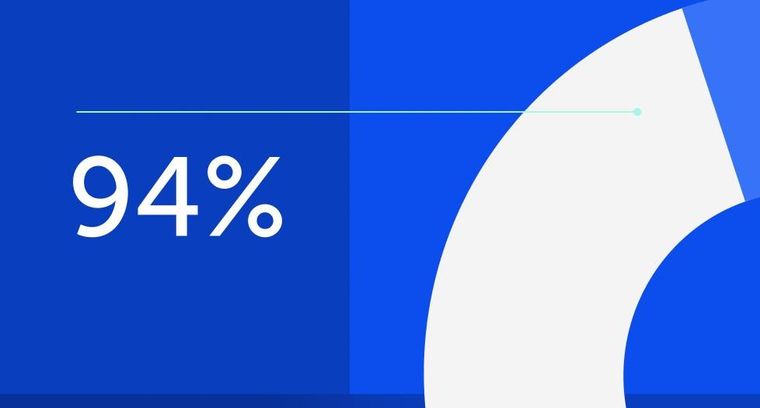
94% of researchers rate our articles as excellent or good
Learn more about the work of our research integrity team to safeguard the quality of each article we publish.
Find out more
ORIGINAL RESEARCH article
Front. Mar. Sci., 30 June 2022
Sec. Marine Ecosystem Ecology
Volume 9 - 2022 | https://doi.org/10.3389/fmars.2022.909528
This article is part of the Research TopicThe Physiology, Ecology and Biogeochemistry of Marine Zooplankton in a Changing Arctic OceanView all 15 articles
Copepods dominate zooplankton biomass of the upper ocean, especially in the highly seasonal boreal and polar regions, for which specific life-cycle traits such as the accumulation of lipid reserves, migration into deep water and diapause are key adaptations. Understanding such traits is central to determining the energetic consequences of high latitude range shifts related to climate change and ultimately, biogeochemical models of carbon flow. Using the calanoid copepod Calanus finmarchicus, we explore a new indicator of diapause, swimming activity, and assess its relationship with respiration. Stage CV copepods were sampled in late summer from shallow (epipelagic) and deep (mesopelagic) water at both slope and basin locations within the Fram Strait at a time when the animals had entered diapause. Using high-throughput quantitative behaviour screening on ex-situ swimming activity, we found that irrespective of sampling station copepods from the mesopelagic show highly reduced activity (88.5 ± 3.4% reduction) when compared to those from the epipelagic with a clearly defined threshold between epi- and mesopelagic animals (~5 beam breaks 30 min-1). Mesopelagic individuals were also larger (12.4 ± 8.8%) and had more lipid reserves (19.3 ± 2.2%) than epipelagic individuals. On average, copepods from the basin station exhibited respiration rates similar to overwintering rates observed elsewhere (1.23 ± 0.76 µg C d-1), while respiration rates of copepods from the shelf station were more consistent with active metabolism (2.46 ± 1.02 µg C d-1). Nevertheless, active and diapausing rates were observed in individuals from both stations at both epi- and mesopelagic depths. We suggest that rapid screening of activity may provide an early indicator of diapause before it becomes fully apparent and consistent in other physiological indicators. Ultimately, swimming activity may provide a useful tool to assess the putative endogenous and exogenous factors involved in diapause onset, provide a handle on the energetics of diapause, and input to biogeochemical carbon models on C. finmarchicus.
High-latitude seas are undergoing rapid climate-driven change, expected to affect the distribution and phenology of marine organisms, including the keystone zooplankton species Calanus finmarchicus (e.g., Wilson et al., 2016a, Wilson et al., 2016b). This copepod dominates zooplankton biomass in Atlantic-influenced seas (Auel et al., 2003), where it occupies a key trophic position as the main food source for commercially important fish like herring (Prokopchuk and Sentyabov, 2006) and endangered marine mammals such as North Atlantic right whales (Greene et al., 2003) and as a food source for many seabirds (Karnovsky et al., 2003; Kwaśniewski et al., 2012; Vogedes et al., 2014). By carrying out large-scale seasonal vertical migrations that often span hundreds of meters (e.g., Baumgartner and Tarrant, 2017) copepodite stages 4 and 5 (CIV-V), move carbon and lipids into deep-water layers in a process known as the lipid pump which has important implications for high-latitude carbon sequestration and pelagic-benthic coupling (Jónasdóttir et al., 2015; Visser et al., 2017).
To survive the food-depleted autumn and winter months at high latitudes, C. finmarchicus typically undergoes a period of dormancy in which CIV and CV copepodites form aggregations at depths below the permanent thermocline (Jónasdóttir et al., 2015). To meet the energetic reserves required to overwinter with subsequent molt and gonad development the following spring, large lipid reserves are accumulated (Hirche, 1996). To lower their metabolism, respiration rate is reduced to 10-20% of that recorded in shallower conspecifics (e.g., Hirche, 1983; Ingvarsdóttir, 1998; Ingvarsdóttir et al., 1999; Auel et al., 2003). Indeed, elevated metabolism impedes survival over the course of diapause, which may last 6 to 8 months (Baumgartner and Tarrant, 2017). Therefore, respiration measurements (as a proxy for metabolic rate) and collection depth, are typically used to distinguish diapausing copepods from those actively feeding and migrating.
Diapause is generally considered to be under endocrine control (e.g., Alldredge et al., 1984; Hirche, 1996; Baumgartner and Tarrant, 2017) and upstream expression of ‘diapause associated’ genes involved in antioxidative defense and lipid catabolism (Tarrant et al., 2008; Häfker et al., 2018a; Häfker et al., 2018b; Lenz et al., 2021; Payton et al., 2022). In addition, body size, lipid sac volume, and ambient temperature are all likely to affect metabolism at this time fueled by protein and lipid stores. These lipids are typically wax esters which undergo phase transitions at low temperatures and high pressure (e.g., Kirkesæter, 1977; Rey-Rassat et al., 2002; Tarling et al., 2022) and have been postulated as providing a means of buoyancy control (Pond and Tarling, 2011). For the copepodid stages CIV and CV, reaching optimal lipid sac fullness in spring has been suggested as a potential trigger for the onset of diapause (e.g., Pond and Tarling, 2011; Maps, 2014; Schmid et al., 2018). However, stored lipid energy reserves are only partially utilized at depth with most being used for re-ascent post diapause, molting, mate finding, and gamete production (Rey-Rassat et al., 2002). Hatching of offspring is then timed to coincide with favorable conditions associated with the spring phytoplankton bloom (Leu et al., 2011).
In addition to reduced respiration in diapause there are also many observational reports of reduced activity, often referred to as torpidity, in calanoid copepods from mid- and high- latitudes (e.g., Hirche, 1983; Alldredge et al., 1984; Hirche, 1987; Bathmann et al., 1990; Hirche, 1996; Auel et al., 2003; Hirche et al., 2006). Campbell (2003), citing Hirche (1983), described torpid Calanus copepods as having a distinctive “overwintering posture” in the laboratory, in which their first antennae is folded ventrally. Low intensity swimming is associated with low metabolic demand (Svetlichny and Hubareva, 2005) and is possibly adaptive in that it is less likely to attract tactile and visual predators than active swimming (e.g., Hirche, 1996; Hirche, 1998; Dale et al., 1999). However, these observations are not consistent with other studies reporting continued swimming and/or feeding during periods of metabolic reduction in boreal copepods such as C. helgolandicus (Corner et al., 1974; Williams and Conway, 1982; Williams and Conway, 1984) and C. euxinus (Svetlichny et al., 1998), as well as other high-latitude copepods (Norrbin, 1996). An “active diapause” that includes continued feeding, was previously reported in freshwater copepods (e.g., Wyngaard, 1988; Naess and Nilssen, 1991) and insects (e.g., Hodek, 2002).
Physiological indicators such as respiration rate, electron motor activity and clock gene expression can be useful detectors of diapause, but they are not immune to sampling and incubation artefacts, motivating studies to identify those indicators that most accurately simulate in-situ overwintering conditions (e.g., Båmstedt, 1980; Mayzaud, 1986; Campbell, 2003). It is likely that swimming activity integrates across such physiological and genetic processes and therefore an objective quantification of this may provide a novel indicator of diapause state. Our approach was to collect high-latitude C. finmarchicus from epipelagic and mesopelagic depths during late summer, with the aims of: i) comparing swimming activity to other metrics of physiological condition including mass-specific respiration rates, body size and lipid content, and in doing so; ii) better characterizing and distinguishing diapausing individuals of C. finmarchicus.
We sampled from the Fram Strait, northern Greenland Sea (Figure 1A), during August 2019 onboard RRS James Clark Ross. This region is a transition between southern boreal waters and northern polar waters and is a key area for pelagic production, where calanoid copepods comprise up to 70% of the zooplankton biomass (Smith, 1988; Hirche, 1991). Samples were collected at one slope station (D6; 79.2 °N, 6.6 °W; 20-22/08/19) and one basin station (F7; 78.9°N, 3.3°W; 13-14/08/19). In this region, C. finmarchicus are reported to enter dormancy in July and August (e.g., Hirche, 1987; Smith, 1988; Hirche, 1991) and D6 and F7 were considered to potentially represent differing overwintering habitats for C. finmarchicus in terms of both depth and temperature (e.g., Pepin et al., 2011; Jónasdóttir et al., 2019). Both stations were ice-free at the time of sampling.
Figure 1 (A) Bathymetric map of the North Atlantic and Arctic Ocean. The area sampled within the Fram Strait (indicated by the red rectangle), shows the locations of slope station D6 and basin station F7 (black circles) west of Svalbard. Light and dark blue contours represent 200 m and 2000 m isobaths respectively. (B, C) Vertical profiles of temperature, salinity and chlorophyll A between the surface and 600 m (temperature and salinity) and 270 m (chlorophyll a), at D6 (B) and F7 (C) also showing total water depth. Light (epipelagic) and dark grey rectangles (mesopelagic) represent the integrated upper and lower depths respectively from which copepods were collected. Physical environment data were provided by Changing Arctic Ocean/Robyn Owen (British Oceanographic Data Centre).
Vertical profiles of temperature and salinity were measured at both stations to 600 m depth by a ship-board conductivity, temperature, and depth profiler (CTD; SBE911plus, SeaBird Electronics). An in-situ chlorophyll fluorometer (Aquatracka III fluorometer, Chelsea Technologies) on the CTD measured chlorophyll a concentration to a depth of 270 m. Niskin bottles collected seawater from 250 m at each station (salinity: ~35‰), which was subsequently filtered (0.2 µm) for use in the copepod swimming activity and respiration experiments.
Copepods were sampled using a Hydrobios® Mammoth MultiNet system, comprising nine individual nets (1 m2 apertures, 300 µm mesh) that were towed vertically at 10 – 20 m min-1 (ascent speed). Two depth strata were targeted as part of a zooplankton sampling campaign, approximately 520 – 285 m and 170 – 5 m, hereafter referred to as mesopelagic and epipelagic, respectively. The upper and lower depths of the multinet system were set according to the vertical position of zooplankton at each station, as determined by an initial exploratory net deployment. In situ temperature ranges at mesopelagic depths were 0.9 – 1.9°C and 1.5 – 2.9°C in D6 and F7 respectively, and at epipelagic depths 3.8 – 6.9°C and 3.1 – 5.5°C at D6 and F7 respectively (Figures 1B, C).
During sampling and deck handling, zooplankton were shielded from changes in light intensity by covering the net cod-ends in dark material. Net contents were immediately transferred into filtered seawater and kept in lidded, light-excluding black buckets, within the onboard light and temperature-controlled room at 2°C. Stage CV C. finmarchicus were manually selected from samples using a stereomicroscope (ZEISS Stemi 2000-C) under a dim red light in an onboard temperature-controlled room, taking care to avoid visibly damaged or dead animals. To distinguish C. finmarchicus from its closely related congener, C. glacialis, we used lack of redness of the antennae, shown to be a good indicator for C. finmarchicus (e.g., Nielsen et al., 2014; Daase et al., 2018). Subsequent molecular analysis of multinet samples collected concurrently at the same stations as described here confirmed that 99 – 100% of the identified sequences returned from both stations were C. finmarchicus. Unknowns comprised less than 5% of the total sequences returned (pers. comm.: Penelope Lindeque, Plymouth Marine Laboratory).
Across both stations, swimming activity was monitored for a total of 357 individual copepods (167 and 190 from meso- and epipelagic depths respectively). At each station, swimming activity was measured for 6 days using a high-throughput quantitative behavioural assay. Specifically, individual copepod activity was monitored using modified LAM10 activity monitors (Trikinetics®). These are infrared (IR) light beam arrays which detect the movement of an individual copepod in a test chamber (clear acrylic tubes of volume 5 ml, diameter 10 mm and length 64 mm, containing 0.2 µm filtered seawater). IR beams were situated 5 mm above the bottom of each tube. Beam breaks were registered on a laptop computer using proprietary Trikinetics® software (filescan.exe). Six 32 channel LAM10 monitors were placed in constant darkness within an incubator at constant 4°C (D6) and 2.8°C (F7), consistent with the approximate water temperatures at the base of the pycnocline at the respective stations. On culmination of the swimming experiments, all copepods (minus 27 and 17 from meso- and epipelagic depths respectively due to death/loss) were photographed laterally using a Carl ZEISS AxioCam MRc5 camera) to provide images used for measurements of body size (prosome areas) and lipid sac area (both in mm2). Length/area measurements were made by digitally tracing perimeters which allowed calculation of the lipid sac area/prosome area (LA/PA) ratios. Calibrated area measurements were made using the open-source software ImageJ (https://imagej.net). The mean number of beam breaks per 30 minute interval per individual were compared between depths (mesopelagic and epipelagic) and stations (slope and basin) using either a one-way ANOVA or Kruskal-Wallis rank sum, after testing for normality (Shapiro-Wilk test) and equal variance (Brown-Forsythe test). Statistical analyses were performed using R v 4.1.3., with an α level of 0.05 for each test. Similar comparisons were performed with the morphometric data (prosome area and LA/PA).
For each station, oxygen consumption was measured in copepods collected from the same sampling events as those collected for the swimming activity experiment (described above). Ten C. finmarchicus CV were randomly sampled from each depth collection (meso- and epipelagic) and incubated for 2 days using a Loligo microplate respirometry system (1700 µl well plates containing 0.2 µm filtered seawater), calibrated to ambient collection temperatures. As with the swimming activity setup, respiration experiments were conducted in the dark within temperature-controlled incubators, at 4°C (D6) and 2.8°C (F7). Mass-specific respiration rates (µg O2 µgDW-1 h-1) were calculated from oxygen consumption data during a 100-minute period after stabilisation using the respR aquatic respiratory analysis package (Harianto et al., 2019; Harianto et al., 2021) within the R computing environment (Development Core Team R, 2008). These rates were first determined per individual copepod (µg O2 h-1) using oxygen concentrations between 80-90% air saturation to ensure independent respiration and adjusting for the background rate (0.2 µm filtered seawater only). Respiration rates were then corrected for copepod mass using a prosome length (PL) to dry weight (DW) regression from C. finmarchicus in the Nansen Basin in summer (DW = 0.0095 × PL3.3839; Hirche and Mumm, 1992). Mass-specific respiration rates were compared between depths and stations as described above.
Average temperatures within the sampled epipelagic and mesopelagic zones at D6 were 5.16 ± 1.17°C and 1.52 ± 0.40°C, respectively. Mean salinity values at D6 were 34.96 ± 0.13 (epipelagic) and 34.96 ± 0.01 (mesopelagic) (Figure 1B). At F7, average temperatures were 3.78 ± 0.53°C in epipelagic and 2.26 ± 0.44°C in mesopelagic zones, respectively. Mean salinities at F7 were 34.85 ± 0.30 (epipelagic) and 34.97 ± 0.02 (mesopelagic) (Figure 1C). Sea surface chlorophyll a concentrations were 2.5 ± 0.4 mg m-3 at D6 (Figure 1B) and 1.1 ± 0.7 mg m-3 at F7 (Figure 1C), and CTD profiles demonstrate that the water column of F7 was highly stratified with chlorophyll a maximum within the thermocline at 26 m (Figure 1B), while D6 had higher concentrations of chlorophyll a above its comparatively weaker thermocline (Figure 1C).
Morphometric analysis revealed that copepods from the mesopelagic had larger bodies and greater lipid fullness (LA/PA ratios) compared to the epipelagic individuals (ANOVA, P < 0.05). LA/PA ratios for both meso- and epipelagic copepods were higher on the slope than in the basin (Kruskal-Wallis ANOVA, P < 0.01, Table 1 and Figures 2, 3).
Table 1 Statistical comparison of each diapause indicator between stations (slope D6 and basin F7) and depths (epi- and meso-pelagic).
Figure 2 Morphometric characteristics of C. finmarchicus CV from epipelagic (yellow) and mesopelagic (blue-green) depths at slope station D6 and basin station F7. Boxplots of mean prosome area (PA) and lipid sac to prosome area (LA/PA) ratios per depth and station (box shows interquartile range around the median as the horizontal line, with black circles representing outliers).
Figure 3 Representative images of C. finmarchicus CV from epi- (left) and mesopelagic depths (right) at slope station D6, showing larger body sizes and lipid sacs in deeper animals. Scale bar = 1mm.
We found no significant difference in respiration rate between the meso- and epipelagic copepods at either station (One-Way ANOVA, P > 0.05, Table 1 and Figure 4). At D6 (4°C), average individual respiration rates were 0.27 ± 0.12 µg O2 h-1 (0.0010 ± 0.0006 µg O2 µgDW-1 h-1) for mesopelagic copepods and 0.34 ± 0.12 µg O2 h-1 (0.0014 ± 0.0005 µg O2 µgDW-1 h-1) for epipelagic copepods. At F7 (2.8°C), mesopelagic and epipelagic rates were 0.14 ± 0.08 µg O2 h-1 (0.0005 ± 0.0002 µg O2 µgDW-1 h-1) and 0.14 ± 0.13 µg O2 h-1 (0.0007 ± 0.0006 µg O2 µgDW-1 h-1) respectively. The mass-specific rates were significantly lower in the epipelagic copepods at the basin station when compared to the slope (Kruskal-Wallis ANOVA, P = 0.007). Mass-specific rates were also lower in mesopelagic copepods from the basin than slope, but the large variability in the data meant that this difference was not statistically significant (Table 1 and Figure 4).
Figure 4 Weight-specific respiration rates of C. finmarchicus CV from epipelagic (yellow) and mesopelagic (blue-green) depths at slope station D6 and basin station F7. Box shows interquartile range around the median as the horizontal line, with black circles representing outliers.
Comparing swimming activity in C. finmarchicus CV between depths, mesopelagic copepods were found to have a ~86% and ~91% reduction in mean number of beam breaks at D6 (1.12 ± 2.86 30 min-1 ind.-1) and F7 (2.11 ± 3.63 30 min-1 ind.-1) respectively when compared to those from the epipelagic (12.3 ± 10.8 30 min-1 ind.-1 and 15.1 ± 17.9 30 min-1 ind.-1, Kruskal-Wallis ANOVA, P < 0.001, Table 1 and Figure 5A). Epipelagic activity levels in individual animals did not differ significantly between stations (Kruskal-Wallis ANOVA, P = 0.91) but mesopelagic activity levels were higher at F7 (Kruskal-Wallis ANOVA P = 0.014, Table 1 and Figure 5B). The cumulative distribution of light beam breaks recorded by each activity monitor was found to follow two different distributions (two sample Kolmogorov-Smirnov test, D = 0.97, p<0.001) with ~5 beam breaks 30 min-1 marking the upper limit of mesopelagic activity and the lower limit of epipelagic activity recorded by each monitor (Figure 5C).
Figure 5 Swimming activity (IR light beam breaks) of C. finmarchicus CV from epipelagic (yellow) and mesopelagic (blue-green) depths at slope station D6 and basin station F7, over 6 days in constant darkness. (A) Mean beam breaks recorded by each activity monitor (n=32 animals) at 30-minute intervals across the 6-day monitoring period. (B) Box plots of mean beam breaks per individual CV copepod per depth and station, averaged over 6-days (showing interquartile range around the median as the horizontal line, with black circles representing outliers). (C) Histogram showing the frequency of mean beam breaks recorded by each activity monitor (n=32 animals) at 30-minute intervals, pooled across both stations. There were slight differences in mesopelagic collection depths at the two stations: 375 – 500 m and 285 – 520 m at D6 and F7 respectively.
We used a high-throughput quantitative behavioural assay to investigate swimming activity in C. finmarchicus in relation to diapause, using samples collected in the Fram Strait in late summer. We found 88.5% reduction in the mean activity of larger, more lipid rich mesopelagic individuals relative to those sampled from the epipelagic, indicating that deeper living individuals had entered diapause during, or prior to, August. Here we discuss the significance of these findings in relation to the suite of other measured diapause indicators and highlight the utility of reductions in swimming activity (torpidity) as a novel indicator of diapause in high-latitude copepods.
Previous observations of stage CV C. finmarchicus in diapause found them to remain motionless even when physically touched (e.g., Hirche, 1983; Hirche, 1987; Hirche et al., 2006) and illuminated (e.g., Alldredge et al., 1984). As well as being an adaptation for conserving energy, becoming torpid during diapause is considered to reduce the risk from tactile gelatinous and semi-gelatinous predators (e.g., Hirche, 1996; Dale et al., 1999). Nevertheless, a variety of terms have been used in earlier work to imply that diapause may be relatively “plastic”, with many qualitative terms used to describe this such as: active-diapause, pseudo-diapause, aleteo-diapause etc. (e.g., Naess and Nilssen, 1991; Hirche, 1996; Norrbin, 1996). This suggests that levels of torpidity may be variable within and between individuals over time. However, a conceptual model for the phases of diapause in Calanus developed by Hirche (1996) proposes that ‘deep torpor’ or ‘refractory phase diapause’ accompanying arrested development is only observed in autumn, after an initial ‘induction phase’ when some degree of metabolic reduction has already taken place. As copepods in the Greenland Sea and Fram Strait are reported to depart surface waters for overwintering territory in July (e.g., Hirche, 1987; Smith, 1988; Hirche, 1991), our finding of low swimming activity in late August (e.g., ≤5 beam breaks 30 min-1, Figure 5C) suggests that behavioural cessation occurs in the ‘induction phase’ of the proposed model by Hirche (1996), at a time when other processes (reduced ingestion / digestive track and metabolism) are still ramping down. Thus, measurements of swimming activity, as shown here, may provide an additional indicator to the physiological states of diapause and a simple and reliable tool to readily distinguish animals not in diapause (e.g., ≥5 beam breaks 30 min-1). Future work to compare these rates with individuals collected later in the season will help to rapidly, and potentially more reliably, distinguishing between ‘induction’, ‘refractory’ or ‘activation’ phases as described by Hirche (1996).
A key aim of this study was to compare measurements of swimming activity with other whole-animal diapause metrics including body size, lipid fullness and respiration. We show strong agreement between swimming activity and morphometric analyses in that animals with larger lipid reserves tend to be in deeper waters and less active. It has been shown that a certain degree of lipid storage is required in the congeners C. glacialis and C. hyperboreus before migration to diapause becomes an advantageous strategy (Schmid et al., 2018), a scenario likely to also hold true for C. finmarchicus at latitudes investigated here. Though LA/PA measurements are not widely available for Calanus copepods, our results from mesopelagic individuals are consistent with other measurements of lipid fullness in dormant C. finmarchicus (Pepin et al., 2011; Jónasdóttir et al., 2015; Schmid et al., 2018, Figure 3). It is therefore highly plausible that the mesopelagic individuals, which had accrued high LA/PA and less variable ratios than animals collected at epipelagic depths, had entered diapause, with a corresponding cessation of swimming activity.
Our results also indicate that mesopelagic individuals were larger in size than shallower counterparts. Differences in size can also be explained by variable feeding rates, quantity, or quality of food available to individuals (Pepin et al., 2011), or timing of development into the CV stage. Mechanistic models have found that individuals with better body condition (i.e., high fat/somatic body mass ratio) are able to afford the less risky option of entering diapause early to avoid predation (Fiksen and Carlotti, 1998). Moreover, in the same model, smaller and low body condition individuals were likely to remain at the surface in late summer, a behaviour that would be adaptive over starvation during the winter (Fiksen and Carlotti, 1998). Irrespective of the proximate factors involved, our data support that there are smaller copepods in the epipelagic and that these are more active than larger animals in the mesopelagic. This suggests that these smaller epipelagic copepods are actively foraging, presumably to increase lipid reserves with which to enter diapause successfully later in the summer or autumn (e.g., Smith, 1988).
Several studies have reported up to 70 – 90% reduction in respiration between active and dormant Calanus (Auel et al., 2003; Bailey, 2010 and references therein; Maps, 2014), yet the magnitude of metabolic reduction during the initiation phase of diapause remains under debate (Baumgartner and Tarrant, 2017). Intense metabolic reduction has been detected in the capital breeder C. hyperboreus sampled from ~2300 m in the Fram Strait (Auel et al., 2003; Hirche et al., 2006). Perhaps the shallower depths from which C. finmarchicus was sampled here (~500 m) explains the absence of consistent observations of reduced respiration rates. C. hyperboreus in the Greenland Sea are reported to migrate to overwintering depths as early as April (Hirche, 1997). Jónasdóttir et al. (2019) compiled C. finmarchicus overwintering respiration data from numerous studies which together encompassed a wide range of habitats in the North Atlantic and sub-Arctic. Over this distributional range, the mean respiration rate was 1.34 µg C d-1 (maximum: 2.20 µg C d-1 and minimum 0.65 µg C d-1). Comparing this with our data, we obtain average values within, or just above, this range. Similarly, Smith (1988) sampled C. finmarchicus (CV, AF) in the Fram Strait in June and July 1984, reporting mass-specific respiration rates of 0.0006 - 0.0026 µg O2 µg DW-1 h-1. This matches well with the distribution of weight specific rates we report in Figure 4, although we did observe several individuals with rates below 0.0006 µg O2 µg DW-1 h-1 at F7, possibly since our study was carried out later in the year, when more animals would be in deep diapause.
Overall, respiration in C. finmarchicus CV show no clear difference between epi- and mesopelagic animals, but rates more closely resembled an overwintering metabolic state at basin station F7, and an active metabolic state at slope station D6. The high variability in respiration rates between individuals at both stations and depths reflect the likelihood of a mix of metabolic states present. This could be due to differences in the time since descent to mesopelagic depths, with late descenders (‘stragglers’; Smith, 1988), still exhibiting higher respiration rates. We also note that swimming activity in mesopelagic individuals was, on the rare occasion, comparable to that of epipelagic animals (Figure 5C). We propose that further work should focus on the relationships between swimming activity and seasonal metabolic cycles using large sample numbers, as well as possible links with diel patterns of gene expression in early diapause (Tarrant et al., 2008; Häfker et al., 2018a; Häfker et al., 2018b; Lenz et al., 2021).
The relationship between respiration rate and swimming activity is not as apparent as with the other metrics measured. The observed reduction in swimming activity in mesopelagic animals without a large drop in respiration warrants further discussion. This could be due in part to our measurement of slope mesopelagic copepods at slightly warmer temperatures than they would experience in situ and that the marginal differences between depths at the slope station (D6) would likely be stronger if the mesopelagic copepods were tested at a colder temperature. However, results from this and other studies suggest that reduced metabolism does not necessarily translate into reduced swimming or feeding activity (Corner et al., 1974; Williams and Conway, 1982; Williams and Conway, 1984; Norrbin, 1996; Svetlichny et al., 1998). This indicates that respiration measurements, by themselves, would not be able to distinguish ‘active’ diapause (Norrbin, 1996) from other diapause states. Interestingly, Båmstedt (1980) found that respiration rates of the copepod Acartia tonsa declined almost immediately after transfer to the lab, while the activity of the electron transport system took several days to change after collection. Other studies have noted unexpected relationships (or lack thereof) between oxygen consumption and weight in carbon (e.g., Ingvarsdóttir, 1998), or between seasonal oxygen consumption and life cycle events (e.g., Campbell, 2003 and references therein). It should be noted that in copepods, swimming typically contributes 30 – 40% to the total maintenance costs (Alcaraz and Strickler, 1988) and hence an active animal should have a much higher respiration rate than an inactive one. This is exemplified in C. helgolandicus in which a six-fold increase in respiration was observed at maximum activity when compared with being motionless (Svetlichny and Umanskaya, 1991). However, individual variability in respiration rate between active and inactive animals has been reported (e.g., Båmstedt, 1980), hence demonstrating that measurements on individuals is important. The microrespirometry approach employed here is ideal for measuring low oxygen consumption rates in individual animals, but the small incubation chambers constrain nearly all activity. Subsequent studies should make activity and respiration measurements on the same individuals.
Here we show that swimming activity, when combined with morphometric data and collection depth, provides an early, clear, and consistent indication of life history transitions in C. finmarchicus. The methodology we describe has potential applications in understanding the advantages, plasticity and energy budgets associated with diapause in this important group of copepods.
The finding that animals which remain in the epipelagic are active is perhaps not surprising. As was reviewed by Varpe (2012), there are trade-offs in remaining active and feeding at this time, with the optimal decision dependent on several exogenous (e.g., depth, temperature, predation pressure and latitude) and endogenous factors (e.g., lipid volume and storage capacity, reproduction strategy). For example, in Arctic fjords with few predators and rich organic food supply, continued feeding during winter at depth, in combination with reduced metabolism, confers an advantage for lipid-poor individuals. This explanation may offer insights into similar observations in other habitats i.e., the ubiquity of high activity in Arctic fjords during the Polar Night as highlighted by Berge et al. (2015). The ‘decision’ to remain active will undoubtedly depend on food availability and the risk from predators dictated by local community structure. How this is scaled up to open ocean environments, where zooplankton populations are more partial to advection and where predators are more ephemeral, would need further investigation.
The insights gained from including swimming activity with a clearly defined threshold between epi- and mesopelagic animals (e.g., ~5 beam breaks 30 min-1, Figure 5) alongside more typical diapause indicators (depth of collection and body size) will help to interpret the duration of energy reserves for torpid and active animals. To do so requires linking individual variability in swimming activity with respiration within the population, thereby improving estimates of respired carbon released at depth during overwintering (Tarling et al., 2022). Such estimates would strengthen future attempts to calculate the magnitude of the Calanus lipid pump (Jónasdóttir et al., 2015; Visser et al., 2017).
The original contributions presented in the study are included in the article/supplementary materials. Further inquiries can be directed to the corresponding author.
All authors (JG, JF, GT, JC and KL) designed the study, contributed to data analysis, and drafted the manuscript. JG, JF and GT additionally collected field samples and carried out the lab work JG and JF contributed equally to this work. All authors contributed to the article and approved the submitted version.
Funding was provided by the CHASE project (NE/R012733/1), part of the Changing Arctic Ocean (CAO) program, jointly funded by the UKRI Natural Environment Research Council (NERC) and the German Federal Ministry of Education and Research (BMBF). Cruise time was supported by the CAO project DIAPOD (NE/P006280/2). Additional funds to support KL and JG were provided by the CAO project Arctic PRIZE (NE/P006302/1).
The authors declare that the research was conducted in the absence of any commercial or financial relationships that could be construed as a potential conflict of interest.
All claims expressed in this article are solely those of the authors and do not necessarily represent those of their affiliated organizations, or those of the publisher, the editors and the reviewers. Any product that may be evaluated in this article, or claim that may be made by its manufacturer, is not guaranteed or endorsed by the publisher.
We express gratitude to cruise leader Prof. David Pond and officers and crew of the R/V James Clark Ross for their professionalism and dedication during sampling operations. We also wish to thank other participating CAO scientists for their support during sampling and on-board lab work. We thank Vaila Grigg for support in morphological analysis, and Helen Parry and Penny Lindeque for providing molecular data.
Alcaraz M., Strickler J. R. (1988). Locomotion in Copepods: Pattern of Movements and Energetics of Cyclops. Hydrobiol 167, 409–414. doi: 10.1007/BF00026333
Alldredge A. L., Robison B. H., Fleminger A., Torres J. J., King J. M., Hamner W. M. (1984). Direct Sampling and In Situ Observation of a Persistent Copepod Aggregation in the Mesopelagic Zone of the Santa Barbara Basin. Mar. Biol. 80, 75–81. doi: 10.1007/BF00393130
Auel H., Klages M., Werner I. (2003). Respiration and Lipid Content of the Arctic Copepod Calanus hyperboreus Overwintering 1 M Above the Seafloor at 2,300 M Water Depth in the Fram Strait. Mar. Biol. 143, 275–282. doi: 10.1007/s00227-003-1061-4
Bailey A. (2010). Lipids and Diapause in Calanus spp. In a High-Arctic Fjord: State-Dependent Strategies? Tracking Lipids Through the Polar Night (UNIS). Norway: PhD thesis University of Svalbard.
Båmstedt U. (1980). ETS Activity as an Estimator of Respiratory Rate of Zooplankton Populations. The Significance of Variations in Environmental Factors. J. Exp. Mar. Biol. Ecol. 42, 267–283. doi: 10.1016/0022-0981(80)90181-1
Bathmann U., Noji T., von Bodungen B. (1990). Copepod Grazing Potential in Late Winter in the Norwegian Sea – A Factor in the Control of Spring Phytoplankton Growth? Mar. Ecol. Prog. Ser. 60, 225–233. doi: 10.3354/meps060225
Baumgartner M. F., Tarrant A. M. (2017). Physiology and Ecology of Diapause in Marine Copepods. Ann. Rev. Mar. Sci. 9, 387–411. doi: 10.1146/annurev-marine-010816-060505
Berge J., Renaud P. E., Darnis G., Cottier F., Last K., Gabrielsen T. M., et al. (2015). In the Dark: A Review of Ecosystem Processes During the Arctic Polar Night. Prog. Oceanogr. 139, 258–271. doi: 10.1016/j.pocean.2015.08.005
Campbell R. W. C. (2003). Overwintering Ecology and Ecophysiology of Neocalanus Plumchrus PhD thesis University of Victoria. Available at: http://hdl.handle.net/1828/8085.
Corner E. D. S., Head R. N., Kilvington C. C., Marshall S. M. (1974). On the Nutrition and Metabolism of Zooplankton. IX. Studies Relating to the Nutrition of Overwintering Calanus. J. Mar. Biol. Ass. U. K. 54, 319–331. doi: 10.1017/S0025315400058574
Daase M., Kosobokova K., Last K. S., Cohen J. H., Choquet M., Hatlebakk M., et al. (2018). New Insights Into the Biology of Calanus spp. (Copepoda) Males in the Arctic. Mar. Ecol. Prog. Ser. 607, 53–69. doi: 10.3354/meps12788
Dale T., Bagøien E., Melle W., Kaartvedt S. (1999). Can Predator Avoidance Explain Varying Overwintering Depth of Calanus in Different Oceanic Water Masses? Mar. Ecol. Prog. Ser. 179, 113–121. doi: 10.3354/meps179113
Development Core Team R. (2008). R: A Language and Environment for Statistical Computing (Vienna: R Foundation for Statistical Computing).
Fiksen C., Carlotti F. (1998). A Model of Optimal Life History and Diel Vertical Migration in Calanus finmarchicus. Sarsia N. Atl. Mar. Science. 83, 129–147. doi: 10.1080/00364827.1998.10413678
Greene C. H., Pershing A. J., Kenney R. D., Jossi J. W. (2003). Impact of Climate Variability on the Recovery of Endangered North Atlantic Right Whales. Oceanography 16, 96–101. doi: 10.5670/oceanog.2003.16
Häfker N. S., Teschke M., Hüppe L., Meyer B. (2018b). Calanus finmarchicus Diel and Seasonal Rhythmicity in Relation to Endogenous Timing Under Extreme Polar Photoperiods. Mar. Ecol. Prog. Ser. 603, 79–92. doi: 10.3354/meps12696
Häfker N. S., Teschke M., Last K. S., Pond D. W., Hüppe L., Meyer B. (2018a). Calanus finmarchicus Seasonal Cycle and Diapause in Relation to Gene Expression, Physiology, and Endogenous Clocks. Limnol. Oceanogr. 63, 2815–2838. doi: 10.1002/lno.11011
Harianto J., Aldridge J., Torres Gabarda S. A., Grainger R. J., Byrne M. (2021). Impacts of Acclimation in Warm-Low pH Conditions on the Physiology of the Sea Urchin Heliocidaris erythrogramma and Carryover Effects for Juvenile Offspring. Front. Mar. 10 doi: 10.3389/fmars.2020.588938
Harianto J., Carey N., Byrne M. (2019). respR – An R Package for the Manipulation and Analysis of Respirometry Data. Methods Ecol. Evol. 10, 912–920. doi: 10.1111/2041-210x.13162
Hirche H.-J. (1983). Overwintering of Calanus finmarchicus and Calanus helgolandicus. Mar. Ecol. Prog. Ser. 11, 281–290. doi: 10.3354/meps011281
Hirche H.-J. (1987). Temperature and Plankton II. Effect on Respiration and Swimming Activity in Copepods From the Greenland Sea. Mar. Biol. 94, 347–356. doi: 10.1007/BF00428240
Hirche H.-J. (1991). Distribution of Dominant Calanoid Copepod Species in the Greenland Sea During Late Fall. Polar Biol. 11, 351–362. doi: 10.1007/BF00239687
Hirche H.-J. (1996). Diapause in the Marine Copepod, Calanus finmarchicus – A Review. Ophelia 44, 129–143. doi: 10.1080/00785326.1995.10429843
Hirche H.-J. (1997). Life Cycle of the Copepod Calanus Hyperboreus in the Greenland Sea. Mar. Biol. 128, 607–618. doi: 10.1007/s002270050127
Hirche H.-J. (1998). Dormancy in Three Calanus Species (C. finmarchicus, C. glacialis and C. hyperboreus) From the North Atlantic. Spec. Issues Adv. Limnol. 52, 359–369.hdl:10013/epic.16802
Hirche H.-J., Mumm N. (1992). Distribution of Dominant Copepods in the Nansen Basin, Arctic Ocean, in Summer. Deep-Sea Res. I: Oceanogr. Res. Pap. 39, S485–S505. doi: 10.1016/S0198-0149(06)80017-8
Hirche H.-J., Muyakshin S., Klages M., Auel H. (2006). Aggregation of the Arctic Copepod Calanus hyperboreus Over the Ocean Floor of the Greenland Sea. Mesopelagic Sea Res. I 53, 310–320. doi: 10.1016/j.dsr.2005.08.005
Hodek I. (2002). Controversial Aspects of Diapause Development. Eur. J. Entomol. 99, 163–173. doi: 10.14411/eje.2002.024
Ingvarsdóttir A. (1998). Seasonal Changes in Physiology and Chemical Composition of Calanus finmarchicus Late Copepodite Stages. Ph.D. Thesis. University of Aberdeen. Available at: https://ethos.bl.uk/OrderDetails.do?uin=uk.bl.ethos.287608.
Ingvarsdóttir A., Houlihan D. F., Heath M. R., Hay S. J. (1999). Seasonal Changes in Respiration Rates of Copepodite Stage V Calanus finmarchicus (Gunnerus). Fish. Oceanogr. 8, 73–83. doi: 10.1046/j.1365-2419.1999.00002.x
Jónasdóttir S. H., Visser A. W., Richardson K., Heath M. R. (2015). Seasonal Copepod Lipid Pump Promotes Carbon Sequestration in the Mesopelagic North Atlantic. Proc. Natl. Acad. Sci. U.S.A. 112, 12122–12126. doi: 10.1073/pnas.1512110112
Jónasdóttir S. H., Wilson R. J., Gislason A., Heath M. J. (2019). Lipid Content in Overwintering Calanus finmarchicus Across the Subpolar Eastern North Atlantic Ocean. Limnol. Oceanogr. 9999, 1–15. doi: 10.1002/lno.11167
Karnovsky N. J., Kwaśniewski S., Węsławski J. M., Walkusz W., Beszczynska-Möller A. (2003). Foraging Behavior of Little Auks in a Heterogeneous Environment. Mar. Ecol. Prog. Ser. 253, 289–303. doi: 10.3354/meps253289
Kwaśniewski S., Gluchowska M., Walkusz W., Karnovsky N. J., Jakubas D., Wojczulanis-Jakubas K., et al. (2012). Interannual Changes in Zooplankton on the West Spitsbergen Shelf in Relation to Hydrography and Their Consequences for the Diet of Planktivorous Seabirds. ICES J. Mar. Sci. 69, 890–901. doi: 10.1093/icesjms/fss076
Lenz P. H., Roncalli V., Cieslak M. C., Tarrant A. M., Castelfranco A. M., Hartline D. K. (2021). Diapause vs. Reproductive Programs: Transcriptional Phenotypes in a Keystone Copepod. Commun. Biol. 4, 426. doi: 10.1038/s42003-021-01946-0
Leu E., Søreide J. E., Hessen D. O., Falk-Petersen S., Berge J. (2011). Consequences of Changing Sea-Ice Cover for Primary and Secondary Producers in the European Arctic Shelf Seas: Timing, Quantity, and Quality. Prog. Oceanogr. 90, 18–32. doi: 10.1016/j.pocean.2011.02.004
Maps F. (2014). A Metabolic Approach to Dormancy in Pelagic Copepods Helps Explaining Inter- and Intra-Specific Variability in Life-History Strategies. J. Plankton Res. 36, 18–30. doi: 10.1093/plankt/fbt100
Mayzaud P. (1986). “Enzymatic Measurements of Metabolic Processes Concerned With Respiration and Ammonia Excretion,” in The Biological Chemistry of Marine Copepods. Eds. Comer E. D. S., O’ Hara S. C. M. (Oxford: Clarendon Press), 226–259.
Naess T., Nilssen J. P. (1991). Diapausing Fertilized Adults: A New Pattern of Copepod Life Cycle. Oecologia 86, 368–371. doi: 10.1007/BF00317602
Nielsen T. G., Kjellerup S., Smolina I., Hoarau G., Lindeque P. (2014). Live Discrimination of Calanus glacialis and C. finmarchicus Females: Can We Trust Phenological Differences? Mar. Biol. 161, 1299–1306. doi: 10.1007/s00227-014-2419-5
Norrbin M. F. (1996). Timing of Diapause in Relation to the Onset of Winter in the High-Latitude Copepods Pseudocalanus acuspes and Acartia longiremis. Mar. Ecol. Prog. Ser. 142, 99–109. doi: 10.3354/meps142099
Payton L., Noirot C., Last K. S., Grigor J. J., Hüppe L., Conway D. V. P., et al. (2022). Annual Transcriptome of a Key Zooplankton Species, the Copepod Calanus finmarchicus. Ecol. Evol. 12, e8605. doi: 10.1002/ece3.8605
Pepin P., Parrish C. C., Head E. J. H. (2011). Late Autumn Condition of Calanus finmarchicus in the Northwestern Atlantic: Evidence of Size-Dependent Differential Feeding. Mar. Ecol. Prog. Ser. 423, 155–166. doi: 10.3354/meps08952
Pond D., Tarling G. (2011). Phase Transition of Wax Esters Adjust Buoyancy in Diapausing Calanoides acutus. Limnol. Oceanogr. 56, 1310–1318. doi: 10.4319/lo.2011.56.4.1310
Pond D. W. (2012). The Physical Properties of Lipids and Their Role in Controlling the Distribution of Zooplankton in the Oceans, J. Plankton. Res., 34(6)443–453. doi: 10.1093/plankt/fbs027
Prokopchuk I., Sentyabov E. (2006). Diets of Herring, Mackerel, and Blue Whiting in the Norwegian Sea in Relation to Calanus finmarchicus Distribution and Temperature Conditions. ICES J. Mar. Sci. J. Cons. 63, 117–127. doi: 10.1016/j.icesjms.2005.08.005
Rey-Rassat C., Irigoien X., Harris R., Carlotti F. (2002). Energetic Cost of Gonad Development in Calanus finmarchicus and C. helgolandicus. Mar. Ecol. Prog. Ser. 238, 301–306. doi: 10.3354/meps238301
Schmid M. S., Maps F., Fortier L. (2018). Lipid Load Triggers Migration to Diapause in Arctic Calanus Copepods – Insights From Underwater Imaging. J. Plankton Res. 40, 311–325. doi: 10.1093/plankt/fby012
Smith S. L. (1988). Copepods in Fram Strait in Summer: Distribution, Feeding and Metabolism. J. Mar. Res. 46, 145–181. doi: 10.1357/002224088785113720
Svetlichny L. S., Hubareva E. S. (2005). The Energetics of Calanus euxinus: Locomotion, Filtration of Food and Specific Dynamic Action. J. Plankton Res. 27, 671–682. doi: 10.1093/plankt/fbi041
Svetlichny L. S., Hubareva E. S., Arashkevich E. G. (1998). Physiological and Behavioural Response to Hypoxia in Active and Diapausing Stage V Copepodites of Calanus euxinus. Arch. Hydrobiol. Spec. Issues Adv. Limnol. 52, 507–519.
Svetlichny L. S., Umanskaya A. V. (1991). The Oxygen Cost of Calanus Helgolandicus Locomotion. Oceanology 31, 770–777.
Tarling G. A., Freer J. J., Banas N. S., Belcher A., Blackwell M., Castellani C., et al. (2022). Can a Key Boreal Calanus Copepod Species Now Complete Its Life-Cycle in the Arctic? Evidence and Implications for Arctic Food-Webs. Ambio 51, 333–344. doi: 10.1007/s13280-021-01667-y
Tarrant A. M., Baumgartner M. F., Verslycke T., Johnson C. L. (2008). Differential Gene Expression in Diapausing and Active Calanus finmarchicus (Copepoda). Mar. Ecol. Prog. Ser. 355, 193–207. doi: 10.3354/meps07207
Varpe Ø (2012). Fitness and Phenology: Annual Routines and Zooplankton Adaptations to Seasonal Cycles. J. Plankton. Res. 34, 267–276. doi: 10.1093/plankt/fbr108
Visser A., Grønning J. B., Jónasdóttir S. H. (2017). Calanus hyperboreus and the Lipid Pump. Limnol. Oceanogr. 62, 1155–1165. doi: 10.1002/lno.10492
Vogedes D., Eiane K., Båtnes A. S., Berge J. (2014). Variability in Calanus spp. Abundance on Fine-to Mesoscales in an Arctic Fjord: Implications for Little Auk Feeding. Mar. Biol. Res. 10, 437–448. doi: 10.1080/17451000.2013.815781
Williams R., Conway D. V. P. (1982). Population Growth and Vertical Distribution of Calanus helgolandicus in the Celtic Sea. Neth. J. Sea. Res. 16, 185–194. doi: 10.1016/0077-7579(82)90029-1
Williams R., Conway D. V. P. (1984). Vertical Distribution, and Seasonal and Diurnal Migration of Calanus helgolandicus in the Celtic Sea. Mar. Biol. 79, 63–73. doi: 10.1007/BF00404986
Wilson R. J., Banas N. S., Heath M. R., Speirs D. C. (2016a). Projected Impacts of 21st Century Climate Change on Diapause in Calanus Finmarchicus. Glob. Change Biol. 22, 3332–3340. doi: 10.1111/gcb.13282
Wilson R. J., Heath M. R., Speirs D. C. (2016b). Spatial Modeling of Calanus finmarchicus and Calanus helgolandicus: Parameter Differences Explain Differences in Biogeography. Front. Mar. Sci. 3. doi: 10.3389/fmars.2016.00157
Keywords: copepod, torpidity, metabolism, lipid, diapause, Fram Strait, Arctic Ocean
Citation: Grigor JJ, Freer JJ, Tarling GA, Cohen JH and Last KS (2022) Swimming Activity as an Indicator of Seasonal Diapause in the Copepod Calanus finmarchicus. Front. Mar. Sci. 9:909528. doi: 10.3389/fmars.2022.909528
Received: 31 March 2022; Accepted: 27 May 2022;
Published: 30 June 2022.
Edited by:
Bernardo Antonio Perez Da Gama, Fluminense Federal University, BrazilReviewed by:
Gerald Francis Darnis, Laval University, CanadaCopyright © 2022 Grigor, Freer, Tarling, Cohen and Last. This is an open-access article distributed under the terms of the Creative Commons Attribution License (CC BY). The use, distribution or reproduction in other forums is permitted, provided the original author(s) and the copyright owner(s) are credited and that the original publication in this journal is cited, in accordance with accepted academic practice. No use, distribution or reproduction is permitted which does not comply with these terms.
*Correspondence: Kim S. Last, a2ltLmxhc3RAc2Ftcy5hYy51aw==
†These authors have contributed equally to this work
Disclaimer: All claims expressed in this article are solely those of the authors and do not necessarily represent those of their affiliated organizations, or those of the publisher, the editors and the reviewers. Any product that may be evaluated in this article or claim that may be made by its manufacturer is not guaranteed or endorsed by the publisher.
Research integrity at Frontiers
Learn more about the work of our research integrity team to safeguard the quality of each article we publish.