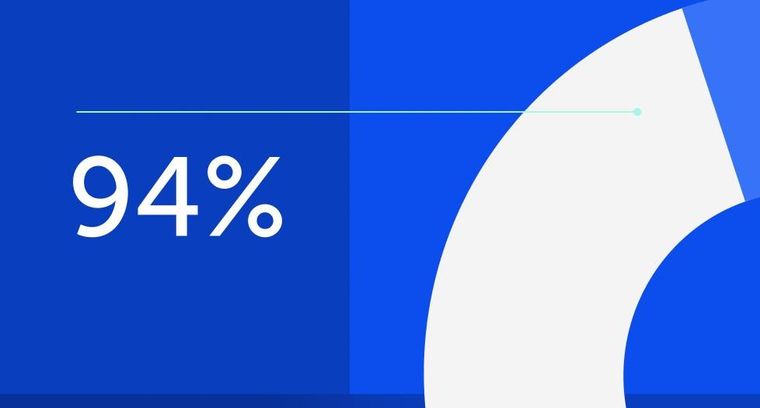
94% of researchers rate our articles as excellent or good
Learn more about the work of our research integrity team to safeguard the quality of each article we publish.
Find out more
REVIEW article
Front. Mar. Sci., 28 July 2022
Sec. Marine Biogeochemistry
Volume 9 - 2022 | https://doi.org/10.3389/fmars.2022.920405
This article is part of the Research TopicBiotechnological Solutions to Assess, Monitor and Remediate Metal Pollution in the Marine Environment.View all 7 articles
Rare earth elements (REEs) or lanthanides are often found together in nature, and they are used in multiple anthropogenic activities from green energy and medical technologies to telecommunications and defense systems. However, the current understanding on the concentration and behavior of REEs in oceans and marine organisms is limited, and no regulatory information or limits have been settled. Here, we present a review of the concentrations of lanthanides in marine waters and biota. REEs reach aquatic ecosystems mainly by continental contributions, and the maximum reported concentrations of REEs are found on the platform surface near the coast due to their continental origin. For coastal waters, we find maximum REE levels in the surface water that decrease with depth until a certain stability. Their concentrations diminish as they move toward the open ocean, where concentrations tend to increase vertically with depth in the water column. Only cerium (Ce) showed different patterns from other REEs caused by Ce different redox states: III and IV, reflecting the oxidation of dissolved Ce (III) to particulate Ce (IV) when reacting with the O2 to form CeO2. In seawater, heavy REEs tend to remain in solution forming complexes usually unavailable for organisms, while light REEs are most likely to be assimilated by them, posing potential biological implications. Bioaccumulation of REEs decreases as marine trophic level increases, showing a trophic dilution pattern. Generally, higher concentrations are found in organisms such as phytoplankton, zooplankton, and algae species, while the lowest concentrations are found in mollusks, corals, and fish species. According to the current trend in the REE industry, the increasing anthropogenic emissions are a fact; therefore, more studies will be needed regarding their fractionation, the transformation processes with which they become bioavailable, and their pathways in marine systems.
The lanthanides (Group VI of the Periodic Table of Elements), lanthanum (La), cerium (Ce), praseodymium (Pr), neodymium (Nd), promethium (Pm; synthetic, made in the laboratory), samarium (Sm), europium (Eu), gadolinium (Gd), terbium (Tb), dysprosium (Dy), holmium (Ho), erbium (Er), thulium (Tm), ytterbium (Yb), and lutetium (Lu) and the two transition elements scandium (Sc) and yttrium (Y), are classified as rare earth elements (hereinafter REEs) for their similar physicochemical properties and their economic importance (Fisher and Kara, 2016) as well as for being usually found together in the same ore deposits in nature. These elements can be divided essentially into three subgroups depending on their atomic numbers: the light REEs (LREEs), with the lowest atomic number (from La to Pm), the medium REEs (MREEs) (generally from Sm to Gd), and the heavy REEs (HREEs) (from Tb to Lu, as well as Sc and Y). Also frequently, their division is limited only to two classifications, LREE and HREE groups (Zepf, 2013). The term “rare” is not due to their low abundance, since many of the REEs are more widespread in the Earth’s crust than other elements commonly used in our daily life but because of the difficulty of separating the different elements and the lack of economically exploitable ore deposits, which are concentrated mainly in China (>90%) (Dushyantha et al., 2020). Within the REE group, 14 elements corresponding to the lanthanides (excluding Pm and Sc) are considered technology-critical elements (TCEs) (Cobelo-García and Filella, 2017), given their importance in emerging technologies. Those are the elements described in the following review.
During the following review, we will break down the available information, to date, on the concentrations of the REEs in marine ecosystems, specifically in the field-collected water column and organisms. Data on REEs in marine ecosystems will be surveyed and gathered from existing scientific literature from a search by keywords (REE/lanthanides AND marine environment/biota/water) in the Web of Science database. Based on the data collected, we will provide an evaluation of the current gaps in knowledge on these elements in marine systems and we will indicate directions for future research. In the next sections, only the REEs belonging to the TCEs will be further discussed but discarding the transition element Y.
In nature, REEs do not exist as individual native metals but are found in a wide range of minerals (Balaram, 2019), and their presence is generally associated with alkaline magmas such as peralkaline silicate rocks and carbonatites (Chakhmouradian and Wall, 2012). Usually, data on different REEs are represented together, as they appear in the environment. REE representation follows a zigzag pattern (effect of Oddo–Harkins rule), being Ce the element with the highest content in the group. Thus, to interpret data and find specific anomalies, they are frequently normalized using both abiotic and biotic samples, with the REE content in natural shale crust as reference. To date, different shale compositions are commonly used, such as the European Shale (EUS) published in the 1930s (but improved in the 1960s), the North American Shale Composite (NASC) from the 1960s, and the Post-Archean Australian Shale (PAAS) from the 1970s, with an average content of total REEs (including Y but discarding Pm) of 246, 194, and 211 mg/kg, respectively (Bau et al., 2018). Even though they were not studied further in the past, like other elements, the reported contents of different REEs are significantly higher than those of the most common studied metals, e.g., Ce with an average content of 63 mg/kg in the continental crust that is 2-fold more abundant than Cu (Rudnick and Gao, 2003).
The use of REEs in industry, medicine, and agriculture has increased exponentially during the last decades (Geagea et al., 2007). Therefore, a parallel increase in their demand and production is expected. The total worldwide production was 81 kt/year in 2000 and 126 kt/year in 2016, while it increased to 280 kt/year in 2021 (an increase of more than 100% in the worldwide production in only 5 years) (Survey USG, 2022). REEs are key components of modern life, as they are part of basic high-tech applications and green technologies (Wen et al., 2006). Some examples of the most important technological applications of REEs are energy-efficient lighting, permanent magnets for wind turbines, batteries for hybrid and electric vehicles, digital cameras, optical glasses, catalysts, or metal alloys (Brioschi et al., 2013). Among them, the LREEs (La, Ce, Pr, and Nd) are the most commonly used group (Massari and Ruberti, 2013). Moreover, REEs are also used in phosphate-based fertilizers for soil amendment (Zhang and Shan, 2001), which, like other fertilizer compounds, would finish in aquatic systems. Their application in fertilizers, bactericides, supplements for animal husbandry, and aquaculture food has been widely extended in China since the early 1970s (Sun et al., 1994; Fang et al., 2007) with questionable short-term success for plant and animal growth and constituting an important route of entry of anthropogenic REEs into the environment and the trophic chain (Gwenzi et al., 2018). Another important use of REEs is in geology as tracers of geochemical processes (Bau and Dulski, 1996; Olías et al., 2018). Despite their coherent behavior as a group, the geochemical processes may affect the REE distribution depending on their atomic number (Elderfield et al., 1990). Thus, REEs will show fractionation depending on the chemical circumstances of the environment (Akagi et al., 2004). In addition, the analysis of the REE series allows, among other utilities, to infer the past conditions and rebuild biogeochemical cycles or water masses’ origin.
In recent years, elevated REE levels have been widely reported in different natural systems, e.g., Ce distinctive positive anomalies mainly in the warm surface layer of the Black Sea (Schijf and de Baar, 1995). In aquatic systems, the most frequently detected anthropogenic REE is Gd, which has been particularly studied since the mid-1990s when it was for the first time reported anthropogenic influence in the Gd natural distribution (Nozaki et al., 2000; Elbaz-Poulichet et al., 2002; Bau et al., 2006; Kulaksiz and Bau, 2007; Lawrence, 2010; Kulaksiz and Bau, 2011; Kulaksiz and Bau, 2013; Hatje et al., 2014; Tepe et al., 2014; Merschel et al., 2015). The observation of Gd anomalies is highly correlated with the use of this metal in contrasting agents for magnetic resonance imaging (MRI), especially in densely populated areas with developed medical and healthcare systems (Bau and Dulski, 1996; Nozaki et al., 2000; Bau et al., 2006; Kulaksiz and Bau, 2007; Kulaksiz and Bau, 2011). Positive anthropogenic Gd anomalies can be used as a pseudo-natural tracer of water discharge from wastewater treatment plants with emerging microcontaminants since Gd cannot be removed by common sewage treatment technologies (Bau et al., 2006; Kulaksiz and Bau, 2007; Lawrence et al., 2009; Lawrence, 2010; Tepe et al., 2014). In addition to Gd, other anthropogenic REEs, such as La and Sm, are also found in industrial wastewater and rivers from catalyst production for petroleum refining (Kulaksiz and Bau, 2013). According to the current scientific interest in REEs, an increase in the use of REEs as tracer elements for monitoring their natural and anthropogenic processes in water systems is expected, which will serve to enhance our understanding of REE inputs and their behavior in marine systems.
The composition of seawater is determined by the rate of supply and removal of elements from the oceans. In oceans, REEs come mainly from continental weathering, being transported through rivers (Figure 1). The riverine REE transport is strongly controlled by particulate matter; higher concentrations are commonly found in the particulate matter transported by rivers than dissolved, with around 30 Gt/year of suspended sediments being transported to the oceans (Pearce et al., 2013). Thus, the impact of the continental supply of REEs from rivers to the oceans depends strongly on the fate of the particulate and colloidal materials during river and seawater mixing, since they are the major component of the riverine REE flux (Elderfield et al., 1990). The REE patterns for the majority of rivers span a wide range depending primarily on local geology, with, in general, enrichment in HREEs in the dissolved phase (Goldstein and Jacobsen, 1988; Elderfield et al., 1990; Nozaki et al., 2000). The fractionation of REEs compared with the continental crust reflects the geochemical processes that occurred during their transport, with physicochemical parameters [e.g., pH, redox (Eh) conditions, and complexation with organic and inorganic ligands] being the most important factors for establishing the REE composition in rivers (Goldstein and Jacobsen, 1988; Elderfield et al., 1990; Olías et al., 2018; Cánovas et al., 2021; Jalali and Lebeau, 2021).
Figure 1 Sources, transport, and sink pathways of lanthanides in seawater, including sediment–seawater exchange and biota interaction.
In aqueous solution, REEs predominantly exist in the trivalent oxidation state (3+) (Elderfield and Greaves, 1982), the only exceptions are Ce (4+) and Eu (2+) (Olías et al., 2005; Olías et al., 2018), although Eu can be reduced (2+) or oxidized (4+) depending on the media conditions (Hatje et al., 2014). The concentration of REEs in rivers is pH-dependent, with usually lower concentrations at higher pH values and more fractionation of the elements with a major abundance of HREEs (Sholkovitz, 1995). On the contrary, acidic pH values lead to increasing concentrations of REE, such as those observed in acid mine drainage environments, with concentrations several orders of magnitude higher than those in natural waters (e.g., Noack et al., 2014; Cánovas et al., 2021). Generally, riverine inputs of REEs to the oceans can be classified as follows: 1) suspended terrigenous particles that usually present an LREE-enriched relative to shale; 2) colloidal material predominant in most river waters, with shale-like pattern although slightly enriched in MREE; and 3) dissolved REEs that account for a minor contribution and whose fractionation is strongly controlled by the filtration strategy (Elderfield et al., 1990). It is generally considered that filtration below 0.22 µm removes notably the presence of suspended particles and colloids. Another source of REE from the continents to the oceans is aeolian dust (Figure 1), which may be transported and solubilized in contact with seawater. However, the fluxes of REE associated with riverine inputs and aeolian dust are not accurately known due to intense removal and remobilization processes in estuaries and unknown dissolution rates of REEs in contact with seawater, respectively (van de Flierdt et al., 2004). There is also an important vertical flux supply in the open ocean to surface waters dependent on upper ocean stratification that inhibits or limits vertical mixing that could be two orders of maginitude larger than terrestrial (riverine/dust) inputs (Chen et al., 2013).
Different processes take place along the continent–ocean interface, known as boundary exchanging, which leads to changes in REE abundances, speciation, and normalized patterns from their terrigenous source to open oceans. In this interface, estuaries play a major role in acting as biogeochemical reactors where riverine fluxes of inorganic and organic matter affect the transfer of elements to the oceans (Andrade et al., 2020). A general removal of dissolved REEs is observed during the mixing of river water with seawater at low salinities due mainly to the salt-induced coagulation of Fe-rich organic colloids (e.g., Elderfield et al., 1990; Sholkovitz, 1995). Much effort has been put into characterizing the particulate REEs formed by estuarine removal; however, the products of estuarine mixing processes are commonly masked by terrigenous material, transported by the rivers, like in the case of Fe (Elderfield et al., 1990). This removal of dissolved REEs also coincides with the fractionation of REEs enhanced by a change in speciation. Thus, HREEs are predominantly bound by stable carbonate complexes, while LREEs exhibit a greater proportion of free metal ions and tend to be more reactive than the MREEs, especially by sorption processes (Sholkovitz, 1995). This fractionation of dissolved REEs during estuarine mixing leads to the characteristic HREE enrichment NASC-normalized seawater (e.g., Alibo and Nozaki, 1999; Crocket et al., 2018), although some processes can modify this fractionation pattern. For instance, high pH values and complexation with organic ligands may inhibit this fractionation (Adebayo et al., 2018). On the other hand, the opposite process is sometimes observed at high salinities in estuaries; REE is released back into the water column from sediments and particulate matter (e.g., Andrade et al., 2020). As reported by Andrade et al. (2020) at the Paraguaçu estuary (Brazil), these desorptive and diffusive processes may also alter the REE fractionation according to the enrichment in MREEs and LREEs over HREEs, opposite to that observed at low salinities.
As the REEs are transported to the oceans via estuaries, the coastal waters exhibit intermediate concentrations and patterns between those of river and ocean waters (Elderfield et al., 1990). Once these elements come out from estuaries, the combination of lateral mixing and gradients in particle fluxes predominates, leading to enhanced deposition and fractionation of particle-reactive elements and the boundary exchanging (particle-dissolved processes) from continental margins to the open sea (Lacan and Jeandel, 2005). In this sense, sediment–seawater interaction during boundary exchange on continental margins (Figure 1) can be a major control on REE composition of oceans, with differences observed worldwide depending on the particulate characteristics and distance from the continental margin, being most important in nearshore environments (Pearce et al., 2013).
The higher pH and water residence times in oceans render REEs less concentrated and more fractionated than those in rivers (Sholkovitz, 1995). The distribution of dissolved REEs along the water column of oceanic waters has also been well studied. Vertical profiles indicate that REE concentrations increase with depth (except for Ce), although this increase is not similar for all REEs; whereas LREEs increase almost linearly with depth, HREEs exhibit more nutrient-like profiles (Nozaki et al., 2000). This distribution of REEs along the water column has been traditionally explained by the dominance of vertical processes affecting the input, scavenging, and release of REEs from particles to the water column. Based on studies about Nd isotopes, Siddall et al. (2008) reported that such REE distribution could be explained by a combination of lateral advection and reversible scavenging (sorption and desorption from particles). However, recent studies also point to biological drivers of REE solubility control in oceans. The dissolution of diatoms may be a major pathway of REE transport to deeper layers (Figure 1), as observed in the water column of the North Pacific Ocean, characterized by high diatom productivity (Akagi, 2013). These authors reported that the concentration of dissolved silica in surface water was close to zero, while REE concentrations were notably reduced. This finding highlights the role of diatoms in the solubility control of REEs in surface oceanic waters. In addition, spatial differences in REE concentrations are observed in oceanic waters, with an overall increase from the North Atlantic to the North Pacific (van de Flierdt et al., 2004; Siddall et al., 2008) depending on the water age and residence time. However, other different sources and processes may alter the distribution and fractionation of REEs in oceans. For instance, Schijf et al. (2015) suggested that manganese oxides may be the dominant scavenger of REEs throughout the abyssal ocean and perhaps even in the upper water column but also supported the need for more sophisticated kinetic models for the redox-driven scavenging of Ce (III). On the other hand, pore fluids from bottom sediments have been previously hypothesized as an REE source in deep waters in some areas of the ocean (Abbott et al., 2015). This finding would support the importance of sediments and pore waters as an oceanic REE source, as recently discussed by Haley et al. (2017). Abbott et al. (2019) move a step forward pointing to authigenic clays as the main mineral phase responsible for benthic REE fluxes. According to these authors, the high correlations previously reported in the literature between Fe and REEs in marine sediments would be not related to REE sorption onto ferromanganese oxides but to Fe-rich clay minerals like glauconite. On the other hand, hydrothermal plumes can also act as sources and sinks of REEs in oceans (Figure 1). In this sense, it has been reported that hydrothermal plumes do not contribute significantly to the dissolved seawater REE budget due to a fast REE removal at the vent site (Siddall et al., 2008). These processes have been even quantified by other researchers. For example, Stichel et al. (2018) reported a significant Nd depletion in hydrothermal plumes equivalent to around 70% of the combined river and dust inputs according to these authors (6%–8% of the global REE flux). Nevertheless, different attempts to quantify REE fluxes based on a mass balance approach of Nd isotopes, a conservative tracer in seawater, identified a missing source of Nd (and REE) that could be due to volcanic arcs (Siddall et al., 2008). Considering that volcanic ashes are much more easily dissolved than materials coming from continental margins (Taylor and McLennan, 1985), volcanic arcs (Figure 1) may play a major role in the distribution of REEs in oceans.
The above-commented sources/sinks and processes strongly control the distribution and concentration of REEs in marine systems. The observed natural concentrations of REEs in seawater are usually in orders of pmol/kg (Bruland and Lohan, 2003), making the determination of REEs in natural seawater a laborious task compared with continental ones where the concentrations are higher (Hatje et al., 2014). For this reason, and due to constraints of measuring a salt matrix, there is a predominance of studies carried out in continental waters in comparison with marine ones. For the determination of REEs in aquatic systems, multielement quantification is needed, with high precision and sensitivity, over a wide concentration range (Hatje et al., 2014). Despite the difficulties and limitations, detectable levels of REEs have been reported worldwide in rivers, lakes, groundwater, coastal and open ocean seawater, and tap water (Akagi et al., 2004; Kulaksiz and Bau, 2007; Tepe et al., 2014; Merschel et al., 2015). Nevertheless, the implementation of international programs in the last years (e.g., Anderson, 2019) is improving the data coverage in marine systems, providing a valuable global dataset of trace element distribution. A bibliography review of the REE contents in seawater can be consulted in Table 1 and extended in Table SI.1. From the limited number of existing studies on REEs in seawater, the highest values have been found for Ce (778 pmol/kg), followed by Gd (181 pmol/kg), La (180 pmol/kg), and Nd (118 pmol/kg) (Akagi and Edanami, 2017) studying of REE concentrations in three port areas of Japan where water samples were taken from 10 cm above the seabed (Table SI.1). However, according to the REE contents observed in open waters and far away from anthropogenic sources, these values in general decrease and the data obtained in Japanese ports could be highly influenced by pollution and the proximity to the seabed. In less human-influenced waters, the highest REE concentrations appear in surface samples taken near the coast, as the case of the Weser Estuary (Kulaksiz and Bau, 2011) and the Queensland Estuary (Lawrence and Kamber, 2006). In these sampling points, however, the Ce content decreased drastically compared to that in Japan ports (more than 7 times), while for the other elements, lanthanide contents are around 1.5–2.3 times lower. It is interesting to remark that a high concentration of REEs was detected in deepwater oceanic samples, specifically in the bottom waters from the Eastern North Pacific Ocean (Piepgras and Jacobsen, 1992) with the highest value of La reported (79.8 pmol/kg), discarding the comparison with values observed in Japan ports. In summary, the distribution of REEs in marine aquatic systems follows a complex process and depends on various factors such as salinity, ionic strength, and especially pH (Cantrell and Byrne, 1987; Elderfield et al., 1990).
Table 1 Minimum and maximum values of REEs (pmol/kg) were observed in different marine waters according to a bibliography revision.
According to compiled data, values of REEs are higher near the coast and in deep oceanic waters (Table SI.1). The first case should be explained due to the continental origin of REEs, previously commented, with the main sources of entry to the marine environment being rivers, runoff from soils, terrestrial landfills or mine wastes, and discharge or runoff from industrial effluents or wastewater systems (Gwenzi et al., 2018). Therefore, the highest REE levels are usually found in semienclosed coastal or port areas (i.e., estuaries), decreasing as we move to the open ocean. On the other hand, the distribution of REEs in the ocean water column follows an increasing pattern with depth, as commented before, with a maximum concentration of HREEs at around 3,000 m, while the LREE maximum can be found even deeper (Piepgras and Jacobsen, 1992) due to moderation by scavenging processes in the upper ocean (Bertram and Elderfield, 1993). However, it must be remarked that this behavior was different for Ce, with decreasing concentration according to depth (but discarding in the deepest waters). The distribution of REEs in seawater is generally dependent on the oceanographic parameters of the area (Cantrell and Byrne, 1987; Piepgras and Jacobsen, 1992) and mixing processes in estuaries (Lawrence and Kamber, 2006). During their transport, REEs tend to form complexes mainly with carbonates but also form sulfate, chloride, silicate, and fluoride complexes (Cantrell and Byrne, 1987; Soli and Byrne, 2017). Finally, the lowest values tend to appear in surface oceanic water samples, except for Ce, despite terrestrial inputs due to subsequent mixing and scavenging. However, it is important to remark that the lowest REE concentrations were registered in the most recent paper (Luong et al., 2018), which suggests that they can be influenced by the development of analytical techniques. Nevertheless, a similar increase in Ce concentration with water depth is also observed in this recent publication, and therefore this issue deserves further investigation.
Several chemical elements have been defined as essential macronutrients or micronutrients for living organisms. In the case of trace elements, the classification is not yet clear, and even, in some cases, the biological functions are extremely complicated because they require synergistic action of different elements to act, both, with positive and negative effects (Gumienna-Kontecka et al., 2017). To date, the biological roles of REEs are still unknown, and they are now grouped as non-essential elements (Blinova et al., 2018; Malhotra et al., 2020). However, several studies have reported that REEs can have both beneficial and adverse biological effects (Blinova et al., 2018; Romero-Freire et al., 2019; Jalali and Lebeau, 2021), which implies potential interactive effects and, therefore, they should be studied also as a group and not only individually. The REEs exist in all environmental compartments, and the background contents of REEs in living organisms have been reported (Blinova et al., 2018). Recent findings demonstrated that REEs possess biological functions in a broad range of enzymes and microorganisms, eliciting the need for research on the role of lanthanides in genetic regulation (Picone et al., 2019). REEs such as Ce3+ and La3+ have been discovered to be required by some bacteria for the methanol oxidation for carbon and energy (Hibi et al., 2011). Moreover, symbionts of deep-sea chemosynthetic mussels living near seepage sites have an enzyme La-dependent involved in converting methane to biomass and carbon dioxide (Wang et al., 2020). These results have promoted the interest in the understanding of connections between REE and microorganisms, such as REE mobilization from solids or immobilization from liquids by metabolic reactions and sorption by biomass processes, respectively (Brandl et al., 2016; Jalali and Lebeau, 2021). The biological properties of REEs derive from their similarity to other ions such as calcium, having been suggested displacement of the divalent charged Ca by the trivalent REE in Ca-binding sites in biological molecules (Jalali and Lebeau, 2021). In this context, the replacement of Ca2+ by Ln3+ ions in biological systems is a well-known strategy in medicine, and these ions’ similarities were the precursor of studies on their potential use in medicine (Wieszczycka et al., 2019).
Current literature data indicate that REEs are present in almost all parts of the human body (Wieszczycka et al., 2019), suggesting that they could have a biological role, although a low level of REEs in human tissues and fluids indicates minimal transfer through the food chain. The concentration of REEs by a factor of 7 in drinking water has been suggested to potentially risk human health (Khan et al., 2017). The pertinent data are scarce in the literature for living organisms or terrestrial compartments, and indeed no information is yet published for marine environments; therefore, the possible role of REEs in marine organisms still needs to be clarified. More studies about their potential biological role and their non-essential classification will be a further discussed topic in the following years.
Table 2 summarized REE contents in marine organisms (extended information can be consulted in Table SI.2). The highest concentrations for all REEs studied were in algae. Maximum values for all REEs were discovered in algae species collected in the Marine Protected Area of the Ligurian Sea (Squadrone et al., 2017) that despite being a preserved area is heavily influenced by the proximity of an industrial and commercial harbor with a high shipping traffic. The highest content in algae is in accordance with the data observed in a review performed by Squadrone et al. (2019), where the highest values of total REE accumulation were found in seaweed (macroalgae), reaching 62 mg REEs/kg, from Peninsular Malaysia. Seaweed species display the highest values for all REE series and also can accurately reflect the source of REEs, seawater solution or silicate particles (Fu et al., 2000). Some mollusks are also characterized by high REE accumulation, especially in tissues. Mollusk bivalves, as filtering organisms, obtain more uptake from food and water than from water alone due to metal exposure occurring in both food (particulate) and solution (Luoma, 1983). Drabaek et al. (1987) reported that the Ce concentration in the mussel Mytilus edulis was up to 3,000 µg/kg, while Riget et al. (1996) reported the baseline concentration for different elements in the same mussel species, having a Ce average concentration of 4,700 µg/kg, which is between 2.7 and 3.5 higher than for other extensively studied metals (i.e., Pb, Cd, and Cr). In the case of crustaceans, REE contents were, in general, lower for the maximum but higher for the minimum values reported for mollusks. Also low, in ranges of 0.098 to 0.001 µg/kg, were the values discovered in coral species collected in the overseas regions of Bermuda North Rock and particularly in the Tarawa atoll, Kiribati (Sholkovitz and Shen, 1995). In addition, exceptionally low (<0.015 µg/kg) were REE concentrations in marine fish species collected from local markets and supermarkets ready for human consumption in the Shandong province of China, located in the Yellow Sea (Yang et al., 2016). Therefore, the lowest REE values were found in wild coral and even lower in fish species. On the other hand, it is interesting to remark that it has been recently found that the macroalgae Gracilaria gracilis was able to effectively remove and recover REEs from low-concentration wastewater (Jacinto et al., 2018), whereas Andrès et al. (2003) also found promising results in REE removal with the use of microbial biomass.
Table 2 Concentrations (maximum, minimum, and mean content) of REEs in different marine species. More detailed information is in Table SI.2..
Given these results, we can infer that REEs can be accumulated in marine organisms, and their accumulation could be dependent on the trophic level, while no biomagnification seems to occur. Concentrations in organisms generally decrease with increasing trophic levels therefore, biomagnification of REEs was not expected. Producer organisms (algae) show the highest REE levels because they absorb the metals present in the water column directly through the cell surface. As we go, shellfishes (mollusks and crustaceans) have lower concentrations compared to those of algae species, whereas corals and fishes are the organisms with the lowest contents of REEs. The bioaccumulation of REEs in living organisms has been started to be studied in the last years. For aquatic organisms in a temperate lake, Amyot et al. (2017) observed that the REE contents are higher in zooplankton and benthos than those in fish, which implies again that REEs tend to undergo trophic dilution along with food webs. These are in accordance with the observed values in this review; however, no transfer studies have been performed in marine environments yet.
Finally, it is an interesting remark that thinking about the possibility of doing studies of risk assessment for REEs could be an interesting point, giving not only REE content values in marine organisms but also (bio)concentration factors (CFs). In this sense, the International Atomic Energy Agency (IAEA) (2004) report recommended CF values for some REEs, specifically from the TCEs, Ce, Sm, Eu, Gd, Tb, Dy, Tm, and Yb for different marine organisms. For fishes, CF values reported by IAEA (2004) were between 50 (Ce) and 300 L/kg (other studied REEs); for crustaceans, from 1,000 (Ce) to 4,000 L/kg; for mollusks, from 2,000 (Ce) to 7,000 L/kg; whereas for macroalgae, values were between 5,000 (Ce) and 3,000 (other studied REEs). In this revision, CF values were not reported in the papers consulted; however, next studies about REEs should have in consideration to add also the bioconcentration factor that will be very helpful for applying risk assessment models for REE.
Nowadays, REEs are not considered a risk in aquatic systems at the current levels they appear in aquatic flora and fauna (Supplementary Table S2), but according to different observed hot spots, the increasing use of these elements could shift this situation in the near future. A high bioaccumulation level has been detected in phytoplankton, suggesting the relevant release of REEs into the aquatic environment (Khan et al., 2017). Detectable concentrations of anthropogenic REEs were already found in the shells of mussels in rivers in Europe and North America (Merschel and Bau, 2015), proving their bioavailability and potential to enter and accumulate into the food chain. The possible risk of REEs to the environment resides mainly in their bioavailability. To estimate REE accumulation in organisms, it is useful to measure the concentration of REEs at which organisms have a response, which will be directly related to REE availability (Fang et al., 2007). However, we should keep in mind that REEs tend to fractionate depending on the chemical conditions (Shan et al., 2003; Wang et al., 2004). As previously mentioned, it was stated that in seawater, HREEs tend to remain in solution, while LREEs tend to be assimilated by organisms or adsorbed onto surfaces (Goldstein and Jacobsen, 1988; Byrne and Kim, 1990; Elderfield et al., 1990; Sholkovitz, 1995; Nozaki et al., 2000). Thus, the fate of REEs in aquatic organisms has shown a higher level of LREEs than MREEs and HREEs (Khan et al., 2017). This is due to the small ionic radii of HREEs that allow them to remain stable in solution through the formation of strong complexes (Piepgras and Jacobsen, 1992; Sholkovitz, 1995). Fractionation of REEs is also observed when their concentrations in organisms are studied, being the free form of REEs preferably assimilated by the organisms (Strady et al., 2015). In solution, there is a small fraction of free REEs, mostly free LREEs, readily to be incorporated into organisms (Strady et al., 2015; Yang et al., 2016; Li et al., 2016; Squadrone et al., 2017) and thus rendering LREEs a potential major biological impact. Another explanation for the REE fractionation in seawater points to diatom involvement (Akagi, 2013). According to this, diatoms absorb REEs on the surface and carry them down; once in deep water, REEs are released from the opal by dissolution and LREEs are preferentially scavenged by carbonate/oxide particles. These two processes (incorporation into opal and carbonate/oxide scavenging) would be responsible for the characteristic HREE-enriched composition in ocean waters.
The complexation of REEs with anthropogenic compounds may also increase the bioavailability in marine systems. For example, Schijf and Christy (2018) measured the stability of gadopentetic acid (Gd–DTPA), commonly used as a magnetic resonance imaging contrast agent, in the presence of Mg and Ca at seawater ionic strength, reporting that competition from Mg and Ca releases a significant amount of this bound Gd (up to 15%). This release back would have significant implications for the bioavailability of this toxic element in sensitive nearshore ecosystems. However, the form in which anthropogenic Gd (Gd-DTPA) is presented is extremely stable in its dissolved form, but several studies demonstrated that it is bioavailable to different marine organisms under exposure concentrations much greater than environmentally relevant levels (e.g., Pereto et al., 2020). The abundance of REEs in marine organisms is related to the composition of sediments or suspended particles and not only to the amount of REEs dissolved in seawater, unless filtering organisms (Akagi and Edanami, 2017). REEs can also be fractionated between the colloidal and the dissolved phase in aquatic systems; e.g., Sholkovitz (1992) observed that colloidal phases are systematically enriched in LREEs relative to HREEs.
There is lacking knowledge on the biochemical transfer of REEs in nature and more specifically in marine systems. Some works have recently studied the bioaccessibility of REEs through passive samplers such as diffuse gradients in thin films (DGTs) in estuarine waters and sediments (Gu et al., 2007; Cánovas et al., 2021; Huang et al., 2021); however, these devices have been scarcely applied in open sea for REE determination. Chevis et al. (2015) studied the flux of REEs in a sandy subterranean estuary, and their results revealed that sediment characteristics (e.g., mineralogy or Fe content) can constrain REE transport. REEs can form strong complexes with many different ligands, and the environmental parameters will affect their distribution and, therefore, their availability in the different natural compartments (Armand et al., 2015). More recently, Amyot et al. (2017) studied the REE transfer in abiotic and food web components of several temperate lakes in Canada and observed that the dissolved organic carbon and oxygen, as well as the REE contents in sediments, were the potential drivers of REE transfer. Additionally, they detected a trophic dilution of REEs along food webs. The REE biochemical cycle in marine systems and their transfer to marine organisms have been reported in two publications in the currently existing scientific literature. Strady et al. (2015) measured, for the first time, REEs in plankton, and they observed that although REE content in plankton was not justified by REE content in seawater, it was well correlated with calculated REEs3+ ions, whereas Macmillan et al. (2017) studied REE contents in different compartments, including marine environments, and observed that the highest REE contents were found in biota at the base food web (belonging to terrestrial and freshwater systems) followed by marine invertebrates (sea urchins and blue mussels). They further mentioned that REE contents decrease with trophic level across the marine ecosystem. Both observed that there was accumulation of many REEs in different biota, but a phenomenon of biomagnification did not appear to occur. The knowledge about the different REE natural cycles will be essential to predict the possible risks associated with REE transfer and will help to better understand REE anthropogenic pollution, but unfortunately, few studies are published addressing the biochemical transfer of REEs in marine systems. Therefore, as an emerging field of research and according to the anthropogenic increase of REEs and the observed potential REE bioaccumulation, more studies about their transfer into the food chain are apparently needed.
Currently, there are substantial gaps in our understanding of the potential adverse effects of REEs on living organisms and human health (Balaram, 2019). The multiple REE-associated adverse effects have been studied in a large number of species from mammals to microbes. Nevertheless, there are also a few studies pointing to the beneficial (hormetic) effects of REEs (Pagano et al., 2019). According to some investigations, REEs may pose a potential hazard to human and animal health (Rim et al., 2013; Pagano et al., 2019). But to date, only a few papers dealing with regulatory levels for REEs (in freshwaters) have been published (Sneller et al., 2000). In general, the existing studies have mainly focused on the acute toxicity of selected lanthanides in fresh aquatic invertebrates or aquatic plants (Mayfield and Fairbrother, 2015). However, Blinova et al. (2018) stated that acute toxicity data of REEs are not reliable and that more chronic tests should be performed. Nevertheless, concentrations due to anthropogenic input do not normally reach levels that induce ecotoxicity in a standard laboratory test performed (Romero-Freire et al., 2019). Therefore, the lack of key ecotoxicological data hinders the assessment of environmental impact and the attainment of environmental guidelines for REEs (Macmillan et al., 2017). Another constraint is that, usually, toxicity tests are performed mostly for individual elements, with a special emphasis on La, Ce, and Gd effects (González et al., 2015; Pagano et al., 2015). To date, information about the toxicity of other less studied REEs remains relatively scarce (Gong et al., 2019). Also, studies on the mixture toxicity of REEs are not very frequent (Romero-Freire et al., 2018). A final remark in this regard is that nearly no studies examine the aquatic toxicity of REEs to marine organisms.
Rogowska et al. (2018) in a review of the gadolinium toxicity (one of the most studied REEs) calculated from a bibliography review (using different living organisms) its ecotoxicological risk assessment and deduced that with the current Gd concentrations in waters, there is little risk to organisms living in the aquatic environment. On the other hand, Henriques et al. (2019) in a chronic test (28-day duration) with Gd and the mussel Mytilus galloprovincialis determined that although no mortality was observed during the test, the exposure to Gd strongly affected the biochemical performance of the mussels. Therefore, we cannot discard elevated Gd levels (or other REEs) in marine environments that could easily represent human risks in species such as M. galloprovincialis that are commercialized for direct consumption. González et al. (2015) studied the toxicity of three REEs (Ce, Gd, Lu) for different aquatic organisms, among them, a marine bacterium, Aliivibrio fischeri, which is one of the most used living organisms for acute toxicity testing. The observed results could be used as representative for marine organisms because the test medium is water enriched with NaCl. They found effective REE concentrations causing a 50% reduction in the luminescence of the bacteria (EC50) of >3,200 µg/L for Lu and >6,400 µg/L for Ce and Gd. However, these values are far higher than those observed in marine environments (Ce, 777.9 pmol/kg; Gd, 180.6 pmol/kg; Lu, 10.2 pmol/kg; S.I. Table 1). In another study using the same bacteria, from the observed toxicity of a mixture of the same three lanthanides, it was deduced that REE mixtures induced greater toxicity than individual components (potential synergistic effect) (Romero-Freire et al., 2019). Although the obtained information is extracted from an acute toxicity test and the calculated threshold is high compared to real marine contents, these findings suggest that there are differences observed between elements if they are studied as a group or individually, which will play a key role in the understanding of potential toxic effects.
REEs or “technology metals” were coined by the US Department of Energy, a group of 17 elements found in the Earth’s crust. These chemical elements are vital and irreplaceable to the world of technology owing to their unique physical, chemical, and light-emitting properties, all of which are beneficial in modern healthcare, telecommunication, and defense. REEs are relatively abundant in the Earth’s crust, with critical qualities to the device’s performance. The reuse and recycling of REEs through different technologies can minimize impacts on the environment; however, there are insufficient data about their biological, bioaccumulation, and health effects. The increasing usage of REEs has raised concerns about environmental toxicity, which may further cause harmful effects on human health. The study aims to review the toxicity analysis of these REEs concerning aquatic biota, considering them to be the sensitive indicators of the environment. Based on the limited reports of REE effects, the review highlights the need for more detailed studies on the hormetic effects of REEs. Aquatic biota is a cheap, robust, and efficient platform to study REE toxicity, mobility of REEs, and biomagnification in water bodies. REE diverse effects on aquatic life forms have been observed due to the lack of safety limits and extensive use in various sectors. Following the available data, we have put in efforts to compile all of the relevant research results in this paper related to the topic. This review approaches a vision on the concentrations of REEs in marine ecosystems with special attention to the water column and marine organisms, critically reviewing the existing information and the prospects of this new area of research.
One important feature of the REEs is their fractionation, showing different accumulation patterns in media between LREEs and HREEs, which reflect the geochemical processes that occurred during their transportation from land to sea. In seawater, LREEs are preferentially assimilated or adsorbed while HREEs tend to remain in solution. This fractionation depends on the physicochemical parameters, especially the media pH and the nature of the particulate matter. The distribution of REEs tends to display higher values near the coast compared with ocean waters mainly due to the continental origin of REEs. In the water column, the concentration increases with depth in ocean waters, which can be explained by sedimentary inputs and remineralization processes. For coastal waters, we find a surface maximum that decreases with depth until a certain stability. These observed trends, however, were not reflected in the case of Ce, which, in general, the lowest values tend to appear in surface oceanic water samples, and its content increases with water depth.
A biological role for REEs is still unknown, although they exist in all environmental compartments. REEs are accumulated in marine organisms, depending on the trophic level, but no biomagnification has been observed yet, which leads us to think that REEs may undergo trophic dilution. There is a lack of studies on the biological transfer from one trophic level to another, especially in the marine environment. Also important is the potential interactive effects of multiple REEs as a group in biological processes, as most of the existing studies, in soils and continental waters, are performed using just one element.
REE use is nowadays constantly increasing and may represent a significant environmental and human health risk. However, our current understanding of REEs as emerging contaminants is still limited, and no regulatory thresholds for REE concentrations have been set yet. We must be aware that when the contents of REEs presented in the environment or food go beyond a certain threshold (although it is not yet defined), they can display toxic effects. A well-grasped knowledge on the biochemical processes and fate of REEs in marine environments (one of the final sinks of pollutants) and mitigation strategies for REE pollution are needed for the safe development of high-tech sectors using these elements.
Future research about the presence and effects of REEs in marine environments should pay special emphasis to the following: 1) novel techniques to determine and monitor REE concentrations and bioavailability in marine systems; 2) the individual fractionation of REEs in marine systems, specifically Ce; 3) the potential biological functions of REEs; 4) the trend of REEs to undergo trophic dilution along with marine food webs (trophic transfer); and 5) the potential harmful effects to marine organisms due to the future increase of REE depositions caused by anthropogenic activities.
PN and AR made the bibliographic search, data curation, and the first draft of the manuscript. PN and AR wrote the final draft, reviewed it, and edit. MB, HQ, and CR critically revised and edited the manuscript. AR obtained the funding acquisition. CR and AC-G made the final revision and English editing and acts as the project supervisors. All authors contributed to the article and approved the submitted version.
This open-access publication was partially funded by the Spanish Ministry of Science and Innovation: IJC2019-041160-I (AR), IJC2018-035056-I (MB), RYC2019-027949-I (CR); and the Junta de Andalusia Researcher Programs: E-RNM-444-UGR20 (AR) and FEDER UHU-202021 (MB).
AR, MB, and CR thanks the Spanish Ministry of Science and Innovation for the Postdoctoral Fellowship granted under application references IJC2019-041160-I, IJC2018-035056-I, and RYC2019-027949-I, respectively. This revision is a contribution to the proyect ‘Metales Críticos para las Nuevas Tecnologías en Sistemas Costeros: Concentraciones, Biodisponibilidad, Transferencia Trófica y Evaluación de Riesgo (TEC-RISK)’ (Ref. PID2019-111261GB-I00).
The authors declare that the research was conducted in the absence of any commercial or financial relationships that could be construed as a potential conflict of interest.
All claims expressed in this article are solely those of the authors and do not necessarily represent those of their affiliated organizations, or those of the publisher, the editors and the reviewers. Any product that may be evaluated in this article, or claim that may be made by its manufacturer, is not guaranteed or endorsed by the publisher.
The Supplementary Material for this article can be found online at: https://www.frontiersin.org/articles/10.3389/fmars.2022.920405/full#supplementary-material.
Abbott A. N., Haley B. A., McManus J., Reimers C. R. (2015). The sedimentary flux of dissolved rare earth elements to the ocean. Geochim. Cosmochim. Acta 154, 186–200. doi: 10.1016/j.gca.2015.01.010
Abbott A. N., Löhr S., Trethewy M. (2019). Are clay minerals the primary control on the oceanic rare earth element budget? Front. Mar. Sci. 6. doi: 10.3389/fmars.2019.00504
Adebayo S. B., Cui M., Hong T., White C. D., Martin E. E., Johannesson K. H. (2018). Rare earth elements geochemistry and Nd isotopes in the Mississippi river and gulf of Mexico mixing zone. Front. Mar. Sci. 5. doi: 10.3389/fmars.2018.00166
Akagi T. (2013). Rare earth element (REE)-silicic acid complexes in seawater to explain the incorporation of REEs in opal and the “leftover” REEs in surface water: New interpretation of dissolved REE distribution profiles. Geochim. Cosmochim. Acta 113, 174–192. doi: 10.1016/j.gca.2013.03.014
Akagi T., Edanami K. (2017). Sources of rare earth elements in shells and soft-tissues of bivalves from Tokyo bay. Mar. Chem. 194, 55–62. doi: 10.1016/j.marchem.2017.02.009
Akagi T., Hashimoto Y., F-F F., Tsuno H., Tao H., Nakano Y. (2004). Variation of the distribution coefficients of rare earth elements in modern coral-lattices: Species and site dependencies. Geochim. Cosmochim. Acta 68, 2265–2273. doi: 10.1016/j.gca.2003.12.014
Alibo D. S., Nozaki Y. (1999). Rare earth elements in seawater: Particle association, shale-normalization, and ce oxidation. Geochim. Cosmochim. Acta 63, 363–372. doi: 10.1016/S0016-7037(98)00279-8
Amyot M., Clayden M. G., Macmillan G. A., Perron T., Arscott-gauvin A. (2017). Fate and trophic transfer of rare earth elements in temperate lake food webs. Env. Sci. Technol. 51, 6009–6017. doi: 10.1021/acs.est.7b00739
Andrade R. L. B., Hatje V., Pedreira R. M. A., Böning P., Pahnke K. (2020). REE fractionation and human gd footprint along the continuum between paraguaçu river to coastal south Atlantic waters. Chem. Geol. 532, 119303. doi: 10.1016/j.chemgeo.2019.119303
Andrès Y., Texier A. C., Le Cloirec P. (2003). Rare earth elements removal by microbial biosorption: A review. Environ. Technol. 24, 1367–1375. doi: 10.1080/09593330309385681
Armand R., Cherubini C., Tuduri J., Pastore N., Pourret O. (2015). Rare earth elements in French stream waters - revisiting the geochemical continental cycle using FOREGS dataset. J. Geochem. Explor. 157, 132–142. doi: 10.1016/j.gexplo.2015.06.006
Balaram V. (2019). Rare earth elements: A review of applications, occurrence, exploration, analysis, recycling, and environmental impact. Geosci. Front. 10, 1285–1303. doi: 10.1016/j.gsf.2018.12.005
Bau M., Dulski P. (1996). Anthropogenic origin of positive gadolinium anomalies in river waters. Earth Planet. Sci. Lett. 143, 245–255. doi: 10.1016/0012-821X(96)00127-6
Bau M., Knappe A., Dulski P. (2006). Anthropogenic gadolinium as a micropollutant in river waters in Pennsylvania and in lake Erie, northeastern united states. Chem. Erde 66, 143–152. doi: 10.1016/j.chemer.2006.01.002
Bau M., Schmidt K., Pack A., Bendel V., Kraemer D. (2018). The European shale: an improved data set for normalisation of rare earth element and yttrium concentrations in environmental and biological samples from Europe. Appl. Geochem. 90, 142–149. doi: 10.1016/j.apgeochem.2018.01.008
Bertram M. A., Elderfield H. (1993). The geochemical balance of the rare earth elements and neodynium isotopes in the oceans. Geochim. Cosmochim. Acta 57, 1956–1957. doi: 10.1016/0016-7037(93)90087-D
Blinova I., Lukjanova A., Muna M., Vija H., Kahru A. (2018). Evaluation of the potential hazard of lanthanides to freshwater microcrustaceans. Sci. Total Environ. 642, 1100–1107. doi: 10.1016/j.scitotenv.2018.06.155
Brioschi L., Steinmann M., Lucot E., Pierret M. C., Stille P., Prunier J., et al. (2013). Transfer of rare earth elements (REE) from natural soil to plant systems: implications for the environmental availability of anthropogenic REE. Plant Soil 366, 143–163. doi: 10.1007/s11104-012-1407-0
Byrne R. H., Kim K.-H. (1990). Rare earth elements scavenging in seawater. Geochim. Cosmochim. Acta 54, 2645–2656. doi: 10.1016/0016-7037(90)90002-3
Cánovas C. R., Basallote M. D., Macías F., Olías M., Pérez-López R., Ayora C., et al. (2021). Geochemical behaviour and transport of technology critical metals (TCMs) by the tinto river (SW Spain) to the Atlantic ocean. Sci. Total Environ. 764, 143796. doi: 10.1016/j.scitotenv.2020.143796
Cantrell K. J., Byrne R. H. (1987). Rare earth element complexation by carbonate and oxalat ions. Geochim. Cosmochim. Acta 51, 597–605. doi: 10.1016/0016-7037(87)90072-X
Chakhmouradian A. R., Wall F. (2012). Rare earth elements: Minerals, mines, magnets. Elements 8, 333–340. doi: 10.2113/gselements.8.5.333
Chen T. Y., Rempfer J., Frank M., Stumpf R., Molina-Kescher M. (2013). Upper ocean vertical supply: A neglected primary factor controlling the distribution of neodynium concentrations of open ocean surface waters? J. Geophysical Research: Oceans 118, 3887–3894. doi: 10.1002/jgrc.20288
Chevis D. A., Johannesson K. H., Burdige D. J., Cable J. E., Martin J. B., Roy M. (2015). Rare earth element cycling in a sandy subterranean estuary in Florida, USA. Mar. Chem. 176, 34–50. doi: 10.1016/j.marchem.2015.07.003
Cobelo-García A., Filella M. (2017). Electroanalytical techniques for the quantification of technology-critical elements in environmental samples. Curr. Opin. Electrochem. 3, 78–90. doi: 10.1016/j.coelec.2017.06.014
Crocket K. C., Hill E., Abell R. E., Johnson C., Gary S. F., Brand T., et al. (2018). Rare earth element distribution in the ne Atlantic: Evidence for benthic sources, longevity of the seawater signal, and biogeochemical cycling. Front. Mar. Sci. 5. doi: 10.3389/fmars.2018.00147
Drabaek I., Eichner P., Rasmussen L. (1987). Concentrations of rare earth elements in sediments, mussels and fish from a Danish marine environment, lillebaelt. j. radioanal. Nucl. Chem. Artic. 114, 29–38. doi: 10.1007/BF02048872
Dushyantha N., Batapola N., Ilankoon I. M. S. K., Rohitha S., Premasiri R., Abeysinghe B., et al. (2020). The story of rare earth elements (REEs): Occurrences, global distribution, genesis, geology, mineralogy and global production. Ore Geol. Rev. 122, 103521. doi: 10.1016/j.oregeorev.2020.103521
Elbaz-Poulichet F., Seidel J., Othoniel C. (2002). Occurrence of an anthropogenic gadolinium anomaly in river and coastal waters of southern France Fran c. Water Res. 36, 1102–1105. doi: 10.1016/S0043-1354(01)00370-0
Elderfield H., Greaves M. J. (1982). The rare earth elements in seawater. Nature 296, 214–219. doi: 10.1038/296214a0
Elderfield H., Upstill-Goddard R., Sholkovitz E. R. (1990). The rare earth elements in rivers, estuaries, and coastal seas and their signficance to the composition of ocean waters. Geochim. Cosmochim. 54, 971–991. doi: 10.1016/0016-7037(90)90432-K
Fang J., Wen B., Shan X. Q., Wang ,. H., Lin J., Zhang S. (2007). Evaluation of bioavailability of light rare earth elements to wheat (Triticum aestivum l.) under field conditions. Geoderma 141, 53–59. doi: 10.1016/j.geoderma.2007.05.014
Fisher A., Kara D. (2016). Determination of rare earth elements in natural water samples - a review of sample separation , preconcentration and direct methodologies. Anal. Chim. Acta 935, 1–29. doi: 10.1016/j.aca.2016.05.052
Fu F., Akagi T., Yabuki S., Iwaki M., Ogura N. (2000). Distribution of rare earth elements in seaweed: Implication of two different sources of rare earth elements and silicon in seaweed. J. Phycology 36, 62–70. doi: 10.1046/j.1529-8817.2000.99022.x
Geagea M. L., Stille P., Millet M., Perrone T. (2007). REE characteristics and Pb C.OMMAS.R.X.X.X and Nd isotopic compositions of steel plant emissions. Sci. Total Environ. 373, 404–419. doi: 10.1016/j.scitotenv.2006.11.011
Goldstein S. J., Jacobsen S. B. (1988). Rare earth elements in river waters. Earth Planet. Sci. Lett. 89, 35–47. doi: 10.1016/0012-821X(88)90031-3
Gong B., He E., Qiu H., Li J., Ji J., Zhao L., et al. (2019). Phytotoxicity of individual and binary mixtures of rare earth elements (Y, la, and ce) in relation to bioavailability. Environ. pollut. 246, 114–121. doi: 10.1016/j.envpol.2018.11.106
González V., Vignati D. A. L., Pons M., Montarges-Pelletier E., Bojic C., Giamberini L. (2015). Lanthanide ecotoxicity: First attempt to measure environmental risk for aquatic organisms nica gonz a ver o. Environ. pollut. 199, 139–147. doi: 10.1016/j.envpol.2015.01.020
Gumienna-Kontecka E., Rowińska-Zyrek M., Luczkowski M. (2018). Recent Advances in Trace Elements, Chapter 9: The Role of Trace Elements in Living Organisms. doi: 10.1002/9781119133780.ch9
Gu T., Shi C., Pu W., Yan W., Liu M., Yan M. (2007). New progress on certified reference materials for platinum group elements. Jour. Geostand. Geoanal. Res 31 (2), 125–132. doi: 10.1111/j.1751-908X.2007.00837.x
Gwenzi W., Mangori L., Danha C., Chaukura N., Dunjana N., Sanganyado E. (2018). Sources, behaviour, and environmental and human health risks of high-technology rare earth elements as emerging contaminants. Sci. Total Environ. 636, 299–313. doi: 10.1016/j.scitotenv.2018.04.235
Haley B. A., Du J., Abbott A. N., McManus J. (2017). The impact of benthic processes on rare earth element and neodymium isotope distributions in the oceans. Front. Mar. Sci. 4. doi: 10.3389/fmars.2017.00426
Hatje V., Bruland K. W., Flegal A. R. (2014). Determination of rare earth elements after pre-concentration using NOBIAS-chelate PA-1 ® resin: Method development and application in the San Francisco bay plume. Mar. Chem. 160, 34–41. doi: 10.1016/j.marchem.2014.01.006
Henriques B., Coppola F., Monteiro R., Pinto J., Viana T., Pretti C., et al. (2019). Toxicological assessment of anthropogenic gadolinium in seawater: Biochemical effects in mussels mytilus galloprovincialis. Sci. Total Environ. 664, 626–634. doi: 10.1016/j.scitotenv.2019.01.341
Hibi Y., Asai K., Arafuka H., Hamajima M., Iwama T., Kawai K. (2011). olecular structure of La3+-induced methanol dehydrogenase-like protein in Methylobacterium radiotolerans. J Biosci Bioeng 111 (5), 547–49. doi: 10.1016/j.jbiosc.2010.12.017
Huang J., Hills J., Teasdale P. R., Panther J. G., Wang F., Welsh D. T. (2021). Evaluation of the chelex-DGT technique for the measurement of rare earth elements in the porewater of estuarine and arine sediments. Talanta 230, 122315. doi: 10.1016/j.talanta.2021.122315
IAEA (2004). Sediment distribution coefficients and concentration factors for biota in the marine environment. International Atomic Energy Agency.
Jacinto J., Henriques B., Duarte A. C., Vale C., Pereira E. (2018). Removal and recovery of critical rare elements from contaminated waters by living gracilaria gracilis. J. Hazard. Mater. 344, 531–538. doi: 10.1016/j.jhazmat.2017.10.054
Jalali J., Lebeau T. (2021). The role of microorganisms in mobilization and phytoextraction of rare earth elements: A review. Front. Environ. Sci. 9. doi: 10.3389/fenvs.2021.688430
Khan A. M., Bakar N. K. A., Bakar A. F. A., Ashraf M. A. (2017). Chemical speciation and bioavailability of rare earth elements (REEs) in the ecosystem: a review. Environ. Sci. pollut. Res. 24, 22764–22789. doi: 10.1007/s11356-016-7427-1
Kulaksiz S., Bau M. (2007). Contrasting behaviour of anthropogenic gadolinium and natural rare earth elements in estuaries and the gadolinium input into the north Sea. Earth Planet. Sci. Lett. 260, 361–371. doi: 10.1016/j.epsl.2007.06.016
Kulaksiz S., Bau M. (2011). Anthropogenic gadolinium as a microcontaminant in tap water used as drinking water in urban areas and megacities. Appl. Geochem. 26, 1877–1885. doi: 10.1016/j.apgeochem.2011.06.011
Kulaksiz S., Bau M. (2013). Anthropogenic dissolved and colloid / nanoparticle-bound samarium , lanthanum and gadolinium in the Rhine river and the impending destruction of the natural rare earth element distribution in rivers. Earth Planet. Sci. Lett. 342, 43–50. doi: 10.1016/j.epsl.2012.11.033
Lacan F., Jeandel C. (2005). Neodymium isotopes as a new tool for quantifying exchange fluxes at the continent-ocean interface. Earth Planet. Sci. Lett. 232, 245–257. doi: 10.1016/j.epsl.2005.01.004
Lawrence M. G. (2010). Detection of anthropogenic gadolinium in the Brisbane river plume in moreton bay, Queensland, Australia. Mar. pollut. Bull. 60, 1113–1116. doi: 10.1016/j.marpolbul.2010.03.027
Lawrence M. G., Kamber B. S. (2006). The behaviour of the rare earth elements during estuarine mixing — revisited. Mar. Chem. 100, 147–161. doi: 10.1016/j.marchem.2005.11.007
Lawrence M. G., Ort C., Keller J. (2009). Detection of anthropogenic gadolinium in treated wastewater in south East Queensland, Australia. Water Res. 43, 3534–3540. doi: 10.1016/j.watres.2009.04.033
Li J. X., Zheng L., Sun C. J., Jiang F. H., Yin X. F., Chen J. H., et al. (2016). Study on ecological and chemical properties of rare earth elements in tropical marine organisms. Chin. J. Anal. Chem. 44, 1539–1546. doi: 10.1016/S1872-2040(16)60963-5
Luoma S. N. (1983). Bioavailability of trace metals to aquatic organisms. A review. Sci. Total Environ. 28, 1–22. doi: 10.1016/S0048-9697(83)80004-7
Luong L. D., Shinjo R., Hoang N., Shakirov R. B., Syrbu N. (2018). Spatial variation in dissolved rare earth element concentrations in the East China Sea water column. Mar. Chem. 20, 1–15. doi: 10.1016/j.marchem.2018.07.004
Macmillan G. A., Chételat J., Heath J. P., Mickpegak R., Amyot M. (2017). Rare earth elements in freshwater, marine, and terrestrial ecosystems in the eastern Canadian Arctic. Environ. Sci. Process. Impacts 18, 1336–1345. doi: 10.1039/C7EM00082K
Malhotra N., Hsu H.-S., Liang S.-T., Roldan M. J. M., Lee J.-S., Ger T.-R., et al (2020). An updated review of toxicity effect of the rare earth elements (rees) on aquatic organisms. Animals 10, 1663. doi: 10.3390/ani10091663
Massari S., Ruberti M. (2013). Rare earth elements as critical raw materials: Focus on international markets and future strategies. Resour. Policy 38, 36–43. doi: 10.1016/j.resourpol.2012.07.001
Mayfield D. B., Fairbrother A. (2015). Examination of rare earth element concentration patterns in freshwater fish tissues. Chemosphere 120, 68–74. doi: 10.1016/j.chemosphere.2014.06.010
Merschel G., Bau M. (2015). Rare earth elements in the aragonitic shell of freshwater mussel corbicula fluminea and the bioavailability of anthropogenic lanthanum, samarium and gadolinium in river water. Sci. Total Environ. 533, 91–101. doi: 10.1016/j.scitotenv.2015.06.042
Merschel G., Bau M., Baldewein L., Dantas E. L., Walde D., Bühn B. (2015). Tracing and tracking wastewater-derived substances in freshwater lakes and reservoirs: Anthropogenic gadolinium and geogenic REEs in lake paranoá, Brasilia. Comptes Rendus - Geosci. 347, 284–293. doi: 10.1016/j.crte.2015.01.004
Noack C. W., Dzombak D. A., Karamalidis A. K. (2014). Rare earth element distributions and trends in natural waters with a focus on groundwater. Environ. Sci. Technol. 48, 4317–4326. doi: 10.1021/es4053895
Nozaki Y., Lerche D., Alibo D. S., Tsutsumi M. (2000). Dissolved indium and rare earth elements in three Japanese rivers and Tokyo bay: Evidence for anthropogenic gd and in. Geochim. Cosmochim. Acta 64, 3975–3982. doi: 10.1016/S0016-7037(00)00472-5
Olías M., Cánovas C. R., Basallote M. D., Lozano A. (2018). Geochemical behaviour of rare earth elements (REE) along a river reach receiving inputs of acid mine drainage. Chem. Geol. 493, 468–477. doi: 10.1016/j.chemgeo.2018.06.029
Olías M., Cerón J. C., Fernández I., de la Rosa J. (2005). Distribution of rare earth elements in an alluvial aquifer affected by acid mine drainage: The guadiamar aquifer (SW Spain). Environ. pollut. 135, 53–64. doi: 10.1016/j.envpol.2004.10.014
Pagano G., Aliberti F., Guida M., Oral R., Siciliano A., Trifuoggi M., et al. (2015). Rare earth elements in human and animal health: State of art and research priorities. Environ. Res. 142, 215–220. doi: 10.1016/j.envres.2015.06.039
Pagano G., Thomas P. J., Di Nunzio A., Trifuoggi M. (2019). Human exposures to rare earth elements: Present knowledge and research prospects. Environ. Res. 171, 493–500. doi: 10.1016/j.envres.2019.02.004
Pearce C. R., Jones M. T., Oelkers E. H., Pradoux C., Jeandel C. (2013). The effect of particulate dissolution on the neodymium (Nd) isotope and rare earth element (REE) composition of seawater. Earth Planet. Sci. Lett. 369–370, 138–147. doi: 10.1016/j.epsl.2013.03.023
Pereto C., Coynel A., Lerat-Hardy A., Gourves P. Y. (2020). Corbicula fluminea: A sentinel species for urban rare earth element origin. Sci. Total Environ. 732, 138552. doi: 10.1016/j.scitotenv.2020.138552
Picone N., Jm H., Camp O. D. Role of rare earth elements in methanol oxidation. Curr. Opin. Chem. Biol. 49, 39–44. doi: 10.1016/j.cbpa.2018.09.019
Piepgras D. J., Jacobsen S. B. (1992). The behavior of rare earth elements in seawater: Precise determination of variations in the north pacific water column. Geochim. Cosmochim. Acta 56, 1851–1862. doi: 10.1016/0016-7037(92)90315-A
Riget F., Johansen P., Asmunf G. (1996). Influence of length on element concentrations in blue mussels (Mytilus edulis). Mar. pollut. Bull. 32, 745–751. doi: 10.1016/0025-326X(96)00067-7
Rim K. T., Koo K. H., Park J. S. (2013). Toxicological evaluations of rare earths and their health impacts to workers: A literature review. Saf. Health Work 4, 12–26. doi: 10.5491/SHAW.2013.4.1.12
Rogowska J., Olkowska E., Ratajczyk W., Wolska L. (2018). Gadolinium as a new emerging contaminant of aquatic environment. Environ. Toxicol. Chem. 37, 1523–1534. doi: 10.1002/etc.4116
Romero-Freire A., Joonas E., Muna M., Cossu-leguille C., Vignati D. A. L., Giamberini L. (2019). Assessment of the toxic effects of mixtures of three lanthanides (Ce , gd , Lu) to aquatic biota. Sci. Total Environ. 661, 276–284. doi: 10.1016/j.scitotenv.2019.01.155
Romero-Freire A., Minguez L., Pelletier M., Cayer A., Caillet C., Devin S., et al. (2018). Assessment of baseline ecotoxicity of sediments from a prospective mining area enriched in light rare earth elements. Sci. Total Environ. 612, 831–839. doi: 10.1016/j.scitotenv.2017.08.128
Rudnick R. L., Gao S. (2003). Composition of the continental crust. Treatise Geochem. 1, 1–64. doi: 10.1016/B0-08-043751-6/03016-4
Schijf J., Christenson E. A., Byrne R. H. (2015). YREE scaveging in seawater: A look at an old model. Mar. Chem. 177, 460–471. doi: 10.1016/j.marchem.2015.06.010
Schijf J., Christy I. J. (2018). Effect of mg and Ca on the stability of the MRI contrast agent gd-DTPA in seawater. Front. Mar. Sci. 5. doi: 10.3389/fmars.2018.00111
Schijf J., de Baar H. J. W. (1995). Rare earth element exchange through the Bosporus: The black Sea as a net source of REEs to the Mediterranean Sea. Geochim. Cosmochim. Acta 59, 3503–3509. doi: 10.1016/0016-7037(95)00233-P
Shan X., Zhongwen W., Weisheng W., Zhang S., Wen B. (2003). Labile rhizosphere soil solution fraction for prediction of bioavailability of heavy metals and rare earth elements to plants. Anal. Bioanal. Chem. 375, 400–407. doi: 10.1007/s00216-002-1711-2
Sholkovitz E. R. (1992). Chemical evolution of rare earth elements: fractionation between colloidal and solution phases of filtered river water. Earth Planet. Sci. Lett. 114, 77–84. doi: 10.1016/0012-821X(92)90152-L
Sholkovitz E. R. (1995). The aquatic chemistry of rare earth elements in rivers and estuaries. Aquat. Geochem. 1, 1–34. doi: 10.1007/BF01025229
Sholkovitz E., Shen G. T. (1995). The incorporation of rare earth elements in modern coral. Geochim. Cosmochim. Acta 59, 2749–2756. doi: 10.1016/0016-7037(95)00170-5
Siddall M., Khatiwala S., van de Flierdt T., Jones K., Goldstein S. L., Hemming S., et al. (2008). Towards explaining the Nd paradox using reversible scavenging in an ocean general circulation model. Earth Planet. Sci. Lett. 274, 448–461. doi: 10.1016/j.epsl.2008.07.044
Soli A. L., Byrne R. H. (2017). Europium silicate complexation at 25C and 0.7 molar ionic strength. Mar. Chem. 195, 138–42. doi: 10.1016/j.marchem.2017.02.006
Sneller F. E. C., Kalf D. F., Weltje L., Van Wezel A. P. (2000). Maximum permissible concentrations and negligible concentrations for rare earth elements (REEs). RIVM (RIVM--601501011). Netherlands.
Squadrone S., Brizio P., Battuello M., Nurra N., Sartor R. M., Benedetto A., et al. (2017). A first report of rare earth elements in northwestern Mediterranean seaweeds. Mar. pollut. Bull. 122, 236–242. doi: 10.1016/j.marpolbul.2017.06.048
Squadrone S., Brizio P., Stella C., Mantia M., Battuello M., Nurra N., et al. (2019). Rare earth elements in marine and terrestrial matrices of northwestern Italy: Implications for food safety and human health. Sci. Total Environ. 660, 1383–1391. doi: 10.1016/j.scitotenv.2019.01.112
Stichel T., Pahnke K., Duggan B., Goldstein S. L., Hartman A. E., Paffrath R., et al. (2018). TAG plume: Revisiting the hydrothermal neodymium contribution to seawater. Front. Mar. Sci. 5. doi: 10.3389/fmars.2018.00096
Strady E., Kim I., Radakovitch O., Kim G. (2015). Rare earth element distributions and fractionation in plankton from the northwestern Mediterranean Sea. Chemosphere 119, 72–82. doi: 10.1016/j.chemosphere.2014.05.049
Sun J., Zhao H., Wang Y. (1994). Study on the contents of trace rare earth elements and their distribution in wheat and rice samples by RNAA. J. Radioanal. Nucl. Chem. 179, 377–383. doi: 10.1007/BF02040174
Taylor S. R., McLennan S. M. (1985). The continental crust: its composition and evolution. (Oxford: Blackwell), 1–312.
Tepe N., Romero M., Bau M. (2014). High-technology metals as emerging contaminants: Strong increase of anthropogenic gadolinium levels in tap water of Berlin, Germany, from 2009 to 2012. Appl. Geochem. 45, 191–197. doi: 10.1016/j.apgeochem.2014.04.006
U.S.GAO (2022) Rare earth materials in the defense supply chain. gao-10-617R 2–36. Available at: http://www.gao.gov/new.items/d.
van de Flierdt T., Frank M., Lee D. C., Halliday A. N., Reynolds B. C., Hein J. R. (2004). New constraints on the sources and behavior of neodymium and hafnium in seawater from pacific ocean ferromanganese crusts. Geochim. Cosmochim. Acta 68, 3827–3843. doi: 10.1016/j.gca.2004.03.009
Wang X., Barrat J. A., Bayon G., Chauvaud L., Feng D. (2020). Lanthanum anomalies as fingerprints of methanotrophy. Geochemical Perspect. Lett. 14, 26–30. doi: 10.7185/geochemlet.2019
Wang X. P., Shan X. Q., Zhang S. Z., Wen B. (2004). A model for evaluation of the phytoavailability of trace elements to vegetables under the field conditions. Chemosphere 55, 811–822. doi: 10.1016/j.chemosphere.2003.12.003
Wen B., Liu Y., Hu X., Shan X. (2006). Effect of earthworms (Eisenia fetida) on the fractionation and bioavailability of rare earth elements in nine Chinese soils. Chemosphere 63, 1179–1186. doi: 10.1016/j.chemosphere.2005.09.002
Wieszczycka K., Staszak K., Wozniak-Budych M. J., Jurga S. (2019). Lanthanides and tissue engineering strategies for bone regeneration. Coord. Chem. Rev. 388, 248–267. doi: 10.1016/j.ccr.2019.03.003
Yang L., Wang X., Nie H., Shao L., Wang G., Liu Y. (2016). Residual levels of rare earth elements in freshwater and marine fish and their health risk assessment from Shandong, China. Mar. pollut. Bull. 107, 393–397. doi: 10.1016/j.marpolbul.2016.03.034
Zepf V. (2013). new approach to the nexus of supply, demand and use: exemplified along the use of neodynium in permanent magnets. doi: 10.1007/978-3-642-35458-8
Keywords: rare earth elements, seawater, marine organisms, concentrations, toxicity, bioaccumulation
Citation: Neira P, Romero-Freire A, Basallote MD, Qiu H, Cobelo-García A and Cánovas CR (2022) Review of the concentration, bioaccumulation, and effects of lanthanides in marine systems. Front. Mar. Sci. 9:920405. doi: 10.3389/fmars.2022.920405
Received: 14 April 2022; Accepted: 30 June 2022;
Published: 28 July 2022.
Edited by:
Gordon T. Taylor, Stony Brook University, United StatesReviewed by:
Nicholas Fisher, Stony Brook University, United StatesCopyright © 2022 Neira, Romero-Freire, Basallote, Qiu, Cobelo-García and Cánovas. This is an open-access article distributed under the terms of the Creative Commons Attribution License (CC BY). The use, distribution or reproduction in other forums is permitted, provided the original author(s) and the copyright owner(s) are credited and that the original publication in this journal is cited, in accordance with accepted academic practice. No use, distribution or reproduction is permitted which does not comply with these terms.
*Correspondence: Patricia Neira, cGF0cmljaWEubmVpcmFAbWFyaW5lLmll; Ana Romero-Freire, YW5hcm9tZXJvZkB1Z3IuZXM=; Antonio Cobelo-Garcia, YWNvYmVsb0BpaW0uY3NpYy5lcw==
†These authors share first authorship
Disclaimer: All claims expressed in this article are solely those of the authors and do not necessarily represent those of their affiliated organizations, or those of the publisher, the editors and the reviewers. Any product that may be evaluated in this article or claim that may be made by its manufacturer is not guaranteed or endorsed by the publisher.
Research integrity at Frontiers
Learn more about the work of our research integrity team to safeguard the quality of each article we publish.