankton diversity through the depths of the Arctic’s central basins. Mar. Biodivers. 41, 29–50. doi: 10.1007/s12526-010-0057-9
Kwong L. E., Henschke N., Pakhomov E. A., Everett J. D., Suthers I. M. (2020). Mesozooplankton and micronekton active carbon transport in contrasting eddies. Front. Mar. Sci. 6. doi: 10.3389/fmars.2019.00825
Le Mézo P. K., Galbraith E. D. (2021). The fecal iron pump: global impact of animals on the iron stoichiometry of marine sinking particles. Limnol. Oceanogr. 66, 201–213. doi: 10.1002/lno.11597
Longhurst A. R., Harrison W. G. (1989). The biological pump: profiles of plankton production and consumption in the upper ocean. Prog. Oceanogr. 22, 47–123. doi: 10.1016/0079-6611(89)90010-4
Maas A. E., Gossner H., Smith M. J., Blanco-Bercial L. (2021). Use of optical imaging datasets to assess biogeochemical contributions of the mesozooplankton. J. Plankton. Res. 43, 475–491. doi: 10.1093/plankt/fbab037
Maas A. E., Liu S., Bolaños L. M., Widner B., Parsons R., Kujawinski E. B., et al. (2020). Migratory zooplankton excreta and its influence on prokaryotic communities. Front. Mar. Sci. 7. doi: 10.3389/fmars.2020.573268
Manno C., Stowasser G., Enderlein P., Fielding S., Tarling G. A. (2015). The contribution of zooplankton faecal pellets to deep-carbon transport in the Scotia Sea (Southern Ocean). Biogeosciences 12, 1955–1965. doi: 10.5194/bg-12-1955-2015
Martin B., Eggert A., Koppelmann R., Diekmann R., Mohrholz V., Schmidt M. (2015). Spatio-temporal variability of zooplankton biomass and environmental control in the northern Benguela upwelling system: Field investigations and model simulation. Mar. Ecol. 36 (3), 637–658. doi: 10.1111/maec.12173
Morales C. E., Bedo A., Harris R. P., Tranter P. R. G. (1991). Grazing of copepod assemblages in the northeast Atlantic: The importance of the small size fraction. J. Plankton. Res. 13, 455–472. doi: 10.1093/plankt/13.2.455
Motoda S. (1959). Devices of simple plankton apparatus. Memoirs of the faculty of fisheries Hokkaido University, 7(1–2), 73–94.
Nowicki M., DeVries T., Siegel D. A. (2022). Quantifying the carbon export and sequestration pathways of the ocean's biological carbon pump. Global Biogeochem. Cycles. 36 (3), e2021GB007083. doi: 10.1029/2021GB007083
Ohman M. D., Frost B. W., Cohen E. B. (1983). Reverse diel vertical migration: An escape from invertebrate predators. Science 220, 1404–1407. doi: 10.1126/science.220.4604.1404
Ohman M. D., Romagnan J.-B. (2016). Nonlinear effects of body size and optical attenuation on diel vertical migration by zooplankton. Limnol. Oceanogr. 61, 765–770. doi: 10.1002/lno.10251
Pauli N.-C., Flintrop C. M., Konrad C., Pakhomov E. A., Swoboda S., Koch F., et al. (2021). Krill and salp faecal pellets contribute equally to the carbon flux at the Antarctic Peninsula. Nat. Commun. 12, 1–12. doi: 10.1038/s41467-021-27436-9
Pearre S. (2003). Eat and run? the hunger/satiation hypothesis in vertical migration: history, evidence and consequences. Biol. Rev. 78, 1–79. doi: 10.1017/S146479310200595X
Perissinotto R., Pakhomov E. A. (1998). Contribution of salps to carbon flux of marginal ice zone of the Lazarev Sea, Southern Ocean. Mar. Biol. 131, 25–32. doi: 10.1007/s002270050292
Pillar S. C. (1984). A comparison of the performance of four zooplankton samplers. S. Afr. J. Mar. Sci. 2 (1), 1–18. doi: 10.2989/02577618409504354
Pinti J., Kiørboe T., Thygesen U. H., Visser A. W. (2019). Trophic interactions drive the emergence of diel vertical migration patterns: A game-theoretic model of copepod communities. Proc. R. Soc. B. 286, 20191645. doi: 10.1098/rspb.2019.1645
Razouls C., Kouwenberg J., Desreumaux N., de Bovée F. (2005). Diversity and geographic distribution of marine planktonic copepods (Accessed June 30, 2019).
Roe H. S. J. (1972). The vertical distribution and diurnal migrations of calanoid copepods collected on the Sond cruise, 1965 IV. Systematic account of families Lucicutiidae to the Candaciidae. The relative abundance of the numerically most important genera. J. Mar. Biol. Assoc. U. K. 52, 1021–1044. doi: 10.1017/S002531540004073X
Roe H. S. J. (1984). The diel migrations and distributions within a mesopelagic community in the north east Atlantic. 4 the copepods. Prog. Oceanogr. 13, 353–388. doi: 10.1016/0079-6611(84)90013-2
Roura Á., Strugnell J. M., Guerra Á., González Á. F., Richardson A. J. (2018). Small copepods could channel missing carbon through metazoan predation. Ecol. Evol. 8 (22), 10868–10878. doi: 10.1002/ece3.4546
Sano M., Maki K., Nishibe Y., Nagata T., Nishida S. (2013). Feeding habits of mesopelagic copepods in Sagami Bay: Insights from integrative analysis. Prog. Oceanogr. 110, 11–26. doi: 10.1016/j.pocean.2013.01.004
Schnack-Schiel S. B., Mizdalski E., Cornils A. (2010). Copepod abundance and species composition in the eastern subtropical/tropical Atlantic. Deep-Sea Res. II. 57, 2064–2075. doi: 10.1016/j.dsr2.2010.09.010
Schnetzer A., Steinberg D. K. (2002). Active transport of particulate organic carbon and nitrogen by vertically migrating zooplankton in the Sargasso Sea. Mar. Ecol. Prog. Ser. 234, 71–84. doi: 10.3354/meps234071
Schukat A., Hagen W., Dorschner S., Acosta J. C., Arteaga E. L. P., Ayón P., et al. (2021). Zooplankton ecological traits maximize the trophic transfer efficiency of the Humboldt Current upwelling system. Prog. Oceanogr. 193, 102551. doi: 10.1016/j.pocean.2021.102551
Schukat A., Teuber L., Hagen W., Wasmund N., Auel H. (2013). Energetics and carbon budgets of dominant calanoid copepods in the northern Benguela upwelling system. J. Exp. Mar. Biol. Ecol. 442, 1–9. doi: 10.1016/j.jembe.2013.01.024
Sigman D. M., Hain M. P. (2012). The biological productivity of the ocean. Nat. Educ. Knowledge 3 (6), 1–16.
Soviadan Y. D., Benedetti F., Brandão M. C., Ayata S.-D., Irisson J.-O., Jamet J. L., et al. (2021). Patterns of mesozooplankton community composition and vertical fluxes in the global ocean. Prog. Oceanogr. 200, 102717. doi: 10.1016/j.pocean.2021.102717
Stamieszkin K., Pershing A. J., Record N. R., Pilskaln C. H., Dam H. G., Feinberg L. R. (2015). Size as the master trait in modeled copepod fecal pellet carbon flux. Limnol. Oceanogr. 60 (6), 2090–2107. doi: 10.1002/lno.10156
Steedman H. F. (1976). General and applied data on formaldehyde fixation and preservation of marine zooplankton. Zooplankton fixation and priservation. Monographs on oceanographic methodology. The Unesco Press, 4, 103–154.
Steinberg D. K., Carlson C. A., Bates N. R., Goldthwait S. A., Madin L. P., Michaels A. F. (2000). Zooplankton vertical migration and the active transport of dissolved organic and inorganic carbon in the Sargasso Sea. Deep-Sea Res. I. 47, 137–158. doi: 10.1016/S0967-0637(99)00052-7
Steinberg D. K., Cope J. S., Wilson S. E., Kobari T. (2008). A comparison of mesopelagic mesozooplankton community structure in the subtropical and subarctic north Pacific Ocean. Deep-Sea Res. II. 55, 1615–1635. doi: 10.1016/j.dsr2.2008.04.025
Steinberg D. K., Landry M. R. (2017). Zooplankton and the ocean carbon cycle. Annu. Rev. Mar. Sci. 9, 413–444. doi: 10.1146/annurev-marine-010814-015924
Steinberg D. K., Lomas M. W., Cope J. S. (2012). Long-term increase in mesozooplankton biomass in the Sargasso Sea: Linkage to climate and implications for food web dynamics and biogeochemical cycling. Glob. Biogeochem. Cycles. 26, GB1004. doi: 10.1029/2010GB004026
Stukel M. R., Ohman M. D., Benitez-Nelson C. R., Landry M. R. (2013). Contributions of mesozooplankton to vertical carbon export in a coastal upwelling system. Mar. Ecol. Prog. Ser. 491, 47–65. doi: 10.3354/meps10453
Stukel M. R., Ohman M. D., Kelly T. B., Biard T. (2019). The roles of suspension-feeding and flux-feeding zooplankton as gatekeepers of particle flux into the mesopelagic ocean in the northeast Pacific. Front. Mar. Sci. 6, 397. doi: 10.3389/fmars.2019.00397
Suess E. (1980). Particulate organic carbon flux in the oceans – surface productivity and oxygen utilization. Nature 288, 260–263. doi: 10.1038/288260a0
Teuber L., Hagen W., Bode M., Auel H. (2019). Who is who in the tropical Atlantic? Functional traits, ecophysiological adaptations and life strategies in tropical calanoid copepods. Prog. Oceanogr. 171, 128–135. doi: 10.1016/j.pocean.2018.12.006
Teuber L., Schukat A., Hagen W., Auel H. (2013). Distribution and ecophysiology of calanoid copepods in relation to the oxygen minimum zone in the eastern tropical Atlantic. PloS One 8, e77590. doi: 10.1371/journal.pone.0077590
Teuber L., Schukat A., Hagen W., Auel H. (2014). Trophic interactions and life strategies of epi-to bathypelagic calanoid copepods in the tropical Atlantic Ocean. J. Plankton. Res. 36, 1109–1123. doi: 10.1093/plankt/fbu030
Thor P., Dam H. G., Rogers D. R. (2003). Fate of organic carbon released from decomposing copepod fecal pellets in relation to bacterial production and ectoenzymatic activity. Aquat. Microb. Ecol. 33, 279–288. doi: 10.3354/ame033279
Timonin A. G. (1997). Diel vertical migrations of Calanoides carinatus and Metridia lucens (Copepoda: Calanoida) in the northern Benguela upwelling area. Oceanology 37, 782–787.
Timonin A. G., Arashkevich E. G., Drits A. V., Semenova T. N. (1992). Zooplankton dynamics in the northern Benguela ecosystem, with special reference to the copepod Calanoides carinatus. South Afr. J. Mar. Sci. 12, 545–560. doi: 10.2989/02577619209504724
Turner J. (2002). Zooplankton fecal pellets, marine snow and sinking phytoplankton blooms. Aquat. Microb. Ecol. 27, 57–102. doi: 10.3354/ame027057
Turner J. T. (2004). The importance of small planktonic copepods and their roles in pelagic marine food webs. Zool. Stud. 43, 255–266.
Turner J. T. (2015). Zooplankton fecal pellets, marine snow, phytodetritus and the ocean’s biological pump. Prog. Oceanogr. 130, 205–248. doi: 10.1016/j.pocean.2014.08.005
Tutasi P., Escribano R. (2020). Zooplankton diel vertical migration and downward C flux into the oxygen minimum zone in the highly productive upwelling region off northern Chile. Biogeosciences 17, 455–473. doi: 10.5194/bg-17-455-2020
van der Jagt H., Wiedmann I., Hildebrandt N., Niehoff B., Iversen M.H. (2020). Aggregate feeding by the copepods Calanus and Pseudocalanus controls carbon flux attenuation in the Arctic shelf sea during the productive period. Front. Mar. Sci. 7, p.543124.
Vinogradov M. E. (1962). Feeding of the deep-sea zooplankton. Rapp. Rv. Réun. Cons. Perm. Int. Explor. Mer., 153, 114–120.
Wassmann P. (1998). “Retention versus export food chains: processes controlling sinking loss from marine pelagic systems,” in Eutrophication in planktonic ecosystems: Food web dynamics and elemental cycling. Eds. Tamminen T., Kuosa H. (Dordrecht: Springer Netherlands), 29–57. doi: 10.1007/978-94-017-1493-8_3
Wassmann P., Olli K., Riser C. W., Svensen C. (2003). “Ecosystem function, biodiversity and vertical flux regulation in the twilight zone,” in Marine science frontiers for Europe. Eds. Wefer G., Lamy F., Mantoura F. (Berlin, Heidelberg, Springer), 279–287. doi: 10.1007/978-3-642-55862-7_19
Winberg G. (1956). Rate of metabolism and food requirements of fish. Fish. Res. Bd. Can. Transl. Ser. 194, 1–253.
Wishner K. F., Gelfman C., Gowing M. M., Outram D. M., Rapien M., Williams R. L. (2008). Vertical zonation and distributions of calanoid copepods through the lower oxycline of the Arabian Sea oxygen minimum zone. Prog. Oceanogr. 78, 163–191. doi: 10.1016/j.pocean.2008.03.001
Woodd-Walker R. S. (2001). Spatial distributions of copepod genera along the Atlantic meridional transect. Hydrobiologia 453 (1), 161–170. doi: 10.1023/A:1013140606293
Woodd-Walker R., Ward P., Clarke A. (2002). Large-scale patterns in diversity and community structure of surface water copepods from the Atlantic Ocean. Mar. Ecol. Prog. Ser. 236, 189–203. doi: 10.3354/meps236189
Yamaguchi A., Watanabe Y., Ishida H., Harimoto T., Furusawa K., Suzuki S., et al. (2002). Community and trophic structures of pelagic copepods down to greater depths in the western subarctic Pacific (WEST-COSMIC). Deep-Sea Res. I. 49, 1007–1025. doi: 10.1016/S0967-0637(02)00008-0
Yebra L., Almeida C., Hernández-León S. (2005). Vertical distribution of zooplankton and active flux across an anti-cyclonic eddy in the Canary Island waters. Deep-Sea Res. I. 52, 69–83. doi: 10.1016/j.dsr.2004.08.010
Keywords: diel vertical migration (DVM), carbon cycle, active carbon flux, zooplankton ingestion, zooplankton respiration, biological carbon pump, zooplankton functional groups, twilight zone
Citation: Oliveira LDFd, Bode-Dalby M, Schukat A, Auel H and Hagen W (2022) Cascading effects of calanoid copepod functional groups on the biological carbon pump in the subtropical South Atlantic. Front. Mar. Sci. 9:920483. doi: 10.3389/fmars.2022.920483
Received: 14 April 2022; Accepted: 19 July 2022;
Published: 26 August 2022.
Edited by:
Amy Elizabeth Maas, Bermuda Institute of Ocean Sciences, BermudaReviewed by:
Lian Elizabeth Kwong, University of British Columbia, CanadaCopyright © 2022 Oliveira, Bode-Dalby, Schukat, Auel and Hagen. This is an open-access article distributed under the terms of the Creative Commons Attribution License (CC BY). The use, distribution or reproduction in other forums is permitted, provided the original author(s) and the copyright owner(s) are credited and that the original publication in this journal is cited, in accordance with accepted academic practice. No use, distribution or reproduction is permitted which does not comply with these terms.
*Correspondence: Maya Bode-Dalby, bWFib2RlQHVuaS1icmVtZW4uZGU=; Lívia Dias Fernandes de Oliveira, bGl2aWEub2xpdmVpcmFAc3RyYXRoLmFjLnVr
†These authors have contributed equally to this work
Disclaimer: All claims expressed in this article are solely those of the authors and do not necessarily represent those of their affiliated organizations, or those of the publisher, the editors and the reviewers. Any product that may be evaluated in this article or claim that may be made by its manufacturer is not guaranteed or endorsed by the publisher.
Research integrity at Frontiers
Learn more about the work of our research integrity team to safeguard the quality of each article we publish.