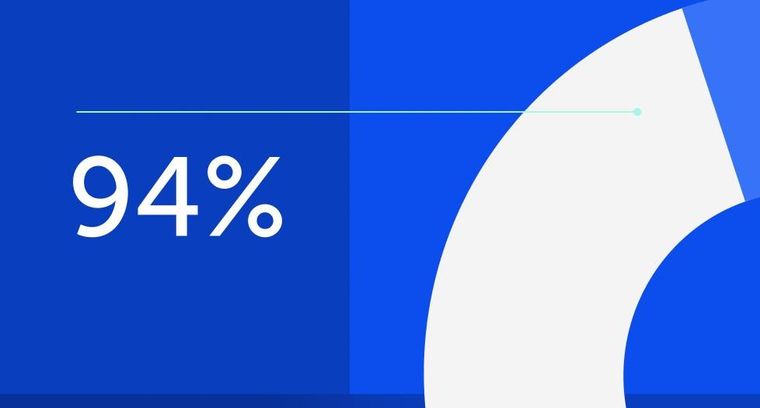
94% of researchers rate our articles as excellent or good
Learn more about the work of our research integrity team to safeguard the quality of each article we publish.
Find out more
ORIGINAL RESEARCH article
Front. Mar. Sci., 11 October 2022
Sec. Deep-Sea Environments and Ecology
Volume 9 - 2022 | https://doi.org/10.3389/fmars.2022.915650
This article is part of the Research TopicUnderstanding Ocean Ridges, a New Frontier for Science and DevelopmentView all 18 articles
Deep-sea mining activities are expected to impact deep-sea biota through the generation of sediment plumes that disperse across vast areas of the ocean. Benthic sessile suspension-feeding fauna, such as cold-water corals, may be particularly susceptible to increased suspended sediments. Here, we exposed the cold-water octocoral, Dentomuricea aff. meteor to suspended particles generated during potential mining activities in a four weeks experimental study. Corals were exposed to three experimental treatments: (1) control conditions (no added sediments); (2) suspended polymetallic sulphide (PMS) particles; (3) suspended quartz particles. The two particle treatments were designed to distinguish between potential mechanical and toxicological effects of mining particles. PMS particles were obtained by grinding PMS inactive chimney rocks collected at the hydrothermal vent field Lucky Strike. Both particle types were delivered at a concentration of 25 mg L-1, but achieved suspended concentrations were 2-3 mg L-1 for the PMS and 15-18 mg L-1 for the quartz particles due to the different particle density. Results of the experiment revealed a significant increase in dissolved cobalt, copper and manganese concentrations in the PMS treatment, resulting from the oxidation of sulphides in contact with seawater. Negative effects of PMS exposure included a progressive loss in tissue condition with necrosis and bioaccumulation of copper in coral tissues and skeletons, and death of all coral fragments by the end of the experiment. Physiological changes under PMS exposure, included increased respiration and ammonia excretion rates in corals after 13 days of exposure, indicating physiological stress and potential metabolic exhaustion. Changes in the cellular stress biomarkers and gene expression profiles were more pronounced in corals exposed to quartz particles, suggesting that the mechanical effect of particles although not causing measurable changes in the physiological functions of the coral, can still be detrimental to corals by eliciting cellular stress and immune responses. We hypothesize that the high mortality of corals recorded in the PMS treatment may have resulted from the combined and potentially synergistic mechanical and toxicological effects of the PMS particles. Given the dispersal potential of mining plumes and the highly sensitive nature of octocorals, marine protected areas, buffer areas or non-mining areas may be necessary to protect deep-sea coral communities.
Polymetallic Sulphide (PMS) deposits produced at hydrothermal vents in the deep sea are of interest by mining companies as an alternative to terrestrial mineral resources, and to respond to increasing demand for rare minerals for technology (Petersen et al., 2016; Sharma, 2017). However, the potential impacts of mining activities on deep-sea fauna and ecosystems are poorly understood (Van Dover et al., 2017; Van Dover et al., 2020), limiting ecological risk assessments (Durden et al., 2017; Washburn et al., 2019; Smith et al., 2020) and hindering the establishment of management standards and guidelines for deep-sea industries and activities (Wedding et al., 2015; Levin et al., 2016; Jones et al., 2019).
Mining for PMS is expected to impact deep-sea ecosystems in several ways (Gwyther, 2008; Boschen et al., 2013; Van Dover, 2014; Levin et al., 2016; Miller et al., 2018; Weaver and Billett, 2019; Christiansen et al., 2020). Physical destruction of the seabed and damage to the habitat and fauna by the mining equipment, along with changes in seafloor topography and geochemical characteristics are expected in the close vicinity of the mining site (Boschen et al., 2013; Miller et al., 2018). In addition, the generation of sediment plumes of fine particulate material by the seabed mining and surface dewatering operations will disperse in the water column and eventually settle on the seafloor, potentially smothering surrounding fauna (Gwyther, 2008; Christiansen et al., 2020; Morato et al., 2022 this issue). These sediment plumes may transport metal complexes trapped in the sediments (e.g., copper, cadmium) that can be released to the water column in concentrations toxic to marine biota (Hauton et al., 2017; Fallon et al., 2019).
Exposure to elevated suspended sediments in the water column can have sublethal and lethal effects on benthic sessile suspension and filter feeding fauna by impairing feeding and respiration (e.g., Erftemeijer et al., 2012; Strachan and Kingston, 2012). The impacts of sediment plumes are of particular concern for cold-water corals that form extensive and highly structured ecosystems in the deep-sea (Roberts et al., 2009). Indeed, recent studies have predicted some degree of overlap between the dispersal of PMS plumes and the distribution of cold-water corals in the Mid Atlantic Ridge and seamounts around the Azores (Morato et al., 2022). In this region, cold-water octocorals are among the most important ecosystem engineers forming dense coral garden communities (Braga-Henriques et al., 2013; Morato et al., 2021). These communities play important ecological roles in carbon and nitrogen cycling (Rakka et al., 2021; Rovelli et al., 2022) and as habitat providers for a variety of invertebrates and commercially important fish species (Pham et al., 2015; Carreiro-Silva et al., 2017; Gomes-Pereira et al., 2017).
Anthropogenic impacts on these ecosystems can be long lasting owing to their slow-growing and long-lived life histories and slow recovery capacity (Roberts et al., 2009; Clark et al., 2019), and thus these ecosystems have been classified as vulnerable marine ecosystems (VMEs) in need of protection (FAO, 2009; OSPAR, 2010). However, the potential impacts of PMS mining plumes on cold-water corals are still poorly understood.
Evidence from tropical environments showed that high sedimentation can cause smothering and burial of coral polyps, resulting in tissue damage, decreased food intake, increased mucus production and polyp movement resulting in increased metabolic costs (Rogers, 1990; Erftemeijer et al., 2012). Prolonged exposure to sedimentation can result in decreased growth rates, reduced reproductive output and increased mortality (Fabricius and Wolanski, 2000; Weber et al., 2006; Jones et al., 2015). The effects of sedimentation on cold-water corals have been investigated for only a few species. Studies on cold-water corals have focused on the impact of natural sediments and drill cuttings originating from hydrocarbon exploration on the scleractinian Lophelia pertusa (recently synonymized as Desmophyllum pertusum: Addamo et al., 2016), showing little impact on its physiology (Brooke et al., 2009; Larsson and Purser, 2011; Allers et al., 2013; Larsson et al., 2013; Baussant et al., 2018; da Rocha et al., 2021). On the other hand, recent studies on the impacts of excessive sedimentation produced by mine tailing deposition on benthic ecosystems in the Norwegian fjords (Liefmann et al., 2018; Scanes et al., 2018), suggest that octocorals (Duva florida, Primnoa resedaeformis) are more sensitive to suspended sediments than scleractinians. Results of these studies showed tissue damage from the sharp morphology of mine tailings and increased energetic demand likely associated with the active removal of sediments from the polyps. However, there are no studies that have evaluated the impact of PMS on cold-water corals.
Moreover, most of the above studies have focused on physiological impacts on tissue condition and metabolic responses (e.g., respiration, excretion). Nevertheless, cellular changes in protein production and gene expression usually occur before physiological damage is evident (Downs et al., 2005). Therefore, cellular biomarkers can be used as important tools to detect sub-lethal responses to anthropogenic impacts (Downs et al., 2005; Downs et al., 2012). These tools provide a means to evaluate the ability of animals to regulate adaptive responses to stress which ultimately will determine their survival. In corals, changes in gene expression and enzyme activity related to cellular stress, detoxification and oxidative damage have been studied to reveal important physiological pathways to cope with environmental stress and chemical contaminants (e.g., Carreiro-Silva et al., 2014; Marangoni et al., 2017; Fonseca et al., 2019; Servetto et al., 2021). Using these biomarkers is particularly important to identify potential ecotoxicological effects caused by chemicals in the PMS particles that cannot be ascertained at the organism level.
In the present study, we investigated the effects of PMS particles from inactive chimney rocks of the Lucky Strike hydrothermal vent field crushed into fine particles, on the octocoral Dentomuricea aff. meteor. This species forms extensive coral gardens in the Azores seamounts between 200 and 400 m depth that can be affected by the horizontal and vertical dispersal of mining plumes. The putative mechanical and toxicological effects of PMS particles were evaluated through measurements of the coral physiological responses at the organism (respiration, nutrient excretion), tissue (bioaccumulation of metals, structural integrity) and cell (enzyme activity and gene expression) levels.
Live colonies of D. aff. meteor were collected at the summit of Condor Seamount (38˚ 08’N, 29˚ 05’W) using the ROV SP at depths between 185-210 m in August 2014, characterized by average bottom temperatures of 15°C and salinity of 36 ppt for this time of the year (Rovelli et al., 2022). Colonies were transferred to the DeepSeaLab aquaria facilities at IMAR/Okeanos in coolers and distributed in two 170 L aquaria in a thermo-regulated room (14 ± 0.7 °C) in darkness, within hours after collection. The aquaria system consisted of a continuous flow-through open system of oligotrophic oceanic seawater pumped from 5 m depth (salinity ca. 36 ppt). Colonies were left to acclimate to atmospheric pressure conditions for two weeks with running natural seawater chilled to match in situ temperature and salinity conditions (15 ± 0.9°C, 36 ± 0.1 ppt) using cooling systems connected to temperature controllers. Six colonies were subsequently fragmented into 15 nubbins (i.e., coral fragments with 5-7 cm), mounted on bases made of inert epoxy putty and silicone tubing, and placed in four 25 L aquaria for further two weeks before the experiment. Seawater in each aquarium was continuously renewed (6 times/day at 5 L h-1) and mixed with submersible Iwaki pumps (4.5 W, 280 L h-1). Corals were fed five days a week, once a day, with a mixture of frozen adult Artemia salina and nauplii, mysids and microplankton (all from OceanNutrition™), and a food supplement composed of proteins, aminoacids, lipids, vitamins, and oligoelements (Marine Active Supplement, Bentos Nutrition).
Hydrothermal polymetallic sulphide. particles representative of plumes produced during the extraction of massive PMS deposits were obtained from an inactive sulphide chimney collected at 1750 m of depth by the ROV VICTOR6000 at the base of the Eiffel Tower structure at the Lucky Strike hydrothermal vent field during the research cruise MoMARSAT-2013 (Blandin et al., 2013) on the RV Pourquoi Pas?. Chimney fragments were ground in a tungsten carbide ring mill pulveriser and subsequently were analysed by laser diffraction (Mastersizer 2000) at the Center of Geology, University of Lisbon - CeGUL, Portugal for particle size analysis. The analysis revealed that 55% of the particles were between 0.5-10 µm in size (23%<2 µm, 32% 2-10 µm), 41% were 10-70 µm and 4% were >70 µm. Energy Dispersive X-Ray Analysis (EDAX) revealed that PMS particles were mainly composed of barite (BaSO4), pyrite (FeS2), and chalcopyrite (CuFeS2), with a minor composition of sphalerite ((ZnFe)S) (Figure S1). Elemental composition of PMS particles show increased concentrations of manganese (Mn), copper (Cu), nickel (Ni), cobalt (Co), zinc (Zn), arsenic (As), and cadmium (Cd) (Fallon et al., 2019). Polymetallic sulphide particle size matched the range expected by Seafloor Mining Tools excavation and dewatering processes, according to the Nautilus Minerals (80% of 0.5-10 µm and 20% of 10-70 µm) (ASA, 2008).
The quartz particles treatment, was prepared from commercially available silicon dioxide (SiO2) particles, by mixing 80% of 0.5-10 µm (#S5631, Sigma-Aldrich, Portugal) and 20% of 50-70 µm grain sizes (#274739, Sigma-Aldrich, Portugal). Particles of PMS and quartz were photographed using light and scanning electron microscopy (SEM). For light microscopy, we used a LEICA DM6000 microscope under 400× magnification. For SEM, particles were mounted on aluminum stubs and sputter-coated with gold. The scanning electron microscope Tescan VEGA3 xmu at 20 keV was used for PMS particles and JEOL JSM-5200LV at 25 keV was used for quartz particles.
Following the acclimation period, coral fragments were exposed to three experimental treatments for a period of 27 days: (1) a control treatment with no sediment addition; (2) suspended PMS particles; (3) suspended quartz particles. The two particles treatments were designed to distinguish between the potential physical and toxicological effects of particle exposure. Coral fragments were distributed in six 10 L aquaria (two aquaria per treatment) so that the same number of fragments of each colony was present in each experimental treatment. Accordingly, 15 coral fragments (five fragments from three different mother colonies) were present in each aquarium at the beginning of the experiment, totaling 30 fragments from six mother colonies in each treatment. Corals were fed once daily, seven days a week, as described in section above. Weekly, the excess food and particles that settled at the bottom of the aquarium were siphoned together with a 25% water replacement for each aquarium before food was added.
A semi-open seawater system was used for the experiment to ensure maximal exposure of the corals to the particle treatment. Thus, 8 hours of running seawater were intercalated with two periods of 4 hours in a closed system. PMS and inert particles were delivered at a concentration of 25 mg L-1 to the sediment exposure treatments. This concentration was chosen based on the concentrations used in drill cuttings experiments with the cold-water coral L. pertusa (Larsson et al., 2013) to allow for cross studies and particle types comparisons. Particle addition was achieved by adding 250 mg of particles daily in one of the 4-hour closed system period, while the food was added in the other 4 hours closed system period to avoid interference between particles and the added food. Water circulation and particle resuspension were achieved using recirculation pumps (skim350, Eheim), linked to a tubing circuit with outflow holes orientated towards the tank walls.
The concentration of suspended PMS and quartz particles in each treatment was measured during an exposure period of 4 hours. Suspended particle concentrations were measured one minute after particle addition, and then at intervals of 5, 15, 30 minutes, 1 hour, 2 hours and 4 hours after particle addition in the PMS and quartz particle treatments and in control aquaria with no particle addition. Samples of 0.5 L were collected from each aquarium 1 minute after particle addition with subsequent collection of 1 L seawater at other times. Seawater was filtered onto pre-combusted and pre-weighed 0.45 µm pore size GF/F filters, then washed with Milli-Q water to remove salt and dried at 60°C to constant weight. The concentration of suspended particles in the PMS and quartz particle treatments were estimated by subtracting the weight of filters with suspended particles in the control (no particle addition aquarium) for the weight of filters in the PMS and quartz treatments.
Seawater physical-chemical parameters were measured daily in each aquarium before feeding corals. Seawater salinity was measured with a S30 SevenEasy™ conductivity meter, pH and temperature with a glass electrode (Crison pH 25+), and oxygen with a Fibox4 (PreSens) with a Oxygen Dipping Probe DP-PSt3. Seawater samples (12 mL) for inorganic nutrient analyses were collected on times 0 (immediately before the start of the experiment), and once a week on days 6, 13, 20 and 27 of the experiment, filtered with 0.45 μm filters (Cytiva Whatman™ GD/X Glass Micro Fiber (GMF) syringe filters) and frozen until analyses. Nutrient concentrations were determined using a colorimetric Autoanalyzer Sanplus with Segmented Flow, applying the methodologies Skalar: M461-318 (EPA 353.2), M155-008R (EPA 350.1) and M503-555R (Standard Method 450-P I).
Trace elements (Co, Cu, Mn) released from the resuspension of PMS particles to the water column were determined using passive sampling coupled with inductively coupled plasma mass spectrometry (ICPMS). The passive samplers used were from DGT® Research Ltd (Lancaster, UK), with the reference LSNM-NP open-pore Loaded DGT device for metals in solution. DGT sequester dissolved labile metal species, including free ions, inorganic complexes and labile organic complexes (Zhang and Davison, 2000), a proxy for determining the potential biological adverse effects (Montero et al., 2012). DGT-holders were deployed in all aquaria and replaced every week (days 6, 13, 20, 27), which gave an integrated temporal variation of metal released to the water column. DGT samples were processed following the methodology described in Bersuder et al. (2021). Briefly, to minimize contamination, all the materials used were acid cleaned (pro-analysis grade), subsequently rinsed with ultra-pure water, and dried in rooms with environmental conditions limiting sample contamination. Powder-free gloves were also used to minimize contamination. DGT units were removed from holders, opened using a plastic screwdriver, and the resin-gel layer was peeled off retrieved with plastic tweezers. Metals from the gel were back-extracted with a 1 M HNO3 solution and measured by ICPMS. The final solution was kept refrigerated (4-6 °C) until analysis. Trace elements in the tissues and skeletons of corals in the different treatments (sampled at days 25-27 for PMS and day 27 for quartz and control treatments) were analyzed after digestion with a mixture of HNO3 and H2O2 to varying temperatures according to the method described by Raimundo et al. (2013). Certified reference material and procedural blanks (coral digestions) were taken through the procedure in the same way as the samples. Metal concentrations in DGT extracts and coral tissues were quantified by a quadrupole ICPMS (Thermo Elemental, X-Series) equipped with a Peltier impact bead spray chamber and a concentric Meinhard nebulizer. Measurements of the stable isotope indium (115In) were used as an online internal standard. The equipment was set up by ensuring low variability of counts (RSD<2%). Typically, 7-point standard calibration curves, including one blank, were used in different dynamic ranges depending on the metal concentration in extracts. Analyzed procedural blanks always accounted for less than 1% of the total element in the samples. The calculation of the DGT concentration was carried out following Zhang and Davison (2000) and using the diffusion coefficients provided by the supplier. The metal concentrations in coral tissues are given in microgram per gram of dry weight tissue (µg g-1; dw).
Coral fragments were examined using light microscopy and SEM to observe tissue and polyps’ condition and integrity under the different treatments. Light microscopy observations were made using a dissecting microscope Leica MZ 16FA under 30× magnification. For SEM, samples were dried using the critical point drying technique, mounted on stubs and sputter-coated with gold, and examined with a scanning electron microscope JEOL JSM-5200LV at 25 keV.
Integrated measurements of coral respiration and inorganic nutrients release rates were carried out by closed-chamber incubation in cylindrical acrylic chambers (volume 320 mL) on days 0, 13 and 27 of the experiment, except for the PMS particle treatment at day 27, when all corals in this treatment had died. Each coral fragment (N=6 per treatment) was incubated for 7-8 h with 0.2 µm filtered seawater from the respective aquarium and continuously stirred with glass-coated magnetic stirrers. Chambers were placed in a water bath, and temperatures maintained at 15 ± 0.3°C on top of a magnetic stirring plate. Respiration rates were derived from the depletion of dissolved O2 recorded before closing and immediately after the chamber using a single channel oxygen meter Fibox4 with a PSt3 sensor (PreSens, Germany). These values were adjusted for rates recorded in chambers without corals to account for microbial respiration. Oxygen saturation inside the chambers remained >70%. Dentomuricea aff. meteor excretion rates of ammonium, during incubations were assessed by calculating the difference between initial and final ammonium concentrations in chambers with and without corals. Nutrient concentrations were determined in 0.2 mL syringe filtered water samples using a colorimetric Autoanalyzer Sanplus with Segmented Flow, applying the described methodologies. Although ammonia (NH3) and ammonium were measured together in our samples, NH3 was considered as negligible in our conditions (1–2% of total ammonium/ammonia at pH 8.0, for a pKa of 9.68– 9.99, Bell et al., 2007). Coral respiration and excretion rates were normalized to the coral skeletal surface area quantified using advanced geometric techniques by approximation of the shape of different sections of the coral fragment to the geometric figure of a cylinder as described by Naumann et al. (2009).
Antioxidant stress related biomarkers in D. aff. meteor tissues were used to evaluate the degree of cellular stress induced by exposure to PMS and quartz particles. Coral fragments were collected from each treatment at times 0 and 13 days and from the control and quartz treatments also at time 27 days, snap frozen in liquid nitrogen, and stored at -80°C until analysis.
From each coral fragment from each treatment, tissues were separated from skeletons and homogenized at a 1:3 w/v ratio, in ice-cold Phosphate-buffered Saline solution (PBS pH 7.3: 0.14 M NaCl, 2.7 mM KCl, 8.1 mM Na2HPO4, 1.47 mM KH2PO4) and 1% (v/v) ProteaseArrest™ (G-Biosciences®), using an Ultra Turrax (Ystral®, D79282) slowly increasing the rotational velocity from 8,000 to 20,000 rpm, during the ~2 min extraction time. The homogenate was centrifuged at 16,000 g for 30 min at 4°C and supernatant fraction was separated into different microtubes and stored at -80 °C for posterior protein quantification and measurements of GST, SOD, CAT and LPO. All enzyme assays were tested with commercial kits obtained from Sigma® and each sample was run in triplicate in a Multiskan™ GO microplate spectrophotometer (Thermo Scientific™).
Protein quantification For enzyme activity normalization, the total soluble protein content was quantified according to the Bradford method (Bradford, 1976), adapted from Bio-Rad Bradford microassay set up in a 96-well microplate. Absorbance was read at 595 nm in a microplate reader (Thermo Scientific™). A calibration curve was created using bovine serum albumin (BSA; Bio-Rad) standards.
Glutathione S−transferase (GST) activity was determined according to the procedure described by Habig et al. (1974), and optimized for a 96-well microplate. This assay uses 1-chloro-2,4-dinitrobenzene (CDNB) as substrate, and upon conjugation of the thiol group of glutathione (GSH) to the CDNB substrate, there is an increase in the absorbance. The reaction product (GS-DNB conjugate), catalyzed by GST, absorbs at 340 nm. The rate of increase in the absorption is directly proportional to the GST activity in the sample. To perform the assay, 180 μL of substrate solution were added to 20 μL of GST standard or sample in each well and the absorbance at 340 nm was recorded every minute for 6 min using a plate reader (Thermo Scientific™). GST activity was calculated using a molar extinction coefficient for CDNB (ϵ= 5.3 mM−1 cm−1). Equine liver GST (Sigma-Aldrich) was used as a positive control to validate the assay. GST activity was calculated using the following equation: [ΔA340/min/0.0053]* [total volume/sample volume]. GST enzymatic activity in coral tissue is expressed as nmol min−1 mg−1 of protein (wet weight).
Superoxide dismutase (SOD) activity was determined spectrophotometrically by an indirect method (Therond et al., 1996) based on competition of SOD with 2-(4-iodophenyl)-3-(4-nitrophenol)-5-phenyltetrazolium chloride (I.N.T) for dismutation of superoxide anion (O-2). In this method, xanthine and xanthine oxidase were used to generate O-2 radicals which react with I.N.T quantitatively to form a red formazan dye. Absorbance was measured at 505 nm and 25 °C, 30 s after the addition of xanthine oxidase as start reagent across a 180 s incubation period (Kit Ransod SD 125, Randox). One unit of SOD is defined as the amount of enzyme that inhibits the rate of formazan dye formation by 50%. The percent inhibition of samples was calculated using the following equation: 100- [[ΔA505/min]/[ΔAblank/min]] *100. Then, a SOD standard curve is used to correlate the percent inhibition of samples with SOD activity. Coral tissue SOD activity is expressed as inhibition Units mg−1 of protein (wet weight).
Catalase (CAT) activity was measured spectrophotometrically, according to Beers and Sizer (1952), by measuring the rate of H2O2 disappearance at 240 nm (extinction coefficient, ϵ = 0, 04 mM−1 cm−1) and 25°C across a 180 s incubation period. In this assay, total reaction volume of 300 µL was obtained with 50 mM potassium phosphate buffer (pH 7.0), 13.5 mM H2O2 as a substrate and initiated by the addition of the sample into well plates. Catalase from bovine liver (Sigma®) was used as a positive control for validation of the assay. Catalase activity was calculated using the following equation: [ΔA240/min/0, 04]* [total volume/sample volume]. CAT enzymatic activity in coral tissue is expressed as μmol min−1 mg−1 of protein (wet weight).
Lipid peroxidation was determined by the quantification of a specific end-product of the oxidative degradation process of lipids, malondialdehyde (MDA) (Kalghatgi et al., 2013). Concentrations of MDA were analyzed using a colorimetric reaction, which uses 1-methyl-2-phenylindole (MPI) as chromogen (Randox Ltd.). Condensation of one molecule of MDA with 2 molecules of MPI under acidic conditions results in the formation of a chromophore with an absorbance maximum at 586 nm. Concentrations of MDA in each tissue were calculated using a standard curve prepared with freshly prepared solutions of malondialdehyde bis [dimethyl acetal] (ACROS Organics™) and values were expressed as nmol mg−1 of protein (wet weight).
Gene expression profiles in D. aff. meteor were used to evaluate the physiological pathways involved in the response to exposure to PMS and quartz particles. Coral fragments were collected from each treatment at times 0, 3 and 13 days and for the control and quartz treatments also at time 27 days, snap frozen in liquid nitrogen and stored at -80°C until subsequent analysis. The sampling time point at day 3 of the experiment was performed to capture changes of gene expression reflected within a short period of exposure to the treatments. Total RNA extraction and further RNA handling until obtaining cDNA from six coral fragments of the same treatment, followed procedures described in Carreiro-Silva et al. (2014). A set of genes regarded as suitable molecular biomarkers were selected to detect changes at sub-cellular level in response to different treatments based on previous studies with cold-water corals in our laboratory (Carreiro-Silva et al., 2014; Servetto et al., 2021): genes involved in cellular stress and antioxidant reaction system (heat shock protein (HSP70), superoxide dismutase (SOD), ferritin), cell structure/integrity [α-carbonic anhydrase, receptor-type protein tyrosine phosphatase (RP Tyr-PH)] and immune responses (toll-like receptor (TLR), lysozyme, rel homology domain (RHD), ferritin). See Table S1 for details on the biological function of each of the protein-encoding genes used in this study. Specific primers amplifying 100–200 bp PCR products were designed for the genes of interest (GOI) based on the nucleotide sequences used in previous studies (see Table S1). Because the selected gene sequences have still not been published for D. aff. meteor, the identity of the sequences was confirmed by polymerase chain reaction amplification followed by sequencing of the PCR products. cDNA samples of D. aff. meteor (40 ng) were amplified in the presence of Reaction Buffer 1X; 1.5 mM MgCl2; 0.2 mM dNTP mixture; 1 µM of each design primer (sense and antisense); and 2.5 U of Taq DNA Polymerase (Invitrogen). The amplification reaction was performed using the Eppendorf MastercyclerR gradient and the following conditions: 5 min at 95°C, followed by 35 cycles of 1 min at 94°C, 1 min at 54°C and 1 min at 70°C, with a final extension of 10 min at 72°C. PCR products were directly extracted from agarose gels and their nucleic acid sequence determined. BLAST analyses were performed to verify the corresponding matching PCR products sequences to the candidate gene sequences present in GenBank database, in order to confirm their identity (Table S1). Transcript levels were determined by Real-Time PCR using the CFX Connect™ Real-Time PCR Detection System (BioRad). First cDNA strand diluted 1:200 aliquots from each sample were used in 20 µL PCR reactions in the presence of 0.5 µM of each primer and Maxima SYBR Green/ROX qPCR Master Mix (Fermentas). The thermocycling protocol was as follows: 10 min at 95°C, followed by 40 cycles of 20 s at 94°C, 45s at 54°C and 25 s at 68°C. A melting curve of PCR products (95°C – 65°C) was performed to ensure the presence of single PCR producst at the end of the qPCR reactions. For the evaluation of the expression level of each mRNA used, the comparative Ct (ΔΔCt) quantitation method was applied, using 18S and β-actin genes as suitable reference genes for their efficiencies and stable expression across all experiments. Each reaction was repeated in triplicate, and negative controls were performed without cDNA in the reaction mixture. Target mRNA genes were normalized using the combination of the expression levels with 18S and β-actin and relative abundances were calculated in relation to gene expression levels just prior to the start of the experiment (T0). The expression level of all genes was converted into relative expression level as follows:
where
Multivariate analysis was performed using the statistical package PRIMER-E v.6 with the permutational multivariate analysis of variance (PERMANOVA) + add on (Anderson et al., 2008) to investigate the response of D. aff. meteor to treatments and through experimental times. This statistical analysis is a powerful non-parametric approach that uses a permutational technique to enable significance tests for small sample sizes to be conducted (Walters and Coen, 2006). The analyses used a resemblance matrix based on Euclidean distance and PERMANOVA was run using 9999 permutations with partial sum of squares and unrestricted permutation of raw data to produce p-values. A repeated measures PERMANOVA was used to test for treatment and experimental time effects on D. aff. meteor respiration and excretion rates and oxidative stress biomarkers. ‘Treatment’ and ‘time’ were used as fixed factors, with interaction terms, whereas corals were used as a random factor to account for repeated measures and mother colony donors. To test for differences in dissolved metal concentrations among treatments and experimental times, the analysis used ‘treatment’ and ‘time’ as fixed factors. To test for differences in total accumulated metal concentrations in corals at the end of the 27 days period, individual metal concentrations measured in coral tissues and skeletons were analysed using a one-way PERMANOVA with ‘treatment’ as a fixed factor. Preliminary data analysis showed that the effect of the nested factor aquaria was low (p > 0.1), thus, in the analysis of the data, replicate aquaria within each treatment were pooled together to increase the power of the test for sediment exposure (Underwood, 1997). Values were considered statistically significant at p< 0.05. No statistical tests were conducted for differences in gene expression fold changes, because of the small number of replicates and high variability. Significant main PERMANOVA tests were followed by pairwise PERMANOVA tests. Permutational P-values (PPERM) were interpreted when the number of unique permutations >100; alternatively, Monte Carlo P-values (PMC) were considered (Anderson and Robinson, 2003). Reported values in the text are mean ± standard deviation.
Monitoring of the concentration of suspended PMS and quartz particles in each treatment revealed that average exposure concentrations differed from the target value of 25 mg L-1 (Figure S2). Suspended PMS particle concentrations were 17 ± 3 mg L-1, 1 minute after particle addition, decreasing to values of 2-3 mg L-1 after 1 hour that remained constant during the remaining exposure time of 4 hours (Figure S2). In the quartz particles treatment, average concentrations were 24 ± 2 mg L-1, 1 minute after particle addition, reaching values of 18 ± 3 mg L-1 after 2 hours and 15 ± 0.4 mg L-1 after 4 hours (Figure S2). Addition of PMS particles slightly changed the pH of aquaria by 0.2-0.6 units for the first 2-3 minutes but returned to “control” values after this short period, with no measurable changes in dissolved oxygen. Physical-chemical conditions for the experimental aquaria over the 27 days remained within the same range for all treatments (Table S2). Examination of particles under light and scanning electron microscopy showed that PMS particles had a more angular shape than quartz particles (Figure 1).
Figure 1 Light microscopy and scanning electron micrographs of the (A, C) polymetallic sulphide particles and (B, D) quartz particles used in the exposure experiment.
Measurements of metal content in seawater in the different experimental treatments showed increased concentration of trace elements (Co, Cu, Mn) released from the resuspension of PMS particles to the water column (Figure 2). Copper showed the highest concentration in seawater with values between 6.6 ± 1 μg L-1 in the control and quartz treatments and 22 ± 8 μg L-1 in the PMS treatment. The concentration of Mn varied between 0.13 ± 0.05 μg L-1 for the control and quartz treatments and 0.26 ± 0.07 μg L-1 in the PMS treatment, while Co concentrations varied between 0.045 ± 0.008 μg L-1 in the control and quartz treatments and 0.26 ± 0.09 μg L-1 in the PMS treatment. The increased dissolved Co, Cu and Mn concentrations in the treatments with PMS were statistically higher than the control and quartz particle treatments (main PERMANOVA, all P<0.05; pairwise tests Co t=6.36-6.53, p<0.05; Cu t=4.97, p<0.05; Mn t=3.40-4.78, p<0.05, Table S3). These concentrations remained consistently higher in the PMS than in other treatments throughout the experiment, with no significant difference among sampling periods (PERMANOVA interaction term, p(perm)>0.05, Table S3).
Figure 2 (A-C) Trace metal concentration in seawater in the treatments with polymetallic sulphide particles (PMS), with quartz particles (Quartz) and a control treatment with no sediment addition at times 6,13, 20 and 27 days of the experiment. N=2, data expressed as mean ± standard deviation.
Although polyp extension behaviour of D. aff. meteor was not quantified, polyps were observed to be more fully extended in the control and quartz particles treatments than in the PMS particle treatment (Figure 3). Corals exposed to PMS particles experienced a progressive accumulation of PMS particles on D. aff. meteor fragments. Small PMS particles tended to form aggregates (Figure 1A) that appeared to coat the coral fragments covering first the coenenchyme surface with some polyps still visible during the first 3-5 days (Figure 3C) but later covering the polyps as well. Examination of coral fragments under light microscopy revealed very small PMS particles covering the polyps of coral fragments, suggesting high adherence of these particles to coral tissues and clogging of polyps (Figures 4A-C). Coral exposed to the quartz particles treatment experienced a general paling of their tissues through time of exposure in comparison with coral in the control treatment, whereas coral in the PMS treatment experienced a darkening and sloughing of the tissue through time, with visible portions of their skeletons in some fragments. Further examination of corals under scanning electron microscopy revealed undamaged tissues and polyps in the control and quartz particles treatments and what appeared as coral tissue degeneration in the PMS treatment (Figures 4D-F).
Figure 3 Dentomuricea aff. meteor fragments exposed to the different treatments (A) Control; (B) Quartz particles; and (C) Polymetallic sulphide particles, after 3 days of treatment exposure.
Figure 4 Tissue and polyps condition of Dentomuricea aff. meteor after 13 days exposure to the different treatments. (A, D) Control treatment; (B, E); Quartz particle treatment; (C, F) Polymetallic sulphide treatment. Photographs A, B, C taken under the dissection microscope; photographs (C-E) taken under the scanning electron microscope.
High mortality was observed in the corals exposed to PMS particles after 13 days (36%), 20 days (80%) and 25 days (95%). The experiment was terminated at time 27 days when all coral fragments were either sampled or dead. While no mortality was noted for D. aff. meteor exposed to inert particles until the end of the 27 days experiment, unexpected mortality was found for one control tank between days 8 and 13. This mortality was only found in this tank and identified as a result of a technical malfunction of the recirculating pump which decreased circulation of the incoming water leading to the observed coral mortality.
Similarly to what was found for the metal content in seawater in the different experimental treatments, Cu presented the highest concentrations in coral tissues exposed to PMS particles (main PERMANOVA, Pseudo-F2,12 = 19.25, p(perm)=0.0016, Figure 5A). Copper concentration in coral tissues after 25-27 days were 13 times higher in the PMS (274 ± 122 µg g-1) than control and quartz treatments (22 ± 7 and 25 ± 8 µg g-1, respectively) (pairwise tests, PMS-Control t=4.24, p(mc)=0.0088, PMS-Quartz t=4.80, p(mc)=0.0026). In addition, concentrations of Cu in coral skeletons (184 ± 112 µg g-1) were 4 to 5 times greater than control and quartz particles treatments (55 ± 32 and 35 ± 12 µg g-1, respectively) (PERMANOVA, pseudo-F2,12 = 6.80, p(perm)=0.0016, pairwise tests, PMS-Control t=4.12, p(mc)=0.028, PMS-Quartz t=4.64, p(perm)=0.008). Concentrations of Mn in coral tissues and skeletons remained within similar levels in all treatments (range 0.7-2.2 for tissues and 0.08-0.76 µg g-1 for skeletons, main PERMANOVA coral tissue pseudo-F2,12 = 0.23, p(perm)=0.7989; coral skeleton pseudo-F2,12 = 0.65, p(perm)=0.5414, Figure 5B). Cobalt concentrations in coral tissues were comparable among treatments (range 0.8-1.9 µg g-1, main PERMANOVA, pseudo-F2,12 = 2.10, p(perm)=0.1376, Figure 5C), but were significantly higher in coral skeletons of coral in the PMS treatment (0.60 ± 0.19 µg g-1) when compared to the quartz treatment but not the control treatment (0.31 ± 0.09 and 0.33 ± 0.11 µg g-1, respectively) (main PERMANOVA, pseudo-F2,12 = 6.07, p(perm)=0.02; pairwise test PMS-Quartz t=3.02, p(mc)=0.0232, Table S3).
Figure 5 (A-C) Metal accumulation in tissue and skeleton fractions of Dentomuricea aff. meteor exposed to the treatments with polymetallic sulphide particles (PMS), with quartz particles (Quartz), and a control treatment with no sediment addition, at times 25-27 days for PMS and 27 days for control and quartz. N=4-5, data presented as µg−1 dry weight tissue or skeleton. The line in the box is the median, and the X is the mean. Whiskers represent the highest and lowest values and outliers are identified by a circle (○).
The permutational MANOVA revealed that responses of respiration rates to the treatments varied with experimental time (interaction term Pseudo-F3,37 = 7.68, p(perm)=0.002, Table S4). D. aff. meteor average respiration rates at time 0 (just prior to the start of the experiment) varied between 1.27 ± 0.31 μmol O2 cm2 coral surface area day–1 in the quartz treatment and 1.74 ± 0.89 μmol O2 cm–2 coral surface area day–1 in the control treatment (Figure 6A), with no statistical differences between treatments (pairwise comparisons, t=0.65-1.23, p(mc)>0.05). After 13 days of treatment exposure, respiration rates in the PMS particle treatment increased 2 times (2.98 ± 0.61 μmol O2 cm–2 day–1) in comparison to baseline values at time 0 (pairwise comparisons t=5.02, p(perm)=0.009), while respiration rates in other treatments did not vary significantly between time 0 and 13 days (pairwise comparisons, t=0.27-0.40, p(mc)>0.05). Dentomuricea aff. meteor respiration rates were 1.6 to 2 times higher in the PMS than the control and quartz particles treatments at this sampling time (pairwise comparisons PMS-Control t=3.95, p(mc)=0.005; PMS-Quartz t=4.87, p(perm)=0.002). No significant differences were found in D. aff. meteor respiration rates between the control and quartz particles treatments at the end of the experiment (pairwise comparison t=0.545, p(mc)=0.603), while all D. aff. meteor used in the closed-chamber incubations had died in the PMS particle treatment by this sampling point.
Figure 6 Physiological measurements on the cold-water octocoral Dentomuricea aff. meteor. (A) respiration and (B) ammonium excretion rates during the experiment (times 0, 13, 27) in treatments with polymetallic sulphide particles (PMS), with quartz particles (Quartz), and a control treatment with no sediment addition. Data normalized to coral surface area. N=3-6, no fragments in the PMS treatment at time 27 days. The line in the box is the median, and the X is the mean. Whiskers represent the highest and lowest values and outliers are identified by a circle (○).
Ammonium released by D. aff. meteor varied significantly between treatments depending on experimental time (PERMANOVA, interaction term Pseudo-F2,38 = 3.10, p(perm)=0.0476, Table S4; Figure 6B). Ammonium excretion rates did not differ significantly between treatments at time 0, with recorded average excretion rates between 0.21 ± 0.14 and 0.36 ± 0.26 μmol cm-2 coral surface area day-1 in the quartz particles treatment and control treatment respectively (pairwise comparisons, t=0.70-1.21, p(mc)> 0.05). At time 13, ammonium excretion rates in the PMS treatment increased 2 times (0.56 ± 0.19 μmol cm-2 day-1) in comparison to baseline values (T0) (0.27 ± 0.19 μmol cm-2 day-1, pairwise comparison, t=2.69, p(perm)=0.0456). Excretion rates at this time were 5 times greater in the PMS than the quartz treatment (0.11 ± 0.12 μmol cm-2 day-1) (pairwise comparisons, t=4.83, p(perm)=0.0027) but not significantly different in the PMS compared with the control treatment (0.43 ± 0.07 μmol cm-2 day-1) (pairwise comparison, t=1.09, p(perm)=0.3172). By the end of the experiment (day 27), ammonium excretion decreased in both the control and quartz treatments in comparison with day 13, with excretion rates in the latter treatment reaching significantly lower values than the control treatment (0.006 ± 0.007 and 0.21 ± 0.05 μmol cm-2 day-1, respectively), (pairwise comparison, t=9.85, p(mc)=0.0001). At this time, all D. aff. meteor used in the closed-chamber incubations had died in the PMS particle treatment.
The activity of the enzyme Glutathione S−transferase (GST) was not different among treatments but marginally increased between time 13 and 27 of the experiment in the quartz treatment (main PERMANOVA time factor pseudo-F=6.91, p(perm)=0.0331; pairwise comparison t=2.55, p(perm)=0.0513, Figures 7A). The activities of the enzymes superoxide dismutase (SOD) and catalase (CAT), and concentration of malondialdehyde (MDA) were not significantly different among treatments and sampling periods (main PERMANOVA pseudo-F=0.04-2.10, p>0.05, Table S5; Figures 7, 8). It was not possible to assess cellular response in corals exposed to PMS treatment at time 27 days due to their death.
Figure 7 Enzyme activities of (A) Glutathione S−transferase (GST), (B) superoxide dismutase (SOD) and (C) catalase (CAT) in Dentomuricea aff. meteor tissues during the experiment (times 0, 13, 27) in treatments with polymetallic particles (PMS), with quartz particles (Quartz) and in the control treatments with no sediment addition. N=3-6, no fragments in the PMS treatment at T27. The line in the box is the median, and the X is the mean. Whiskers represent the highest and lowest values and outliers are identified by a circle (○).
Figure 8 Concentrations of malondialdehyde (MDA) in Dentomuricea aff. meteor tissues during the experiment (times 0, 13, 27) in treatments with polymetallic particles (PMS), with quartz particles (Quartz) and in the control treatments with no sediment addition. N=3-6, no fragments in the PMS treatment at T27. The line in the box is the median, and the X is the mean. Whiskers represent the highest and lowest values and outliers are identified by a circle (○).
During the first 13 days of the experiment, targeted genes were generally downregulated in relation to expression levels at time 0 (T0) just prior to the start of the experiment (relative fold change <1, Figure 9). However, by the end of the 27 days exposure, several genes involved in cellular stress (HSP70, ferritin), immune response (RHD, ferritin), antioxidant defense (SOD) and in cell cycle control (RP Tyr–PH) were upregulated in the quartz treatment in relation to T0 values (relative fold change >1, Figure 9). The genes encoding for ferritin, RP Tyr-PH and RHD were also upregulated in the control treatment by the end of the 27-days period. It was not possible to assess gene expression response in corals exposed to PMS treatment at time 27-days due to their death.
Figure 9 Relative gene expression of selected genes for the Dentomuricea aff. meteor during the experiment (times 3, 13, 27) in treatments with polymetallic particles (PMS), with quartz particles (Quartz) and in the control treatments with no sediment addition. Values are means of the relative gene expression normalized with the expression of two reference genes - 18S and β-actin - and relative to expression levels in T0, just prior to the start of the experiment. Expression levels (fold change) for the different target genes, Lysozyme, RHD (Rel Homology Domain), TLR (Toll-like receptor), HSP70 (heat shock protein 70), RP Tyr-PH (receptor-type protein tyrosine phosphatase), SOD (Superoxide Dismutase), Ferritin and Carb Anhy (alpha-carbonic anhydrase) are represented in different colour bars. Data expressed as mean ± standard error. Three technical replicates and n=6 for all treatments at times T3 and T13 days, n=2 for control treatment at T27, no fragments in the PMS treatment at T27.
Using a controlled aquaria experiment, we have observed how exposure to suspended polymetallic sulphide particles (PMS) from inactive chimney crushed rocks affected an important habitat-forming octocoral in the Azores, Dentomuricea aff. meteor. The two particles treatments, PMS and inert quartz particles, allowed us to explore the potential mechanical and toxicological effects of particles on corals. We attempted to mimic a dewatering sediment plume scenario that exposed corals to a PMS particle concentration of 25 mg L-1. Nevertheless, due to the high density of PMS particles, a high proportion (92%), likely the larger size fraction, settled at the bottom of the aquaria, with only 2-3 mg L-1 of the smaller size fraction remaining in suspension after 1 hour of particle addition. Quartz particles added to the experimental aquaria also partly settled at the bottom of the aquaria, but 60-70% (15-18 mg L-1) remained in suspension during the exposure period.
Despite discrepancies between the target and achieved concentration of suspended particles, low suspended particles values (1.2-4 mg L-1) are the most widespread concentrations projected by model simulations of the dispersal of PMS dewatering sediment plumes in Mid Atlantic Ridge around the Azores (Morato et al., 2022). Their simulations revealed that, while there are projected marked differences among sites, making generalizations of dewatering plume dispersal patterns difficult to make, the probability of having sediment plumes above 25 mg L-1 threshold (our initial concentration) would likely be restricted to a small area of about 0.6 km2 around the discharge points. Instead, the dispersal of plumes with 2-3 mg L-1 (our achieved concentration) is predicted to reach an area from 25 to 150 km2 (Morato et al., 2022). This suggests that our study truly simulated a scenario of particle emissions, where most of the particles settle close to the discharge point and only a small fraction travels to distant areas influencing other neighboring environments. Despite the low concentrations of PMS particles in suspension, our results show that D. aff. meteor was very sensitive to resuspension of PMS particles, with the death of all coral fragments by the end of the 27-day exposure. Coral fragments exposed to suspended quartz particles also demonstrated physiological impairment but no coral mortality was found until the end of the experiment. The lethal and sublethal responses involved in these different results are discussed below.
The two types of particles used in the present study, PMS and quartz, differed in mineralogy, elemental composition and grain shape, which influenced the different responses of D. aff. meteor to the experimental treatments. Polymetallic sulphide particles had a more angular shape and were partly composed by toxic trace metals (mainly Cu), while quartz particles were rounder and did not contain metals. Corals exposed to PMS particles experienced a rapid accumulation of particles in their tissues, resulting in the reduction of polyp expansion behavior and feeding activity within only a few days (3-5 days) from the start of the experiment (Figure 3). This was followed by the progressive necrosis and loss of tissue, and death of all the corals fragments after 27 days in the PMS treatment. In contrast, even though some polyp retraction and tissue paling resulting from the deposition of quartz particles were also noticed in the treatment with quartz particles, polyps regularly opened in response to food and there was no coral mortality.
The differential responses of corals to different types of sediments have been reported in several studies with tropical and cold-water corals. Studies with tropical corals showed that sandy grain size fractions are rejected more effectively than nutrient-rich fine sediments (mud and silt-sized), possibly due to the greater stickiness (adhesion) of the latter (Fabricius and Wolanski (2000); Weber et al., 2006), with the accumulation of fine sediments ultimately resulting in the smothering and death of corals. The stickiness (adhesion) effect of barite has also been reported for L. pertusa, with particle accumulation in the dead portions of coral skeletons, whereas natural sediments were more easily removed (Larsson and Purser, 2011; Larsson et al., 2013). In the present study, the high adhesion of PMS particles to coral tissues likely hampered proper rejection of particles by D. aff. meteor, with the progressive “coating” of the coral fragments, while quartz particles were more easily rejected by the corals. Polyp retraction observed in D. aff. meteor may have conferred some protection to reduce the risk of sediment abrasion (Rogers, 1990; Erftemeijer et al., 2012), but the absence of substantial mucus production to actively reject particles, may have contributed to the rapid accumulation of PMS particles. Mucus has been shown to be important for the removal of drill cuttings in the scleractinian L. pertusa (Larsson and Purser, 2011; Larsson et al., 2013; Allers et al., 2013; Zetsche et al., 2016; Baussant et al., 2018; da Rocha et al., 2021) and in the alcyonacean Primnoa resedaeformis (Liefmann et al., 2018), reducing the risk of damage and particle accumulation on coral tissues. Thus, the different ability of corals to produce mucus could potentially lead to species-specific responses to mining impacts.
At the same time, based on SEM examinations, the sharp shape of the PMS appeared to cause degeneration or breakage of D. aff. meteor tissues (Figure 4). Injuries inflicted by sharp-edged mine tailings have also been observed in Duva florida and P. resedaeformis using histological analysis, while no damage was observed from smooth glass beads (Liefmann et al., 2018). Artic-boreal deep-water sponges were also found to be more sensitive to sharper drill cuttings (barite particles) than to natural occurring sediments (Kutti et al., 2015; Edge et al., 2016; Fang et al., 2018), with negative impacts likely resulting from a combination of the sharp characteristics and toxicity of barite particles (Edge et al., 2016).
Exposure of D. aff. meteor to dissolved metals in the PMS treatment, resulting from the oxidation of sulphides in contact with seawater (Fallon et al., 2019), caused significant bioaccumulation of Cu in its tissues and skeletons (Figure 5). Trace metals can be mainly incorporated into corals by a combination of uptake of seawater-soluble metals and via polyp feeding (Ferrier-Pagès et al., 2005). Higher bioaccumulation of metals in tissues in comparison to skeletons, such as the ones observed here (274 ± 122 µg g-1 and 184 ± 112 µg g-1 of Cu in the tissues and skeleton, respectively) has been reported in several studies (e.g. Esslemont et al., 2000; Reichelt-Brushett and McOristb, 2003). This phenomenon has been explained by the primary incorporation of metals into tissues and subsequent transfer from the tissue to the skeleton (Harland and Brown, 1989; Ferrier-Pagès et al., 2005) and/or discrimination against metals in coral’s biogenic precipitation of the skeleton (Reichelt-Brushett and McOristb, 2003).
Values of Cu recorded for corals exposed to PMS were several orders of magnitude higher than those recorded in tropical coral species in metal-polluted areas (1-63 µg.g-1: Brown and Holley, 1982; Sabdono, 2009; Mohammed and Dar, 2010; Ali et al., 2011; Berry et al., 2013), and corals experimentally exposed to copper (Bielmyer et al., 2010; Chan et al., 2012; Siddiqui and Bielmyer-Fraser, 2015). Mucus production by cnidarians has been described as an important response to prevent metal uptake in corals exposed to Cu (Reichelt and Jones, 1994; Mitchelmore et al., 2003; Mitchelmore et al., 2007). The lack of substantial mucus production generally observed for the species D. aff. meteor may explain the extensive accumulation of Cu in coral tissues and skeletons under PMS particle exposure. However, it should be noted that although care was taken to remove PMS particles attached to coral tissue, it is possible that very small particles remained attached and may have contributed to the high Cu concentrations reported in coral tissues.
Copper concentrations in D. aff. meteor under control and quartz conditions (22 ± 7 and 25 ± 8 µg g-1 respectively), although up to 10 times lower than those recorded in the PMS treatment, were higher than concentrations recorded as baseline values for this species in the Azores region (2.4-4.3 µg.g-1, Raimundo et al., 2013). However, Raimundo et al. (2013) reported Cu concentrations for the whole individual, whereas in the present study trace metals were analyzed in tissue and skeleton, separately. These differences may also be associated with the collection site of the corals, reflecting a natural input of this element, presumably from hydrothermal origin.
Physiological impacts of PMS particles were noted by the significant increase in respiration (a proxy of basal metabolism) and ammonium excretion rates after 13 days of coral exposure to PMS particles, in comparison with other treatments (Figure 6). Previous studies on the effects of suspended particles on corals reported differing effects on respiration rates. Increased respiration has been related to increased polyp activity or increased mucus production in shallow-water scleractinian corals and the deep-water octocoral P. resedaeformis (Abdel-Salam et al., 1988; Telesnicki and Goldberg, 1995; Scanes et al., 2018). Reduced respiration rates have been related to reduced polyp activity or to tissue loss in other shallow-water scleractinian and alcyonacean corals (Riegl and Branch, 1995; Riegl and Bloomer, 1995). In contrast, no significant effects of drill cuttings on respiration were reported for L. pertusa (Larsson et al., 2013; Baussant et al., 2018), suggesting physiological resilience of this species to drill cutting exposure.
In our study, increased respiration cannot be explained by increased polyp activity or increased energetic costs of mucus production. It could, instead, reflect physiological stress to the toxicity of Cu that bioaccumulate in coral tissues and to the mechanical injury caused by particles, as exposure to metals, such as Cu, may increase energy costs for detoxification, cellular protection and repair (Rainbow, 2002). Increased respiration rates in response to Cu toxicity have been previously recorded for the coral reef symbiont bearing foraminifer Amphistegina gibbosa (Marques et al., 2020) and recently for the the octocoral Viminella flagellum in the Azores (Martins et al., 2022). Other studies, however, showed that Cu may compromise energy production by inhibiting energy metabolism enzymes in tropical corals (Fonseca et al., 2019; Fonseca et al., 2021). Contrasting responses to Cu in these studies may reflect inter-specific variability. However, it should be noted that it was not possible to remove PMS particles encrusted in tissues of coral used in respiration incubations. Therefore, we cannot rule out the possibility that increased oxygen consumption could also resulted from increased activity of microorganisms associated with PMS particles or oxidation of redox-sensitive elements in particles.
Animals produce ammonia mainly as a byproduct of the catabolism of protein and amino acids (Baldwin, 1964). Carbon –rich lipids and carbohydrates are the main general sources of energy used by organisms, and nitrogen-rich proteins will only be used when lipids and carbohydrates (glycogen reserves) are exhausted, resulting in increased nitrogen excretion (Gabbott, 1983). Therefore, the 2-5 fold increase ammonium excretion in corals exposed to PMS particles after 13 days exposure, could signal a transition from carbohydrate and lipid reserves to the catabolism of tissue proteins (Baldwin, 1964). Ammonia excretion rate varies with the nutritional state of the individual (Baldwin, 1964) and has been reported to increase with starvation in echinoderms and molluscs (Diehl and Lawrence, 1979; Okumura et al., 2002). Although we did not measure feeding rates in the present study, coral polyps under the PMS treatment were clogged with particles (Figure 4C), preventing corals from feeding, possibly requiring the use of proteins in body tissue of D. aff. meteor for energy production. Disturbances to energy metabolism under conditions of metal exposure have been reported for coastal and deep-sea mussels (Canesi et al., 2007; Zhou et al., 2021), with organisms enhancing their energy requirements by utilizing existing carbohydrate reserves to counteract the toxicity posed by metals (Canesi et al., 2007). In the present study, the increased ammonia excretion in D. aff. meteor tissues may indicate a disturbance in energy metabolism induced by Cu toxicity.
The potential effects of metals accumulated in coral tissues were evaluated through cellular stress biomarkers and gene expression profiles. Copper is required at trace concentrations for many cellular processes and physiological functions as cofactors of enzymes (Rainbow, 2002). However, Cu concentrations above ambient concentrations have been reported to cause physiological impairment in corals and other cnidarians (Bielmyer et al., 2010; Marangoni et al., 2017; Fonseca et al., 2019). At the cellular level, excess in Cu has been shown to cause oxidative stress through the generation of oxygen reactive species (ROS) and subsequent damages to cells (Mitchelmore et al., 2007; Schwarz et al., 2013; Siddiqui and Bielmyer-Fraser, 2015). Thus, the examination of antioxidant enzymes such as superoxide dismutase (SOD), catalase (CAT) and glutathione peroxidase (GPx) used to neutralize the harmful effects of ROS (Lushchak, 2011), provides a useful diagnosis to detect physiological effects of metals on corals (Downs et al., 2012).
Our study showed that the increase in Cu concentration in coral tissues was not followed by significant changes in anti-oxidant stress enzyme activities (GST, SOD and CAT) in the PMS particles treatment (Figure 7). In addition, gene expression of enzymes involved in cellular stress (HSP), antioxidant (SOD), and immune defense (ferritin, RHD, TLR) in corals exposed to PMS were generally downregulated in relation to expression levels just prior to the start of the experiment (T0) (Figure 9). This suggests a general physiological reaction marked by the inhibition of the cellular stress response of D. aff. meteor caused by exposure to PMS particles. This inhibitory response was rather fast during the course of the experiment, to an extent which toxic metals exposure may have caused a systemic damage to the whole organism, leading to mortality after 4 weeks.
Studies with marine invertebrates showed that while exposure to metals generally activates the enzymatic antioxidant defense system, excessive levels of ROS induced by copper toxicity can promote enzymatic inactivation when cellular defenses are unable to maintain the oxidant-antioxidant balance (Regoli and Giuliani, 2014; Zhou et al., 2021). Moreover, excessive presence of ROS may directly affect the signal transduction pathways regulating the transcriptional activity of antioxidant and immunity genes to an extent where the cellular integrity is no longer maintained (Rhee et al., 2013), and the molecular mechanisms of copper immunotoxicity seriously compromises the animal survival (Nguyen et al., 2018).
In contrast to coral response to PMS particles, corals exposed to quartz particles displayed an increasing trend, although not statistically significant, in CAT activity in relation to control levels by the end of the experimental period (Figure 7). This response was also accompanied by lipid peroxidation, as indicated by slightly higher MDA concentrations in this treatment (Figure 8). Lipid peroxidation generally indicates that levels of ROS are overwhelming the antioxidant pathways, accumulating and damaging cellular membrane lipids, thus indicating ongoing oxidative stress (Lesser, 2006). Therefore, the general loss of tissue condition (paling and thinning of tissue) observed in the quartz treatment could reflect this cellular response. The upregulation of genes involved in cellular stress (HSP70) and in immune response (Ferritin and RDH) could also reflect the worsened coral condition during exposure to quartz particles (Figure 9). Although the oxidative stress response to sedimentation exposures, or increased suspended particles, has seldom been studied, a similar response of oxidative stress (CAT increase) has recently been reported for the tropical scleractinian coral species Porites astreoides from South Florida coral reefs, in response to experimental exposure to sediments (Rushmore et al., 2021).
The present study included two particles treatments, PMS and inert quartz particles, to distinguish potential toxicological and mechanical effects of PMS particles. Our results showed that PMS particles induced physiological changes in the energy metabolism of D. aff. meteor, while changes in the cellular stress biomarkers and gene expression profiles were more pronounced in corals exposed to quartz particles. This suggests that the mechanical effect of quartz particles, although not causing measurable changes in the physiological functions of the coral over the 4 weeks experiment, can still elicit cellular stress and immune responses, possibly as a reaction to the particle abrasive effect on coral tissues. These cellular responses together with other observed sub-lethal effects, such as loss of tissue coloration and a progressive reduction in polyp activity towards the end of the experiment, indicate an increasing decline of coral health to exposure to quartz particles that could be potentially damaging beyond the experimental period.
Although it is not clear why a rapid death of D. aff. meteor was observed upon PMS particles exposure, a probable mechanistic explanation may rely on the combined and potentially synergistic mechanical and toxicological effects of these particles. Previous studies on the lethal and sub-lethal toxicological effects of Cu added to D. aff. meteor in dissolved form (Martins et al., 2018) revealed that dissolved concentrations twice as high as to the ones recorded in the PMS treatment (60 µg. L-1), did not cause death, in spite of the inflicted physiological stress. Thus, it is plausible that D. aff. meteor death in our experiment may have resulted from a combination of factors and not solely from the exposure of high Cu levels.
While metal toxicity may have contributed to the physiological changes in the energy metabolism by increasing the energy costs for detoxification and protection (Rainbow, 2002), the high adhesion of PMS particles to coral tissues also likely contributed to the reported metabolic responses. PMS particles coated the polyps and coenenchyme surface, preventing the coral from extending its polyps and feeding, which over time resulted in changes in energy metabolism and ultimately in the smothering and death of coral tissue. At the same time, the angular shape of PMS particles inflicted injuries on coral tissues further contributed to tissue degeneration and necrosis. As reported for tropical corals, tissue damage from sediment particles might leave the corals vulnerable to bacterial attack and subsequent tissue necrosis (Harvell et al., 2007).
One potential additional cause for the rapid tissue deterioration and death in the PMS particles that was not examined in the present study is the possibility that the accumulation of PMS particles at the coral tissue surface might have created a sulphur-rich micro-environment favorable to bacteria growth. The presence of bacteria was detected by scanning electron microscopy examination of coral tissues in the PMS treatment (M Carreiro-Silva, unpublished data). A similar situation has been reported for tropical coral exposed to high sediments enriched with organic matter (Weber et al., 2012) and the cold-water coral L. pertusa exposed to sediments from Tisler reef (Allers et al., 2013). In both these cases, sediment coverage gradually decreased oxygen at the coral surface with release of hydrogen sulphide, presumably by sulfate-reducing microbial communities associated to sediments. Weber et al. (2012) showed how the organic matter in the sediments triggered microbial decomposition of coral tissues and mucus, with concomitant release of hydrogen sulfide that diffused to neighboring tissues leading to their damage and death. In the present case, the sulphur in the PMS particles might have directly induced bacteria growth. Hydrogen sulfide concentration can be highly toxic to corals leading to tissue damage and increased mortality (Bagarinao, 1992).
Future studies using microsensors to closely monitor changes in oxygen, pH and hydrogen sulphide at the surface of the coral following PMS particle coverage may elucidate whether the rapid death of corals under PMS exposure was microbial-mediated (Weber et al., 2012).
Deep-sea mining is listed as one of the major potential stressors on the marine environment (Jones et al., 2019). Contrary to other ocean-based industries such as oil and gas, that have decades of implementation, the deep-sea mining industry is only now developing protocols for good practice to reduce environmental hazards (Jones et al., 2019). However, establishing environmental management approaches for the deep-sea mining industry is challenged by the limited knowledge on the biology of deep-sea organisms, and the little information on the effects of deep-sea mining activities upon them. As such, experimental studies testing the physical and ecotoxicological effects of deep-sea mining on key benthic ecosystems are important to inform environmental planning and impact assessments aiming to avoid and minimize the impact created by the sediment plume on this type of organisms. The slow recovery capacity of deep-sea organisms to human impacts, related to their slow-growing and long-lived life histories, further emphasizes the need to avoid and minimize the impacts of mining activities on deep-sea ecosystems.
Our results show how relatively low concentrations of suspended small PMS particles can impair the physiology of cold-water octocorals, ultimately resulting in their death within a short period of time. The small size fraction of PMS particles is likely to stay in suspension for long periods of time and disperse further away from the emission point, potentially impacting fauna in the flanks and summits of close topographic features (Morato et al., 2022). The ecological consequences of these impacts may include loss of biodiversity, ecosystem functioning and the provision of goods and services by deep-sea ecosystems (Boschen et al., 2016; Le et al., 2017; Van Dover et al., 2017; Niner et al., 2018; Orcutt et al., 2020; Boschen-Rose et al., 2021).
These results highlight the need for a detailed understanding of both the mining plume dispersal and the physiological response of organisms to those plumes in the early phases of the mining project. These are key information that needs to be incorporated in the Environmental Risk Assessments and Environmental Management and Monitoring plans for the mining activities. These results also call the industry for the development of tools to monitor and minimize the creation and dispersal of particles and plume at the impact reference zones and preservation reference zones. The presented results also call the regulators of the paramount importance to implement effective area-based management tools such non-mining areas or marine protected areas (Durden et al., 2017; Jones et al., 2019; Clark et al., 2020), for the protection of cold-water coral ecosystems.
The raw data supporting the conclusions of this study are available at PANGAEA ( Carreiro-Silva et al. 2022: https://doi.pangaea.de/10.1594/PANGAEA.948414).
Conceived and designed experiment: MC-S, IM, VR, AC. Performed experiment: MC-S, VR, IM Processed the samples: MC-S, AG, IM, JR, MC, MR, RB, VR. Analyzed data: MC-S, IM, JR, MC, MR, TC, RB. All authors contributed to the writing of the paper. All authors contributed to the article and approved the submitted version.
The research leading to these results has received funding from the European Union Seventh Framework Programme (FP7/2007-2013) under the MIDAS project (grant agreement n° 603418) the European Union’s Horizon 2020 programme under grant agreements No 678760 (ATLAS) and No 818123 (iAtlantic), by Fundação para a Ciência e a Tecnologia, I.P. (FCT) and Direção-Geral de Política do Mar (DGPM) project Mining2/0005/2017. We also acknowledge funds and support from the FCT through the strategic project (UIDB/05634/2020 and UIDP/05634/2020) granted to OKEANOS and through the FCT Regional Government of the Azores under the project M1.1.A/REEQ.CIENTÍFICO UI&D/2021/010. This output reflects only the authors’ views and the European Union and the Regional Government of the Azores cannot be held responsible for any use that may be made of the information contained therein. AC, MCS and TM were supported by Program Stimulus of Scientific Employment (CEECIND/00101/2021, CCCIND/03346/2020 and CCCIND/03345/2020, respectively) from the Fundação para a Ciência e Tecnologia, AC, IM and RB were funded by Projeto Investigadores Mar AZ PO Açores 2020 (ACORES 01 0145 FEDER 000140), TC was supported by FRCT fellowship (M3.1.a/F/016/2018). AG and MR were supported by the H2020 iAtlantic project (Grant Agreement No 818123). MCS was also supported by EU FP7 project MORPH (EC/ICT/2011/7/288704). TM was also supported by Program Investigador FCT (IF/01194/2013), IFCT Exploratory Project (IF/01194/2013/CP1199/CT0002) from the Fundação para a Ciência e Tecnologia (POPH and QREN) and PO2020 MapGES. (Acores-01-0145-FEDER-000056). MC and JR acknowledge the strategic project UIDB/04423/2020 granted to CIIMAR and to the JPIOceans project MiningImpact2-Mining2/0004/2017 funded by the Portuguese FCT.
We would like to thank the captain and chief scientist of the MoMARSAT 2013 cruise (https://doi.org/10.17600/13030040) for the recovery of the inactive sulfide blocks as well as the ROV Victor6000 team. We acknowledge V. Lopes, C. Mourão and J.M.R.S. Relvas at the Geology department, Faculdade de Ciências Universidade de Lisboa (FCUL), Portugal for the preparation and granulometry analysis of the PMS particles and Sven Petersen at GEOMAR Helmholtz Centre for Ocean Research for the EDAX analyses of the grounded material. We also acknowledge Telmo Nunes at FCUL for scanning electron microscopy of quartz particles and Max Wisshak at the Senckenberg am Meer, Marine Research Department, Germany for scanning electron microscopy of polymetallic sulphide particles. We are grateful to the crew of RV Águas Vivas and Renato Bettencourt for the collection of coral colonies. We are also grateful to Noelie Reydet, Kristell de Potter and Hugo Parra for their help with the maintenance of corals in aquaria and monitoring of the experiment.
The authors declare that the research was conducted in the absence of any commercial or financial relationships that could be construed as a potential conflict of interest.
All claims expressed in this article are solely those of the authors and do not necessarily represent those of their affiliated organizations, or those of the publisher, the editors and the reviewers. Any product that may be evaluated in this article, or claim that may be made by its manufacturer, is not guaranteed or endorsed by the publisher.
The Supplementary Material for this article can be found online at: https://www.frontiersin.org/articles/10.3389/fmars.2022.915650/full#supplementary-material
Abdel-Salam H. A., Porter J. W., Hatcher B. G. (1988). ““Physiological effects of sediment rejection on photosynthesis and respiration in three Caribbean reef corals,”,” in Proccedings of the 6th international coral reef symposium, eds Choat J. H., Barnes D., Borowitzka M. A., Coll J. C., Davies P. J., Flood P., et al, Townsville (Australia), Vol. 2. , 285–292.
Addamo A. M., Vertino A., Stolarski J., García-Jiménez R., Taviani M., Machordom A. (2016). Merging scleractinian genera: The overwhelming genetic similarity between solitary Desmophyllum and colonial Lophelia. BMC Evol. Biol. 16 (1), 108. doi: 10.1186/s12862-016-0654-8
Ali A. A. M., Hamed M. A., El-Azim H. A. (2011). Heavy metals distribution in the coral reef ecosystems of the northern red Sea. Helgol. Mar. Res. 65, 67–80. doi: 10.1007/s10152-010-0202-7
Allers E., Abed R. M., Wehrmann L. M., Wang T., Larsson A. I., Purser A., et al. (2013). Resistance of Lophelia pertusa to coverage by sediment and petroleum drill cuttings. Mar. Poll. Bull. 74, 132–140. doi: 10.1016/j.marpolbul.2013.07.016
Anderson M. J., Gorley R. N., Clarke K. R. (2008). PERMANOVA+ for PRIMER: Guide for software and statistical methods (PRIMER-E: Plymouth, UK.).
Anderson M. J., Robinson J. (2003). Generalized discriminant analysis based on distances. Aust. N. Z. J. Stat. 45 (3), 301–318. doi: 10.1111/1467-842X.00285
ASA (2008). ““Modelling the dispersion of the returned water discharge plume from the solwara 1 seafloor mining project,”,” in Coffey Natural system 2008 (Papua New Guinea: Environmental Impact Statement: Solwara 1 Project, Volume B. Nautilus Minerals Niugini Limited). Available at: https://www.yumpu.com/en/document/read/12085050/appendices-5-13-nautilus-cares-nautilus-minerals.
Bagarinao T. (1992). Sulfide as an environmental factor and toxicant: tolerance and adaptations in aquatic organisms. Aquat. Toxicol. 24, 21–62. doi: 10.1016/0166-445X(92)90015-F
Baldwin E. (1964). An introduction to comparative biochemistry. 4th edition (Cambridge, England: Cambridge University Press).
Baussant T., Nilsen M., Ravagnan E., Westerlund S., Ramanand S. (2018). Effects of suspended drill cuttings on the coral Lophelia pertusa using pulsed and continuous exposure scenarios. J. Toxicol. Environ. Health Part A. 81 (10), 361–382. doi: 10.1080/15287394.2018.1444375
Beers R. F., Sizer I. W. (1952). A spectrophotometric method for measuring the breakdown of hydrogen peroxide by catalase. J. Biol. Chem. 195, 133–140. doi: 10.1016/S0021-9258(19)50881-X
Bell T. G., Johnson M. T., Jickells T. D., Liss P. S. (2007). Ammonia/ammonium dissociation coefficient in seawater: a significant numerical correction. Environ. Chem. 4, 183–258. doi: 10.1071/EN07032
Berry K. L., Seemann J., Dellwig O., Struck U., Wild C., Leinfelder R. R. (2013). Sources and spatial distribution of heavy metals in scleractinian coral tissues and sediments from the bocas del toro archipelago, Panama. Environ. Monit. Assess. 185, 9089–9099. doi: 10.1007/s10661-013-3238-8
Bersuder P., Amouroux I., Belzunce-Segarra M. J., Bolam T., Caetano M., Carvalho I., et al. (2021). Concurrent sampling of transitional and coastal waters by diffusive gradient in thin-films (DGT) and spot sampling for trace metals analysis. MethodX 8, 101462. doi: 10.1016/j.mex.2021.101462
Bielmyer G. K., Gillette P., Grosell M., Bhagooli R., Baker A. C., Langdon C., et al. (2010). Effects of copper exposure on three species of scleractinian corals. Aquat. Toxicol. 97, 125–133. doi: 10.1016/j.aquatox.2009.12.021
Blandin J., Sarradin P.-M., Cannat M. (2013). MOMARSAT2013 cruise, RV pourquoi pas? French Oceanographic Cruises, available at: https://campagnes.flotteoceanographique.fr/campagnes/13030040/
Boschen-Rose R. E., Clark M. R., Rowden A. A., Gardner J. P. (2021). Assessing the ecological risk to deep-sea megafaunal assemblages from seafloor massive sulfide mining using a functional traits sensitivity approach. Ocean Coast. Manage. 210, 105656. doi: 10.1016/j.ocecoaman.2021.105656
Boschen R. E., Rowden A. A., Clark M. R., Gardner J. P. (2013). Mining of deep-sea seafloor massive sulfides: a review of the deposits, their benthic communities, impacts from mining, regulatory frameworks and management strategies. Ocean Coast. Manage. 84, 54–67. doi: 10.1016/j.ocecoaman.2013.07.005
Boschen R. E., Rowden A. A., Clark M. R., Pallentin A., Gardner J. P. A. (2016). Seafloor massive sulfide deposits support unique megafaunal assemblages: implications for seabed mining and conservation. Mar. Environ. Res. 115, 78–88. doi: 10.1016/j.marenvres.2016.02.005
Bradford M. M. (1976). Rapid and sensitive method for quantification of microgram quantities of protein utilizing principle of protein-dye binding. Anal. Biochem. 72, 248–254. doi: 10.1016/0003-2697(76)90527-3
Braga-Henriques A., Porteiro F. M., Ribeiro P. A., De Matos V., Sampaio Í., Ocaña O., et al. (2013). Diversity, distribution and spatial structure of the cold-water coral fauna of the Azores (NE Atlantic). Biogeosciences 10, 4009–4036. doi: 10.5194/bg-10-4009-2013
Brooke S. D., Holmes M. W., Young C. M. (2009). Sediment tolerance of two different morphotyopes of the deep-sea coral Lophelia pertusa from the gulf of Mexico. Mar. Ecol. Prog. Ser. 390, 137–144. doi: 10.3354/meps08191
Brown B., Holley M. C. (1982). Metal levels associated with tin dredging and smelting and their effect upon intertidal reef flats at ko phuket, Thailand. Coral Reefs 1, 131–137. doi: 10.1007/BF00301695
Canesi L., Ciacci C., Lorusso L. C., Betti M., Gallo G., Pojana G., et al. (2007). Effects of triclosan on Mytilus galloprovincialis hemocyte function and digestive gland enzyme activities: possible modes of action on non target organisms. Comp. Biochem. Phys. C. 145, 464–472. doi: 10.1016/j.cbpc.2007.02.002
Carreiro-Silva M., Cerqueira T., Godinho A., Caetano M., Santos R. S., Bettencourt R. (2014). Molecular mechanisms underlying the physiological response of the cold-water coral Desmophyllum dianthus to ocean acidification. Coral Reefs 33, 465–476. doi: 10.1007/s00338-014-1129-2
Carreiro-Silva M., Ocaña O. V., Stanković D., Sampaio I., Porteiro F., Fabri M.-C., et al. (2017). Zoanthids associated with cold-water corals in the Azores region: hidden diversity in the deep-sea. Front. Mar. Sci. 4. doi: 10.3389/fmars.2017.00088
Carreiro-Silva M., Martins I., Raimundo J., Caetano M., Bettencourt R., Cerqueira T., et al. (2022). Results of an ex-situ experiment testing the effects of mining-generated sediment plumes on the cold-water octocoral Dentomuricea aff. meteor in the Azores. PANGAEA. doi: 10.1594/PANGAEA.948414
Chan I., Tseng L. C., Kâ S., Chang C. F., Hwang J. S. (2012). An experimental study of the response of the gorgonian coral Subergorgia suberosa to polluted seawater from a former coastal mining site in Taiwan. Zool. Stud. 51 (1), 27–37. available at: http://zoolstud.sinica.edu.tw/Journals/51.1/27.pdf
Christiansen B., Denda A., Christiansen S. (2020). Potential effects of deep seabed mining on pelagic and benthopelagic biota. Mar. Pol. 114, 103442. doi: 10.1016/j.marpol.2019.02.014
Clark M. R., Bowden D. A., Rowden A. A., Stewart R. (2019). Little evidence of benthic community resilience to bottom trawling on seamounts after 15 years. Front. Mar. Sci. 6. doi: 10.3389/fmars.2019.00063
Clark M. R., Durden J. M., Christiansen S. (2020). Environmental impact assessments for deep-sea mining: Can we improve their future effectiveness? Mar. Pol. 114, 103363. doi: 10.1016/j.marpol.2018.11.026
da Rocha I. V., Reis E. C., da Silva P. R., de Hollanda Cavalcanti G., Coutinho R., Reynier M. V. (2021). Deep-sea coral Lophelia pertusa laboratory maintenance and exposure to barite using water recirculation systems. Toxicol. Environ. Health Sci. 13 (1), 1–17. doi: 10.5897/JTEHS2021.0486
Diehl W. J. III, Lawrence J. M. (1979). Effect of nutrition on the excretion rate of soluble nitrogenous products of Luidia clathrata (Say) (Echinodermata; asteroidea). Comp. Biochem. Physiol. 62 (4), 801–806. doi: 10.1016/0300-9629(79)90006-9
Downs C. A., Fauth J. E., Robinson C. E., Curry R., Lanzendorf B., Halas J. C., et al. (2005). Cellular diagnostics and coral health: declining coral health in the Florida Keys. Mar. Poll. Bull. 51 (5-7), 558–569. doi: 10.1016/j.marpolbul.2005.04.017
Downs C. A., Ostrander G. K., Rougee L., Rongo T., Knutson S., Williams D. E., et al. (2012). The use of cellular diagnostics for identifying sub-lethal stress in reef corals. Ecotoxicology 21 (3), 768–782. doi: 10.1007/s10646-011-0837-4
Durden J. M., Murphy K., Jaeckel A., Van Dover C. L., Christiansen S., Gjerde K., et al. (2017). A procedural framework for robust environmental management of deep-sea mining projects using a conceptual model. Mar. Pol. 84, 193–201. doi: 10.1016/j.marpol.2017.07.002
Edge K. J., Johnston E. L., Dafforn K. A., Simpson S. L., Kutti T., Bannister R. J. (2016). Sub-Lethal effects of water-based drilling muds on the deep-water sponge Geodia barretti. Environ. pollut. 212, 525–534. doi: 10.1016/j.envpol.2016.02.047
Erftemeijer P. L., Riegl B., Hoeksema B. W., Todd P. A. (2012). Environmental impacts of dredging and other sediment disturbances on corals: a review. Mar. pollut. Bull. 64 (9), 1737–1765. doi: 10.1016/j.marpolbul.2012.05.008
Esslemont G., Harriot V. J., McConchie D. M. (2000). Variability of trace metal concentrations within and between colonies of Pocillopora damicornis. Mar. pollut. Bull. 40 (7), 637–642. doi: 10.1016/S0025-326X(00)00068-0
Fabricius K. E., Wolanski E. (2000). Rapid smothering of coral reef organisms by muddy marine snow. Estuar. Coast. Shelf. Sci. 50 (1), 115–120. doi: 10.1006/ecss.1999.0538
Fallon E. K., Frische M., Petersen S., Brooker R. A., Scott T. B. (2019). Geological, mineralogical and textural impacts on the distribution of environmentally toxic trace elements in seafloor massive sulfide occurrences. Minerals 9 (3), 162. doi: 10.3390/min9030162
Fang J. K., Rooks C. A., Krogness C. M., Kutti T., Hoffmann F., Bannister R. J. (2018). Impact of particulate sediment, bentonite and barite (oil-drilling waste) on net fluxes of oxygen and nitrogen in Arctic-boreal sponges. Environ. pollut. 238, 948–958. doi: 10.1016/j.envpol.2017.11.092
FAO (2009). International guidelines for the management of deep-sea fisheries in the high seas. Food and Agricultural Organization of the United Nations, Rome, Italy. 73pp.
Ferrier-Pagès C., Houlbrèque F., Wyse E., Richard C., Allemand D., Boisson F. (2005). Bioaccumulation of zinc in the scleractinian coral Stylophora pistillata. Coral Reefs 24 (4), 636–645. doi: 10.1007/s00338-005-0045-x
Fonseca J. S., Marangoni L. F. B., Marques J. A., Bianchini A. (2019). Energy metabolism enzymes inhibition by the combined effects of increasing temperature and copper exposure in the coral Mussismilia harttii. Chemosphere 236, 124420. doi: 10.1016/j.chemosphere.2019.124420
Fonseca J. S., Zebral Y. D., Bianchini A. (2021). Metabolic status of the coral Mussismilia harttii in field conditions and the effects of copper exposure in vitro. Comp. Biochem. Physiol. C. Toxicol. Pharmacol. 240, 108924. doi: 10.1016/j.cbpc.2020.108924
Gabbott P. A. (1983). ““Developmental and seasonal activities in marine molluscs”,” in Environmental biochemistry and physiology 2. the Mollusca, vol. 2 . Ed. Hochachka P. W. (New York: Academic Press). doi: 10.1016/B978-0-12-751402-4.50012-1
Gomes-Pereira J. N., Carmo V., Catarino D., Jakobsen J., Alvarez H., Aguilar R., et al. (2017). Cold-water corals and large hydrozoans provide essential fish habitat for Lappanella fasciata and Benthocometes robustus. Deep-Sea Res. II.: Top. Stud. Oceanogr. 145, 33–48. doi: 10.1016/j.dsr2.2017.09.015
Gwyther D. (2008) Environmental impact statement, solwara 1 project. nautilus minerals niugini limited, main report Coffey natural systems, Brisbane. Available at: https://www.yumpu.com/en/document/view/38646617/environmental-impact-statement-nautilus-cares-nautilus-minerals.
Habig W. H., Pabst M. J., Jakoby W. B. (1974). Glutathione s-transferases - first enzymatic step in mercapturic acid formation. J. Biol. Chem. 249, 7130–7139. doi: 10.1016/S0021-9258(19)42083-8
Harland A. D., Brown B. E. (1989). Metal tolerance in the scleractinian coral Porites lutea. Mar. pollut. Bull. 20 (7), 353–357. doi: 10.1016/0025-326X(89)90159-8
Harvell D., Jordán-Dahlgren E., Merkel S., Rosenberg E., Raymundo L., Smith G., et al. (2007). Coral disease, environmental drivers, and the balance between coral and microbial associates. Oceanography 20, 172–195. doi: 10.5670/oceanog.2007.91
Hauton C., Brown A., Thatje S., Mestre N. C., Bebianno M. J., Martins I., et al. (2017). Identifying toxic impacts of metals potentially released during deep-sea mining–a synthesis of the challenges to quantifying risk. Front. Mar. Sci. 4, 368. doi: 10.3389/fmars.2017.00368
Jones D. O., Durden J. M., Murphy K., Gjerde K. M., Gebicka A., Colaço A., et al. (2019). Existing environmental management approaches relevant to deep-sea mining. Mar. Policy 103, 172–181. doi: 10.1016/j.marpol.2019.01.006
Jones R., Ricardo G. F., Negri A. P. (2015). Effects of sediments on the reproductive cycle of corals. Mar. pollut. Bull. 100, 13–33. doi: 10.1016/j.marpolbul.2015.08.021
Kalghatgi S., Spina C. S., Costello J. C., Liesa M., Morones-Ramirez J. R., Slomovic S., et al. (2013). Bactericidal antibiotics induce mitochondrial dysfunction and oxidative damage in mammalian cells. Sci. Transl. Med. 5 (192), 192ra85. doi: 10.1126/scitranslmed.3006055
Kutti T., Bannister R. J., Fosså J. H., Krogness C. M., Tjensvoll I., Søvik G. (2015). Metabolic responses of the deep-water sponge Geodia barretti to suspended bottom sediment, simulated mine tailings and drill cuttings. J. Exp. Mar. Biol. Ecol. 473, 64–72. doi: 10.1016/j.jembe.2015.07.017
Larsson A. I., Purser A. (2011). Sedimentation on the cold-water coral Lophelia pertusa: cleaning efficiency from natural sediments and drill cuttings. Mar. Poll. Bull. 62, 1159–1168. doi: 10.1016/j.marpolbul.2011.03.041
Larsson A. I., van Oevelen D., Purser A., Thomsen L. (2013). Tolerance to long-term exposure of suspended benthic sediments and drill cuttings in the cold-water coral Lophelia pertusa. Mar. Poll. Bull. 70, 176–188. doi: 10.1016/j.marpolbul.2013.02.033
Le J. T., Levin L. A., Carson R. T. (2017). Incorporating ecosystem services into environmental management of deep-seabed mining. Deep-Sea Res. II.: Top. Stud. Oceanogr. 137, 486–503. doi: 10.1016/j.dsr2.2016.08.007
Lesser M. P. (2006). Oxidative stress in marine environments: biochemistry and physiological ecology. Annu. Rev. Physiol. 68, 253–278. doi: 10.1146/annurev.physiol.68.040104.110001
Levin L. A., Mengerink K., Gjerde K. M., Rowden A. A., Van Dover C. L., Clark M. R., et al. (2016). Defining “serious harm” to the marine environment in the context of deep-seabed mining. Mar. Policy 74, 245–259. doi: 10.1016/j.marpol.2016.09.032
Liefmann S., Järnegren J., Johnsen G., Murray F. (2018). Eco-physiological responses of cold-water soft corals to anthropogenic sedimentation and particle shape. J. Exp. Mar. Biol. Ecol. 504 (2), 61–71. doi: 10.1016/j.jembe.2018.02.009
Lushchak V. I. (2011). Environmentally induced oxidative stress in aquatic animals. Aquat. Toxicol. 101, 13–30. doi: 10.1016/j.aquatox.2010.10.006
Marangoni L. F. B., Marques J. A., Duarte G. A. S., Pereira C. M., Calderon E. N., Castro C. B. (2017). Copper effects on biomarkers associated with photosynthesis, oxidative status and calcification in the Brazilian coral Mussismilia harttii (Scleractinia, mussidae). Mar. Environ. Res. 130, 248–257. doi: 10.1016/j.marenvres.2017.08.002
Marques J. A., Abrantes D. P., Laura F. B., Marangoni L. F. B., Bianchini A. (2020). Ecotoxicological responses of a reef calcifier exposed to copper, acidification and warming: A multiple biomarker approach. Environ. pollut. 257, 113572. doi: 10.1016/j.envpol.2019.113572
Martins I., Godinho A., Goulart J., Carreiro-Silva M. (2018). Assessment of Cu sub-lethal toxicity (LC50) in the cold-water gorgonian Dentomuricea meteor under a deep-sea mining activity scenario. Environ. pollut. 240, 903–907. doi: 10.1016/j.envpol.2018.05.040
Martins I., Godinho A., Rakka M., Carreiro-Silva M. (2022). Beyond deep-sea mining sublethal effects: Delayed mortality from acute Cu exposure of the cold-water gorgonian Viminella flagellum. Mar. Poll. Bull. 183, 114051. doi: 10.1016/j.marpolbul.2022.114051
Miller K. A., Thompson K. F., Johnston P., Santillo D. (2018). An overview of seabed mining including the current state of development, environmental impacts, and knowledge gaps. Front. Mar. Sci. 4 (1). doi: 10.3389/fmars.2017.00418
Mitchelmore C. L., Verde A. E., Ringwood A. H., Weis V. M. (2003). Differential accumulation of heavy metals in the sea anemone Anthopleura elegantissima as a function of symbiotic state. Aquat. Toxicol. 64, 317–329. doi: 10.1016/j.envpol.2019.113572
Mitchelmore C. L., Verde E. A., Weis V. M. (2007). Uptake and partitioning of copper and cadmium in the coral Pocillopora damicornis. Aquat. Toxicol. 85, 48–56. doi: 10.1016/j.aquatox.2007.07.015
Mohammed T. A. A., Dar M. A. (2010). Ability of corals to accumulate heavy metals, northern red Sea, Egypt. Environ. Earth Sci. 59, 1525–1534. doi: 10.1007/s12665-009-0138-x
Montero N., Belzunce-Segarra M. J., Gonzalez J. L., Larreta J., Franco J. (2012). Evaluation of diffusive gradients in thin-films (DGTs) as a monitoring tool for the assessment of the chemical status of transitional waters within the water framework directive. Mar. Poll. Bull. 64, 31–39. doi: 10.1016/j.marpolbul.2011.10.028
Morato T., Dominguez-Carrió C., Mohn C., Ocaña V. O., Ramos M., Rodrigues L., et al. (2021). Dense cold-water coral garden of Paragorgia johnsoni suggests the importance of the mid-Atlantic ridge for deep-sea biodiversity. Ecol. Evol. 11 (23), 16426–16433. doi: 10.1002/ece3.8319
Morato T., Juliano M., Pham C. K., Carreiro-Silva M., Martins I., Colaço A. (2022). Modelling the dispersion of seafloor massive sulphide mining plumes in the mid Atlantic ridge around the Azores. Front. Mar. Sci. 9, 910940. doi: 10.3389/fmars.2022.910940
Naumann M. S., Niggl W., Laforsch C., Glaser C., Wild C. (2009). Coral surface area quantification - evaluation of established methods by comparison with computer tomography. Coral Reefs 28, 109–117. doi: 10.1007/s00338-008-0459-3
Nguyen T. V., Alfaro A. C., Merien F., Lulijwa R., Young T. (2018). Copper-induced immunomodulation in mussel (Perna canaliculus) haemocytes. Metallomics 10 (7), 965–978. doi: 10.1039/c8mt00092a
Niner H. J., Ardron J. A., Escobar E. G., Gianni M., Jaeckel A., Jones D. O., et al. (2018). Deep-sea mining with no net loss of biodiversity–an impossible aim. Front. Mar. Sci. 5. doi: 10.3389/fmars.2018.00053
Okumura T., Nagasawa T., Hayashi I., Sato Y. (2002). Effects of starvation on RNA: DNA ratio, glycogen content, and c: N ratio in columellar muscle of the Japanese turban shell Turbo (Batillus) cornutus (Gastropoda). Fish. Sci. 68 (2), 306–312.2. doi: 10.1046/j.1444-2906.2002.00426.x
Orcutt B. N., Bradley J. A., Brazelton W. J., Estes E. R., Goordial J. M., Huber J. A., et al. (2020). Impacts of deep-sea mining on microbial ecosystem services. Limnol. Oceanogr. 65 (7), 1489–1510. doi: 10.1002/lno.11403
OSPAR (2010). Background document for coral gardens, biodiversity series. Commission. Available at: https://www.ospar.org/documents?d=7217
Petersen S., Krätschell A., Augustin N., Jamieson J., Hein J. R., Hannington M. D. (2016). News from the seabed–geological characteristics and resource potential of deep-sea mineral resources. Mar. Pol. 70, 175–187. doi: 10.1016/j.marpol.2016.03.012
Pham C. K., Vandeperre F., Menezes G., Porteiro F., Isidro E., Morato T. (2015). The importance of deep-sea vulnerable marine ecosystems for demersal fish in the Azores. Deep-Sea Res. I.: Oceanogr. Res. Pap. 96, 80–88. doi: 10.1016/j.dsr.2014.11.004
Raimundo J., Vale C., Caetano M., Anes B., Carreiro-Silva M., Martins I., et al. (2013). Element concentrations in cold-water gorgonians and black coral from Azores region. Deep-Sea Res. II.: Top. Stud. Oceanogr. 98, 129–136. doi: 10.1016/j.dsr2.2013.01.012
Rainbow P. (2002). Trace metal concentrations in aquatic invertebrates: why and so what? Environ. pollut. 120, 497–507. doi: 10.1016/S0269-7491(02)00238-5
Rakka M., Maier S. R., Van Oevelen D., Godinho A., Bilan M., Orejas C., et al. (2021). Contrasting metabolic strategies of two co-occurring deep-sea octocorals. Sci. Rep. 11, 10633. doi: 10.1038/s41598-021-90134-5
Regoli F., Giuliani M. E. (2014). Oxidative pathways of chemical toxicity and oxidative stress biomarkers in marine organisms. Mar. Environ. Res. 93, 106–117. doi: 10.1016/j.marenvres.2013.07.006
Reichelt-Brushett A. J., McOristb G. (2003). Trace metals in the living and nonliving components of scleractinian corals. Mar. pollut. Bull. 46 (12), 1573–1582. doi: 10.1016/S0025-326X(03)00323-0
Reichelt A. J., Jones G. B. (1994). Trace metal as tracers of dredging activity in Cleveland bay–field and laboratory studies. Aust. J. Mar. Freshw. Res. 45, 1237–1257. doi: 10.1071/MF9941237
Rhee J.-S., Yu I. T., Kim B.-M., Jeong C.-B., Lee K.-W., Kim M.-J., et al. (2013). Copper induces apoptotic cell death through reactive oxygen species-triggered oxidative stress in the intertidal copepod Tigriopus japonicus. Aquat. Toxicol. 132-133, 182–189. doi: 10.1016/J.AQUATOX.2013.02.013
Riegl B., Bloomer J. P. (1995). ““Tissue damage in hard and soft corals due to experimental exposure to sedimentation”,” in Proceedings 1st European regional meeting ISKS, vol. 20. (Vienna: Beitrage zur Palaeontologie von Oesterreich), 51–63.
Riegl B., Branch G. M. (1995). Effects of sediment on the energy budgets of four scleractinian (Bourne 1900) and five alcyonacean (Lamouroux 1816) corals. J. Exp. Mar. Biol. Ecol. 186, 259–275. doi: 10.1016/0022-0981(94)00164-9
Roberts J. M., Wheeler A., Freiwald A., Cairns S. (2009). Cold-water corals: the biology and geology of deep-sea coral habitats (Cambridge University Press: Cambridge, UK.).
Rogers C. S. (1990). Responses of coral reefs and reef organisms to sedimentation. Mar. Ecol. Progr. Ser. 62 (1), 185–202. doi: 10.3354/meps062185
Rovelli L., Carreiro-Silva M., Attard K. M., Rakka M., Dominguez-Carrió C., Bilan M., et al. (2022). Benthic O2 uptake by coral gardens at the condor seamount (Azores). Mar. Ecol. Progr. Ser. 688, 19–31. doi: 10.3354/meps14021
Rushmore M. E., Ross C., Fogarty N. D. (2021). Physiological responses to short-term sediment exposure in adults of the Caribbean coral Montastraea cavernosa and adults and recruits of Porites astreoides. Coral Reefs 40, 1579–1591. doi: 10.1007/s00338-021-02156-0
Sabdono A. (2009). Heavy metal levels and their potential toxic effect on coral Galaxea fascicularis from Java Sea, Indonesia. Res. J. Environ. Sci. 3 (1), 96–102. doi: 10.3923/rjes.2009.96.102
Scanes E., Kutti T., Fang J. K. H., Johnston. E. L., Ross P. M., Bannister R. J. (2018). Mine waste and acute warming induce energetic stress in the deep-Sea sponge Geodia atlantica and coral Primnoa resedeaformis: Results from a mesocosm study. Front. Mar. Sci. 5. doi: 10.3389/fmars.2018.00129
Schwarz J. A., Mitchelmore C. L., Jones R., O’Dea A., Seymour S. (2013). Exposure to copper induces oxidative and stress responses and DNA damage in the coral Montastraea franksi. Comp. Biochem. Phsiol. Part C. 157, 272–279. doi: 10.1016/j.cbpc.2012.12.003
Servetto N., de Aranzamendi M. C., Bettencourt R., Held C., Abele D., Movilla J., et al. (2021). Molecular mechanisms underlying responses of the Antarctic coral Malacobelemnon daytoni to ocean acidification. Mar. Environ. Res. 170, 105430. doi: 10.1016/j.marenvres.2021.105430
Sharma R. (2017). Deep-sea mining: Resource potential, technical and environmental considerations (Springer, Cham). doi: 10.1007/978-3-319-52557-0
Siddiqui S., Bielmyer-Fraser G. K. (2015). Responses of the sea anemone, Exaiptasia pallida, to ocean acidification conditions and copper exposure. Aquat. Toxicol. 167, 228–239. doi: 10.1016/j.aquatox.2015.08.012
Smith C. R., Tunnicliffe V., Colaço A., Drazen J. C., Gollner S., Levin L. A., et al. (2020). Deep-sea misconceptions cause underestimation of seabed-mining impacts. Trends Ecol. Evol. 35 (10), 853–857. doi: 10.1016/j.tree.2020.07.002
Strachan M. F., Kingston P. F. (2012). A comparative study on the effects of barite, ilmenite and bentonite on four suspension feeding bivalves. Mar. Poll. Bull. 64, 2029e2038. doi: 10.1016/j.marpolbul.2012.06.023
Telesnicki G. J., Goldberg W. M. (1995). Effects of turbidity on the photosynthesis and respiration of two south Florida reef coral species. Bull. Mar. Sci. 57, 527–539.
Therond P., Gerbaud P., Dimon S., Anderson W. B., EvainBrion D., Raynaud F. (1996). Antioxidant enzymes in psoriatic fibroblasts and erythrocytes. J. Invest. Dermatol. 106, 1325–1328. doi: 10.1111/1523-1747.ep12349055
Underwood A. J. (1997). Experiments in ecology: Their logical design and interpretation using analysis of variance (Cambridge: Cambridge University Press).
Van Dover C. L. (2014). Impacts of anthropogenic disturbances at deep-sea hydrothermal vent ecosystems: A review. Mar. Environ. Res. 102, 59–72. doi: 10.1016/j.marenvres.2014.03.008
Van Dover C. L., Ardron J. A., Escobar E., Gianni M., Gjerde K. M., Jaeckel A., et al. (2017). Biodiversity loss from deep-sea mining. Nat. Geosci. 10, 464–465. doi: 10.1038/ngeo2983
Van Dover C. L., Colaço A., Collins P. C., Croot P., Metaxas A., Murton B. J., et al. (2020). Research is needed to inform environmental management of hydrothermally inactive and extinct polymetallic sulfide (PMS) deposits. Mar. Pol. 121, 104183. doi: 10.1016/j.marpol.2020.104183
Walters K., Coen L. D. (2006). A comparison of statistical approaches to analyzing community convergence between natural and constructed oyster reefs. J. Exp. Mar. Biol. Ecol. 330 (1), 81–95. doi: 10.1016/J.JEMBE.2005.12.018
Washburn T. W., Turner P. J., Durden J. M., Jones D. O., Weaver P., Van Dover C. L. (2019). Ecological risk assessment for deep-sea mining. Ocean Coast. Manage. 176, 24–39. doi: 10.1016/j.ocecoaman.2019.04.014
Weaver P. P. E., Billett D. (2019). “Environmental impacts of nodule, crust and sulphide mining: An overview,” in Environmental issues of deep-Sea mining, vol. pp . Ed. Sharma R. (Cham: Springer), 27–62). doi: 10.1007/978-3-030-12696-4_3
Weber M., de Beer D., Lott C., Polerecky L., Kohls K., Abed R. M. M., et al. (2012). Mechanisms of damage to corals exposed to sedimentation. Proc. Natl. Acad. Sci. U.S.A. 109, E1558–E1567. doi: 10.1073/pnas.1100715109
Weber M., Lott C., Fabricius K. E. (2006). Sedimentation stress in a scleractinian coral exposed to terrestrial and marine sediments with contrasting physical, organic and geochemical properties. J. Exp. Mar. Biol. Ecol. 336, 18–32. doi: 10.1016/j.jembe.2006.04.007
Wedding L. M., Reiter S. M., Smith C. R., Gjerde K. M., Kittinger J. N., Friedlander A. M., et al. (2015). Managing mining of the deep seabed. Science 349 (6244), 144–145. doi: 10.1126/science.aac6647
Zetsche E.-M., Baussant T., Meysman F. J. R., van Oevelen D. (2016). Direct visualization of mucus production by the cold-water coral Lophelia pertusa with digital holographic microscopy. PloS One 11 (2), e0146766. doi: 10.1371/journal.pone.0146766
Zhang H., Davison W. (2000). Direct in situ measurements of labile inorganic and organically bound metal species in synthetic solutions and natural waters using diffusive gradients in thin films. Anal. Chem. 72, 4447–4457. doi: 10.1021/ac0004097
Keywords: anthropogenic impact, hydrothermal vent, metals, Northeast Atlantic, physiology, sedimentation, Cnidaria
Citation: Carreiro-Silva M, Martins I, Riou V, Raimundo J, Caetano M, Bettencourt R, Rakka M, Cerqueira T, Godinho A, Morato T and Colaço A (2022) Mechanical and toxicological effects of deep-sea mining sediment plumes on a habitat-forming cold-water octocoral. Front. Mar. Sci. 9:915650. doi: 10.3389/fmars.2022.915650
Received: 08 April 2022; Accepted: 09 August 2022;
Published: 11 October 2022.
Edited by:
Philip Weaver, Seascape Consultants Ltd, United KingdomReviewed by:
Sandra Brooke, Florida State University, United StatesCopyright © 2022 Carreiro-Silva, Martins, Riou, Raimundo, Caetano, Bettencourt, Rakka, Cerqueira, Godinho, Morato and Colaço. This is an open-access article distributed under the terms of the Creative Commons Attribution License (CC BY). The use, distribution or reproduction in other forums is permitted, provided the original author(s) and the copyright owner(s) are credited and that the original publication in this journal is cited, in accordance with accepted academic practice. No use, distribution or reproduction is permitted which does not comply with these terms.
*Correspondence: Marina Carreiro-Silva, bWFyaW5hLnBjLnNpbHZhQHVhYy5wdA==; Y2FycmVpcm9zaWx2YW1hcmluYUBnbWFpbC5jb20=
Disclaimer: All claims expressed in this article are solely those of the authors and do not necessarily represent those of their affiliated organizations, or those of the publisher, the editors and the reviewers. Any product that may be evaluated in this article or claim that may be made by its manufacturer is not guaranteed or endorsed by the publisher.
Research integrity at Frontiers
Learn more about the work of our research integrity team to safeguard the quality of each article we publish.