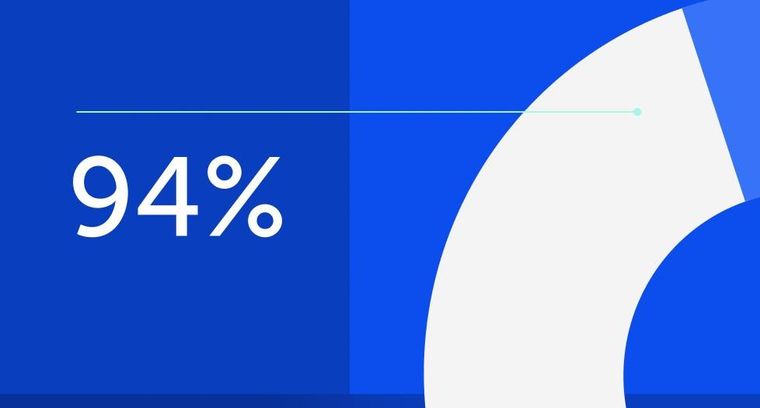
94% of researchers rate our articles as excellent or good
Learn more about the work of our research integrity team to safeguard the quality of each article we publish.
Find out more
ORIGINAL RESEARCH article
Front. Mar. Sci., 12 August 2022
Sec. Marine Ecosystem Ecology
Volume 9 - 2022 | https://doi.org/10.3389/fmars.2022.908638
This article is part of the Research TopicThe Physiology, Ecology and Biogeochemistry of Marine Zooplankton in a Changing Arctic OceanView all 15 articles
The Arctic Ocean is rapidly changing. Air temperature is rising two to four times faster in the Arctic than the global average, with dramatic consequences for the ecosystems. Polar zooplankton species have to cope with those increasing temperatures, whilst simultaneously facing increasing competition by boreal-Atlantic sister species advected into the Arctic Ocean via a stronger Atlantic inflow. To assess the sensitivity of Arctic and Atlantic zooplankton to rising temperatures, respiration rates of dominant Arctic species (Calanus hyperboreus, Calanus glacialis, Paraeuchaeta glacialis, Themisto libellula) and their co-occurring Atlantic congeners (Calanus finmarchicus, Paraeuchaeta norvegica, Themisto abyssorum) were measured at ambient temperatures and simulated conditions of ocean warming from 0 to 10°C during three expeditions with RV Polarstern to the Arctic Fram Strait. Arctic zooplankton showed only slowly increasing respiration rates with increasing temperatures, also indicated by low Q10 ratios. In contrast, boreal-Atlantic representatives responded to higher temperatures by a rapid and steeper increase in their respiration rates (higher Q10), suggesting higher metabolic activity. These results imply that Arctic species are physiologically more tolerant to ocean warming than expected but might be outcompeted by their Atlantic congeners beyond a certain temperature threshold in areas of strong distribution overlap. Thus, the ‘Atlantification’ of the Arctic zooplankton community seems to be driven rather by ecological interactions than by physiological limitations. Changes in zooplankton community composition and biodiversity will have major consequences for trophodynamics and energy flux in Arctic ecosystems, since polar species tend to be larger than their southern counterparts and have a higher lipid content, providing more energy-rich food for higher trophic levels.
The Arctic Ocean and adjacent ice-covered seas are the regions that are most rapidly affected by global warming. Air surface temperature is rising two to four times faster in the Arctic than the global average (Bekryaev et al., 2010; Masson-Delmotte et al., 2013; Meredith et al., 2019), and the sea surface and Atlantic Water core temperatures in the Arctic Ocean have been increasing for the last decades (Polyakov et al., 2012; Polyakov et al., 2013; Hartmann et al., 2013; Meredith et al., 2019).
The increasing northward inflow of warm and saline Atlantic waters is referred to as ‘Atlantification’ or ‘Borealization’ of the Arctic (Polyakov et al., 2017). Associated with Atlantic water masses are boreal-Atlantic species, which are rapidly expanding their ranges poleward (Beaugrand et al., 2009). For instance, copepods (Beaugrand et al., 2002; Weydmann et al., 2014), gelatinous zooplankton (Mańko et al., 2020) and fishes (Fossheim et al., 2015; Vihtakari et al., 2018) are shifting northward in their biogeographic distribution. This is emphasized by first evidence of reproductive success of boreal zooplankton species, such as the amphipod Themisto compressa and the euphausiid Thysanoessa raschii near the Svalbard archipelago in Fram Strait and in Kongsfjorden, Svalbard, respectively (Buchholz et al., 2012; Kraft et al., 2013). Thus, the Atlantic-influenced regions of the Arctic Ocean are increasingly invaded and successfully colonized by boreal-Atlantic species, resulting in the Atlantification of the Arctic fauna.
In Fram Strait, the only deep-water connection of the Arctic with the Atlantic Ocean, three species of Calanus co-occur, with two ‘true polar-Arctic’ species, Calanus hyperboreus and C. glacialis, and one expatriate from the boreal-Atlantic, C. finmarchicus (Wassmann et al., 2015). Copepods of the genus Calanus are a key component in the Arctic marine food web, as they usually dominate in terms of biomass and represent a crucial link between primary production and higher trophic levels (Mumm et al., 1998; Auel and Hagen, 2002; Kosobokova and Hirche, 2009). The three species share similarities considering morphology and life-history traits; however, they differ particularly considering size, life-cycle duration and phenology (Møller and Nielsen, 2020; Daase et al., 2021). Both Arctic species are characterized by larger size, higher lipid content and life-cycles exceeding one year (Daase et al., 2021). Reproductive strategies of polar Calanus species are highly adapted to the harsh and strongly seasonal Arctic conditions. C. hyperboreus is a capital breeder, i.e. using stored lipid resources for reproduction (Daase et al., 2021). Hence, C. hyperboreus can reproduce independent of immediate food supply and is able to initiate reproduction prior to the onset of the ice algal bloom. By the time the spring bloom is commencing, nauplii of C. hyperboreus have reached their feeding stage (from naupliar stage 3 onward) and are able to utilize the early ice algal bloom for growth and development (Jung-Madsen et al., 2013; Daase et al., 2021). C. glacialis is also well adapted due to a high flexibility in its reproductive strategy, being able to fuel reproduction both by lipid reserves and by utilizing the early ice algal bloom (Hirche and Kattner, 1993; Wold et al., 2011). C. finmarchicus, in contrast, is smaller in size, hence possesses comparably smaller lipid reserves, has a one-year life-cycle and is an income breeder, i.e. reproduction is dependent on direct food intake (Daase et al., 2021).
Shifting from a community dominated by large and lipid-rich copepods with multi-year life cycles to one with smaller, less lipid-rich and shorter-lived species could have consequences for the entire Arctic food web. For instance, the Arctic planktivorous little auk (Alle alle) and juvenile polar cod (Boreogadus saida) are strongly dependent on the larger Arctic Calanus species and, in the case of little auks, even extend their foraging trips to find their preferred prey (Karnovsky et al., 2010; Kwaśniewski et al., 2010; Bouchard and Fortier, 2020). It is, thus, crucial to better understand the processes driving transitions in community composition.
As a consequence of species-specific temperature tolerance limits and temperature-dependent effects on performance, organisms occupy distinct thermal niches, which are an important factor in defining their biogeographical distribution (Schulte et al., 2011). Polar marine ectotherms are considered to have a comparably narrow optimum at low temperatures and a limited ability to cope with rising environmental temperatures (Peck, 2002). This stenothermy is particularly pronounced in Antarctic ectotherms (Pörtner et al., 2007; Peck et al., 2010; Sandersfeld et al., 2015), but has also been reported for Arctic invertebrates and fishes (Franklin et al., 2013; Huenerlage and Buchholz, 2015).
The concept of oxygen- and capacity-limited thermal tolerance (OCLTT) explains temperature effects on the aerobic scope as a main driver in influencing energy availability for performance, and thus eventually the biogeographic distribution of species (Pörtner et al., 2017). The aerobic scope is determined by the difference between the standard and maximum metabolic rate and represents excess capacity of aerobic energy available for achieving fitness and supporting biological functions. Beyond a threshold temperature (pejus temperature), a mismatch between oxygen supply capacity and oxygen demand occurs, reducing the aerobic scope, and thus limiting available energy for essential biological processes such as foraging, growth and reproduction. While temperatures beyond the pejus temperature do not necessarily influence the survival of a species yet, they reduce its performance, and hence its competitive fitness (Pörtner, 2001; Verberk et al., 2016).
To assess the sensitivity of polar-Arctic versus boreal-Atlantic zooplankton to rising temperatures, we conducted respiration measurements as a proxy for metabolic activity at five temperatures ranging from 0 to 10°C with the three co-existing Calanus species in Arctic Fram Strait. In addition, pairs of polar versus boreal-Atlantic congeners of other zooplankton taxa, the predatory copepods Paraeuchaeta glacialis and P. norvegica and the hyperiid amphipods Themisto libellula and T. abyssorum, were included in the study. Specifically, we hypothesize that (1) polar species show a stenothermic response, i.e. rapidly increasing metabolic rates at lower temperatures (high Q10 value), followed by a narrow optimum and a sharp drop, including increased mortality at higher temperatures; (2) mass-specific respiration rates are generally higher in the smaller boreal-Atlantic species compared to their larger polar congeners; (3) the mechanism behind the Atlantification of the Arctic fauna is mainly driven by physiological limits of Arctic zooplankton to increasing temperatures.
Sampling was conducted at the end of summer during three cruises with RV Polarstern to Arctic Fram Strait in July-September 2016, 2017, and 2019 (PS100, PS107 and PS121, respectively, Figure 1).
Figure 1 Location of stations where zooplankton specimens were collected for respiration experiments during cruises PS100, PS107 and PS121. Numbers above dots indicate respective station number.
In situ temperatures were obtained with a CTD (Conductivity Temperature Depth) profiling system prior to each sampling of zooplankton. In situ temperatures did not vary distinctly between years, but rather between regions in Fram Strait. The western part of Fram Strait is under strong influence of the East Greenland Current (EGC), exporting cold and fresh Polar Surface Water out of the Arctic Ocean. Temperature profiles of the stations during the three cruises were characterized by temperatures below 0°C in epipelagic layers (Supplement Material S1). Between 200 to 300 m, temperatures increased up to a maximum of 3°C and then decreased again to around 0°C in 1000 m.
In the eastern part of Fram Strait, the West Spitsbergen Current (WSC) flows northward and transports rather warm, saline Atlantic water into the Arctic Ocean. Profiles during the three cruises showed high temperatures of 5 to 9°C in surface waters. With increasing depth temperatures steadily decreased to ≤0°C below 600 m.
The zone in between the two currents, here referred to as central Fram Strait, is characterized by a highly dynamic and turbulent hydrographic regime (Kawasaki and Hasumi, 2016; von Appen et al., 2018). While partly under influence of the EGC, branches of the WSC turn westward to ‘recirculate’ and subduct below the Polar Surface Water (Hattermann et al., 2016). The variability of the central Fram Strait was reflected in the temperature profiles during the three expeditions. Four stations showed similar profiles as the ones under influence of the EGC (PS100 Stns 48, 49 and PS121 Stns 29, 36), three showed a mix of both characteristics (PS100 Stns 46, 102 and PS107 Stn 21) and one was influenced by the WSC (PS107 Stn 34; Supplement Material S1).
Zooplankton sampling was conducted using either a multiple opening and closing net equipped with five nets for stratified vertical hauls (Hydrobios Multinet Midi, mouth opening 0.25 m2, mesh size 150 µm, hauling speed 0.5 m s-1) or a bongo net (mesh size: 200 to 500 µm, towing speed 1-2 kn) with filtering cod-ends, respectively. Vertical hauls with the Multinet usually sampled the entire water column from the seafloor or a maximum sampling depth of 1,500 m to the surface (Kanzow, 2017; Schewe, 2018; Metfies, 2020). Specimens used for respiration measurements were sorted immediately after the hauls in a temperature-controlled lab container (2 to 4°C). Only apparently healthy and active individuals were selected and kept at 0°C. 0°C were chosen, as it was within the in situ temperature range for all species.
Respiration rates of zooplankton organisms were measured on board at different temperatures (0, 4, 6, 8 and 10°C) non-invasively by optode respirometry using a 10-Channel Fiber Optic Oxygen Meter (OXY-10, PreSens, Precision Sensing GmbH, Regensburg, Germany). Each individual was only measured once at one of the experimental temperatures and they were selected randomly for the respective temperatures. Prior to the measurements, specimens were transferred from 0°C to the incubator, brought slowly to their respective experimental temperature and acclimated for at least 24 hours.
For the experiments, individuals were carefully transferred into gas-tight glass bottles containing 13 ml of 0.2 µm GF/F filtered and oxygenated seawater. The number of individuals per bottle depended on size, ontogenetic stage and metabolic activity with a maximum of six specimens per bottle (for Calanus finmarchicus copepodite stage C5). Experiments were run in a temperature-controlled incubator in the dark (refrigerator with external precision thermostat) for generally 8 to 24 hours with continuous measurements every 15 seconds. If oxygen levels fell below a critical level (below 60% air saturation), experiments were terminated earlier (usually in case of larger animals such as the amphipods Themisto spp.). In each experimental run, two channels of the multi-channel optode respirometer were used for animal-free controls to compensate for potential microbial oxygen consumption or production.
After the experiments, the taxonomic identification of species and developmental stages was confirmed under a dissecting microscope (Leica MS5) and amphipods were measured for body length. Calanus glacialis and C. finmarchicus are two sister species that are morphologically difficult to distinguish. Differentiation based on prosome length is a commonly used method to discriminate between the two congeners (Daase et al., 2018). However, due to an overlap of size distributions in regions of co-occurrence (Gabrielsen et al., 2012; Choquet et al., 2018) and depth-dependent size distributions (Kaiser et al., 2021), this can lead to individuals being misidentified, usually with a bias towards an underestimation of C. glacialis (Choquet et al., 2018; Daase et al., 2018). Nevertheless, C. glacialis reaches distinctly larger sizes than C. finmarchicus. Therefore, to avoid the overlapping size ranges, only larger individuals of the same stage were used for experiments with C. glacialis and smaller individuals for C. finmarchicus.
All individuals were deep-frozen at -80°C for subsequent dry mass (DM) determination after lyophilization for 48 hours, in the home laboratory at the University of Bremen, Germany. Deep-frozen at -80°C for subsequent dry mass (DM) determination after lyophilization for 48 hours, in the home laboratory at the University of Bremen, Germany. Individuals were then weighed using a microbalance (Sartorius MC215).
The first two hours of each measurement (or 20 min when measurements had to be terminated early due to oxygen depletion) were excluded from further analysis to avoid potential bias by handling stress or temperature adjustments at the start of the experiments. Animal-free controls were treated equally. The mean decline in dissolved oxygen concentration in the animal-free controls was subtracted from the respective measurements with animals of the same experimental run.
To compare respiration between different species, mass-specific respiration rates (MSRR) were calculated. Statistical analyses were performed using GraphPad Prism (version 8.0.2). Differences between MSRR during the three years (2016, 2017 and 2019) were tested for significance using Welch-ANOVA, as homogeneity of variance was not always met and sample size was highly variable, followed by the Games-Howell’s post hoc multiple comparison test. Both analyses were chosen, as they do not assume equal variances and sample sizes. No significant differences were detected in MSRR of the seven species between the three years at each temperature; thus, the experimental results from the different years were pooled for each species and stage.
MSRR were standardized for all species to exclude the factor body mass on respiration. For the standardization, log10 transformed MSRR of all species at 0°C were plotted against log10 transformed dry masses to obtain the scaling coefficient b (-0.25) from the linear slope of the regression line. Standardized MSRR were calculated as follows:
where RMSRR is the mass-specific respiration rate, DM is the respective dry mass, stDM is the genus- and stage-specific mean dry mass (2 mg for Calanus females, 1.2 mg for Calanus C5, 5.6 mg for Paraeuchaeta females, 1.8 mg for Paraeuchaeta C5, 7 mg for Themisto). Genus- and stage-specific DMs were obtained by averaging dry masses of all sampled individuals of the respective genus and stage.
Intraspecific temperature-dependent differences in MSRR were tested for significance using Welch-ANOVA, followed by Games-Howell’s post hoc multiple comparison test. Differences in slopes of linear regression performed on log10 transformed MSRR were tested for significance between species of the same genus to identify significant differences in the species-specific increase of MSRR.
Finally, species- and stage-specific Q10 values were calculated based on exponential regression of all MSRR data at the different temperatures. With the obtained exponential function, calculated MSRR rates for 10°C were divided by rates at 0°C, providing the Q10 values, i.e. the factorial increase associated with a 10°C rise in temperature. To exclude that Calanus females and C5s from greater depths had an impact on MSRR, Q10 values of specimens from the surface layers (0 – 100 m) were compared to Q10 values including individuals from deeper layers. Q10 values did not distinctly change, whether deeper-dwelling individuals were included in the analysis or not. We therefore assume that these specimens did not yet enter diapause and included all Calanus individuals in the analyses.
In total, 517 measurements of seven different polar (Calanus hyperboreus, Calanus glacialis, Paraeuchaeta glacialis, Themisto libellula) and boreal-Atlantic (Calanus finmarchicus, Paraeuchaeta norvegica, Themisto abyssorum) zooplankton species were conducted at 0, 4, 6, 8 and 10°C (Table 1 and Figure 2).
Table 1 Individual, mass-specific and standardized mass-specific respiration rates of polar-Arctic and boreal-Atlantic (grey highlighted) zooplankton species measured at different ambient temperatures (Exp. temp.) during three expeditions PS100 (2016), PS107 (2017) and PS121 (2019) with RV Polarstern to Arctic Fram Strait in summer (July-September).
Figure 2 Mass-specific (A, B) and standardized mass-specific (C) respiration rates (Stand. MSRR) of polar-Arctic (blue) versus boreal-Atlantic (red) zooplankton species from Fram Strait. Stand. MSRR were used for Themisto species, as they had highly variable dry masses. Box represents the interquartile range (lower edge: 25th percentile, the upper edge: 75th percentile, central line: median, whiskers extend to minimum and maximum values within 1.5 times the interquartile range). Dots represent potential outliers that are above or below 1.5 times the interquartile range, respectively. Asterisks show significant differences in standardized respiration rates between species at the respective temperature (*p≤ 0.05, **p≤ 0.01, ***p≤ 0.001). Values above the boxplots show number of measurements. (A) Calanus spp., (B) Paraeuchaeta spp., (C) Themisto spp.
In general, mass-specific respiration rates (MSRR) increased with temperature for all species and stages (Table 1 and Figure 2). Adult females of polar Calanus hyperboreus and C. glacialis showed a slow, but steady increase in MSRR (Figure 2A and Table 1). Respiration rates in these organisms doubled from 0 to 8°C, which represents a significant increase for C. hyperboreus (p<0.001) and C. glacialis females (p< 0.001; Games-Howell’s multiple comparison test). The increase from 0 to 6°C was also significant in C. glacialis (p= 0.05). A summary of all statistical analyses can be found in the Supplement Material S2.
In C. hyperboreus C5, MSRR remained rather stable between 0 and 6°C (mean values around 12 nmol O2 h-1 mg-1 DM for all three temperatures), but at 8 and 10°C an increase was noticeable to 20 and 27 nmol O2 h-1 mg-1 DM, respectively (0 and 8°C: p< 0.001). In C. glacialis C5 no distinct increase in MSRR was observed between 0 and 8°C (p> 0.999; Figure 2A and Table 1).
Adult females of C. finmarchicus showed similar MSRR at 0°C as their Arctic congeners (Figure 2A and Table 1). However, in contrast to the slow and steady increase of Arctic Calanus species, MSRR of boreal-Atlantic C. finmarchicus females showed a much more rapid and steeper increase, with a more than threefold increase in mean MSRR from 0 to 6°C (i.e. 24 to 90 nmol O2 h-1 mg-1 DM, Table 1 and Figure 2A). At 8°C the distinct rise in MSRR of C. finmarchicus females flattened into a plateau. MSRR of C. finmarchicus females were significantly different between 0 and 4°C (p= 0.04), 0 and 6°C (p< 0.001) and 4 and 6°C (p= 0.02). The slope of the regression line of log10 transformed MSRR with increasing temperature of C. finmarchicus females was significantly higher than the slope of females of C. hyperboreus (p= 0.0089) and C. glacialis (p= 0.0117).
Copepodite stages C5 of C. finmarchicus also showed a significant increase in MSRR from 0 to 8°C, with mean values increasing from 15 to 44 nmol O2 h-1 mg-1 DM, respectively (0 and 8°C: p= 0.02, 4 and 10°C: p= 0.01). The increase leveled off at 10°C (Figure 2A). The slope of the linear regression of MSRR with increasing temperature was significantly higher in C. finmarchicus C5 compared to C. hyperboreus C5 (p= 0.0004) and C. glacialis C5 (p= 0.0027).
Adult females of the predatory copepod species Paraeuchaeta glacialis and P. norvegica showed similar trends among each other with little change in MSRR between 0 and 4°C and an increase at 8°C (Table 1 and Figure 2B). At all temperatures, mean MSRR of the Arctic P. glacialis females were higher than those of their Atlantic congener P. norvegica (Table 1). Respiration of P. glacialis C5 changed only slightly with increasing temperature, with essentially no difference in mean MSRR from 0 to 10°C (20 and 21 nmol O2 h-1 mg-1 DM, respectively). While mean MSRR of P. norvegica C5 was similar to P. glacialis C5 at 0°C, a distinct increase of mean values could be observed for P. norvegica C5 at temperatures above 4°C (Table 1 and Figure 2B).
In the amphipods Themisto libellula and T. abyssorum mean MSRR increased only slightly with temperature for both species. Mean standardized MSRR of the Arctic T. libellula were higher than those of the boreal-Atlantic T. abyssorum at all temperatures (Table 1 and Figure 2C).
Q10 values were generally higher for boreal-Atlantic congeners than for polar-Arctic species (Figure 3). These differences in Q10 coefficients were most pronounced in Calanus species. Polar-Arctic C. hyperboreus and C. glacialis had Q10 values between 1.3 and 2.7. In contrast, the boreal-Atlantic C. finmarchicus had much higher Q10 values of 4.2 in C5s and 5.9 in adult females.
Figure 3 Q10 values of polar-Arctic (blue) versus boreal-Atlantic (red) zooplankton species based on exponential regression of mass-specific respiration rates at different temperatures (0, 4, 6, 8 and 10°C). C. hyp, Calanus hyperboreus; C. glac, Calanus glacialis; C. fin, Calanus finmarchicus; P. glac, Paraeuchaeta glacialis; P. norv, Paraeuchaeta norvegica; T. libel: Themisto libellula; T. abys, Themisto abyssorum; f, adult female; C5, copepodite stage C5.
Similar, but less pronounced differences in Q10 values between polar-Arctic and boreal-Atlantic sister species were determined for Paraeuchaeta spp. and Themisto spp., with higher values for the Atlantic species (P. norvegica C5: 2.3, females: 1.8; T. abyssorum: 2.5) compared to the Arctic congeners (P. glacialis C5: 1.0, females: 1.7; T. libellula: 1.7; Figure 3).
Mortality of the species was generally low (Table 1). In Calanus species, survival of Arctic C. hyperboreus C5s and females was exceptionally high, with 100% at temperatures from 0 to 10°C (Table 1). Survival of the Arctic congener C. glacialis was 100% at lower temperatures (0 to 4°C). At 6°C mortality of females rose to 50% (mind the low number of replicates). At 8°C mortality was below 10% in females and C5s, respectively. During one experimental run at simulated ambient temperatures of 12°C with C. hyperboreus (females, C5) and C. glacialis (C5) mortality of both Arctic species was 100%. In contrast, boreal-Atlantic C. finmarchicus females and C5 experienced a low mortality (≤5%) at all temperatures. At temperatures ≥4°C (females) and ≥8°C (C5) survival was 100%.
In Arctic Paraeuchaeta glacialis the mortality of females and C5s was low (<15%) at temperatures below 8 and 10°C, respectively. At 8°C mortality increased to 29% in females and at 10°C to 50% in C5s (mind low number of replicates). In boreal-Atlantic P. norvegica survival rates were high. Mortality was only observed at 8°C in C5s (17%).
In both Themisto species mortalities were only observed at 0°C with 36% for T. libellula and 9% for T. abyssorum (Table 1).
Between 1982 and 2017 the summer-mixed layer of the seasonally ice-free regions of the Arctic basin has warmed on average by 0.5°C per decade (Meredith et al., 2019), i.e. by more than 1.5°C in total. This warming trend is observable throughout various areas of the Arctic Ocean, e.g. in the warming of the Atlantic Water inflow (Beszczynska-Möller et al., 2012), in the northern Barents Sea (Lind et al., 2018) and even in the Deep Water of the Greenland Sea and Eurasian Basin (von Appen et al., 2015). With steadily increasing temperatures, it is expected that the Arctic community composition will shift towards an Atlantic regime (Chust et al., 2014; Fossheim et al., 2015). As polar zooplankton species are generally bigger and accumulate larger lipid stores (Kattner and Hagen, 2009), a shift in species composition towards smaller, less lipid-rich Atlantic congeners negatively affects food quality for higher trophic levels, with consequences for food-web structure and energy flux in the entire Arctic ecosystem (Møller and Nielsen, 2020). In the current study we discuss species-specific temperature tolerances and performance ranges of Arctic and co-occurring boreal-Atlantic zooplankton species to elucidate mechanisms causing the ongoing regime shifts.
During our experiments, all Arctic species generally survived up to rather high temperatures of 10°C. Polar zooplankton species showed a slow increase in their metabolic rates, also emphasized by low Q10 values, which is contradicting the expected pattern for typical stenotherm species, i.e. a rapid increase of respiration, followed by a narrow optimum and a sharp decline (high Q10, Pörtner et al., 2017). Hence, our first hypothesis of polar stenothermy in these Arctic species has to be rejected. At one experimental run conducted at 12°C, the mortality of Calanus glacialis and C. hyperboreus was high, suggesting that this temperature is close to their lethal limit. Similar or even higher (15°C) critical temperatures were reported for both Arctic Calanus species by Hirche (1987) for short-term non-feeding experiments with individuals from the Greenland Sea and Hildebrandt et al. (2014) for long-time feeding experiments with specimens from Fram Strait.
The hypothesis of polar stenothermy, i.e. the inability of polar species to cope with a broader temperature range in polar species, was mainly based on experiments with invertebrates and fishes from the Southern Ocean (Pörtner et al., 2007; Peck et al., 2010; Sandersfeld et al., 2015). In contrast to the Antarctic, the Arctic Ocean cold-water ecosystem is comparably young and much less isolated (Buchholz et al., 2012); thus, Arctic species may be subjected to a much higher variability of temperatures than their southern counterparts (Pörtner et al., 2007). During the present study, in situ water temperatures ranged from -1.8 up to 9.3°C for Arctic zooplankton species (Table 1), with higher abundances usually between -1.8 and 3.5°C. Distributional ranges of Arctic Calanus species extend from Norwegian fjords and the Gulf of Maine, USA, to the high Arctic. Hence, they cover a wide ambient temperature range from -2 to 8°C (Hirche and Niehoff, 1996; Niehoff and Hirche, 2005), suggesting robustness and adaptive mechanisms to cope with such variable temperatures.
Contradictory to our second hypothesis, larger Arctic Paraeuchaeta glacialis and Themisto libellula had higher MSRR at each temperature compared to their smaller Atlantic congeners P. norvegica and T. abyssorum, respectively (except Paraeuchaeta C5 at 8°C). For larger specimens, the experimental setup may not have been optimal, possibly due to increased stress in the relatively small incubation bottles. Closed-system incubations of larger specimens may also result in rapid depletion of dissolved oxygen concentrations with additional stress. To exclude that stressed large individuals were responsible for higher MSRR, we checked for species-specific correlation between dry mass and MSRR. For T. libellula, expected trends were observed, i.e. smaller individuals having higher MSRR than larger ones. Further, smaller-sized T. libellula with comparable dry mass to T. abyssorum generally had higher MSRR. Measured rates of T. libellula were within the reported range (Auel and Werner, 2003; Darnis et al., 2017). It is, thus, unlikely that the higher rates were due to handling stress and/or oxygen deficits.
Although further proof is necessary, our results suggest a metabolic adaptation to the cold environment in those polar-Arctic zooplankton species. This is indicated by oxygen consumption, and thus metabolic activity, being generally higher in species of Arctic Themisto and Paraeuchaeta compared to their Atlantic congeners (see Sandersfeld et al., 2017 for higher metabolic rates in Antarctic fish). The concept of metabolic cold adaptation postulates that polar ectotherms have elevated metabolic rates compared to temperate species, as metabolic costs are higher at low temperatures. It is, however, one of the more controversially discussed hypotheses in polar ecophysiology (Peck and Conway, 2000).
Aerobic respiration reflects an organism’s current demand of energy (Clarke, 2003). At 0°C, Arctic and Atlantic Calanus species showed the same ranges of mass-specific respiration rates (MSRR) in the present study, suggesting similar requirements. However, with increasing temperatures, the rise in MSRR differed significantly between Arctic and Atlantic Calanus species. While the MSRR of Arctic C. glacialis and C. hyperboreus showed only a slow increase (Q10 between 1.3 and 2.7), the MSRR of boreal-Atlantic C. finmarchicus increased much more rapidly (Q10 4.2 and 5.9).
A steep increase in respiration rates could be either stress-related or due to higher metabolic activity (e.g. growth, reproduction, digestion). C. finmarchicus is usually found in highest abundances in temperatures from 0 to 10°C (Bonnet et al., 2005; Strand et al., 2020), but occurrences in waters <0°C and ≥15°C have also been documented (Hirche, 1990; Turner et al., 1993; Bonnet et al., 2005). In situ temperatures for C. finmarchicus (0 to 9°C) during the present study were within the range of experimental temperatures. Absence of thermal stress is further supported by low mortality rates at high temperatures in the present and similar studies (Hirche et al., 1997). Physiological performances of C. finmarchicus at higher temperatures show that this boreal-Atlantic species is thriving with increasing temperatures. For instance, growth, egg production and feeding rates steadily increase with increasing temperatures (measurements from -1.5 to 15°C; Hirche et al., 1997; Campbell et al., 2001; Pasternak et al., 2013; Smolina et al., 2015), emphasizing a higher energy demand due to higher performance rates.
Adaptation to higher temperatures is further supported by gene regulation, as C. finmarchicus down-regulates proteins that are associated with thermal stress response at 5 and 10°C (Smolina et al., 2015), which indicates the absence of stress within this temperature range. In contrast to these performance experiments, specimens were not fed in the present study. Therefore, a stress-related increase in metabolic activity due to starvation cannot be completely ruled out. However, starvation generally did not last longer than a couple of days, suggesting that internal reserves could have been utilized to maintain a high metabolism. Especially C5 individuals had large lipid reserves due to the imminent diapause (mean lipid content >40% DM). To exclude that elevated metabolic rates in C. finmarchicus were stress-related at higher temperatures during starving conditions, additional studies are needed.
The weak response in MSRR of Arctic Calanus species suggests that they do not experience a distinct increase in their energy demand, neither for metabolic processes nor for a thermal stress response. Apparently, they cannot benefit from higher temperatures with an increase in their performance. The increase in respiration rates in C. glacialis and C. hyperboreus could solely be due to a passive response (Havird et al., 2020) and, thus, indicates a reduced sensitivity of metabolic processes to temperature. Indeed, our results are supported by a study on the genetic activity of fed C. glacialis, which showed no response in up- or down-regulation of protein expression with increasing temperatures from 0 to 10°C during short- and long-term experiments (Smolina et al., 2015).
Our results and the findings by Smolina et al. (2015) on genetic regulation in C. glacialis emphasize the absence of a thermal stress response in Arctic Calanus. For species inhabiting cold environments, several studies suggest that they accumulate heat shock proteins at a constant level to compensate for the damage associated with protein folding at low temperatures (Buckley et al., 2004; Place et al., 2004; Place and Hofmann, 2005; Huenerlage et al., 2016). This adaptation to cold temperatures results in a loss of thermal sensitivity of genes that encode such thermal stress proteins due to constantly high gene expression (Buckley et al., 2004).
Although respiration rates and genetic activity are not affected by rising temperatures in Arctic Calanus species, their physiological performances are reduced at higher temperatures. This has been shown for decreasing assimilation efficiency (experiments with C. glacialis up to 10°C; Grote et al., 2015), declining egg production rates (above 5°C for C. glacialis; Pasternak et al., 2013) and depression of gonad maturation (at 10°C for C. hyperboreus; Hildebrandt et al., 2014). The performance losses observed in Arctic Calanus species can be explained by a reduction of available energy associated with a decline in aerobic scope, as the pejus temperature is approached.
Hence, with increasing temperatures, Arctic zooplankton species may already reach their pejus temperature, while boreal-Atlantic congeners might still have sufficient energy available for their performance and fitness. High basal metabolic rates, as observed for boreal-Atlantic species in the current study, allow for a greater absolute aerobic scope, and thus a more active lifestyle (Clarke, 2003). Temperatures around 5 to 6°C apparently mark a threshold at which C. finmarchicus may outcompete its Arctic congeners (Henriksen et al., 2012; Kjellerup et al., 2012; Jung-Madsen and Nielsen, 2015). This is in line with our results of a much higher increase in metabolic activity at 4 to 6°C in C. finmarchicus than in the Arctic Calanus species. Thus, the Atlantification of Arctic zooplankton communities might be driven rather by ecological interactions, biological fitness and reproductive potential at higher ambient temperatures than by absolute physiological temperature limits, stenothermy and lower resilience against rising temperatures.
In conclusion, our third hypothesis, stating that the main driving force of the Atlantification would be physiological limits of Arctic species owing to increasing temperatures, has to be rejected. Additional experiments are necessary to exclude that those higher metabolic rates of Atlantic species were a stress response and long-term monitoring of community dynamics in regions of co-occurrence are essential to observe the consequences of different metabolic adaptations.
In the current study, we discussed direct physiological responses of Arctic versus boreal-Atlantic zooplankton species to rising temperatures, suggesting a higher competitive ability of Atlantic species with increasing temperatures due to higher metabolic activity. Yet, the Arctic Ocean is a harsh environment, which requires efficient adaptation. For instance, Arctic Calanus, especially C. hyperboreus, are able to start reproducing early in the year before sea-ice break-up, prior to the onset of a phytoplankton bloom, relying on internal energy reserves (Daase et al., 2021). Arctic Calanus species therefore have a competitive advantage over boreal C. finmarchicus in regions with strongly pulsed primary production regimes (Hatlebakk et al., 2022a, Hatlebakk et al., 2022b).
However, due to climate change, environmental conditions in the Arctic Ocean are shifting more and more to boreal regimes. The earlier sea-ice retreat and associated earlier phytoplankton production may result in increasing habitat suitability for boreal species and may further facilitate the successful establishment of boreal-Atlantic zooplankton populations in the Arctic Ocean (Freer et al., 2021). Indeed, Tarling et al. (2022) found first evidence of locally recruiting C. finmarchicus in Fram Strait, indicating that the system is becoming more suitable for boreal species.
At the same time, recent studies show that the shrinking of sea-ice cover results in a northward shift in the core distribution of C. hyperboreus and C. glacialis (Ershova et al., 2021) and in a mismatch between ice algae production and Calanus recruitment (Dezutter et al., 2019), adding to the factors that possibly decrease the competitive ability of Arctic zooplankton species.
A future Arctic ecosystem comprising boreal zooplankton communities with higher Q10 values may result in a system of higher metabolic activity, and thus a shift to a regime with higher turnover rates (Renaud et al., 2018). Individual size and lipid content of zooplankton species may generally become smaller and life cycles shorter, however, due to the faster growth and higher reproduction rates of boreal species, the future Arctic may be more efficient (Renaud et al., 2018). Yet, in a future Arctic scenario, highly prey-selective predators, such as little auks or young polar cods, will have to adapt to smaller and less energy-rich prey, or they will perish.
The present study demonstrates that, besides physiological effects, knowledge about complex ecological interactions is essential for a better understanding of global change implications on marine communities and ecosystems. These interactions should be included in models to develop realistic and reliable predictive capacities. This is especially important in the Arctic, where the Atlantification of the Arctic fauna is accompanied by the strong alternation of the environment. The loss of sea-ice and associated ice algal primary production results in drastic changes in habitat suitability, plankton phenology and overall productivity, affecting the entire Arctic marine ecosystem.
The original contributions presented in the study are included in the article/Supplementary Material. Further inquiries can be directed to the corresponding author.
HA and PK conducted the fieldwork. PK analyzed the samples and conducted the data analyses. PK drafted the manuscript. PK, WH, MB-D, and HA significantly contributed to improving the final manuscript. All authors contributed to the article and approved the submitted version.
Ship time was provided under grants AWI-PS100_07, AWI_PS107_10 and AWI_PS121_05, respectively.
We would like to thank the captains and crews of RV Polarstern during PS100, PS107 and PS121 for their skillful support during the cruises.
The authors declare that the research was conducted in the absence of any commercial or financial relationships that could be construed as a potential conflict of interest.
All claims expressed in this article are solely those of the authors and do not necessarily represent those of their affiliated organizations, or those of the publisher, the editors and the reviewers. Any product that may be evaluated in this article, or claim that may be made by its manufacturer, is not guaranteed or endorsed by the publisher.
The Supplementary Material for this article can be found online at: https://www.frontiersin.org/articles/10.3389/fmars.2022.908638/full#supplementary-material
Auel H., Hagen W. (2002). Mesozooplankton community structure, abundance and biomass in the central Arctic Ocean. Mar. Biol. 140, 1013–1021. doi: 10.1007/s00227-001-0775-4
Auel H., Werner I. (2003). Feeding, respiration and life history of the hyperiid amphipod Themisto libellula in the Arctic marginal ice zone of the Greenland Sea. J. Exp. Mar. Biol. Ecol. 269, 183–197. doi: 10.1016/S0022-0981(03)00321-6
Beaugrand G., Luczak C., Edwards M. (2009). Rapid biogeographic plankton shifts in the North Atlantic Ocean. Glob. Change Biol. 15, 1790–1803. doi: 10.1111/j.1365-2486.2009.01848.x
Beaugrand G., Reid P. C., Ibañez F., Lindley J. A., Edwards M. (2002). Reorganization of North Atlantic marine copepod biodiversity and climate. Science 296, 1692–1694. doi: 10.1126/science.1071329
Bekryaev R. V., Polyakov I. V., Alexeev V. A. (2010). Role of polar amplification in long-term surface air temperature variations and modern Arctic warming. J. Clim. 23, 3888–3906. doi: 10.1175/2010JCLI3297.1
Beszczynska-Möller A., Fahrbach E., Schauer U., Hansen E. (2012). ariability in Atlantic water temperature and transport at the entrance to the Arctic Ocean 1997 – 2010. ICES J. Mar. Sci. 69, 852–863. doi: 10.1093/icesjms/fss056
Bonnet D., Richardson A., Harris R., Hirst A., Beaugrand G., Edwards M., et al. (2005). An overview of Calanus helgolandicus ecology in European waters. Prog. Oceanogr. 65, 1–53. doi: 10.1016/j.pocean.2005.02.002
Bouchard C., Fortier L. (2020). The importance of Calanus glacialis for the feeding success of young polar cod: A circumpolar synthesis. Polar Biol. 43, 1095–1107. doi: 10.1007/s00300-020-02643-0
Buchholz F., Werner T., Buchholz C. (2012). First observation of krill spawning in the high Arctic Kongsfjorden, West Spitsbergen. Polar Biol. 35, 1273–1279. doi: 10.1007/s00300-012-1186-3
Buckley B. A., Place S. P., Hofmann G. E. (2004). Regulation of heat shock genes in isolated hepatocytes from an Antarctic fish, Trematomus bernacchii. J. Exp. Biol. 207, 3649–3656. doi: 10.1242/jeb.01219
Campbell R. G., Wagner M. W., Teegarden G. J., Boudreau C. A., Durbin E. G. (2001). Growth and development rates of the copepod Calanus finmarchicus reared in the laboratory. Mar. Ecol. Prog. Ser. 221, 161–183. doi: 10.3354/meps221161
Choquet M., Kosobokova K., Kwaśniewski S., Hatlebakk M., Dhanasiri A. K. S., Melle W., et al. (2018). Can morphology reliably distinguish between the copepods Calanus finmarchicus and C. glacialis, or is DNA the only way? Limnol. Oceanogr. Methods 16, 237–252. doi: 10.1002/lom3.10240
Chust G., Castellani C., Licandro P., Ibaibarriaga L., Sagarminaga Y., Irigoien X. (2014). Are Calanus spp. shifting poleward in the North Atlantic? A habitat modelling approach. ICES J. Mar. Sci. 71, 241–253. doi: 10.1093/icesjms/fst147
Clarke A. (2003). Costs and consequences of evolutionary temperature adaptation. Trends Ecol. Evol. 18, 573–581. doi: 10.1016/j.tree.2003.08.007
Daase M., Kosobokova K., Last K. S., Cohen J. H., Choquet M., Hatlebakk M., et al. (2018). New insights into the biology of Calanus spp. (Copepoda) males in the Arctic Ocean. Mar. Ecol. Prog. Ser. 607, 53–69. doi: 10.3354/meps12788
Daase M., Berge J., Søreide J. E., and Falk-Petersen S. (2021). “Ecology of Arctic Pelagic Communities” in Arctic Ecology, (Ed) Thomas D.N., John Wiley & Sons, New Jersey, USA, pp. 219–259. doi: 10.1002/9781118846582.ch9
Darnis G., Hobbs L., Geoffroy M., Grenvald J. C., Renaud P. E., Berge J., et al. (2017). From polar night to midnight sun: Diel vertical migration, metabolism and biogeochemical role of zooplankton in a high Arctic fjord (Kongsfjorden, Svalbard). Limnol. Oceanogr. 62, 1586–1605. doi: 10.1002/lno.10519
Dezutter T., Lalande C., Dufresne C., Darnis G., Fortier L. (2019). Mismatch between microalgae and herbivorous copepods due to the record sea ice minimum extent of 2012 and the late sea ice break-up of 2013 in the Beaufort Sea. Prog. Oceanogr. 173, 66–77. doi: 10.1016/j.pocean.2019.02.008
Ershova E. A., Kosobokova K. N., Banas N. S., Ellingsen I., Niehoff B., Hildebrandt N., et al. (2021). Sea Ice decline drives biogeographical shifts of key Calanus species in the central Arctic Ocean. Glob. Change Biol. 27, 2128–2143. doi: 10.1111/gcb.15562
Fossheim M., Primicerio R., Johannesen E., Ingvaldsen R. B., Aschan M. M., Dolgov V. (2015). Recent warming leads to rapid borealization of fish communities in the Arctic. Nat. Clim. Change 5, 673–677. doi: 10.1038/NCLIMATE2647
Franklin C. E., Farrell A. P., Altimiras J., Axelsson M. (2013). Thermal dependence of cardiac function in arctic fish: Implications of a warming world. J. Exp. Biol. 216, 4251–4255. doi: 10.1242/jeb.087130
Freer J. J., Daase M., Tarling G. A. (2021). Modelling the biogeographic boundary shift of Calanus finmarchicus reveals drivers of Arctic Atlantification by subarctic zooplankton. Glob. Change Biol. 28, 429–440. doi: 10.1111/gcb.15937
Gabrielsen T. M., Merkel B., Søreide J. E., Johansson-Karlsson E., Bailey A., Vogedes D., et al. (2012). Potential misidentifications of two climate indicator species of the marine arctic ecosystem: Calanus glacialis and C. finmarchicus. Polar Biol. 35, 1621–1628. doi: 10.1007/s00300-012-1202-7
Grote U., Pasternak A., Arashkevich E., Halvorsen E., Nikishina A. (2015). Thermal response of ingestion and egestion rates in the Arctic copepod Calanus glacialis and possible metabolic consequences in a warming ocean. Polar Biol. 38, 1025–1033. doi: 10.1007/s00300-015-1664-5
Hartmann D. L., Klein Tank A. M. G., Rusticucci M., Alexander L. V., Brönnimann S., Charabi Y., et al. (2013). “Observations: Atmosphere and surface,” in Climate change 2013: The physical science basis. Contribution of Working Group I to the Fifth Assessment Report of the Intergovernmental Panel on Climate Change (Cambridge, United Kingdom and New York, NY, USA: Cambridge University Press), 97 pp.
Hatlebakk M., Kosobokova K., Daase M., Søreide J. E. (2022a). Contrasting life traits of sympatric Calanus glacialis and C. finmarchicus in a warming Arctic revealed by a year-round study in Isfjorden, Svalbard. Front. Mar. Sci. 9. doi: 10.3389/fmars.2022.877910
Hatlebakk M., Niehoff B., Choquet M., Hop H., Wold A., Hoarau G., et al. (2022b). Seasonal enzyme activities of sympatric Calanus glacialis and C. finmarchicus in the high-Arctic. Front. Mar. Sci. 9. doi: 10.3389/fmars.2022.877904
Hattermann T., Isachsen P. E., von Appen W. J., Albretsen J., Sundfjord A. (2016). Eddy-driven recirculation of Atlantic water in Fram Strait. Geophys. Res. Lett. 43, 3406–3414. doi: 10.1002/2016GL068323
Havird J. C., Neuwald J. L., Shah A. A., Mauro A., Marshall C. A., Ghalambor C. K. (2020). Distinguishing between active plasticity due to thermal acclimation and passive plasticity due to Q10 effects: Why methodology matters. Funct. Ecol. 34, 1015–1028. doi: 10.1111/1365-2435.13534
Henriksen M. V., Jung-Madsen S., Nielsen T. G., Møller E. F., Henriksen K. V., Markager S., et al. (2012). Effects of temperature and food availability on feeding and egg production of Calanus hyperboreus from Disko Bay, western Greenland. Mar. Ecol. Prog. Ser. 447, 109–126. doi: 10.3354/meps09421
Hildebrandt N., Niehoff B., Sartoris F. J. (2014). Long-term effects of elevated CO2 and temperature on the Arctic calanoid copepod Calanus glacialis and C. hyperboreus. Mar Pollut. Bull. 80, 59–70. doi: 10.1016/j.marpolbul.2014.01.050
Hirche H. J. (1987). Temperature and plankton. II. Effect on respiration and swimming activity in copepods from the Greenland Sea. Mar. Biol. 94, 347–356. doi: 10.1007/BF00428240
Hirche H. J. (1990). Egg production of Calanus finmarchicus at low temperature. Mar. Biol. 106, 53–58. doi; 10.1007/BF02114674
Hirche H. J., Kattner G. (1993). Egg production and lipid content of Calanus glacialis in spring: Indication of a food-dependent and food-independent reproductive mode. Mar. Biol. 117, 615–622. doi: 10.1007/BF00349773
Hirche H. J., Meyer U., Niehoff B. (1997). Egg production of Calanus finmarchicus: effect of temperature, food and season. Mar. Biol. 127, 609–620. doi. 10.1007/s002270050051
Hirche H. J., Niehoff B. (1996). Reproduction of the Arctic copepod Calanus hyperboreus in the Greenland Sea - field and laboratory observations. Polar Biol. 16, 209–219. doi: 10.1007/BF02329209
Huenerlage K., Buchholz F. (2015). Thermal limits of krill species from the high-Arctic Kongsfjord (Spitsbergen). Mar. Ecol. Prog. Ser. 535, 89–98. doi: 10.3354/meps11408
Huenerlage K., Cascella K., Corre E., Toomey L., Lee C. Y., Buchholz F., et al. (2016). Responses of the arcto-boreal krill species Thysanoessa inermis to variations in water temperature: Coupling Hsp70 isoform expression with metabolism. Cell Stress Chaperones 21, 969–981. doi: 10.1007/s12192-016-0720-6
Jung-Madsen S., Nielsen T. G. (2015). Early development of Calanus glacialis and C. finmarchicus. Limnol. Oceanogr. 60, 934–946. doi: 10.1002/lno.10070
Jung-Madsen S., Nielsen T. G., Grønkjær P., Hansen B. W., Møller E. F. (2013). Early development of Calanus hyperboreus nauplii: Response to a changing Ocean. Limnol. Oceanogr. 58, 2109–2121. doi: 10.4319/lo.2013.58.6.2109
Kaiser P., Hagen W., von Appen W. J., Niehoff N., Hildebrandt N., Auel H. (2021). Effects of a submesoscale oceanographic filament on zooplankton dynamics in the Arctic marginal ice zone. Front. Mar. Sci. 8. doi: 10.3389/fmars.2021.625395
Kanzow T. (2017). The Expedition PS100 of the Research Vessel POLARSTERN to the Fram Strait in 2016. Rep. Polar Mar. Res. 705, 1–175. doi: 10.2312/BzPM_0717_2018
Karnovsky N., Harding A., Walkusz W., Kwaśniewski S., Goszczko I., Wiktor J., et al. (2010). Foraging distributions of little auks Alle alle across the Greenland Sea: implications of present and future Arctic climate change. Mar. Ecol. Prog. Ser. 415, 283–293. doi: 10.3354/meps08749
Kattner G., Hagen W. (2009). “Lipids in marine copepods: Latitudinal characteristics and perspective to global warming, p. 257-280,” in Lipids in aquatic ecosystems. Eds. Arts M. T., Brett M., Kainz M. (Berlin: Springer), 377 p.
Kawasaki T., Hasumi H. (2016). The inflow of Atlantic water at the Fram Strait and its interannual variability. J. Geophys. Res. Oceans 121, 502–519. doi: 10.1002/2015JC011375
Kjellerup S., Dünweber M., Swalethorp R., Nielsen T. G., Møller E. F., Markager S., et al. (2012). Effects of a future warmer ocean on the coexisting copepods Calanus finmarchicus and C. glacialis in Disko Bay, western Greenland. Mar. Ecol. Prog. Ser. 447, 87–108. doi: 10.3354/meps09551
Kosobokova K. N., Hirche H. J. (2009). Biomass of zooplankton in the eastern Arctic Ocean – a base line study. Prog. Oceanogr. 82, 265–280. doi: 10.1016/j.pocean.2009.07.006
Kraft A., Nöthig E. M., Bauerfeind E., Wildish D. J., Pohle G. W., Bathmann U. V., et al. (2013). First evidence of reproductive success in a southern invader indicates possible community shifts among Arctic zooplankton. Mar. Ecol. Prog. Ser. 493, 291–296. doi: 10.3354/meps10507
Kwaśniewski S., Gluchowska M., Jakubas D., Wojczulanis-Jakubas K., Walkusz W., Karnovsky N., et al. (2010). The impact of different hydrographic conditions and zooplankton communities on provisioning little auks along the West coast of Spitsbergen. Prog. Oceanogr. 87, 72–82. doi: 10.1016/j.pocean.2010.06.004
Lind S., Ingvaldsen R. B., Furevik T. (2018). Arctic warming hotspot in the northern Barents Sea linked to declining sea-ice import. Nat. Clim. Change 8, 634–639. doi: 10.1038/s41558-018-0205-y
Mańko M. K., Gluchowska M., Weydmann-Zwolicka A. (2020). Footprints of Atlantification in the vertical distribution and diversity of gelatinous zooplankton in the Fram Strait (Arctic Ocean). Prog. Oceanogr. 189, 102414. doi: 10.1016/j.pocean.2020.102414
Masson-Delmotte V., Schulz M., Abe-Ouchi A., Beer J., Ganopolski A., González Rouco J. F., et al. (2013). “Information from paleoclimate archives,” in Climate change 2013: The physical science basis. Contribution of Working Group I to the Fifth Assessment Report of the Intergovernmental Panel on Climate Change (Cambridge, United Kingdom and New York, NY, USA: Cambridge University Press), 82 p.
Meredith M., Sommerkorn M., Cassotta S., Derksen C., Ekaykin A., Hollowed A., et al. (2019). “Polar regions,” in IPCC Special Reports on the Ocean and Cryosphere in a Changing Climate. Eds. Pörtner H. O., Roberts D. C., Masson-Delmotte V., Zhai P., Tignor M., Poloczanska E., Mintenbeck K., Alegría A., Nicolai M., Okem A., Petzold J., Rama B., Weyer N. M. (Geneva: IPCC, Intergovernmental Panel on Climate Change), 203–320.
Metfies K. (2020). “The expedition PS121 of the research vessel POLARSTERN to the Fram Strait in 2019” in Rep. Polar Mar. Res, vol. 738, 95. doi: 10.2312/BzPM_0738_2020
Møller E. F., Nielsen T. G. (2020). Borealization of Arctic zooplankton – smaller and less fat zooplankton species in Disko Bay, Western Greenland. Limnol. Oceanogr. 65, 1175–1188. doi: 10.1002/lno.11380
Mumm N., Auel H., Hanssen H., Hagen W., Richter C., Hirche H. J. (1998). Breaking the ice: Large-scale distribution of mesozooplankton after a decade of Arctic and transpolar cruises. Polar Biol. 20, 189–197. doi: 10.1007/s003000050295
Niehoff B., Hirche H. J. (2005). Reproduction of Calanus glacialis in the Lurefjord (western Norway): indication for temperature-induced female dormancy. Mar. Ecol. Prog. Ser. 285, 107–115. doi: 10.3354/meps285107
Pasternak A. F., Arashkevich E. G., Grothe U., Nikishina A. B., Solovyev K. A. (2013). Different effects of increased water temperature on egg production of Calanus finmarchicus and C. glacialis. Oceanol. 53, 547–553. doi: 10.1134/S0001437013040085
Peck L. S. (2002). Ecophysiology of Antarctic marine ectotherms: Limits to life. Polar Biol. 25, 31–40. doi: 10.1007/s003000100308
Peck L. S., Conway L. Z. (2000). The myth of metabolic cold adaptation: oxygen consumption in stenothermal Antarctic bivalves. Geol. Soc Spec. Pub. 177, 441–450. doi: 10.1144/GSL.SP.2000.177.01.29
Peck L. S., Morley S. A., Clark M. S. (2010). Poor acclimation capacities in Antarctic marine ectotherms. Mar. Biol. 157, 2051–2059. doi: 10.1007/s00227-010-1473-x
Place S. P., Hofmann G. E. (2005). Constitutive expression of a stress-inducible heat shock protein gene, hsp70, in phylogenetically distant Antarctic fish. Polar Biol. 28, 261–267. doi: 10.1007/s00300-004-0697-y
Place S. P., Zippay M. L., Hofmann G. E. (2004). Constitutive roles for inducible genes: Evidence for the alteration in expression of the inducible hsp70 gene in Antarctic notothenioid fishes. Am. J. Physiol. Regul. Integr. Comp. Physiol. 287, R249–R436. doi: 10.1152/ajpregu.00223.2004
Polyakov I. V., Bhatt U. S., Walsh J. E., Abrahamsen E. P., Pnyushkov A. V., Wassmann P. F. (2013). RecentOceanic changes in the Arctic in the context of long-term observations. Ecol. Appl. 23, 1745–1764. doi: 10.1890/11-0902.1
Polyakov I. V., Pnyushkov A. V., Alkire M. B., Ashik I. M., Baumann T. M., Carmack E. C., et al. (2017). Greater role for Atlantic inflow on sea-ice loss in the Eurasian Basin of the Arctic Ocean. Science 356, 285–291. doi: 10.1126/science.aai8204
Polyakov I. V., Pnyushkov A. V., Timokhov L. A. (2012). Warming of the intermediate Atlantic water of the Arctic Ocean in the 2000s. J. Clim. 25, 8362–8370. doi: 10.1175/JCLI-D-12-00266.1
Pörtner H. O. (2001). Climate change and temperature-dependent biogeography: Oxygen limitation of thermal tolerance in animals. Naturwissenschaften 88, 137–146. doi: 10.1007/s001140100216
Pörtner H. O., Bock C., Mark F. C. (2017). Oxygen- and capacity-limited thermal tolerance: bridging ecology and physiology. J. Exp. Biol. 220, 2685–2696. doi: 10.1242/jeb.134585
Pörtner H. O., Peck L., Somero G. (2007). Thermal limits and adaptation in marine Antarctic ectotherms: An integrative view. Phil. Trans. Soc B 362, 2233–2258. doi: 10.1098/rstb.2006.1947
Renaud P. E., Daase M., Banas N. S., Gabrielsen T. M., Søreide J. E., Varpe Ø., et al. (2018). Pelagic food-webs in a changing Arctic: A trait-based perspective suggests a mode of resilience. ICES J. Mar. Sci. 75, 1871–1881. doi: 10.1093/icesjms/fsy063
Sandersfeld T., Davison W., Lamare M. D., Knust R., Richter C. (2015). Elevated temperature causes metabolic trade-offs at the whole-organism level in the Antarctic fish Trematomus bernacchii. J. Exp. Biol. 218, 2373–2381. doi: 10.1242/jeb.122804
Sandersfeld T., Mark F. C., Knust R. (2017). Temperature-dependent metabolism in Antarctic fish: Do habitat temperature conditions affect thermal tolerance ranges? Polar Biol. 40, 141–149. doi: 10.1007/s00300-016-1934-x
Schewe I. (2018). “The expedition PS107 of the research vessel POLARSTERN to the Fram Strait and the AWI-HAUSGARTEN in 2017” in Rep. Polar Mar. Res. 717, 120. doi: 10.2312/BzPM_0717_2018
Schulte P. M., Healy T. M., Fangue N. A. (2011). Thermal performance curves, phenotypic plasticity, and the time scales of temperature exposure. Integr. Comp. Biol. 51, 691–702. doi: 10.1093/icb/icr097
Smolina I., Kollias S., Møller E. F., Lindeque P., Sundaram A. Y. M., Fernandes J. M. O., et al. (2015). Contrasting transcriptome response to thermal stress in two key zooplankton species, Calanus finmarchicus and C. Glacialis. Mar. Ecol. Prog. Ser. 534, 79–93. doi: 10.3354/meps11398
Strand E., Bagøien E., Edwards M., Broms C., Klevjer T. (2020). Spatial distribution and seasonality of four Calanus species in the Northeast Atlantic. Prog. Oceanogr. 185, 102344. doi: 10.1016/j.pocean.2020.102344
Tarling G. A., Freer J. J., Banas N. S., Belcher A., Blackwell M., Castellani C., et al. (2022). Can a key boreal Calanus copepod species now complete its life-cycle in the Arctic? Evidence and implications for Arctic food-webs. Ambio 51, 333–344. doi: 10.1007/s13280-021-01667-y
Turner J. T., Tester P. A., Strickler J. R. (1993). Zooplankton feeding ecology: A cinematographic study of animal-to-animal variability in the feeding behavior of Calanus finmarchicus. Limnol. Oceanogr. 38, 255–264. doi: 10.4319/lo.1993.38.2.0255
Verberk W. C. E. P., Overgaard J., Ern R., Bayley M., Wang T., Boardman L., et al. (2016). Does oxygen limit thermal tolerance in arthropods? A critical review of current evidence. Comp. Biochem. Physiol. Part A 192, 64–78. doi: 10.1016/j.cbpa.2015.10.020
Vihtakari M., Welcker J., Moe B., Chastel O., Tartu S., Hop H., et al. (2018). Black-legged kittiwakes as messengers of Atlantification in the Arctic. Sci. Rep. 8, 1178. doi: 10.1038/s41598-017-19118-8
von Appen W. J., Schauer U., Somavilla R., Bauerfeind E., Beszczynska-Möller A. (2015). Exchange of warming deep waters across Fram Strait. Deep-Sea Res. I 103, 86–100. doi: 10.1016/j.dsr.2015.06.003
von Appen W. J., Wekerle C., Hehemann L., Schourup-Kristensen V., Konrad C., Iversen M. H. (2018). Observations of a submesoscale cyclonic filament in the marginal ice zone. Geophys. Res. Lett. 45, 6141–6149. doi: 10.1029/2018GL077897
Wassmann P., Kosobokova K. N., Slagstad D., Drinkwater K. F., Hopcroft R. R., Moore S. E., et al. (2015). The contiguous domains of Arctic Ocean advection: Trails of life and death. Prog. Oceanogr. 139, 42–65. doi: 10.1016/j.pocean.2015.06.011
Weydmann A., Carstensen J., Goszczko I., Dmoch K., Olszewska A., Kwasniewski S. (2014). Shift towards the dominance of boreal species in the Arctic: inter-annual and spatial zooplankton variability in the West Spitsbergen Current. Mar. Ecol. Prog. Ser. 501, 41–52. doi: 10.3354/meps10694
Keywords: Calanus, respiration, aerobic metabolism, cold adaptation, climate change, Fram Strait, Atlantification, Borealization
Citation: Kaiser P, Hagen W, Bode-Dalby M and Auel H (2022) Tolerant but facing increased competition: Arctic zooplankton versus Atlantic invaders in a warming ocean. Front. Mar. Sci. 9:908638. doi: 10.3389/fmars.2022.908638
Received: 30 March 2022; Accepted: 30 June 2022;
Published: 12 August 2022.
Edited by:
Malin Daase, UiT The Arctic University of Norway, NorwayReviewed by:
Gerald Francis Darnis, Laval University, CanadaCopyright © 2022 Kaiser, Hagen, Bode-Dalby and Auel. This is an open-access article distributed under the terms of the Creative Commons Attribution License (CC BY). The use, distribution or reproduction in other forums is permitted, provided the original author(s) and the copyright owner(s) are credited and that the original publication in this journal is cited, in accordance with accepted academic practice. No use, distribution or reproduction is permitted which does not comply with these terms.
*Correspondence: Patricia Kaiser, cGF0cmljaWEua2Fpc2VyQHVuaS1icmVtZW4uZGU=
Disclaimer: All claims expressed in this article are solely those of the authors and do not necessarily represent those of their affiliated organizations, or those of the publisher, the editors and the reviewers. Any product that may be evaluated in this article or claim that may be made by its manufacturer is not guaranteed or endorsed by the publisher.
Research integrity at Frontiers
Learn more about the work of our research integrity team to safeguard the quality of each article we publish.