- 1Coasts and Ocean Research, Oceans and Atmosphere, Commonwealth Scientific and Industrial Research Organisation, Canberra, ACT, Australia
- 2School of Environment and Science, Griffith University, Nathan, QLD, Australia
- 3Climate Science Centre, Oceans and Atmosphere, Commonwealth Scientific and Industrial Research Organisation, Aspendale, VIC, Australia
- 4Centre for Coastal Biogeochemistry, Faculty of Science and Engineering, Southern Cross University, Lismore, NSW, Australia
- 5International Laboratory for Air Quality and Health, School of Earth and Atmospheric Sciences, Queensland University of Technology, Brisbane, QLD, Australia
Marine dimethylsulfide (DMS) is an important source of natural sulfur to the atmosphere, with potential implications for the Earth’s radiative balance. Coral reefs are important regional sources of DMS, yet their contribution is not accounted for in global DMS climatologies or in model simulations. This study accounts for coral-reef-derived DMS and investigates its influence on the atmosphere of the Great Barrier Reef (GBR), Australia, using the Australian Community Climate and Earth System Simulator Atmospheric Model version 2 (ACCESS-AM2). A climatology of seawater surface DMS (DMSw) concentration in the GBR and an estimate of direct coral-to-air DMS flux during coral exposure to air at low tide are incorporated into the model, increasing DMS emissions from the GBR region by 0.02 Tg yr-1. Inclusion of coral-reef-derived DMS increased annual mean atmospheric DMS concentration over north-eastern Australia by 29%, contributing to an increase in gas-phase sulfate aerosol precursors of up to 18% over the GBR. The findings suggest that the GBR is an important regional source of atmospheric sulfur, with the potential to influence local-scale aerosol-cloud processes. However, no influence on sulfate aerosol mass or number concentration was detected, even with a reduction in anthropogenic sulfur dioxide emissions, indicating that DMS may not significantly influence the regional atmosphere at monthly, annual or large spatial scales. Further research is needed to improve the representation of coral-reef-derived DMS in climate models and determine its influence on local, sub-daily aerosol-cloud processes, for which observational studies suggest that DMS may play a more important role.
1 Introduction
Aerosols and clouds play a key role in the Earth’s radiative budget and climate by governing the amount of incoming and outgoing solar radiation and heat (Ramanathan et al., 1989; Andreae, 1995). Since the industrial revolution, anthropogenic greenhouse gas (GHG) emissions have exerted an average global radiative forcing of +2.72 (1.96 to 3.84) W m-2 (IPCC, 2021). Anthropogenic and natural aerosols have partially offset this warming effect through scattering of incoming short-wave radiation (aerosol-radiation interactions) (McCormick and Ludwig, 1967) and influencing the albedo, lifetime and cover of clouds (aerosol-cloud interactions) (Twomey et al., 1984).
In comparison to GHG emissions, the influence of aerosols on the climate is complex and less well quantified due to uncertainties in aerosol source strengths and their radiative effects (Twomey, 1974; Andreae and Rosenfeld, 2008). Consequently, estimates of aerosol radiative forcing range from -1.9 to -0.1 W m-2 (IPCC, 2013; IPCC, 2014). Approximately 45% of the variance in aerosol radiative forcing since the pre-industrial period is derived from uncertainties in natural aerosol sources, including marine dimethylsulfide (DMS) (Carslaw et al., 2013).
The precursor of DMS, dimethylsulfoniopropionate (DMSP), is produced throughout the ocean by a number of marine organisms including planktonic and benthic algae (Stefels, 2000; Sunda et al., 2002), corals and their endosymbiotic dinoflagellates (Raina et al., 2013), and marine bacteria (Curson et al., 2017). DMSP is produced for a range of physiological and ecological functions (Stefels, 2000; McParland and Levine, 2019). Catabolism of DMSP is mediated by the demethylation and cleavage pathways, with the latter producing DMS (Bullock et al., 2017). Seawater surface DMS (DMSw) is ventilated to the marine boundary layer (MBL) where it is rapidly oxidized, primarily by hydroxyl radicals (OH), to sulfate aerosol precursor gases including sulfur dioxide (SO2), methanesulfonic acid (MSA), hydroperoxymethyl thioformate (HPMTF) and sulfuric acid (H2SO4) (Andreae and Crutzen, 1997; Berndt et al., 2019; Hodshire et al., 2019; Veres et al., 2020).
Sulfuric acid, an aerosol precursor, may condense onto existing particles, facilitating growth to cloud condensation nuclei (CCN), or nucleate to form new non-sea salt sulfate (nss-SO4) aerosol (Andreae and Crutzen, 1997; Woodhouse et al., 2013). The latter primarily occurs in the free troposphere, where background aerosol concentration and temperature are relatively low and conditions are optimal for the nucleation of trace gases (Korhonen et al., 2008; Sanchez et al., 2018). New nss-SO4 particles may be entrained from the free troposphere to the MBL and increase the number of CCN and cloud droplets.
When high concentrations of fine aerosol grow rapidly to CCN, cloud droplet number increases and cloud droplet diameter decreases (assuming constant cloud liquid water content), enhancing the albedo and lifetime of clouds and suppressing precipitation (Rosenfeld et al., 2007; Dave et al., 2019). Conversely, when CCN activation size is not met, water vapor may remain in the atmosphere, suppressing local precipitation and increasing precipitation downwind if conditions for particle growth are more favorable (Andreae and Rosenfeld, 2008; Fan et al., 2018).
The influence of DMS on Earth’s radiative balance is dependent on the efficiency of DMS oxidation and nucleation to secondary nss-SO4, which ranges from 0.14-0.95 in the MBL (Von Glasow and Crutzen, 2004). The high range in efficiency is due to regional variability in pre-existing aerosols and the aerosol condensational sink (Woodhouse et al., 2013; Hoffmann et al., 2016). The annual mean contribution of DMS to CCN is estimated to be 3.3% in the Northern Hemisphere and 9.9% in the relatively cleaner Southern Hemisphere (Woodhouse et al., 2010). This response varies with season and location, with the greatest response occurring in pristine regions of high biological activity (Korhonen et al., 2008; Lana et al., 2012; Gabric et al., 2013; Woodhouse et al., 2013; Sanchez et al., 2018; Zavarsky et al., 2018). For example, when phytoplankton productivity (and DMS production) increases, DMS-derived sulfates contribute up to 46% of CCN over the Southern Ocean (30-45°S) in summer (Korhonen et al., 2008), and 33% of CCN over the North Atlantic in spring (Sanchez et al., 2018) via new particle nucleation and particle growth to CCN.
Coral reefs are identified as important regional sources of DMS (Broadbent and Jones, 2004; Swan et al., 2017; Jones et al., 2018). In the coral holobiont, DMS(P) production is upregulated in response to physiological stress caused by exposure to high sea surface temperature (SST), solar irradiance and changes in salinity (Raina et al., 2013; Deschaseaux et al., 2014; Gardner et al., 2016; Hopkins et al., 2016), all of which can be exacerbated during exposure to air at low tide (Buckee et al., 2020). This biogenic sulfur source could facilitate nss-SO4 aerosol nucleation and growth to CCN, increasing the lifetime and albedo of low-level clouds (LLC) over coral reefs (similarly to Charlson et al., 1987). Evaporation over shallow, warm coral reef waters contributes to the formation of a convective boundary layer (~65-130 m), with relatively high humidity and temperature that is favourable for low-level cloud formation (McGowan et al., 2019). It has been hypothesized that DMS emissions facilitate the formation of a local or regional negative feedback within the coral reef boundary layer, shading and cooling the coral reef below (Fischer and Jones, 2012; Jones, 2015; Jones et al., 2017).
Global modelling studies have found that marine DMS is an important source of nss-SO4 aerosol, influencing regional aerosol and cloud microphysical properties (Thomas et al., 2010; Woodhouse et al., 2010; Gabric et al., 2013; Mahajan et al., 2015) and providing a cooling effect of up to 0.45°C (Fiddes et al., 2018). However, with the exception of only two model studies (Fiddes et al., 2021, 2022), the coral reef source of DMS is not currently accounted for in DMS climatologies or in climate models.
Global climate models typically prescribe DMSw concentrations using the Kettle et al. (1999) or Lana et al. (2011) monthly mean climatologies. These climatologies were derived from more than three decades of DMSw observations over most of the global ocean (Kettle et al., 1999). However, very few observations were included for coral reef regions and extrapolation did not account for seasonal or spatial variability across coral reef flats and lagoon waters.
Only two model studies have investigated the importance of DMS flux from coral reefs on the climate (Fiddes et al., 2021; Fiddes et al., 2022). Both of these studies accounted for the coral reef source of DMSw by adding a scaled laboratory-derived estimate of 50 nmol L-1 to the Lana et al. (2011) DMSw climatology for coral reef regions. This method added 0.03 nmol L-1 to the global average seawater concentration and increased global sea-air emissions by 0.3 Tg yr-1 of DMS, representing 1.7% of global DMS emission estimates (Fiddes et al., 2021). This is higher than the estimate of 0.06-0.08 Tg yr-1 of DMS estimated to be released from tropical coral reefs based on field observations (Jones et al., 2018; Jackson et al., 2021).
Despite the relatively high coral reef DMSw and DMS sea-air flux source, Fiddes et al. (2021) report only a small response in nucleation and Aitken mode aerosol number concentration and mass when the coral reef source of DMS was removed from the global ACCESS-UKCA (Australian Community Climate and Earth System Simulator-United Kingdom Chemistry and Aerosol) model. This suggests that tropical coral reef DMS emissions have no robust impact on contemporary global or regional climate, possibly due to confounding impacts of industrial sulfate emissions and other anthropogenic pollutants dominating the aerosol signal. Fiddes et al. (2022) demonstrated similar findings in the GBR region using the regional WRF-Chem (Weather Research and Forecasting model coupled with chemistry) model. However, observational studies suggest that the coral reef source of DMS may influence sulfate aerosol and LLC properties, at spatial or temporal scales that are perhaps not captured by global or regional climate models (Modini et al., 2009; Swan et al., 2016).
For example, positive correlations have been identified between the number concentration of atmospheric particles ranging from 0.5-2.5 μm (wet diameter) and both DMS sea-air flux (Deschaseaux et al., 2022) and DMSa concentration (Jackson et al., 2020) at Heron Island in the southern GBR. The magnitude of the correlation between DMSa and particle number concentration increased during daylight hours, when DMS oxidation rates over relatively clean oceans are highest (Gabric et al., 2008; Galí et al., 2013), and when wind speed was low (< 2 m s-1) (Jackson et al., 2020). Although the observed size range was larger than newly nucleated particles, the positive correlation during calm, daylight hours may have reflected local condensational growth of existing fine particles (< 0.5 μm) to the larger, detectable size range (Jackson et al., 2020). Therefore, DMS-derived sulfates may play an important role in aerosol composition over the GBR.
A parameterization of DMSw in the Great Barrier Reef (GBR), Australia, was recently derived from observational data obtained during Marine National Facility RV Investigator voyage IN2016_V05 (RVI). This parameterization was used to calculate a 19-year climatology of DMSw and DMS flux from the GBR using remotely sensed observations (Jackson et al., 2021). The calculated DMSw climatology reproduced observed seasonal and spatial variability well in the GBR (summarized in Jones et al., 2018) and estimates that the 347,000 km2 of coral reefs and lagoon waters in the GBR release 0.03-0.05 Tg yr-1 DMS (0.015-0.026 Tg yr-1 S) (Jackson et al., 2021).
Here, we build on previous modelling work by investigating the influence of coral-reef-derived DMS on the contemporary and a relatively clean regional atmosphere. Coral-reef-derived DMS is accounted for by incorporating a climatology of DMSw calculated from observational data in the GBR, and an estimate of direct coral-air DMS flux, into the Australian Community Climate and Earth-System Simulator Atmospheric Model version 2 (ACCESS-AM2). The accuracy of DMS sources in the model is evaluated by comparing model output with observations, and the influence of coral-reef-derived DMS on the regional atmosphere over north-eastern Australia is quantified.
2 Materials and methods
2.1 ACCESS-AM2 description
ACCESS-AM2 is a global physical climate model, which contributed to the World Climate Research Programme’s Coupled Model Intercomparison Project Phase 6 (CMIP6). The physical atmospheric model in ACCESS is the Met Office Unified Model (UM) version 10.6 (Walters et al., 2019). This version uses the Global Atmosphere 7.1 (GA7.1) configuration and includes the Global Model of Aerosol Processes (GLOMAP) aerosol scheme (Mann et al., 2010; Mann et al., 2012).
GLOMAP-mode resolves aerosol mass, number concentration, size distribution, composition, and optical properties. Prognostic aerosol species included in GLOMAP-mode are sulfate, black carbon, organic carbon and sea salt, which are internally mixed within five modes corresponding to soluble nucleation (dry diameter < 5 nm), Aitken (dry diameter 5-50 nm), accumulation (dry diameter 50-500 nm), coarse (dry diameter > 500 nm) and insoluble Aitken mode. Processes simulated within GLOMAP-mode include primary emissions, new particle nucleation, particle growth via coagulation, condensation and cloud processes, particle removal by dry deposition, and in-cloud and below-cloud scavenging (Mann et al., 2010; Mann et al., 2012). Oxidant concentrations necessary for the sulfur cycle are prescribed from monthly varying inputs.
Atmospheric variables are resolved at a horizontal resolution of 1.25° latitude x 1.875° longitude (~135 km x ~200 km resolution in the low-latitudes), with 85 vertical levels (50 below 18 km and 35 above) reaching a model top of 85 km. Further details on the physical parameterizations in UM GA7.1 are described in detail in Walters et al. (2019). ACCESS-AM2 uses an identical implementation, of which a detailed description is provided in Bi et al. (2020). In this work, an atmosphere-only (ACCESS-AM2) configuration is used (Bodman et al., 2020).
2.2 Experimental design
2.2.1 Seawater surface DMS concentration
In ACCESS-AM2, DMSw is prescribed from the global Kettle et al. (1999) monthly mean climatology, henceforth K99 (Figure 1A). As discussed above, the K99 climatology was derived from DMSw observations over most of the global ocean (Kettle et al., 1999). However, very few observations were included for coral reef regions and extrapolation did not account for seasonal or spatial variability across coral reef flats and lagoon waters. For this analysis, K99 is modified to include a climatology of DMSw derived from an empirical relationship in the GBR (Jackson et al., 2021), henceforth referred to as the GBR climatology. The GBR climatology calculated in Jackson et al. (2021) (0.25-degree grid) was scaled up to the model grid by binning the values to a resolution of 1.25 x 1.875 degrees. K99 was then modified by substituting pixels within the GBR region (10.5-25°S; 142-154°E) with the GBR climatology, which added an average of 0.5 nmol to the GBR seawater surface concentration (Figure 1C).
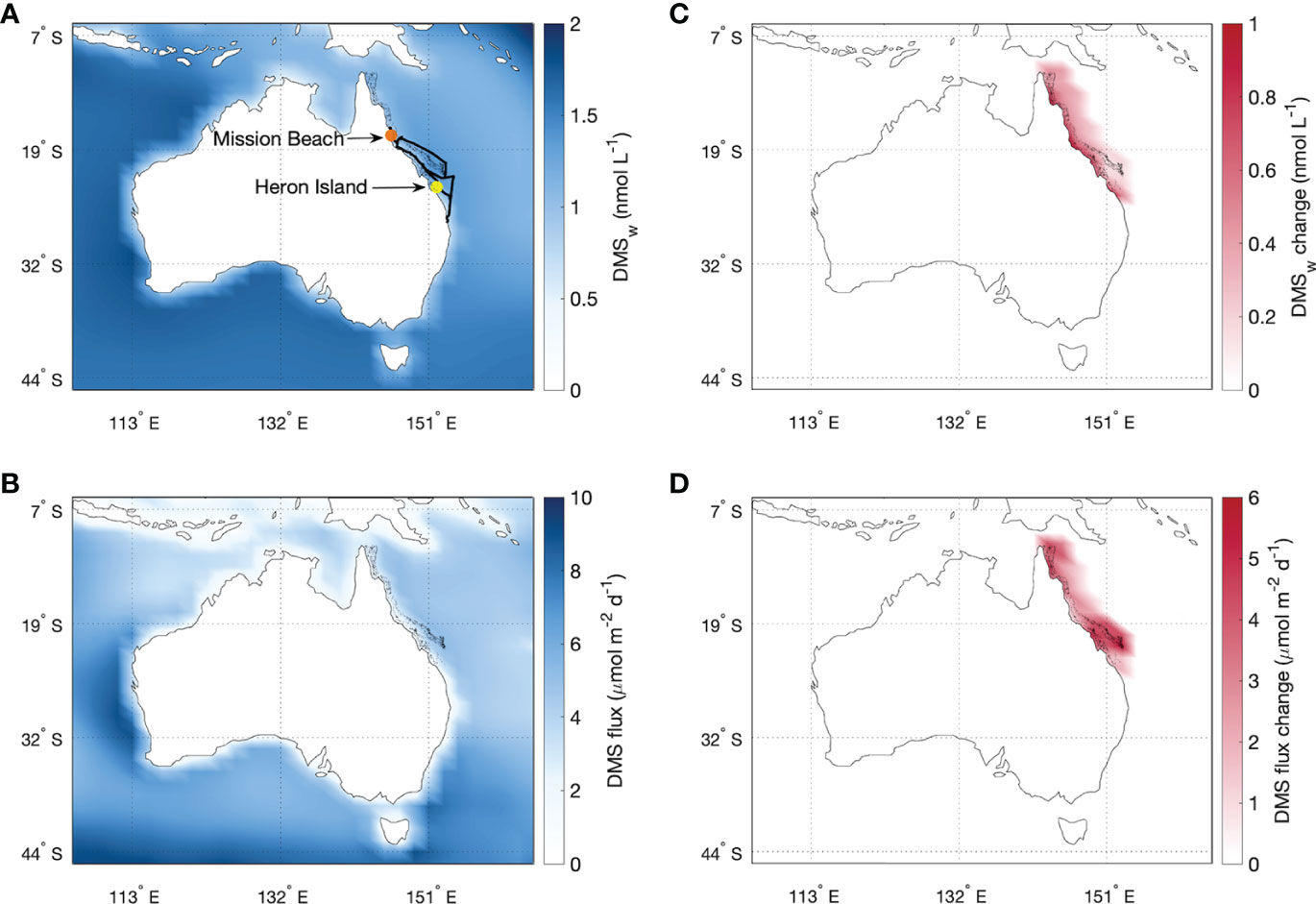
Figure 1 Annual mean (A) DMSw and (B) DMS sea-air flux based on the K99 climatology, and the change in each variable due to the inclusion of (C) the GBR DMS climatology and (D) the GBR DMS climatology and coral-air DMS flux. The RVI path (black), Mission Beach (orange) and Heron Island (yellow) survey locations are shown in (A).
The GBR climatology was derived from an empirical relationship between DMSw, SST and PAR, measured during the RVI surveys from 28 September to 24 October 2016. The RVI surveys were undertaken as part of the ‘Great Barrier Reef as a significant source of climatically relevant aerosol particles’ project, known as the ‘Reef to Rainforest’ (R2R) campaign. The RVI path is shown in Figure 1A. The calculated GBR DMS climatology agrees with the range of observed seasonal and spatial (lagoon versus reef flat) seawater DMS concentrations at several locations in the GBR (summarized in Jones et al., 2018) and is considered to be a reasonable representation of DMSw produced by corals and other marine organisms in GBR waters.
2.2.2 DMS sea-air and coral-air flux
DMS sea-air flux is calculated using the Liss and Merlivat (1986) parameterisation (Figure 1B). Current DMS sea-air flux parameterisations do not account for direct release of DMS from coral mucous when the reef is exposed to air during low tide. Coral aerial exposure is an important, albeit intermittent, source of atmospheric DMS (DMSa) (Andreae et al., 1983), that can lead to rapidly released plumes of DMSa over coral reefs (Jones et al., 2007; Swan et al., 2017). DMSa plumes over aerially exposed coral reefs are significantly more concentrated than the background DMSa signal, for which the seasonal average ranges from approximately 1 nmol m-3 (~25 ppt) in winter to 4 nmol m-3 (~100 ppt) in summer over the GBR (Swan et al., 2017).
It has been estimated that Acropora corals exposed to air release 9-35 μmol m-2 d-1 (mean 22 μmol m-2 d-1), according to measurements of gas-phase DMSa in chamber experiments containing Acropora corals exposed to air (Hopkins et al., 2016). To account for direct coral-air DMS flux, the mean of the Hopkins et al. (2016) laboratory-derived estimate was added to the modelled DMS sea-air flux, scaled by the percentage of coral reef cover (i.e. coral-air flux = reef fraction x 22). The fraction of reef cover was calculated as the number of reef pixels within a 0.25-degree grid (as determined in Jackson et al., 2021), using a database of coral reef locations obtained from ReefBase (https://www.refbase.org) in MATLAB R2020a. The estimate of coral cover across the GBR ranged from 0.01-34% within the 0.25 degree-resolution grid.
The estimate of coral-air DMS flux used in Jackson et al. (2021) was scaled up to the model grid by binning the mean values to a resolution of 1.25 x 1.875 degrees. The resulting coral-air DMS flux estimate ranged from 0.2-2.6 μmol m-2 d-1 and added an average of 1.4 μmol m-2 d-1 of DMS to the regional sea-air flux. Our estimate may be a conservative representation of coral-air DMS flux, given that it is two orders of magnitude lower than the 0.01-0.02 Tg yr-1 estimate of Hopkins et al. (2016).
The approach used to estimate coral-air DMS flux is limited as it assumes that Acropora spp. are the sole source of DMS and it does not account for variability in the extent of coral exposure or the complexity of the reef environment (Hopkins et al., 2016). Further research is needed to reduce the uncertainty in coral-air DMS flux, to accurately scale laboratory-derived fluxes to the natural coral reef environment, and to account for diurnal variability in DMS coral-air flux and DMSa concentration with variability in tidal cycles and photo-oxidation rates, respectively. Nevertheless, inclusion of the coral-air DMS flux estimate provides a more accurate estimate of total DMS flux from the GBR.
Incorporating both the GBR climatology and coral-air DMS flux into the model increased DMS flux from the GBR by an average of 3 μmol m-2 d-1 (Figure 1D); equivalent to 0.02 Tg yr-1 of DMS. In this study, coral-reef-derived DMS refers to the total contribution of DMS from corals and other organisms within the GBR, and includes the GBR DMSw climatology and coral-air DMS flux estimates described above.
2.3 Analysis
Simulations were run for a 12-month period from January to December 2016, to coincide with three surveys undertaken as part of the R2R campaign in February and September-October 2016. Meteorology in the model was nudged to the ERA-5 data set (Hersbach et al., 2020). Although nudging dampens meteorological responses, this method allows simulated responses to be attributed to a change in an independent variable (e.g. DMSw and coral-air DMS flux), while eliminating the need to run computationally expensive, long-term simulations to account for internal model variability. Nudging also allows the best comparison with field observations, by minimising uncertainty associated with meteorology.
As part of the R2R campaign, measurements were taken on board the RVI from 28 September to 24 October 2016. During the same period, shore-based measurements were made using the Atmospheric Integrated Research Facility for Boundaries and Oxidative Experiments (AIRBOX) mobile atmospheric chemistry laboratory at Mission Beach (17.82°S; 146.12°E) from 20 September to 16 October 2016. An overview paper describing these two field campaigns and datasets is currently in preparation (Trounce et al.,). A prior survey was undertaken at Heron Island (23.44°S; 151.91°E) on the southern GBR from 5 to 18 February 2016 (Swan and Jones, 2017; see also Deschaseaux et al., 2022). The RVI path, Mission Beach and Heron Island survey locations are shown in Figure 1A. A brief description of these surveys undertaken for the R2R campaign and methods used are provided in the Supplementary Information (SI).
To investigate whether the model output represents observations, modelled DMSa (parts per trillion or pmol mol-1) and wind speed (m s-1) are compared with hourly mean observations for the RVI, Mission Beach and Heron Island survey periods and coordinates (± 0.05 degrees). Given the coarse model resolution, modelled DMSa and wind speed were first interpolated to a 0.1-degree (~10 km) grid using a linear interpolation in MATLAB v2020a.
2.3.1 Experiment 1
For the control simulation, DMSw was prescribed from the oceanic K99 climatology (Figure 1A). For the experimental simulation, DMSw in the GBR region is prescribed from the GBR climatology and includes the estimate of coral-air DMS flux (henceforth GBR DMS). The change in annual mean DMSa, gas-phase SO2 and H2SO4 (ppt), sulfate aerosol mass (ppt), the number concentration of aerosol in the four soluble GLOMAP size modes (m-3), aerosol with dry diameter greater than 3 nm (N3) (cm-3), CCN with dry diameter greater than 70 nm (CCN70) (cm-3) and cloud droplets (Nd) (m-3), and surface downwelling short-wave radiation (SWR) (W m-2) is then quantified between the control and experimental simulations over north-eastern Australia (9.5-26°S; 135-155°E). The analysis focused on this region to capture changes due to the inclusion of coral-reef-derived DMS.
2.3.2 Experiment 2
Given the global and Australian Government initiatives to shift towards renewable energy, anthropogenic sulfur emissions may decline in future. Therefore, it is important to understand the relative importance of biogenic and anthropogenic sulfate aerosol sources, and the effects of a change in emissions on the aerosol system. The influence of coral-reef-derived DMS in a relatively clean atmosphere is investigated by repeating the control and experimental simulations described above, with high level anthropogenic SO2 emissions from north-eastern Australia removed from the model. ‘High level’ anthropogenic SO2 in ACCESS-AM2 includes 100% of energy sector emissions, and 50% of industrial emissions. Given that the focus of this study is on atmospheric sulfate, other anthropogenic emissions (e.g. black carbon) were not modified, but could also have an impact.
3 Results
3.1 Comparison of observed and modelled data
Simulations using K99 and GBR DMS performed similarly in predicting observations of DMSa at the local, hourly scale (Figures 2A–C). Both K99 and GBR DMS sources overestimated observed DMSa during the R2R surveys (Table 1). Given that the nudged model simulations predicted observed wind speed moderately well (r > 0.5, p < 0.001) (Figures 2D–F), the differences between observed and modelled DMSa are largely due to DMSw and coral-air DMS flux sources in the model. Nevertheless, modelled DMSa was within the range of concentrations previously reported for the GBR, which range from approximately 50-200 ppt (mean ~100 ppt) in summer (Jones et al., 2007; Swan et al., 2017; Swan and Jones, 2020; 2017) and can exceed 500 ppt over aerially exposed coral reefs (Jones et al., 2007; Swan et al., 2017). Therefore, the DMSw climatologies in the model are assumed to represent the upper range of observed DMS in the GBR.
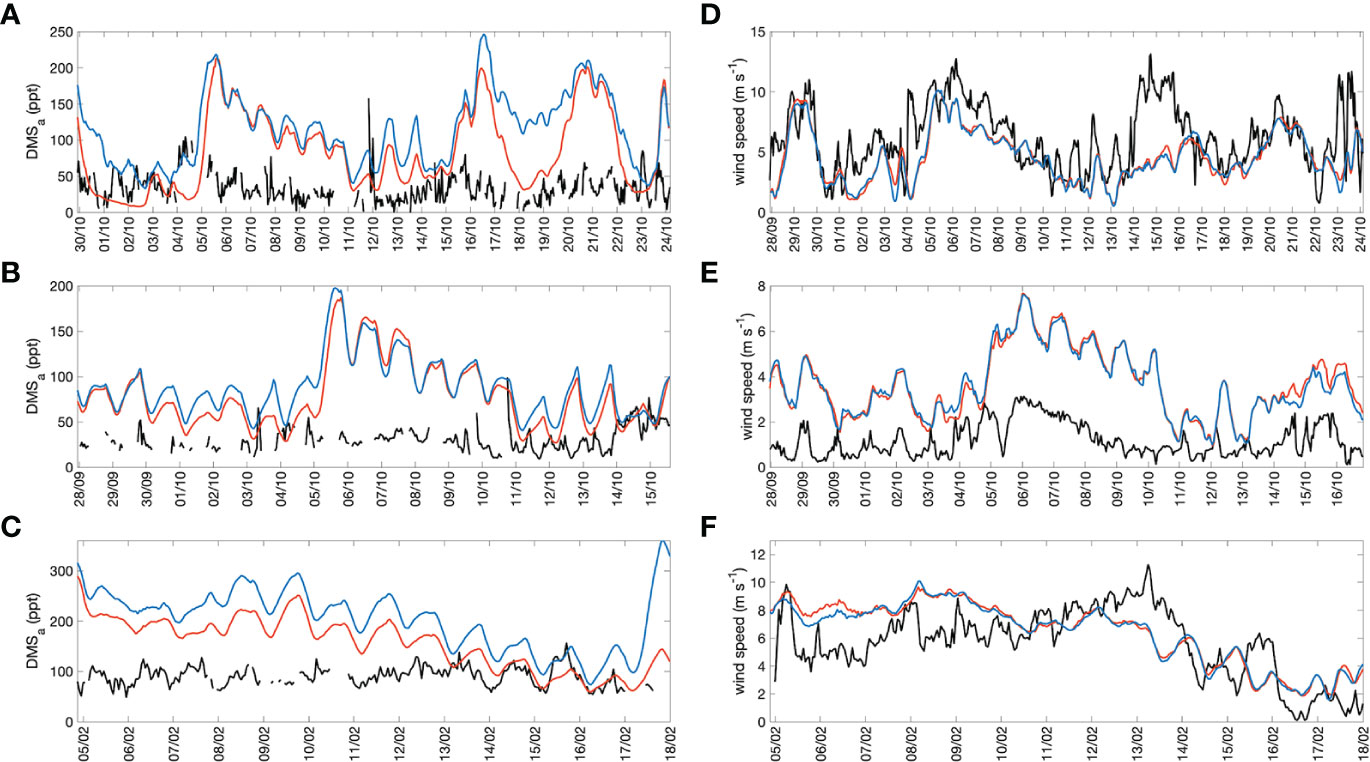
Figure 2 Time-series of observed (black) and modelled (left panels) DMSa and (right panels) wind speed for the K99 (orange) and GBR DMS (blue) simulations during the (A, D) RVI, (B, E) Mission Beach and (C, F) Heron Island surveys.
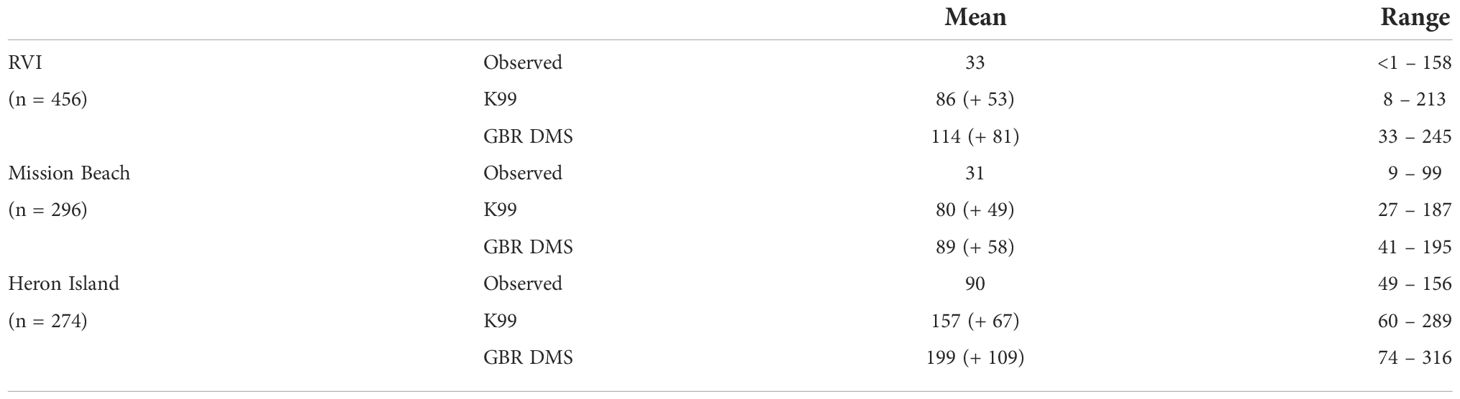
Table 1 Mean and range of observed and modelled DMSa (ppt or pmol mol-1) during the RVI, Mission Beach and Heron Island surveys of the R2R campaign [concentration (deviation from observed mean)].
DMSa concentration varies with the rate of DMS sea-air flux (which is primarily dependent on seawater surface DMS concentration, SST and wind speed) (Yang et al., 2011), coral-air DMS flux (Swan et al., 2017) and photochemical processes such as production of OH and subsequent oxidation of DMSa to sulfate aerosol precursor compounds (Ayers and Gillett, 2000; Barnes et al., 2006). Consequently, observed and modelled DMSa display a diel cycle (Figures 2A–C), decreasing around midday when solar radiation and the abundance of oxidative free radicals are highest (Gabric et al., 2008; Galí et al., 2013; Jackson et al., 2020). Increases in modelled DMSa often aligned with modelled wind speed, reflecting wind-driven sea-air flux (Figure 2). Occasional pulses in modelled DMSa occurred for the GBR DMS simulation during low wind speeds, reflecting the addition of coral-air flux (e.g. on 18 February 2016; Figure 2C). Conversely, observations of DMSa and wind speed did not align well, possibly reflecting advection to and from the measurement platform.
Observations of DMSa at Heron Island were approximately two-times higher than the RVI and Mission Beach observations, highlighting the variability in DMS concentrations in the GBR. It was expected that observed DMSa would be highest at Heron Island, given that measurements were taken less than 100 m from the coral reef flat and were more likely to capture the high end of atmospheric concentrations over the reef. Further, the RVI and Mission Beach surveys took place six months after the March-April 2016 mass coral bleaching event on the GBR, which led to the loss of up to 40% of hard coral cover by November 2016 (Hughes et al., 2018). Observed DMSw (not shown here) and DMSa were lower during the RVI and Mission Beach surveys than previously reported for the GBR region (Swan and Jones, 2017; Jones et al., 2018, 2020), which may have been in part due to the loss of DMS-producing hard corals. Prior to the 2016 mass coral bleaching event, DMSa measured at Heron Island ranged from 49-156 ppt (Table 1). However, with the exception of a pulse of DMSa on 12 October 2016, DMSa was below 100 ppt during the RVI and Mission Beach surveys (Figures 2A, B).
Considering that the K99 and GBR DMS sources are monthly mean climatologies (and fixed coral-air DMS flux) with no diurnal variation, a degree of variability was expected between the observed and coarse resolution modelled DMSa. While modelled DMSa represents the upper range of observations previously reported for the GBR region, simulations using K99 and GBR DMS sources still provide valuable insight into the influence of coral-reef-derived DMS on the regional atmosphere. Further research will improve our understanding of the complex coral reef sulfur cycle and will further improve the representation of coral-reef-derived DMS in climate models.
3.2 Influence of coral-reef-derived DMS over north-eastern Australia
Inclusion of coral-reef-derived DMS increased annual mean DMSa concentration over the GBR by approximately 150% (Figures 3A, D), resulting in an area-averaged increase of 29% (29.4 ppt) over north-eastern Australia (Table 2). Similarly, annual mean gas-phase SO2 and H2SO4 increased by a respective 1% and 3% over north-eastern Australia, in part due to the increase in DMSa over the GBR, where the strongest increase in SO2 (up to 17.6%) and H2SO4 (up to 14.9%) occurred (Figure 3). Regardless of the increase in SO2 and H2SO4 (FigureS 3E, F), concentrations over marine areas are negligible in comparison to industrial regions of coastal and inland Australia (Figures 3B, C).
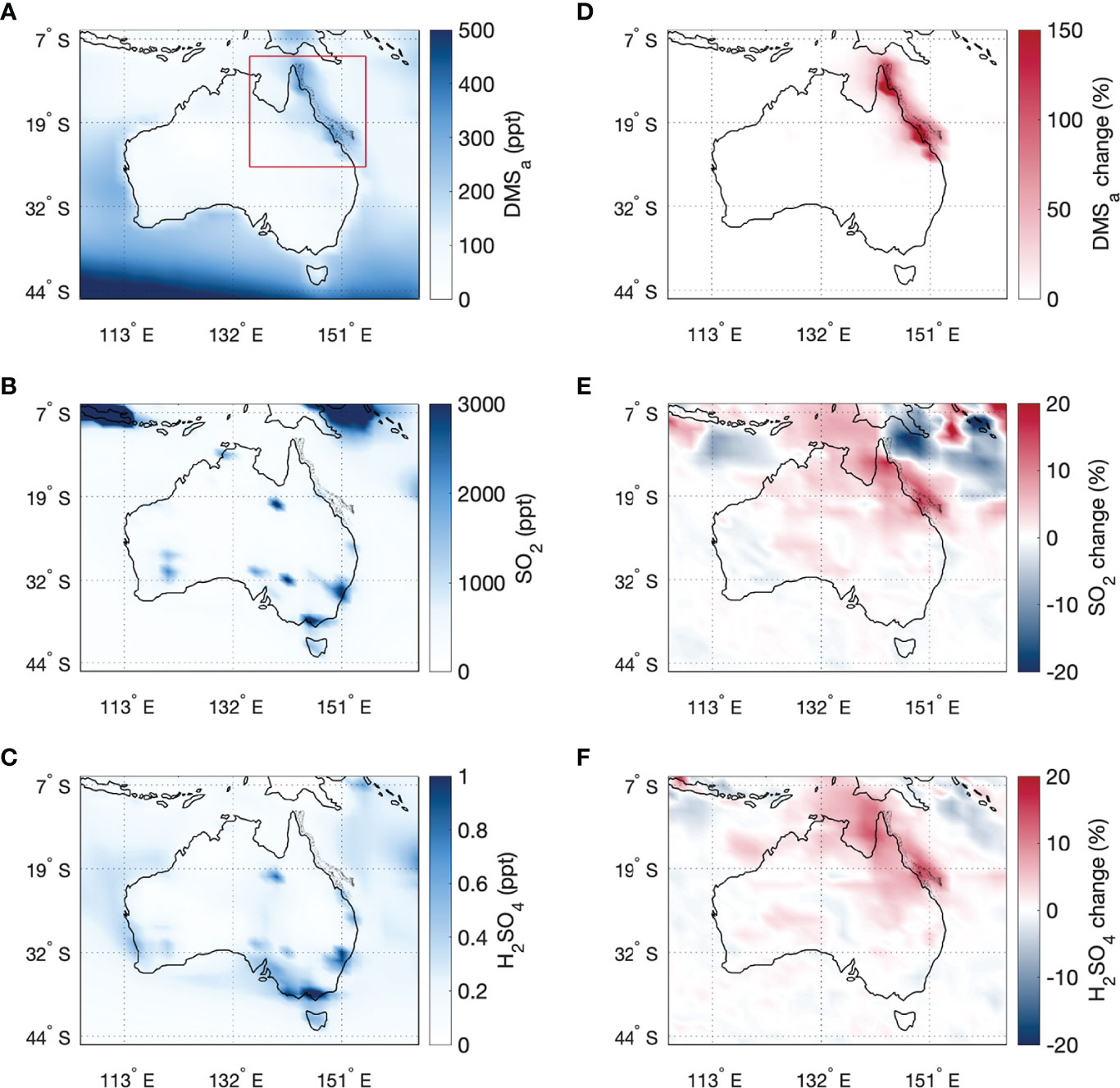
Figure 3 Annual mean (A) DMSa, (B) SO2 and (C) H2SO4 for the GBR DMS simulation, and (D–F) the percentage change in each variable between the GBR DMS and K99 simulations. The north-eastern Australian region (9.5-26°S; 135-155°E) for which the area-averaged changes are calculated for Table 2 is shown in red in panel (A).
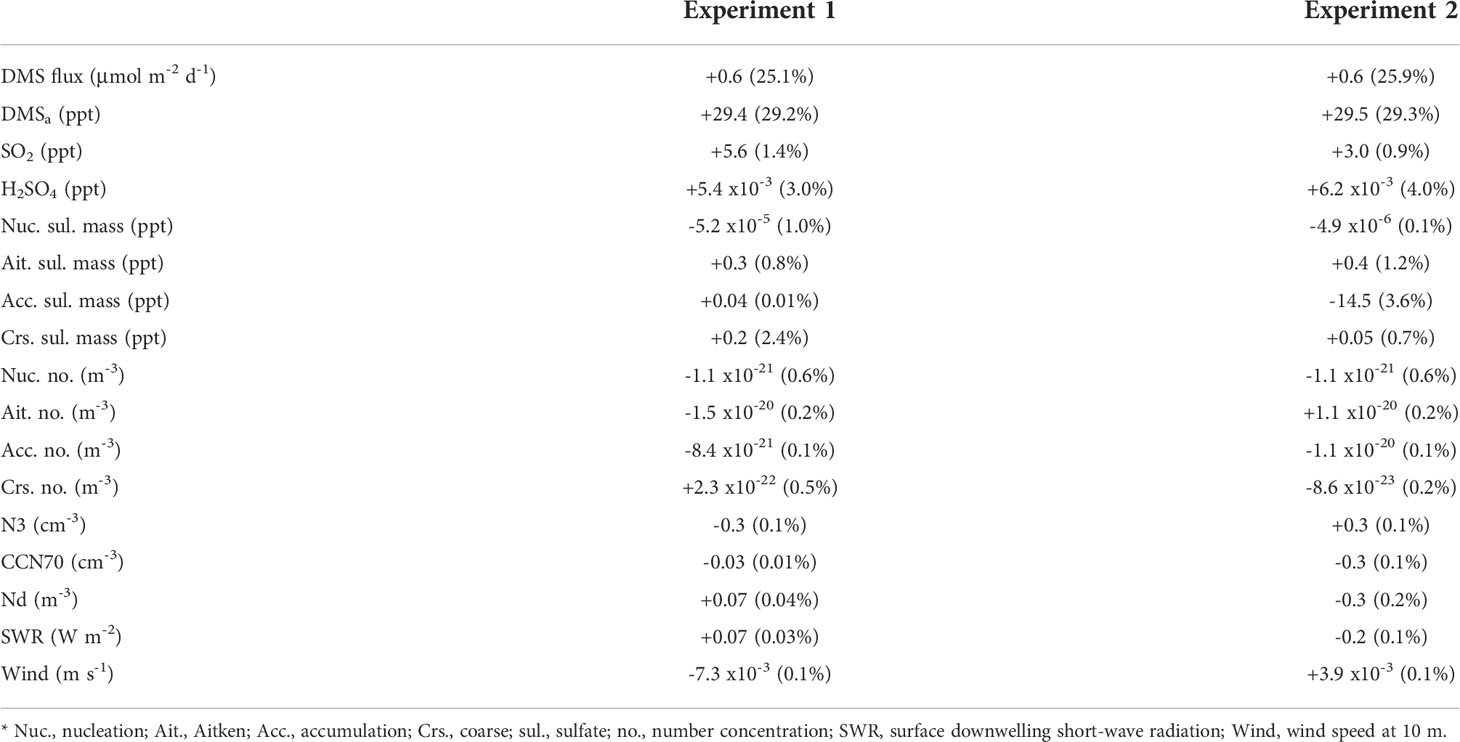
Table 2 Annual mean change [actual (percentage)] between the GBR DMS and K99 simulations area-averaged over north-eastern Australia.
Changes for annual mean sulfate aerosol mass and number concentration over north-eastern Australia were small (≤ 2.4%), yet revealed some interesting results. Nucleation mode sulfate mass and number concentration decreased by a respective -1% and -0.6% over north-eastern Australia, while sulfate mass increased by similar magnitude for the Aitken mode (+0.8%) and to a lesser extent the accumulation mode (+0.01%) (Table 2). A small increase in sulfate mass (+2.4%) and number concentration (+0.5%) also occurred for coarse mode aerosols. The small increase in Aitken and accumulation mode mass without a corresponding increase in number suggests that additional sulfate contributed to the growth of these size modes via condensation or coagulation on existing particles.
Negligible changes occurred for N3, CCN70, Nd and surface SWR (≤ 0.1%) that were likely driven by internal model and meteorological variability. While meteorology in the model was nudged to the ERA-5 dataset, minor differences in meteorological fields can still occur between model runs (as occurred in this study for wind speed; see Table 2). A time-series of each variable area-averaged over north-eastern Australia is provided in SI Figure 1. Mapped changes for sulfate aerosol mass, particle number concentrations and SWR are provided in SI Figures 2–4.
Similarly to previous model studies (Fiddes et al., 2021; Fiddes et al.,2022), the weak response to the addition of coral-reef-derived DMS may be due to the dominant influence of anthropogenic sulfur and other emissions from north-eastern Australia (Chen et al., 2019). Anthropogenic sources can dominate aerosol emissions, particularly over coastal and inland regions. Consequently, the dominant pathway for biogenic trace gases in the marine boundary layer is condensation or coagulation onto existing particles, often resulting in aerosol growth rather than new particle nucleation (Woodhouse et al., 2013; Hoffmann et al., 2016).
Given the response detected for DMSa and gas-phase sulfate aerosol precursor compounds (SO2 and H2SO4) at the annual mean time-scale, a stronger response in aerosol mass and number concentration may occur at local or sub-daily time scales as observational studies have suggested (Modini et al., 2009; Swan et al., 2016; Cropp et al., 2018), or with a reduction in anthropogenic pollutants. The latter is investigated below, where the influence of coral-reef-derived DMS is quantified when high-level anthropogenic SO2 emissions (e.g. from industrial chimneys, shown as hotspots exceeding 2000 ppt in Figure 3B) are removed from north-eastern Australia.
3.3 Influence of coral-reef-derived DMS in a relatively clean atmosphere
After removing high level anthropogenic SO2 emissions, the inclusion of coral-reef-derived DMS again increased DMSa, SO2 and H2SO4 concentrations over north-eastern Australia (Table 2). The increases are similar in space and magnitude to the results presented in section 3.2 above, however are stronger over the GBR (Figure 4). The stronger response is likely due to the addition of DMS-derived sulfur to a reduced pool of atmospheric SO2 and H2SO4 concentrations.
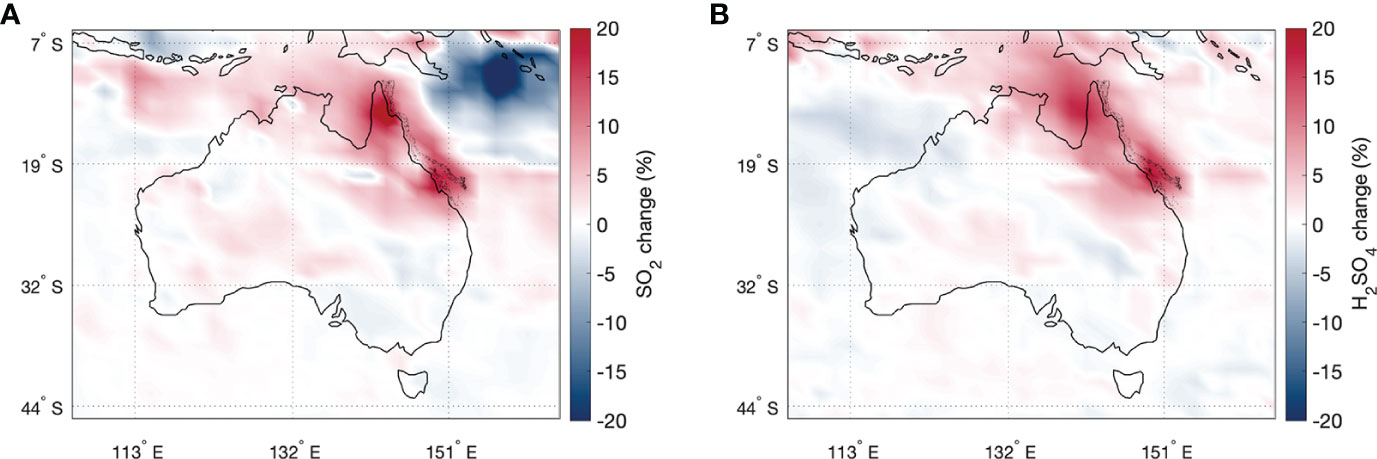
Figure 4 Change in annual mean (A) SO2 and (B) H2SO4 between the GBR DMS and K99 simulations, when high anthropogenic SO2 emissions from north-eastern Australia have been removed.
The change in sulfate aerosol mass and particle number concentration between the K99 and GBR DMS simulations were small (≤ 3.6%) (Table 2; SI Figure 5). Sulfate aerosol mass and number concentration decreased for the nucleation and accumulation mode, yet increased for the Aitken mode. For coarse mode aerosols, there was an increase in sulfate mass, without a corresponding increase in number concentration (Table 2). As for section 3.2, the inconsistent changes in sulfate mass and number concentration between aerosol size modes suggests that DMS-derived sulfate may be a more important contributor to aerosol mass and growth of existing aerosols, rather than nucleation of new particles at the monthly and annual mean time-scale, even with a reduction in anthropogenic sulfur emissions.
4 Discussion
Inclusion of coral-reef-derived DMS added an average of 0.5 nmol L-1 to the sea surface DMS climatology and 3 μmol m-2 d-1 (0.02 Tg yr-1) to the DMS sea-air flux for the GBR region. The additional DMS increased modelled annual mean DMSa concentration by approximately 150% over the GBR and by 29% over north-eastern Australia. The concentration of gas-phase sulfate aerosol precursor compounds (SO2 and H2SO4) increased by up to 17.6% over the GBR, supporting previous findings that the GBR is an important regional source of atmospheric sulfur (Broadbent and Jones, 2004; Jones et al., 2018; Jackson et al., 2021).
The increase in DMSa and subsequent increase in sulfate aerosol precursors is primarily attributed to the inclusion of coral-to-air DMS flux. The GBR DMSw climatology did not substantially increase seawater surface DMS concentration (< 1 nmol L-1; Figure 1C), and likely had a minor contribution to the ~150% increase in DMSa (Figure 3D). Previous field work has suggested that coral-air DMS flux is a stronger, although intermittent, contributor to DMSa than sea-air flux in the GBR (Swan et al., 2017), leading to plumes of DMSa above coral reefs that can exceed 500 ppt (Jones et al., 2007; Swan et al., 2017). This study supports this hypothesis, where an increase in DMSa, SO2 and H2SO4 was detected over the GBR (Figure 3), primarily due to the inclusion of coral-air DMS flux.
Gas-phase SO2 may be further oxidised to H2SO4, which may condense onto existing particles as the dominant pathway within the marine boundary layer (Woodhouse et al., 2013; Hoffmann et al., 2016), or nucleate to form new nss-SO4 particles (Andreae and Crutzen, 1997). Despite the increase in gas-phase sulfate aerosol precursors, coral-reef-derived DMS had no clear influence on modelled sulfate aerosol mass or number concentration at the monthly or annual mean time-scale, supporting previous model studies (Fiddes et al., 2021; Fiddes et al., 2022) which demonstrate that DMS-derived sulfates do not play an important role in aerosol formation or growth over large temporal and spatial scales. Fiddes et al. (2021) estimated that DMSw derived from global tropical coral reefs contributed 0.3 Tg yr-1 S to the global sea-air flux in the global ACCESS-UKCA atmospheric model. Despite modelled DMS flux being an order of magnitude higher than the current study, Fiddes et al. (2021) found that coral-reef-derived DMS had only a minor influence on sulfate aerosol mass and number concentration for nucleation and Aitken mode aerosols, and no significant influence on cloud-relevant particles or the Earth’s radiative balance. Fiddes et al. (2022) reported similar findings in the GBR region using the regional WRF-Chem model.
Small (≤ 2.4%) and inconsistent changes in sulfate aerosol mass and number concentration over north-eastern Australia in the present study are more likely due to internal model variability and minor changes in meteorology, than to coral-reef-derived DMS. While meteorology was nudged to the ERA-5 dataset, minor differences between model runs can occur. In this analysis, annual mean wind speed at 10 m differed by ± 0.1% between the control and experimental simulations (Table 2).
In comparison to the increase in gas-phase sulfate aerosol precursors, the negligible change in aerosol mass and number concentration may be due to the dominant influence of anthropogenic and other natural emissions (e.g. dust) from north-eastern Australia (Chen et al., 2019). To investigate whether the sensitivity of aerosol to DMS increased in a relatively less polluted atmosphere, we repeated the simulations with reduced anthropogenic SO2 emissions from north-eastern Australia; representing an optimistic future scenario where a shift to renewable energy may reduce anthropogenic pollutants. It was hypothesised that by reducing emissions from industrial hotspots, such as Gladstone in south-east Queensland, a stronger response in aerosol mass and number concentration to DMS might be detected. However, the results presented in section 3.3 highlight the complexity and non-linearity of the aerosol system, where a change in aerosol sources does not necessarily result in a change in aerosol composition or concentration of the same direction or magnitude.
Addition of coral-reef-derived DMS to a simulated cleaner regional atmosphere did increase DMSa, SO2 and H2SO4. However, no clear influence was detected for sulfate aerosol mass or particle number concentration, suggesting that even with a reduction in anthropogenic sulfur emissions, coral-reef-derived DMS may not play a significant role in aerosol production and processing over the GBR. Again, negligible (≤ 3.6%) changes occurred that were not restricted to the GBR region. These changes are more likely due to model and meteorological variability than coral-reef-derived DMS and so are not discussed further here.
While this analysis provides further insight into the influence of coral-reef-derived DMS on the contemporary and relatively clean regional atmosphere, further research is needed to improve DMS sources in the model. Simulations using both K99 and GBR DMS overestimated observed DMSa during the 2016 R2R campaign, yet modelled concentrations were still within the range previously reported for the GBR (Swan and Jones, 2017; Jones et al., 2018; Swan and Jones, 2020). As discussed above, the R2R surveys were taken approximately two weeks prior, and six months after the 2016 mass coral bleaching event, which led to the loss of up to 40% of hard coral cover in the GBR (Hughes et al., 2018). The loss of DMS-producing corals may be one reason why observed DMSw and DMSa during the RVI and Mission Beach surveys were low compared to previous surveys and modelled concentrations, highlighting the complexity in predicting coral-reef-derived DMS.
Further research is also required to understand the aerosol chemistry system and how it responds to changes in anthropogenic and natural emissions sources. While DMS emissions from the GBR did not influence aerosol mass or number concentration at the scales modelled in this study, observational studies suggest that DMS may be more important in local or sub-daily feedbacks. Nucleation events forming new particles that primarily consist of sulfates have been observed over the GBR (Modini et al., 2009), and coincided with elevated DMSa concentrations on one occasion in the southern GBR (Swan et al., 2016). Further, DMS emissions and DMSa concentration are positively correlated with atmospheric particle number concentration (>0.5 μm wet diameter) at Heron Island, likely reflecting condensational growth of smaller aerosols to the detectable size range (Jackson et al., 2020; Deschaseaux et al., 2022). Given that DMS emissions from the GBR are comparable to other highly productive regions such as the Southern Ocean or North Atlantic, where DMS-derived sulfates account for a significant portion of CCN (Korhonen et al., 2008; Sanchez et al., 2018), it is possible that DMS influences aerosol processes in the GBR at scales that are not captured by the coarse resolution global model.
5 Conclusions
This modelling study supports previous findings that the GBR is an important regional source of atmospheric sulfur. Inclusion of coral-reef-derived DMS increased the concentration of atmospheric DMS by 150% and sulfate aerosol precursor compounds by up to 18% over the GBR, with potential implications for local or sub-daily aerosol processes. However, no influence on modelled sulfate aerosol mass or particle number concentration was detected in this study. While reducing anthropogenic SO2 emissions did not strengthen the aerosol response to coral-reef-derived DMS, other anthropogenic and natural (e.g. dust) emissions from north-eastern Australia may confound the biogenic sulfur signal, particularly over large temporal and spatial scales. A stronger response to coral-reef-derived DMS may occur at local, sub-daily time-scales, that are perhaps not captured by global or regional climate models, or in the absence of anthropogenic emissions. However, further research is needed to disentangle the relative influence of natural and anthropogenic aerosol sources. Given the predicted rates of coral reef degradation and the global initiatives to shift towards renewable energy, it is important to understand how the complex aerosol-cloud system will respond to changes in natural and anthropogenic emissions.
Data availability statement
The ACCESS-AM2 atmospheric model used for this study is a licensed product of the UK Met Office and is available to specific users under a license agreement. The model output can be provided on request. The Heron Island DMSa field data (Swan and Jones, 2017) are available from the Southern Cross University online repository (https://researchportal.scu.edu.au/). The RVI underway data (in2016_v05uwy5min_csv.zip) is available from the CSIRO Marine National Facility repository (https://marlin.csiro.au/) under a Creative Commons Attribution 4.0 License. The Heron Island wind speed data is available from H. B. Swan and E. S. M. Deschaseaux upon request. The RVI DMSa data, and Mission Beach DMSa and wind speed data are available from the relevant institutions upon request.
Author contributions
RJ completed the model simulations, data analysis and prepared the manuscript. MW provided guidance on the model setup, experimental design and analysis. HS, ED and HT provided field data. All authors contributed to the review and finalisation of the manuscript.
Funding
Research undertaken on Marine National Facility RV Investigator voyage IN2016_V05 (RVI) and the work of G. B. Jones, ED and HS at Heron Island was supported by Australian Research Council Discovery Project grant DP150101649 ‘The Great Barrier Reef as a significant source of climatically relevant aerosol particles’. RJ was supported by an Australian Government Research Training Program Scholarship, Commonwealth Scientific and Industrial Research Organisation post-graduate scholarship, and the Griffith University School of Environment and Science.
Acknowledgments
We acknowledge the use of the CSIRO Marine National Facility in undertaking this research. The authors gratefully acknowledge Professor Zoran Ristovski, Chief Scientist on the voyage IN2016_V05 ‘The Great Barrier Reef as a significant source of climatically relevant aerosol particles’, the crew of the Marine National Facility RV Investigator, and all Mission Beach onsite personnel from CSIRO, Southern Cross University and Queensland University of Technology for the provision of DMSa and wind speed measurements during the September-October 2016 field campaign. We also acknowledge the Australian Research Council for funding under the Discovery Project DP150101649. HS, ED and G. B. Jones are gratefully acknowledged for making available their Heron Island DMSa and wind speed datasets. ED and HS would also like to thank the staff of the Heron Island Research Station for support during their field work. The modelling was undertaken using the National Computational Infrastructure facilities, supported by the Australian Government. The work forms part of RJ’s PhD thesis, entitled ‘Coral reefs as a source of dimethylsulfide (DMS) and the influence on the atmosphere of the Great Barrier Reef’, which will be available on the Griffith University online repository (https://research-repository.griffith.edu.au) from 14 June 2023.
Conflict of interest
The authors declare that the research was conducted in the absence of any commercial or financial relationships that could be construed as a potential conflict of interest.
Publisher’s note
All claims expressed in this article are solely those of the authors and do not necessarily represent those of their affiliated organizations, or those of the publisher, the editors and the reviewers. Any product that may be evaluated in this article, or claim that may be made by its manufacturer, is not guaranteed or endorsed by the publisher.
Supplementary material
The Supplementary Material for this article can be found online at: https://www.frontiersin.org/articles/10.3389/fmars.2022.910423/full#supplementary-material
References
Andreae M. O. (1995). “Climatic effects of changing atmospheric aerosol levels,” in In world survey of climatology (Netherlands: Elsevier), vol. 16, 347–398. doi: 10.1016/S0168-6321(06)80033-7
Andreae M. O., Barnard W. R., Ammons J. M. (1983). The biological production of dimethylsulfide in the ocean and its role in the global atmospheric sulfur budget. Ecol. Bulletins. 35, 167–177.
Andreae M. O., Crutzen P. J. (1997). Atmospheric aerosols: Biogeochemical sources and role in atmospheric chemistry. Science 276 (5315), 1052–1058. doi: 10.1126/science.276.5315.1052
Andreae M. O., Rosenfeld D. (2008). Aerosol–cloud–precipitation interactions. part 1. the nature and sources of cloud-active aerosols. Earth-Science. Rev. 89 (1–2), 13–41. doi: 10.1016/j.earscirev.2008.03.001
Ayers G. P., Gillett R. W. (2000). DMS and its oxidation products in the remote marine atmosphere: Implications for climate and atmospheric chemistry. J. Sea. Res. 43 (3–4), 275–286. doi: 10.1016/S1385-1101(00)00022-8
Barnes I., Hjorth J., Mihalopoulos N. (2006). Dimethyl sulfide and dimethyl sulfoxide and their oxidation in the atmosphere. Chem. Rev. 106 (3), 940–975. doi: 10.1021/cr020529+
Berndt T., Scholz W., Mentler B., Fischer L., Hoffmann E. H., Tilgner A., et al. (2019). Fast peroxy radical isomerization and OH recycling in the reaction of OH radicals with dimethyl sulfide. J. Phys. Chem. Lett. 10 (21), 6478–6483. doi: 10.1021/acs.jpclett.9b02567
Bi D., Dix M., Marsland S., O’Farrell S., Sullivan A., Bodman R., et al. (2020). Configuration and spin-up of ACCESS-CM2, the new generation Australian Community Climate and Earth System Simulator Coupled Model. J. South. Hemisphere. Earth Syst. Sci. 70 (1), 225–251. doi: 10.1071/ES19040
Bodman R. W., Karoly D. J., Dix M. R., Harman I. N., Srbinovsky J., Dobrohotoff P. B., et al. (2020). Evaluation of CMIP6 AMIP climate simulations with the ACCESS-AM2 model. J. South. Hemisphere. Earth Syst. Sci. 70 (1), 166–179. doi: 10.1071/ES19033
Broadbent A. D., Jones G. B. (2004). DMS and DMSP in mucus ropes, coral mucus, surface films and sediment pore waters from coral reefs in the Great Barrier Reef. Mar. Freshw. Res. 55 (8), 849–855. doi: 10.1071/MF04114
Buckee J., Pattiaratchi C., Verduin J. (2020). Partial mortality of intertidal corals due to seasonal daytime low water levels at the Houtman Abrolhos Islands. Coral. Reefs. 39 (3), 537–543. doi: 10.1007/s00338-019-01887-5
Bullock H. A., Luo H., Whitman W. B. (2017). Evolution of dimethylsulfoniopropionate metabolism in marine phytoplankton and bacteria. Front. Microbiol. 8. doi: 10.3389/fmicb.2017.00637
Carslaw K. S., Lee L. A., Reddington C. L., Pringle K. J., Rap A., Forster P. M., et al. (2013). Large Contribution of natural aerosols to uncertainty in indirect forcing. Nature 503 (7474), 67–71. doi: 10.1038/nature12674
Charlson R. J., Lovelock J. E., Andreae M. O., Warren S. G. (1987). Oceanic phytoplankton, atmospheric sulphur, cloud albedo and climate. Nature 326 (6114), 655–661. doi: 10.1038/326655a0
Chen Z., Schofield R., Rayner P., Zhang T., Liu C., Vincent C., et al. (2019). Characterization of aerosols over the Great Barrier Reef: The influence of transported continental sources. Sci. Total. Environ. 690, 426–437. doi: 10.1016/j.scitotenv.2019.07.007
Cropp R. A., Gabric A. J., van Tran D., Jones G., Swan H., Butler H. (2018). Coral reef aerosol emissions in response to irradiance stress in the Great Barrier Reef, Australia. Ambio 47 (6), 671–681. doi: 10.1007/s13280-018-1018-y
Curson A. R., Liu J., Martı́nez A. B., Green R. T., Chan Y., Carrión O., et al. (2017). Dimethylsulfoniopropionate biosynthesis in marine bacteria and identification of the key gene in this process. Nat. Microbiol. 2, 17001–17009. doi: 10.1038/nmicrobiol.2017.9
Dave P., Patil N., Bhushan M., Venkataraman C. (2019). “Aerosol influences on cloud modification and rainfall suppression in the south Asian monsoon region,” in Climate change signals and response. Eds. Venkataraman C., Mishra T., Ghosh S., Karmakar S. (Springer), 21–37. doi: 10.1007/978-981-13-0280-0_2
Deschaseaux E. S. M., Jones G. B., Deseo M. A., Shepherd K. M., Kiene R. P., Swan H. B., et al. (2014). Effects of environmental factors on dimethylated sulfur compounds and their potential role in the antioxidant system of the coral holobiont. Limnol. Oceanography. 59 (3), 758–768. doi: 10.4319/lo.2014.59.3.0758
Deschaseaux E. S. M., Swan H. B., Maher D., Jones G. B., Schulz K., Koveke E., et al. (2022). The interplay between dimethyl sulfide (DMS) and methane (CH4) in a coral reef ecosystem. Front. Mar. Science.: Coral. Reef. Res. doi: 10.3389/fmars.2022.910441
Fan J., Rosenfeld D., Zhang Y., Giangrande S. E., Li Z., Machado L. A. T., et al. (2018). Substantial convection and precipitation enhancements by ultrafine aerosol particles. Science 359 (6374), 411–418. doi: 10.1126/science.aan8461
Fiddes S. L., Woodhouse M. T., Lane T. P., Schofield R. (2021). Coral-reef-derived dimethyl sulfide and the climatic impact of the loss of coral reefs. Atmospheric. Chem. Phys. 21 (8), 5883–5903. doi: 10.5194/acp-21-5883-2021
Fiddes S. L., Woodhouse M. T., Nicholls Z., Lane T. P., Schofield R. (2018). Cloud, precipitation and radiation responses to large perturbations in global dimethyl sulfide. Atmospheric. Chem. Phys. 18 (14), 10177–10198. doi: 10.5194/acp-18-10177-2018
Fiddes S. L., Woodhouse M. T., Utembe S., Schofield R., Alexander S. P., Alroe J., et al. (2022). The contribution of coral-reef-derived dimethyl sulfide to aerosol burden over the Great Barrier Reef: A modelling study. Atmospheric. Chem. Phys. 22 (4), 2419–2445. doi: 10.5194/acp-22-2419-2022
Fischer E., Jones G. (2012). Atmospheric dimethysulphide production from corals in the Great Barrier Reef and links to solar radiation, climate and coral bleaching. Biogeochemistry 110 (1), 31–46. doi: 10.1007/s10533-012-9719-y
Gabric A. J., Matrai P. A., Kiene R. P., Cropp R. A., Dacey J. W. H., DiTullio G. R., et al. (2008). Factors determining the vertical profile of dimethylsulfide in the Sargasso Sea during summer. Deep. Sea. Res. Part II.: Topical Stud. Oceanography. 55 (10–13), 1505–1518. doi: 10.1016/j.dsr2.2008.02.002
Gabric A. J., Qu B., Rotstayn L., Shephard J. M. (2013). Global simulations of the impact on contemporary climate of a perturbation to the sea-to-air flux of dimethylsulfide. Aust. Meteorolog. Oceanographic. J. 63 (3), 365–376. doi: 10.22499/2.6303.002
Galí M., Simó R., Vila-Costa M., Ruiz-González C., Gasol J. M., Matrai P. (2013). Diel patterns of oceanic dimethylsulfide (DMS) cycling: Microbial and physical drivers. Global Biogeochem. Cycles. 27 (3), 620–636. doi: 10.1002/gbc.20047
Gardner S. G., Nielsen D. A., Laczka O., Shimmon R., Beltran V. H., Ralph P. J., et al. (2016). Dimethylsulfoniopropionate, superoxide dismutase and glutathione as stress response indicators in three corals under short-term hyposalinity stress. Proc. R. Soc. B.: Biol. Sci. 283 (1824), 20152418. doi: 10.1098/rspb.2015.2418
Hersbach H., Bell B., Berrisford P., Hirahara S., Horányi A., Muñoz-Sabater J., et al. (2020). The ERA5 global reanalysis. Q. J. R. Meteorolog. Soc. 146 (730), 1999–2049. doi: 10.1002/qj.3803
Hodshire A. L., Campuzano-Jost P., Kodros J. K., Croft B., Nault B. A., Schroder J. C., et al. (2019). The potential role of methanesulfonic acid (MSA) in aerosol formation and growth and the associated radiative forcings. Atmospheric. Chem. Phys. 19 (5), 3137–3160. doi: 10.5194/acp-19-3137-2019
Hoffmann E. H., Tilgner A., Schroedner R., Bräuer P., Wolke R., Herrmann H. (2016). An advanced modeling study on the impacts and atmospheric implications of multiphase dimethyl sulfide chemistry. Proc. Natl. Acad. Sci. 113 (42), 11776–11781. doi: 10.1073/pnas.1606320113
Hopkins F. E., Bell T. G., Yang M., Suggett D. J., Steinke M. (2016). Air exposure of coral is a significant source of dimethylsulfide (DMS) to the atmosphere. Sci. Rep. 6 (1), 1–11. doi: 10.1038/srep36031
Hughes T. P., Kerry J. T., Baird A. H., Connolly S. R., Dietzel A., Eakin C. M., et al. (2018). Global warming transforms coral reef assemblages. Nature 556 (7702), 492–496. doi: 10.1038/s41586-018-0041-2
IPCC (2013). Climate change 2013: The physical science basis. contribution of working group I to the fifth assessment report of the intergivernmental panel on climate change. Eds. Stocker T., Qin G., Plattner G., Tignor M., Allen S., Boschung J., et al (United Kingdom and NewYork, NY, USA: Cambridge University Press), pp. 1535.
IPCC (2021). “Summary for policymakers. in: Climate change 2021: The physical science basis,” in Contribution of working group I to the sixth assessment report of the intergovernmental panel on climate change. Eds. Masson-Delmotte V., Zhai P., Pirani A., Connors S. L., Pean C., Berger S., Caud N., Chen Y., Goldfarb L., Gomis M. I., Huang M., Leitzell K., Lonnoy E., Matthews J. B. R., Maycock T. K., Waterfield T., Yelekci O., Yu R., Zhou B. (United Kingdom and New York, NY, USA: Cambridge University Press), pp. 3–32.
IPCC (2014). Climate change 2014: Synthesis report. Contribution of working groups I, II and III to the fifth assessment report of the intergovernmental panel on climate change. [Core Writing Team, R. K. Pachauri and L. A. Meyer (eds.)]. IPCC, Geneva, Switzerland, pp. 151.
Jackson R. L., Gabric A. J., Matrai P. A., Woodhouse M. T., Cropp R. A., Jones G. B., et al. (2021). Parameterizing the impact of seawater temperature and irradiance on dimethylsulfide (DMS) in the Great Barrier Reef and the contribution of coral reefs to the global sulfur cycle. J. Geophysical. Research.: Oceans. 126 (3), e2020JC016783. doi: 10.1029/2020JC016783
Jackson R. L., Gabric A. J., Woodhouse M. T., Swan H. B., Jones G. B., Cropp R. A., et al. (2020). Coral reef emissions of atmospheric dimethylsulfide and the influence on marine aerosols in the southern Great Barrier Reef, Australia. J. Geophysical. Research.: Atmospheres. 125 (7), e2019JD031837. doi: 10.1029/2019JD031837
Jones G. B. (2015). “The reef sulphur cycle: Influence on climate and ecosystem services,” in In ethnobiology of corals and coral reefs (Switzerland: Springer), 27–57. doi: 10.1007/978-3-319-23763-3_3
Jones G. B., Curran M., Broadbent A., King S., Fischer E., Jones R. (2007). Factors affecting the cycling of dimethylsulfide and dimethylsulfoniopropionate in coral reef waters of the Great Barrier Reef. Environ. Chem. 4 (5), 310–322. doi: 10.1071/EN06065
Jones G. B., Curran M., Deschaseaux E. S. M., Omori Y., Tanimoto H., Swan H. B., et al. (2018). The flux and emission of dimethylsulfide from the Great Barrier Reef region and potential influence on the climate of NE Australia. J. Geophysical. Research.: Atmospheres. 123 (24), 13835–13856. doi: 10.1029/2018JD029210
Jones G. B., Curran M., Swan H. B., Deschaseaux E. S. M. (2017). Dimethylsulfide and coral bleaching: Links to solar radiation, low level cloud and the regulation of seawater temperatures and climate in the Great Barrier Reef. Am. J. Climate Change 6 (02), 328. doi: 10.4236/ajcc.2017.62017
Kettle A. J., Andreae M. O., Amouroux D., Andreae T. W., Bates T. S., Berresheim H., et al. (1999). A global database of sea surface dimethylsulfide (DMS) measurements and a procedure to predict sea surface DMS as a function of latitude, longitude, and month. Global Biogeochem. Cycles. 13 (2), 399–444. doi: 10.1029/1999GB900004
Korhonen H., Carslaw K. S., Spracklen D. V., Mann G. W., Woodhouse M. T. (2008). Influence of oceanic dimethyl sulfide emissions on cloud condensation nuclei concentrations and seasonality over the remote southern hemisphere oceans: A global model study. J. Geophysical. Research.: Atmospheres. 113 (D15). doi: 10.1029/2007JD009718
Lana A., Bell T. G., Simó R., Vallina S. M., Ballabrera-Poy J., Kettle A. J., et al. (2011). An updated climatology of surface dimethlysulfide concentrations and emission fluxes in the global ocean. Global Biogeochem. Cycles. 25 (1), GB1004. doi: 10.1029/2010GB003850
Lana A., Simó R., Vallina S. M., Dachs J. (2012). Potential for a biogenic influence on cloud microphysics over the ocean: A correlation study with satellite-derived data. Atmospheric. Chem. Phys. 12 (17), 7977–7993. doi: 10.5194/acp-12-7977-2012
Liss P. S., Merlivat L. (1986). Air-sea gas exchange rates: Introduction and synthesis. in. Role. air-sea. exchange. geochem. cycling. (Dordrecht: Springer) 185, 113–127. doi: 10.1007/978-94-009-4738-2_5
Mahajan A. S., Fadnavis S., Thomas M. A., Pozzoli L., Gupta S., Royer S., et al. (2015). Quantifying the impacts of an updated global dimethyl sulfide climatology on cloud microphysics and aerosol radiative forcing. J. Geophysical. Research.: Atmospheres. 120 (6), 2524–2536. doi: 10.1002/2014JD022687
Mann G. W., Carslaw K. S., Ridley D. A., Spracklen D. V., Pringle K. J., Merikanto J., et al. (2012). Intercomparison of modal and sectional aerosol microphysics representations within the same 3-d global chemical transport model. Atmospheric. Chem. Phys. 12 (10), 4449–4476. doi: 10.5194/acp-12-4449-2012
Mann G. W., Carslaw K. S., Spracklen D. V., Ridley D. A., Manktelow P. T., Chipperfield M. P., et al. (2010). Description and evaluation of GLOMAP-mode: A modal global aerosol microphysics model for the UKCA composition-climate model. Geoscientific. Model. Dev. 3 (2), 519–551. doi: 10.5194/gmd-3-519-2010
McCormick R. A., Ludwig J. H. (1967). Climate modification by atmospheric aerosols. Science 156 (3780), 1358–1359. doi: 10.1126/science.156.3780.1358
McGowan H., Sturman A., Saunders M., Theobald A., Wiebe A. (2019). Insights from a decade of research on coral reef–atmosphere energetics. J. Geophysical. Research.: Atmospheres. 124 (8), 4269–4282. doi: 10.1029/2018JD029830
McParland E. L., Levine N. M. (2019). The role of differential DMSP production and community composition in predicting variability of global surface DMSP concentrations. Limnol. Oceanography. 64 (2), 757–773. doi: 10.1002/lno.11076
Modini R. L., Ristovski Z. D., Johnson G. R., He C., Surawski N., Morawska L., et al. (2009). New particle formation and growth at a remote, sub-tropical coastal location. Atmospheric. Chem. Phys. 9 (19), 7607–7621. doi: 10.5194/acp-9-7607-2009
Raina J. B., Tapiolas D. M., Forêt S., Lutz A., Abrego D., Ceh J., et al. (2013). DMSP biosynthesis by an animal and its role in coral thermal stress response. Nature 502 (7473), 677–680. doi: 10.1038/nature12677
Ramanathan V., Cess R. D., Harrison E. F., Minnis P., Barkstrom B. R., Ahmad E., et al. (1989). Cloud-radiative forcing and climate: Results from the earth radiation budget experiment. Science 243 (4887), 57–63. doi: 10.1126/science.243.4887.57
Rosenfeld D., Dai J., Yu X., Yao Z., Xu X., Yang X., et al. (2007). Inverse relations between amounts of air pollution and orographic precipitation. Science 315 (5817), 1396–1398. doi: 10.1126/10.1126/science.1137949
Sanchez K. J., Chen C. L., Russell L. M., Betha R., Liu J., Price D. J., et al. (2018). Substantial seasonal contribution of observed biogenic sulfate particles to cloud condensation nuclei. Sci. Rep. 8 (1), 1–14. doi: 10.1038/s41598-018-21590-9
Stefels J. (2000). Physiological aspects of the production and conversion of DMSP in marine algae and higher plants. J. Sea. Res. 43 (3–4), 183–197. doi: 10.1016/S1385-1101(00)00030-7
Sunda W., Kieber D. J., Kiene R. P., Huntsman S. (2002). An antioxidant function for DMSP and DMS in marine algae. Nature 418 (6895), 317–320. doi: 10.1038/nature00851
Swan H. B., Crough R. W., Vaattovaara P., Jones G. B., Deschaseaux E. S. M., Eyre B. D., et al. (2016). Dimethyl sulfide and other biogenic volatile organic compound emissions from branching coral and reef seawater: Potential sources of secondary aerosol over the Great Barrier Reef. J. Atmospheric. Chem. 73 (3), 303–328. doi: 10.1007/s10874-016-9327-7
Swan H. B., Jones G. B. (2017). Atmospheric dimethylsulfide surface concentrations and supermicron particle number concentrations at heron island (23.44° s, 151.91° e) February 2016 (Southern Cross University). doi: 10.4226/47/59c460fbe8322
Swan H. B., Jones G. B. (2020). Database of surface level atmospheric dimethylsulfide (DMS) collected for the project “The Great Barrier Reef as a significant source of climatically relevant aerosol particles.” (Southern Cross University). doi: 10.25918/5c772187f39b6
Swan H. B., Jones G. B., Deschaseaux E. S. M., Eyre B. D. (2017). Coral reef origins of atmospheric dimethylsulfide at Heron Island, Southern Great Barrier Reef, Australia. Biogeosciences 14 (1), 229–239. doi: 10.5194/bg-14-229-2017
Thomas M. A., Suntharalingam P., Pozzoli L., Rast S., Devasthale A., Kloster S., et al. (2010). Quantification of DMS aerosol-cloud-climate interactions using the ECHAM5-HAMMOZ model in a current climate scenario. Atmospheric. Chem. Phys. 10 (15), 7425–7438. doi: 10.5194/acp-10-7425-2010
Trounce H., Ristovsky Z., Miljevic B., Cravigan L., Alroe J., Osuagwa C., et al. (in preparation) Great Barrier Reef aerosol and cloud data from the reef to rainforest campaign. Earth System. Sci. Data Discussions.
Twomey S. (1974). Pollution and the planetary albedo. Atmospheric. Environ. (1967). 8 (12), 1251–1256. doi: 10.1016/0004-6981(74)90004-3
Twomey S., Piepgrass M., Wolfe T. L. (1984). An assessment of the impact of pollution on global cloud albedo. Tellus. B. 36 (5), 356–366. doi: 10.1111/j.1600-0889.1984.tb00254.x
Veres P. R., Neuman J. A., Bertram T. H., Assaf E., Wolfe G. M., Williamson C. J., et al. (2020). Global airborne sampling reveals a previously unobserved dimethyl sulfide oxidation mechanism in the marine atmosphere. Proc. Natl. Acad. Sci. 117 (9), 4505–4510. doi: 10.1073/pnas.1919344117
Von Glasow R., Crutzen P. J. (2004). Model study of multiphase DMS oxidation with a focus on halogens. Atmospheric. Chem. Phys. 4 (3), 589–608. doi: 10.5194/acp-4-589-2004
Walters D., Baran A. J., Boutle I., Brooks M., Earnshaw P., Edwards J., et al. (2019). The met office unified model global atmosphere 7.0/7.1 and JULES global land 7.0 configurations. Geoscientific. Model. Dev. 12 (5), 1909–1963. doi: 10.5194/gmd-12-1909-2019
Woodhouse M. T., Carslaw K. S., Mann G. W., Vallina S. M., Vogt M., Halloran P. R., et al. (2010). Low sensitivity of cloud condensation nuclei to changes in the sea-air flux of dimethyl-sulphide. Atmospheric. Chem. Phys. 10 (16), 7545–7559. doi: 10.5194/acp-10-7545-2010
Woodhouse M. T., Mann G. W., Carslaw K. S., Boucher O. (2013). Sensitivity of cloud condensation nuclei to regional changes in dimethyl-sulphide emissions. Atmospheric. Chem. Phys. 13 (5), 2723–2733. doi: 10.5194/acp-13-2723-2013
Yang M., Blomquist B., Fairall C., Archer S., Huebert B. (2011). Air-sea exchange of dimethylsulfide in the Southern Ocean: Measurements from SO GasEx compared to temperate and tropical regions. J. Geophysical. Research.: Oceans. 116, C00F05. doi: 10.1029/2010JC006526
Keywords: coral reef, dimethylsulfide (DMS), sulfate, aerosol, ACCESS
Citation: Jackson RL, Woodhouse MT, Gabric AJ, Cropp RA, Swan HB, Deschaseaux ESM and Trounce H (2022) Modelling the influence of coral-reef-derived dimethylsulfide on the atmosphere of the Great Barrier Reef, Australia. Front. Mar. Sci. 9:910423. doi: 10.3389/fmars.2022.910423
Received: 01 April 2022; Accepted: 19 July 2022;
Published: 12 August 2022.
Edited by:
Rafel Simó, Institut de Ciències del Mar (ICM-CSIC), SpainReviewed by:
Michael Kingsford, James Cook University, AustraliaZhenming Ji, Sun Yat-sen University, China
Copyright © 2022 Jackson, Woodhouse, Gabric, Cropp, Swan, Deschaseaux and Trounce. This is an open-access article distributed under the terms of the Creative Commons Attribution License (CC BY). The use, distribution or reproduction in other forums is permitted, provided the original author(s) and the copyright owner(s) are credited and that the original publication in this journal is cited, in accordance with accepted academic practice. No use, distribution or reproduction is permitted which does not comply with these terms.
*Correspondence: Rebecca L. Jackson, cmViZWNjYS5qYWNrc29uQGNzaXJvLmF1
†Present address: Rebecca L. Jackson, Coasts and Ocean Research, Oceans and Atmosphere, Commonwealth Scientific and Industrial Research Organisation, Canberra, ACT, Australia