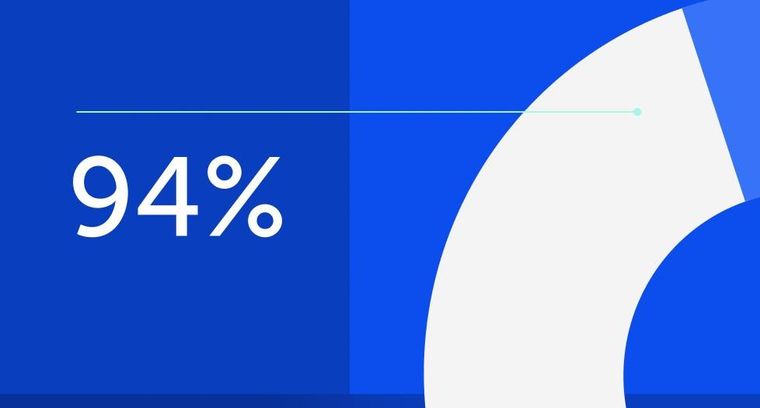
94% of researchers rate our articles as excellent or good
Learn more about the work of our research integrity team to safeguard the quality of each article we publish.
Find out more
ORIGINAL RESEARCH article
Front. Mar. Sci., 12 September 2022
Sec. Marine Ecosystem Ecology
Volume 9 - 2022 | https://doi.org/10.3389/fmars.2022.910417
This article is part of the Research TopicOpening the Black Box of Kelps: Response of Early Life Stages to Anthropogenic StressorsView all 9 articles
Kelp forests are experiencing substantial declines due to climate change, particularly ocean warming and marine heatwaves, and active interventions are necessary to halt this decline. A new restoration approach termed “green gravel” has shown promise as a tool to combat kelp forest loss. In this approach, substrata (i.e. small gravel) are seeded with kelp propagules, reared in controlled conditions in the laboratory before out-planting to degraded reefs. Here, we tested the feasibility of cultivating Australia’s dominant kelp, Ecklonia radiata on green gravel with the aim of optimising the seeding conditions for E.radiata. We seeded substrata (i.e. gravel), that had different surface texture and size, with E. radiata gametophytes at two average seeding densities: high density of ~230 fragments mL-1 and low density of ~115 fragments mL-1. The tested substrata were small basalt, large basalt, crushed laterite and limestone. Gametophytes successfully adhered to all four tested substrata, however, gametophytes that adhered to the limestone gravel (the natural reef type off Western Australia) suffered extreme tissue bleaching likely due to dissolution and decrease in seawater pH. Gametophytes that adhered to the three other test substrata were healthy, fertilised following seeding and microscopic sporophytes were observed attaching to the gravel. Substrata and seeding density did not affect sporophyte growth (i.e. length) at the time of transferring into aquarium tanks (after three months of rearing in incubators) but over time substrata showed a significant effect on maximum lengths. After 12 months in aquarium tanks, sporophytes on both small and large basalt gravel were significantly larger than those on the crushed laterite. Gametophytes were also found to not only survive on the gravel itself but also detach from the gravel, settle successfully, fertilise and develop into healthy sporophytes ex situ on the surrounding substratum through lateral transfer. Substrata had a significant effect on density of detached gametophytes with rougher and larger gravel showing higher densities of detachment. Our results show the potential for green gravel to be a vector of dispersal for restoration in Western Australia where natural recovery of kelp forests has failed.
Ongoing climate change is affecting marine ecosystems through warming ocean temperatures, rising sea levels, ocean acidification, increasing ocean stratification and decreasing sea ice cover (IPCC, 2022). These stressors are altering the structure and function of ecosystems, and are reducing global and local biodiversity (Vörösmarty et al., 2010; Halpern et al., 2019). Hence, interventions aimed to halt biodiversity loss and restore degraded ecosystems are essential (Duarte et al., 2020).
It is difficult for some degraded ecosystems to naturally return to historic states within a reasonable time scale (Lotze et al., 2011). Even after the causes of degradation are removed, recovery can still take decades or even centuries (Dobson et al., 1997; Lotze et al., 2011) and sometimes does not occur at all (Cox et al., 2017). Therefore, conservation that only focuses on removing, lessening, or ameliorating environmental stressors may not be effective in stopping or reversing trajectories of change (McCrackin et al., 2017; Lindegren et al., 2018). Combining traditional conservation with active restoration (i.e. anthropogenic interventions to accelerate the recovery of degraded ecosystems) is necessary to address the rapid changes in marine ecosystems (Basconi et al., 2020). Recognising this, the United Nations has declared 2021-2030 as the ‘UN Decade on Ecosystem Restoration’ and the ‘UN Decade of Ocean Science for Sustainable Development’ (Waltham et al., 2020). With both these UN calls to action, marine restoration ecology may thus become the leading field in ecological science over the next decade (Basconi et al., 2020).
Kelp forests are created by large habitat-forming brown macroalgae predominantly from the order Laminariales and extend over approximately 25% of the world’s temperate and polar coastline (Wernberg et al., 2019b). Kelps are foundation species that support local biodiversity (Norderhaug et al., 2005; Teagle et al., 2017) and provide critical ecosystem services to human societies (Smale et al., 2013; Bennett et al., 2016; Blamey and Bolton, 2018) that are worth billions of USD year-1 (Eger et al., 2021). Long-term monitoring programs (> 20 years) show that 40% to 60% of the global kelp forests are declining (Krumhansl et al., 2016; Wernberg et al., 2019b). Many of these declines are due to anthropogenic climate change and ocean warming, either directly or indirectly (Smale, 2020, Wernberg et al., 2019b). Warming can shift kelp forests from diverse, three-dimensional habitats to structurally simple turf-dominated reefs (Filbee-Dexter and Wernberg, 2018; Pessarrodona et al., 2021). Many turf reefs have not recovered (Coleman et al., 2020; Filbee-Dexter et al., 2020; Fredriksen et al., 2020) demonstrating discontinuous phase shifts with hysteresis (Scheffer et al., 2001), and future projections suggest further losses (Assis et al., 2018; Martínez et al., 2018; Davis et al., 2022). Hence, active strategies such as restoration and rehabilitation are needed to regrow kelp forests in these areas (Coleman et al., 2020; Eger et al., 2022b).
Restoration of kelp forests provide an unparalleled means to enhance biodiversity and support healthy coastal environments (Feehan et al., 2021; Filbee-Dexter et al., 2022). Kelp forest restoration efforts have shown variable success rates, with projects often either focusing on transplanting healthy individuals to a degraded area, adding new reefs or structures, and/or the removal of the cause of decline (e.g. sea urchins; Eger et al., 2020; Layton et al., 2020). While some of these efforts resulted in short-term improvements, long-term success has been limited (Layton et al., 2020). Furthermore, many kelp restoration efforts have been small scale relative to the scales of loss (less than 100 m2, Eger et al., 2022b). Moreover, for restoration to be successful, it is imperative to remove pressures on kelp forests from climate change, herbivory, sedimentation and pollution (Duarte et al., 2020).
Some kelp restoration efforts have focused on harvesting donor plants for transplanting into degraded reefs. These plants must survive environmental stressors, such as wave and storm exposure, until reproduction occurs (Campbell et al., 2014; Vergés et al., 2020). Other techniques involve seeding reefs with early life-stages of kelps using spore bags or adding kelp propagules directly. However, this comes with its own set of challenges. Although kelp propagules (i.e. zoospores) can easily be released in the laboratory (e.g. Alsuwaiyan et al., 2019), they are challenging to grow and upkeep due to their high sensitivity to laboratory conditions. Kelp zoospores are also difficult to plant or scatter onto deteriorated reefs (Vanderklift et al., 2020) especially in areas where turf algae have established (e.g. Moy and Christie, 2012; Filbee-Dexter et al., 2016; Feehan et al., 2019). “Green gravel” (Fredriksen et al., 2020) is a new restoration technique where kelp propagules are seeded onto small gravel in the laboratory (Figure 1), then out-planted back onto degraded reefs. This approach proved to be a promising method to combat kelp forest declines (Fredriksen et al., 2020). Green gravel has the potential for up-scaling to treat large degraded areas due to its low cost and low maintenance (Fredriksen et al., 2020), however, this method may be sensitive to high wave exposure or could introduce foreign substances into the ocean. Indeed, important questions around the green gravel approach remain unanswered such as what methods are optimal for gametophyte survival and whether other kelp species can be seeded in this way.
Figure 1 Green gravel workflow (A-D) and zoospore development into juvenile sporophytes (E-J). (A) Collection of fertile thalli with inset showing sori tissue, (B) inducing zoospore release in the laboratory, (C) establishing gametophyte stock cultures from the zoospore suspension, (D) seeding green gravel by adding the fragmented gametophyte suspension to trays containing small rocks. (E) Settled zoospore (1 day), (F) male and female gametophytes (2 weeks), (G, H) microscopic sporophytes on green gravel (1, 3 months), and (I, J) macroscopic juvenile sporophytes on green gravel (6, 15 months). Photo credits: (A, C, D, E-I) N. Alsuwaiyan, (B) A Minne, (J) M. Sullivan.
Here, we tested the green gravel method on Australia’s dominant underwater forest forming species, Ecklonia radiata, which has undergone declines on Australia’s east and west coasts (Wernberg et al., 2019a). Notably, E. radiata experienced one of the most severe marine heatwaves in recorded history during the austral summer of 2011. This heatwave caused mortality and range contractions of E. radiata across several hundred kilometers of coastline (Wernberg et al., 2016), with some populations entirely collapsing (i.e. the low latitude range edge populations; Wernberg et al., 2018). Species distribution models under future climate change scenarios further predict that E. radiata could lose 49% to 71% of its current distribution range under RCP 2.6 and 6.0 emissions scenarios (Martínez et al., 2018). Along Western Australia’s coastline, E. radiata populations show patterns of genetic structuring and adaptation to local thermal environments (Vranken et al., 2021), thus making E. radiata a logical target species for assisted adaptation and restoration in areas where it has disappeared.
In this study, we focused on the first step of the green gravel approach and tested the feasibility of cultivating E. radiata gametophytes on different gravel substrata. In addition, we tested the potential for gametophytes that detach from the gravel to settle and develop ex situ on the surrounding substratum. This is important in understanding if green gravel can, in some instances, help overcome the problem of recruitment limitation in degraded reefs by providing a way to add reproductive material directly to the reef surface. This study provides new insights into optimising green gravel for restoration.
Gametophytes of Ecklonia radiata used in this experiment came from gametophyte stock cultures kept at the Indian Ocean Marine Research Centre, Crawley, Western Australia (31°58’52.0” S 115°48’57.7” E) under controlled red light and temperature conditions, as described in Alsuwaiyan et al. (2021). Stock cultures were originally established from sporophytes haphazardly collected from 11 m depth by SCUBA divers from Hamelin Bay, Western Australia (34°15’22.07” S, 115°0’33.48” E) in April 2019. Four types of gravel with different surface texture and size were selected for seeding (Figure 2): small basalt (smooth texture, surface area of ~50 mm2), large basalt (smooth, ~150 mm2), crushed laterite (rough, ~200 mm2) and limestone (rough, ~250 mm2). The gravel was washed under running DI water, then soaked in DI water for one week prior to the experiments. To seed the gravel, healthy gametophyte mass from mixed genetic lines (three parents) were separated into smaller filaments (~0.1 mm) using a handheld electric blender. The suspension was then added to sixteen 1.7 L plastic containers (20 x 20 x 7 cm) containing the different gravel type separately at two average seeding densities: high density of ~230 fragments mL-1 and low density of ~115 fragments mL-1. There were two replicate container per gravel type in each seeding density treatment. The containers were filled with 700 mL of autoclaved seawater enriched with half strength Provasoli’s Enrichment Solution (PES; Provasoli, 1968), and germanium dioxide (GeO2) was added to inhibit the growth of contaminating diatoms (Lüning and Neushul, 1978). Plastic containers were moved into four incubators (set at 19°C, 50 μmol photons m-2 s-1 using Sylvania Luxline Plus FHO 24W/835 fluorescent lamps, 12 hr light:12 hr dark photoperiod) and left undisturbed for two weeks, to allow the gametophyte filaments to adhere onto the gravel. PES medium was changed after two weeks and weekly from then on. Seawater pH in each container was measured 30 days post seeding using Hanna IC-HI2002-02 Edge pH/ORP meter. Limestone was excluded from all experiments because it affected seawater pH causing all gametophytes to bleach and die.
Figure 2 Microscopic view of the different surface texture of the tested gravel: (A) small basalt, (B) large basalt, (C) crushed laterite, and (D) limestone.
After three months of rearing in the incubators, a subset of green gravel (n = 216) was transferred into twelve 24 L flow-through aquarium tanks (39 x 29 x 26 cm) set up at the Indian Ocean Marine Research Centre, Watermans Bay, Western Australia (31°51’07.2” S 115°45’05.9” E), as out-planting into the field was not possible due to COVID-related setbacks and permit restrictions. Gravel seeding density was kept separate with six tanks for the high and six tanks for the low seeding density. Each tank contained 18 pieces of gravel (n = 6 per gravel type) and was kept under the following hydrodynamic conditions: flow rate of ~100 L hr-1 using 0.8 μm sand-filtered and UV-treated seawater at 19 ± 1°C and a photoperiod of 12 hr light:12 hr dark. Small wave makers (1000 L hr-1) were added to the tanks to provide water movement. Seawater temperature was controlled using Hailea HC-2200BH Chiller and Heater. Light was provided by LED lamps (Ledzeal Zeus Aquarium Lighting System, France) and levels were set at 130 µmol m-2 s-1 photons, which prompted growth of red algae and was eventually dropped to ~40 - 50 µmol m-2 s-1 after two months. Following this contamination, approximately 50% of the gravel could no longer be assessed and thus were removed. The remaining gravel were pooled together and moved into one 200 L tank (90 x 50 x 40 cm) with the following hydrodynamic conditions: flow rate of ~200 L hr-1 using 0.8 μm sand-filtered and UV-treated seawater at 19 ± 1°C, 40 - 50 µmol m-2 s-1 and a photoperiod of 12 hr light:12 hr dark. Temperature and light were controlled using the same chiller and lamps as above. Two wave makers (Jebao Propeller Pump PP-15 15000 L hr-1) were added to the tank to provide water movement. Every four to six weeks, germanium dioxide (GeO2) was added to the tanks to reduce diatom contamination at a concentration of 2 mL L-1 of seawater. The gravel were monitored over 12 months and the maximum length of the largest sporophyte on each gravel was photographed and measured using ImageJ software. Measurements were recorded at the time of transferring into aquarium tanks from a subset of the transferred gravel (total n = 36, n = 3 per replicate container of gravel x seeding density treatment), then after two, six and 12 months from all surviving gravel (total n = 108, n = 36 per gravel type).
Two separate laboratory trials were run to assess the ability of gametophytes that detach from the gravel to settle onto surrounding substrata, each with a distinct post gravel seeding time: 30 days and 100 days post-seeding. In both trials, two pieces of gravel were haphazardly selected from every replicate container (n = 4 per combination of gravel x seeding density treatment) and moved into 24 Petri dishes (60 × 15 mm) filled with 30 mL half strength PES, with a single piece of gravel in each dish. Petri dishes were cultured in incubators set at 19°C, 50 μmol photons m-2 s-1 using Sylvania Luxline Plus FHO 24W/835 fluorescent lamps and a photoperiod of 12 hr light:12 hr dark. Petri dishes were monitored under a microscope on Days eight, 16, 23, 38 and 56 of the experiment, and the total surface area (21.5 cm2) of every dish was scanned. At each time point, gametophyte detachment and reproductive success of detached gametophytes were estimated. Gametophyte detachment from gravel (i.e. gametophyte density in the surrounding dish) was evaluated through the total number of gametophytes adhered to the dish surface around the gravel. Reproductive success (i.e. sporophyte density) was evaluated through the total number of sporophytes adhered to the dish surface.
All analysis were performed in R statistical environment version 4.0.3 (R Core Team, 2020). Assumptions of normality and homogeneity of variance were assessed using Shapiro-Wilks normality and Levene’s tests respectively and data were log transformed when necessary (Underwood, 1997). Sporophyte growth (i.e. maximum length on gravel) in aquarium tanks was analysed at two time points separately (at the time of transferring into tanks and after 12 months). For the first time point, a nested ANOVA (analysis of variance) with substrata (fixed factor with 3 levels: small basalt, large basalt and crushed laterite), seeding density (fixed factor with 2 levels: high and low) and replicate container (nested, random factor) was applied. For the second time point (12 months after transferring), a one-way ANOVA with substrata (fixed factor with 3 levels) was applied. Gametophyte detachment density and reproductive success were analysed at the end of the experiment (Day 56) using a three-way ANOVA with substrata (fixed factor with 3 levels), seeding density (fixed factor with 2 levels) and post-seeding time (fixed factor with 2 levels: 30 days and 100 days post-seeding). When appropriate, ANOVA main tests were followed by pairwise comparisons.
Gametophyte fragments from stock cultures were able to adhere to all four tested substrata successfully, however, gametophytes that adhered to the limestone gravel (the natural reef type off Western Australia) suffered extreme tissue bleaching and died. Gametophytes that adhered to the small and large basalt and laterite gravel were healthy, fertilised following seeding and microscopic sporophytes were visible within 14 days. Seawater pH in containers with limestone gravel ranged between 3.88 and 4.07, whereas in containers with the other tested substrata, pH ranged between 7.18 and 7.70.
Sporophyte length at the time of transferring from incubators to aquarium tanks was similar among substrata (nested ANOVA; substrate, F2,6 = 0.84, p = 0.476; Figure 3A and 4) and between seeding densities (nested ANOVA; seeding density, F1,6 = 0.02, p = 0.890; Figure 3A). No significant differences were observed within the interaction term (nested ANOVA; substrate x seeding density, F2,6 = 1.24, p = 0.353; Figure 3A). Maximum lengths were on average (± SE) 4.1 ± 0.5 mm on the small basalt, 3.7 ± 0.3 mm on the large basalt and 3.3 ± 0.2 mm on the crushed literate. Sporophyte continued to grow on green gravel (Figures 3B and 4), and after 12 months of rearing in aquarium tank, substrata showed a significant effect on sporophyte length (one-way ANOVA; substrata, F2,105 = 10.60, p < 0.001; Figure 4). Pairwise comparisons showed that sporophytes growing on both basalt gravel were larger than those on the crushed laterite (small basalt: 95% CI = 0.032 to 0.259, p = 0.008; large basalt: 95% CI = 0.101 to 0.327, p < 0.001). Between the two basalt gravel, lengths were similar (p = 0.324). Further, sporophyte density on green gravel changed over time from many microscopic sporophytes covering the gravel at the time of transferring into aquarium tanks to only 1 - 4 juvenile sporophytes on each gravel after 12 months.
Figure 3 Sporophyte length (mean ± SE) on green gravel for each substratum and seeding density (A) at the time of transferring into aquarium tanks (three months post-seeding), and (B) two months after transferring. Colours refer to the initial seeding density.
Figure 4 Sporophyte length (mean ± SE) on green gravel over time after transferring into aquarium tanks. Colours refer to the type of substrata.
Gametophyte fragments that detached from the gravel were able to adhere to surrounding petri dish surface successfully in all trials. The density of detached gametophytes varied significantly within the interaction of substrata and post-seeding time (ANOVA; substrate x post-seeding time, F2, 36 = 8.74, p < 0.001; Table 1 and Figures 5A, B), but not seeding density (ANOVA; substrate x seeding density x post-seeding time, F2, 36 = 0.46, p = 0.636; Table 1). Pairwise comparisons showed that gametophyte density 30 days post-seeding was higher around the crushed laterite than the small basalt gravel (95% CI = 0.419 to 1.087, p < 0.001). Between the two basalt gravel types, gametophyte density was higher around the large basalt (95% CI = 0.177 to 0.845, p = 0.001). In contrast, density of detached gametophytes 100 days post-seeding did not significantly differ between the crushed laterite and both small and large basalt (p = 1.000; and p = 0.337 respectively) nor between the two basalt gravel (p = 0.738). Further comparisons showed that for all three tested substrata, the density of detached gametophytes was significantly greater 30 days post-seeding than 100 days post-seeding (small basalt: 95% CI = 0.002 to 0.542, p = 0.048; large basalt: 95% CI = 670 to 1.210, p < 0.001; crushed laterite: 95% CI = 0.695 to 1.235, p < 0.001).
Table 1 ANOVA main test results testing for the effect of substrata (fixed factor), seeding density (fixed factor), post-seeding time (fixed factor) and their interactions on Ecklonia radiata gametophyte detachment from green gravel on Day 56.
Figure 5 Effects of substrata and post-seeding time on Ecklonia radiata (A, B) gametophyte detachment and (C, D) reproductive success of detached gametophytes over time. Gametophyte detachment from gravel (i.e. gametophyte density) is expressed as the number of gametophytes cm-2 adhered to the dish surface around the gravel. Reproductive success (i.e. sporophyte density) is expressed as the number of sporophytes cm-2 adhered to the dish surface around the gravel. Data represent mean ± SE. Colours refer to the type of substrata. Please note different scale on Y-axis.
Reproductive success of detached gametophytes varied significantly among substrata (ANOVA; substrate, F2,36 = 77.51, p < 0.001; Table 2 and Figures 5C, D), but not between seeding density (ANOVA; seeding density, F1,36 = 1.48, p = 0.231; Table 2), post-seeding time (ANOVA; post-seeding time, F1,36 = 3.96, p = 0.054; Table 2) or their interaction (ANOVA; substrate x seeding density x post-seeding time, F2,36 = 1.81, p = 0.178; Table 2). Pairwise comparisons among substrata showed that sporophyte density was greater around the crushed laterite than both small and large basalt gravel, suggesting a significant effect of substratum roughness (95% CI = 0.831 to 1.257, p < 0.001; 95% CI = 0.173 to 0.599, p < 0.001 respectively). Between the two basalt gravel types, sporophyte density was greater around the large basalt than the small basalt gravel, suggesting a significant effect of substratum size (95% CI = 0.445 to 0.871, p < 0.001).
Table 2 ANOVA main test results testing for the effect of substrata (fixed factor), seeding density (fixed factor), post-seeding time (fixed factor) and their interactions on reproductive success of detached Ecklonia radiata gametophytes from green gravel on Day 56.
A key challenge in the current Decade of Ecosystem Restoration is scaling up restoration tools to meet the rapid rates of loss (Saunders et al., 2020; Filbee-Dexter et al., 2022). Considering that kelp forests have fast growth rates and have declined over large areas of their global range, there is great opportunity to increase efforts to protect and restore kelp forests and secure the many fundamental ecosystem services that they provide to human society (Feehan et al., 2021). Green gravel is a simple, cost-effective and scalable restoration technique that involves two main processes: culturing kelp propagules in the laboratory and out-planting them back into the field (Fredriksen et al., 2020). Here, we tested the feasibility of cultivating Ecklonia radiata gametophytes on gravel and the ability of gametophytes that detach from gravel to develop ex situ. Our results showed that E. radiata gametophytes from stock cultures were able to adhere and develop successfully on gravel, demonstrating green gravel to be a promising option to restore E. radiata populations. Moreover, we also showed that gametophytes detached from gravel can develop on the surrounding substratum, making green gravel a versatile tool to seed degraded reefs either directly through juvenile sporophytes attaching onto the gravel or indirectly by providing a way to disseminate reproductive material onto the underlying reef to enrich restored or degraded sites.
In this study, we focused on optimising the first step of the green gravel process: culturing E. radiata gametophytes on small gravel. This is challenging because early life-stages of kelp are extremely sensitive to contamination (Redmond et al., 2014) and most culture studies on E. radiata do not continue beyond the microscopic sporophyte stage. Substrata type (i.e. gravel) had some effects on the survival of E. radiata gametophytes. The extreme tissue bleaching in gametophytes that adhered to the limestone gravel highlights the importance of testing the choice of gravel prior to starting experiments. Some gravel can potentially modify seawater chemistry (Brockmann and Janse, 2008) and adversely affect the survival of the very small early stages of kelp, thus resulting in ineffective interventions and wasting valuable resources. Gametophytes that adhered to the three other tested gravel types, however, developed into healthy juvenile sporophytes, and surprisingly, seeding density had no effect on sporophyte length. This result was unexpected because intraspecific competition for nutrient and space increases with density, and therefore growth in high density cultures is reduced due to competition, compared to low density cultures (Reed et al., 1991; Steen, 2003). However, it is possible that this competition could have induced density-dependent mortality (Reed, 1990), thus partially explaining the low number of sporophytes that were eventually growing on the gravel after 12 months. Further, both seeding densities used in this study are significantly lower than densities previously used for kelp aquaculture (e.g. Flavin et al., 2013; Redmond et al., 2014), yet seeding with considerably different densities yielded similar results with no differences in growth in Saccharina latissima (Fredriksen et al., 2020). This suggests that seeding density may not limit the successful development of sporophytes on the gravel. This is particularly important when green gravel is being seeded with gametophytes from stock cultures, where material could be limited.
Besides the successful adhesion and development of E. radiata gametophytes on the gravel itself, gametophytes that detached from the gravel were able to settle onto the surrounding substratum, then fertilise and develop into juvenile sporophytes. Given that eventually only few sporophytes will grow into large kelp on each gravel regardless of initial seeding density, as seen in our results and Fredriksen et al. (2020), the ability of microscopic stages to settle onto surrounding substrata after detaching from the gravel can help to increase the number of juveniles that may directly attach to the reef and reach the adult stage at the restoration site. Substrata type had a significant effect on the density of detached gametophytes, with larger and rougher textured gravel showing higher densities of detachment. This result was expected given that propagule settlement was found to be higher on rougher substratum than on smooth substratum (Falace et al., 2018), and thus more gametophytes will detach as they grow larger and competition for space increases. Notably, timing in which green gravel was moved did affect the density of detached gametophytes, with higher densities observed 30 days post-seeding compared to 100 days. This could be because most gametophytes had undergone gametogenesis during the longer incubation time in the laboratory, which reflected on the low density of detached gametophytes 100 days post-seeding. These results also suggest that with time, gametophytes may become solidly attached to the gravel and might not be capable of detaching, which highlights the importance of timing when out-planting the gravel into the field. It is suggested that the out-planting process should be carried out within 1-2 months of cultivating in the laboratory, as kelp cultured for long periods in laboratory settings may perform poorly in the field (Eger et al., 2022a). However, this might not be possible with realistic time frames of cultivation. Whilst the rates of gametophyte detachment in our experiments became significantly low 100 days post-seeding, questioning the effectiveness of green gravel as a vector of dispersal, reproductive success (i.e. sporophyte density on the surrounding substratum) was similar between the two post-seeding times. This suggests that sporophytes that developed on the gravel may also detach and adhere successfully onto surrounding substrata even after a few months of seeding on the gravel. This was also supported by observations in our aquarium experiments, where sporophytes were observed growing on other structures in the tank many months after transferring from the incubators (e.g. surrounding trays, wave makers). This was only observed in aquarium tanks with green gravel, which shows that it was not an artefact from inflowing seawater. The presence of sporophytes away from the gravel could also be due to the mobility of kelp gametes (Lüning, 1990). Whilst kelp eggs are immobile, they have the potential to break away from the fertile gametophyte after gametogenesis. This may result in an instance where a detached egg is fertilised by a mobile sperm and thus develop into a sporophyte on surrounding substrata. Indeed, kelp propagules will need suitable substrate to settle and develop on after detaching from the gravel, which is often not available in degraded areas (e.g. turf algae). In some instances, substrates can be added to overcome this limitation. In addition, the abundance of turf algae regularly displays seasonal patterns (Diaz-Pulido and Garzón-Ferreira, 2002), thus carrying out the out-planting process during periods when abundance is low may help increase the successful adhesion of kelp propagules onto surrounding substrata (Eger et al., 2022a). Factors that could challenge the successful settlement of kelp early-life stages in the field require more investigation including sedimentation, grazing activity and wave action.
In this study, full scale field trials of green gravel were not possible due to delays in receiving restoration permits, highlighting that the permitting and regulatory process could be a significant barrier to developing these techniques efficiently (Shumway et al., 2021). Although regulations, policies and legislation guide restoration programs, such governance can be a major hurdle to the success and sustainability of restoration programs (Sapkota et al., 2018). Indeed, current restoration governance needs improvement and strengthening in order to successfully implement restoration programs (Richardson and Lefroy, 2016).
Contamination is a major challenge in cultivating kelp and other seaweeds in an aquarium setting (Redmond et al., 2014). Microscopic epiphytic algae were found to likely be the primary cause of kelp degeneration and mortality in aquarium tanks (Tew et al., 2011). Aquarium settings reported in this study were developed after many challenges we faced in early trials, including diatom and red algal contaminations. Indeed, seawater carries many microorganisms that can graze on and compete with kelp in aquariums (Redmond et al., 2014) and using sand-filtered and UV-treated seawater was sufficient to remove biological contaminants. Reducing light levels and adding germanium dioxide (GeO2) also helped in controlling contamination levels. Yet, poor quality seawater and contamination could be a limitation for green gravel success in some aquaria and for some kelp species. In this study we established some fundamental knowledge for optimum cultivation of E. radiata not only for green gravel application context, but also for the seaweed farming industry (Buschmann et al., 2017; Filbee-Dexter et al., 2022). It should also be noted that while we were successful in culturing E. radiata in aquarium tanks for over 12 months, growth rates of these kelp are low, and their size was small compared to wild juvenile kelp (Wernberg et al., 2019a). This underdevelopment is likely to be a consequence of being cultured in aquaria, thus further efforts to optimize such settings are required.
Collectively, our results show the potential in applying green gravel to restore lost or declining E. radiata populations. Indeed, determining the ability of E. radiata gametophytes to adhere and develop successfully on green gravel, as well as on surrounding substrata through lateral movement, is a critical first step in optimising this tool for restoration programs in Australia. Further research is required to improve and finesse green gravel for restoration and conservation, including field tests of this tool. Studies that take into consideration potential stressors and competitors that can inhibit kelp recruitment (e.g. sediments and turf algae; Watanabe et al., 2016; Layton et al., 2019) are necessary to optimise the out-planting process and increase restoration success.
The raw data supporting the conclusions of this article will be made available by the authors, without undue reservation.
NAA, KF-D, MAC and TW conceived and designed the study. SV carried out culture preparation. NAA carried out the experiment. CB carried out aquarium monitoring. NAA analysed the data, produced all tables and figures, and took the lead in writing the manuscript. KF-D helped with data analysis. All authors contributed to the article and approved the submitted version
This work was funded through the Australian Research Council grants DP160100114 (TW, MAC), DP190100058 (TW, MAC, KF-D), LP1931001500 (TW, MAC, KF-D) and DE1901006192 (KFD). NAA was supported by a postgraduate scholarship from Qassim University, Kingdom of Saudi Arabia. CB was supported by a Forrest Research Foundation scholarship and Australian Government Research Training Program scholarship.
The authors declare that the research was conducted in the absence of any commercial or financial relationships that could be construed as a potential conflict of interest.
All claims expressed in this article are solely those of the authors and do not necessarily represent those of their affiliated organizations, or those of the publisher, the editors and the reviewers. Any product that may be evaluated in this article, or claim that may be made by its manufacturer, is not guaranteed or endorsed by the publisher.
Alsuwaiyan N. A., Mohring M. B., Cambridge M., Coleman M. A., Kendrick G. A., Wernberg T. (2019). A review of protocols for the experimental release of kelp (Laminariales) zoospores. Ecol. Evol. 9 (14), 8387–8398. doi: 10.1002/ece3.5389
Alsuwaiyan N. A., Vranken S., Filbee-Dexter K., Cambridge M., Coleman M. A., Wernberg T. (2021). Genotypic variation in response to extreme events may facilitate kelp adaptation under future climates. Mar. Ecol. Prog. Ser. 672, 111–121. doi: 10.3354/meps13802
Assis J., Araújo M. B., Serrão E. A. (2018). Projected climate changes threaten ancient refugia of kelp forests in the north Atlantic. Glob. Change Biol. 24, e55–e66. doi: 10.1111/gcb.13818
Basconi L., Cadier C., Guerrero-Limón G. (2020). “Challenges in marine restoration ecology: How techniques, assessment metrics, and ecosystem valuation can lead to improved restoration success,” in YOUMARES 9 - the oceans: Our research, our future. Eds. Jungblut S., Liebich V., Bode-Dalby M. (Cham: Springer) 83–99.
Bennett S., Wernberg T., Connell S. D., Hobday A. J., Johnson C. R., Poloczanska E. S. (2016). The ‘Great southern reef’: Social, ecological and economic value of australia’s neglected kelp forests. Mar. Freshw. Res. 67, 47–56. doi: 10.1071/MF15232
Blamey L. K., Bolton J. J. (2018). The economic value of south African kelp forests and temperate reefs: Past, present and future. J. Mar. Syst. 188, 172–181. doi: 10.1016/j.jmarsys.2017.06.003
Brockmann D., Janse M. (2008). “Calcium and carbonate in closed marine aquarium systems,” in Advances in coral husbandry in public aquariums. Eds. Leewis R. J., Janse M. (Arnhem, The Netherlands: Burgers’Zoo) 133–142.
Buschmann A. H., Camus C., Infante J., Neori A., Israel Á., Hernández-González M. C., et al. (2017). Seaweed production: Overview of the global state of exploitation, farming and emerging research activity. Eur. J. Phycol. 52 (4), 391–406. doi: 10.1080/09670262.2017.1365175
Campbell A. H., Marzinelli E. M., Vergés A., Coleman M. A., Steinberg P. D. (2014). Towards restoration of missing underwater forests. PloS One 9, e84106. doi: 10.1371/journal.pone.0084106
Coleman M. A., Wood G., Filbee-Dexter K., Minne A. J. P., Goold H. D., Vergés A., et al. (2020). Restore or redefine: Future trajectories for restoration. Front. Mar. Sci. 7 (237). doi: 10.3389/fmars.2020.00237
Cox C., Valdivia A., McField M., Castillo K., Bruno J. F. (2017). Establishment of marine protected areas alone does not restore coral reef communities in Belize. Mar. Ecol. Prog. Ser. 563, 65–79. doi: 10.3354/meps11984
Davis T. R., Champion C., Coleman M. A. (2022). Ecological interactions mediate projected loss of kelp biomass under climate change. Divers. Distrib. 28 (2), 306–317. doi: 10.1111/ddi.13462
Diaz-Pulido G., Garzón-Ferreira J. (2002). Seasonality in algal assemblages on upwelling-influenced coral reefs in the Colombian Caribbean. Bot. Mar. 45 (3), 284–292. doi: 10.1515/BOT.2002.028
Dobson A. P., Bradshaw A. D., Baker A. J. M. (1997). Hopes for the future: Restoration ecology and conservation biology. Science 277 (5325), 515–522. doi: 10.1126/science.277.5325.515
Duarte C. M., Agusti S., Barbier E., Britten G. L., Castilla J. C., Gattuso J.-P., et al. (2020). Rebuilding marine life. Nature 580 (7801), 39–51. doi: 10.1038/s41586-020-2146-7
Eger A. M., Layton C., McHugh T. A., Gleason M., Eddy N. (2022a). “Lessons learned from kelp restoration projects around the world,” in Kelp restoration guidebook (Arlington, VA, USA: The Nature Conservancy).
Eger A. M., Marzinelli E. M., Baes R., Blain C., Blamey L., Carnell P. E., et al. (2021). The economic value of fisheries, blue carbon, and nutrient cycling in global marine forests. EcoEvoRxiv [Preprint]. doi: 10.32942/osf.io/n7kjs
Eger A. M., Marzinelli E. M., Christie H., Fagerli C. W., Fujita D., Gonzalez A. P., et al. (2022b). Global kelp forest restoration: Past lessons, present status, and future directions. Biol. Rev 97, 1449–1475. doi: 10.1111/brv.12850
Eger A. M., Vergés A., Choi C. G., Christie H., Coleman M. A., Fagerli C. W., et al. (2020). Financial and institutional support are important for large-scale kelp forest restoration. Front. Mar. Sci. 7, 535277. doi: 10.3389/fmars.2020.535277
Falace A., Kaleb S., de la Fuente G., Asnaghi V., Chiantore M. (2018). Ex situ cultivation protocol for Cystoseira amentacea var. stricta (Fucales, phaeophyceae) from a restoration perspective. PloS One 13 (2), e0193011. doi: 10.1371/journal.pone.0193011
Feehan C. J., Filbee-Dexter K., Wernberg T. (2021). Embrace kelp forests in the coming decade. Science 373 (6557), 863–863. doi: 10.1126/science.abl3984
Feehan C. J., Grace S. P., Narvaez C. A. (2019). Ecological feedbacks stabilize a turf-dominated ecosystem at the southern extent of kelp forests in the Northwest Atlantic. Sci. Rep. 9, 7078. doi: 10.1038/s41598-019-43536-5
Filbee-Dexter K., Feehan C. J., Scheibling R. E. (2016). Large-Scale degradation of a kelp ecosystem in an ocean warming hotspot. Mar. Ecol. Prog. Ser. 543, 141–152. doi: 10.3354/meps11554
Filbee-Dexter K., Wernberg T. (2018). Rise of turfs: A new battlefront for globally declining kelp forests. Bioscience 68 (2), 64–76. doi: 10.1093/biosci/bix147
Filbee-Dexter K., Wernberg T., Barreiro R., Coleman M. A., de Bettignies T., Feehan C. J., et al. (2022). Leveraging the blue economy to transform marine forest restoration. J. Phycol. 58, 198–207. doi: 10.1111/jpy.13239
Filbee-Dexter K., Wernberg T., Grace S. P., Thormar J., Fredriksen S., Narvaez C. N., et al. (2020). Marine heatwaves and the collapse of marginal north Atlantic kelp forests. Sci. Rep. 10, 13388. doi: 10.1038/s41598-020-70273-x
Flavin K., Flavin N., Flahive B. (2013) Kelp farming manual: A guide to the processes, techniques, and equipment for farming kelp in new England waters. saco, ME: Ocean approved LLC. Available at: https://maineaqua.org/wp-content/uploads/2020/06/OceanApproved_KelpManualLowRez.pdf.
Fredriksen S., Filbee-Dexter K., Norderhaug K. M., Steen H., Bodvin T., Coleman M. A., et al. (2020). Green gravel: A novel restoration tool to combat kelp forest decline. Sci. Rep. 10, 3983. doi: 10.1038/s41598-020-60553-x
Halpern B. S., Frazier M., Afflerbach J., Lowndes J. S., Micheli F., O’Hara C., et al. (2019). Recent pace of change in human impact on the world’s ocean. Sci. Rep. 9, 11609. doi: 10.1038/s41598-019-47201-9
IPCC (2022). Climate change 2022: Impacts, adaptation, and vulnerability. Contribution of Working Group II to the Sixth Assessment Report of the Intergovernmental Panel on Climate Change [Pörtner H.-O., Roberts D. C., Tignor M., Poloczanska E. S., Mintenbeck K., Alegría A., Craig M., Langsdorf S., Löschke S., Möller V., Okem A., Rama. B. (eds.)] (Cambridge, UK and New York, USA: Cambridge University Press), 3056 pp., doi: 10.1017/9781009325844
Krumhansl K. A., Okamoto D. K., Rassweiler A., Novak M., Bolton J. J., Cavanaugh K. C., et al. (2016). Global patterns of kelp forest change over the past half-century. Proc. Natl. Acad. Sci. U. S. A 113 (48), 13785–13790. doi: 10.1073/pnas.1606102113
Layton C., Cameron M. J., Shelamoff V., Fernández P. A., Britton D., Hurd C. L., et al. (2019). Chemical microenvironments within macroalgal assemblages: Implications for the inhibition of kelp recruitment by turf algae. Limnol. Oceanogr. 64 (4), 1600–1613. doi: 10.1002/lno.11138
Layton C., Coleman M. A., Marzinelli E. M., Steinberg P. D., Swearer S. E., Vergés A., et al. (2020). Kelp forest restoration in Australia. Front. Mar. Sci. 7, 74. doi: 10.3389/fmars.2020.00074
Lindegren M., Holt B. G., MacKenzie B. R., Rahbek C. (2018). A global mismatch in the protection of multiple marine biodiversity components and ecosystem services. Sci. Rep. 8, 4099. doi: 10.1038/s41598-018-22419-1
Lotze H. K., Coll M., Magera A. M., Ward-Paige C., Airoldi L. (2011). Recovery of marine animal populations and ecosystems. Trends Ecol. Evol. 26 (11), 595–605. doi: 10.1016/j.tree.2011.07.008
Lüning K. (1990). “Their environment, biogeography, and ecophysiology,” in Seaweeds (New York, N.Y: Wiley).
Lüning K., Neushul M. (1978). Light and temperature demands for growth and reproduction of laminarian gametophytes in southern and central California. Mar. Biol. 45 (4), 297–309. doi: 10.1007/bf00391816
Martínez B., Radford B., Thomsen M. S., Connell S. D., Carreño F., Bradshaw C. J. A., et al. (2018). Distribution models predict large contractions of habitat-forming seaweeds in response to ocean warming. Divers. Distrib. 24 (10), 1350–1366. doi: 10.1111/ddi.12767
McCrackin M. L., Jones H. P., Jones P. C., Moreno-Mateos D. (2017). Recovery of lakes and coastal marine ecosystems from eutrophication: A global meta-analysis. Limnol. Oceanogr. 62 (2), 507–518. doi: 10.1002/lno.10441
Moy F. E., Christie H. (2012). Large-Scale shift from sugar kelp (Saccharina latissima) to ephemeral algae along the south and west coast of Norway. Mar. Biol. Res. 8 (4), 309–321. doi: 10.1080/17451000.2011.637561
Norderhaug K. M., Christie H., Fosså J. H., Fredriksen S. (2005). Fish–macrofauna interactions in a kelp (Laminaria hyperborea) forest. J. Mar. Biol. Assoc. U.K 85 (5), 1279–1286. doi: 10.1017/S0025315405012439
Pessarrodona A., Filbee-Dexter K., Alcoverro T., Boada J., Feehan C. J., Fredriksen S., et al. (2021). Homogenization and miniaturization of habitat structure in temperate marine forests. Glob. Change Biol. 27 (20), 5262–5275. doi: 10.1111/gcb.15759
Provasoli L. (1968). “Media and prospects for the cultivation of marine algae,” in Cultures and collections of algae. Eds. Watanabe A., Hattori A. (Hakone, Japan: Japanese Society Plant Physiology), 63–75.
R Core Team (2020). “R: A language and environment for statistical computing,” in R foundation for statistical computing(Vienna, Austria). Available at: https://www.R-project.org/
Redmond S., Green L., Yarish C., Kim J., Neefus C. (2014). New England seaweed culture handbook - nursery systems (Groton, Connecticut, USA: Connecticut Sea Grant CTSG-14-01, University of Connecticut).
Reed D. C. (1990). The effects of variable settlement and early competition on patterns of kelp recruitment. Ecology 71 (2), 776–787. doi: 10.2307/1940329
Reed D. C., Neushul M., Ebeling A. W. (1991). Role of settlement density on gametophyte growth and reproduction in the kelps Pterygophora californica and Macrocystis pyrifera (Phaeophyceae). J. Phycol. 27 (3), 361–366. doi: 10.1111/j.0022-3646.1991.00361.x
Richardson B. J., Lefroy T. (2016). Restoration dialogues: Improving the governance of ecological restoration. Restor. Ecol. 24 (5), 668–673. doi: 10.1111/rec.12391
Sapkota R. P., Stahl P. D., Rijal K. (2018). Restoration governance: An integrated approach towards sustainably restoring degraded ecosystems. Environ. Dev. 27, 83–94. doi: 10.1016/j.envdev.2018.07.001
Saunders M. I., Doropoulos C., Bayraktarov E., Babcock R. C., Gorman D., Eger A. M., et al. (2020). Bright spots in coastal marine ecosystem restoration. Curr. Biol. 30 (24), R1500–R1510. doi: 10.1016/j.cub.2020.10.056
Scheffer M., Carpenter S., Foley J. A., Folke C., Walker B. (2001). Catastrophic shifts in ecosystems. Nature 413 (6856), 591–596. doi: 10.1038/35098000
Shumway N., Bell-James J., Fitzsimons J. A., Foster R., Gillies C., Lovelock C. E. (2021). Policy solutions to facilitate restoration in coastal marine environments. Mar. Policy 134, 104789. doi: 10.1016/j.marpol.2021.104789
Smale D. A. (2020). Impacts of ocean warming on kelp forest ecosystems. New Phytol. 225, 1447–1454. doi: 10.1111/nph.16107
Smale D. A., Burrows M. T., Moore P., O’Connor N., Hawkins S. J. (2013). Threats and knowledge gaps for ecosystem services provided by kelp forests: A northeast Atlantic perspective. Ecol. Evol. 3 (11), 4016–4038. doi: 10.1002/ece3.774
Steen H. (2003). Intraspecific competition in Sargassum muticum (Phaeophyceae) germlings under various density, nutrient and temperature regimes. Bot. Mar. 46, 36–43. doi: 10.1515/BOT.2003.006
Teagle H., Hawkins S. J., Moore P. J., Smale D. A. (2017). The role of kelp species as biogenic habitat formers in coastal marine ecosystems. J. Exp. Mar. Bio. Ecol. 492, 81–98. doi: 10.1016/j.jembe.2017.01.017
Tew K. S., Meng P. J., Leu M. Y. (2011). Factors correlating with deterioration of giant kelp Macrocystis pyrifera (Laminariales, heterokontophyta) in an aquarium setting. J. Appl. Phycol. 24, 1269–1277. doi: 10.1007/s10811-011-9775-z
Underwood A. J. (1997). Experiments in ecology: Their logical design and interpretation using analysis of variance (Cambridge: Cambridge Cambridge University Press).
Vanderklift M. A., Doropoulos C., Gorman D., Leal I., Minne A. J. P., Statton J., et al. (2020). Using propagules to restore coastal marine ecosystems. Front. Mar. Sci. 7, 724. doi: 10.3389/fmars.2020.00724
Vergés A., Campbell A. H., Wood G., Kajlich L., Eger A. M., Cruz D., et al. (2020). Operation crayweed: Ecological and sociocultural aspects of restoring sydney’s underwater forests. Ecol. Manage. Restor. 21 (2), 74–85. doi: 10.1111/emr.12413
Vörösmarty C. J., McIntyre P. B., Gessner M. O., Dudgeon D., Prusevich A., Green P., et al. (2010). Global threats to human water security and river biodiversity. Nature 467, 555–561. doi: 10.1038/nature09440
Vranken S., Wernberg T., Scheben A., Severn-Ellis A., Batley J., Philipp B. E., et al. (2021). Genotype-environment mismatch of kelp forests under climate change. Mol. Ecol. 30, 3730–3746. doi: 10.1111/mec.15993
Waltham N. J., Elliott M., Lee S. Y., Lovelock C., Duarte C. M., Buelow C., et al. (2020). UN Decade on ecosystem restoration 2021–2030—What chance for success in restoring coastal ecosystems? Front. Mar. Sci. 7, 71. doi: 10.3389/fmars.2020.00071
Watanabe H., Ito M., Matsumoto A., Arakawa H. (2016). Effects of sediment influx on the settlement and survival of canopy-forming macrophytes. Sci. Rep. 6, 18677. doi: 10.1038/srep18677
Wernberg T., Bennett S., Babcock R. C., de Bettignies T., Cure K., Depczynski M., et al. (2016). Climate-driven regime shift of a temperate marine ecosystem. Science 353 (6295), 169–172. doi: 10.1126/science.aad8745
Wernberg T., Coleman M. A., Babcock R. C., Bell S. Y., Bolton J. J., Connell S. D., et al. (2019a). “Biology and ecology of the globally significant kelp ecklonia radiata,” in Oceanography and marine biology: An annual review. Eds. Hawkins S. J., Allcock A. L., Bates A. E., Firth L. B., Smith I. P., Swearer S. E., Todd P. A. (Milton, United Kingdom: Taylor & Francis Group) 265–324.
Wernberg T., Coleman M. A., Bennett S., Thomsen M. S., Tuya F., Kelaher B. P. (2018). Genetic diversity and kelp forest vulnerability to climatic stress. Sci. Rep. 8, 1851. doi: 10.1038/s41598-018-20009-9
Keywords: Ecklonia radiata, gametophyte, kelp forests, seaweed restoration, seeding, sporophyte
Citation: Alsuwaiyan NA, Filbee-Dexter K, Vranken S, Burkholz C, Cambridge M, Coleman MA and Wernberg T (2022) Green gravel as a vector of dispersal for kelp restoration. Front. Mar. Sci. 9:910417. doi: 10.3389/fmars.2022.910417
Received: 01 April 2022; Accepted: 22 August 2022;
Published: 12 September 2022.
Edited by:
Elisabeth Marijke Anne Strain, University of Tasmania, AustraliaReviewed by:
Cayne Layton, University of Tasmania, AustraliaCopyright © 2022 Alsuwaiyan, Filbee-Dexter, Vranken, Burkholz, Cambridge, Coleman and Wernberg. This is an open-access article distributed under the terms of the Creative Commons Attribution License (CC BY). The use, distribution or reproduction in other forums is permitted, provided the original author(s) and the copyright owner(s) are credited and that the original publication in this journal is cited, in accordance with accepted academic practice. No use, distribution or reproduction is permitted which does not comply with these terms.
*Correspondence: Nahlah A. Alsuwaiyan, bi5hbHN1d2FpeWFuQHF1LmVkdS5zYQ==; Thomas Wernberg, dGhvbWFzLndlcm5iZXJnQHV3YS5lZHUuYXU=
Disclaimer: All claims expressed in this article are solely those of the authors and do not necessarily represent those of their affiliated organizations, or those of the publisher, the editors and the reviewers. Any product that may be evaluated in this article or claim that may be made by its manufacturer is not guaranteed or endorsed by the publisher.
Research integrity at Frontiers
Learn more about the work of our research integrity team to safeguard the quality of each article we publish.