Corrigendum: The presence of silver nanoparticles reduces demand for dissolved phosphorus to the benefit of biological nitrogen fixation in the coastal eastern Mediterranean Sea
- 1Plymouth Marine Laboratory, Plymouth, United Kingdom
- 2School of Biological and Marine Sciences, University of Plymouth, Plymouth, United Kingdom
- 3Oceanography Division, Istituto Nazionale di Oceanografia e di Geofisica Sperimentale, Trieste, Italy
- 4Hellenic Centre for Marine Research, Institute of Marine Biology, Biotechnology and Aquaculture, Heraklion, Greece
- 5Hellenic Centre for Marine Research, Institute of Oceanography, Heraklion, Greece
The release of silver into the marine environment is of growing concern as its impact on marine life is not fully understood. Despite previous experiments that have shown toxic effects of silver as nanoparticles (AgNPs) and as free ions (Ag+) on microbial organisms, the impact on important biogeochemical processes, such as marine nitrogen fixation, remains relatively unexplored. The present study investigated the impact of AgNPs and Ag+ on nitrogen fixation activity in oligotrophic coastal ecosystems. Nine mesocosm enclosures were set-up in Crete, Greece, for twelve days during May 2019. Three mesocosms were left unamended at ambient light and temperature; three were manipulated with 50 ng AgNPs L-1d-1 and three were amended with 50 ng Ag+ L-1d-1. Over the duration of the experiment, mean nitrogen fixation rates proved higher in treated waters; 0.28 ± 0.24 nmol N L-1d-1 and 0.21 ± 0.19 nmol N L-1d-1 in AgNP and Ag+ which were 2.2 (p< 0.001) and 1.6 (not significant) times higher than control rates of 0.13 ± 0.07 nmol N L-1d-1 respectively. Changes in nitrogen fixation rates were paralleled by significantly higher concentrations of phosphate and silicate in treated versus control mesocosms, suggesting an inhibition of the uptake of these nutrients by non-diazotrophic groups. Sequencing of 16S and 18S rRNA genes showed shifts in community composition over time but only very minor changes could be linked to the Ag treatments. Decreases to the relative abundance of three diatom species were observed in the Ag treatments but these were considered insufficient to support the relative differences in nutrient concentration. It is therefore speculated that there was physiological disruption of nutrient uptake mechanisms of the non-diazotrophic community. A number of potential diazotrophs were detected and the relative sequence abundance of a number of known nitrogen fixing taxa, including Burkholderiaceae, Oceanospirillales and Pseudomonadales correlated with measured nitrogen fixation rates. Phosphorus limitation of diazotrophic activity was therefore lowered relative to other microbial groups in silver amended treatments and significant increases in particulate nitrogen for both Ag treatments indicate an enhancement in cellular N for the nitrogen fixing communities.
Introduction
As a result of their antibacterial properties, silver nanoparticles (AgNPs) are amongst the most commercialised nanomaterials. They are widely used for medical purposes, as consumer products for domestic cleaning, food packaging and processing, and within other applications in the energy, electronics and biotechnology sectors (Henryka and Krystyna, 2015; Guo et al., 2019). Engineered AgNPs are manufactured nanomaterials with at least one external dimension in the size range between 1 and 100 nm (Auffan et al., 2009) and their global production is estimated to be in the order of 320,000 tons per year (Nowack et al., 2011). Once released into the environment, AgNPs can undergo transformations that modify their properties; this in turn may alter their transport, fate, and toxicity (Levard et al., 2012).
Although silver nanoparticles are considered a relatively recent invention of nanotechnology, products containing nanoscale silver particles have been in use for more than 100 years and colloidal silver has been recorded since 1954 as a biocidal material in the United States (Nowack et al., 2011). The use of nanosilver is regulated internationally, but legislative controls vary between countries (Faunce and Watal, 2010). The consequences of AgNPs entering waterways are still partly unexplored, although there is significant evidence that AgNPs are toxic to both freshwater and marine biota (Navarro et al., 2008), and that their indiscriminate use may cause the development of microbial resistance to silver (Tsiola et al., 2018). Whilst small AgNPs dissolve more rapidly in natural waters, larger particles (>50 nm) can persist for an extended period of time, potentially representing a continuous source of Ag+ for the environment (Dobias and Bernier-Latmani, 2013), with concern that some AgNPs deposited into freshwater environments could persist for sufficient periods of time to successfully enter estuarine and marine systems (Chinnapongse et al., 2011).
The most documented effects of AgNPs on marine biota include the inhibition of bacterioplankton productivity, changes in microbial metabolic activity and reduced phytoplanktonic growth rates (Das et al., 2012; Das et al., 2014; Tsiola et al., 2017a; Tsiola et al., 2018). Recent studies show that exposure to AgNPs can alter nitrogen cycling processes such as nitrification (Beddow et al., 2017; Tsiola et al., 2018; McGee, 2020) and also the potential to influence nitrogen fixation (diazotrophy) though the effect is neither clear nor consistent (McGee, 2020). The marine nitrogen cycle is one of the most complex biogeochemical cycles in the sea and is driven by different processes and microbial transformations, which include nitrogen fixation, assimilation, nitrification and denitrification (Gruber, 2008). The most abundant form of nitrogen in the sea is dinitrogen (N2), which can only be accessed by nitrogen-fixing organisms (Voss et al., 2013). Nitrogen fixation is a critical process in the marine nitrogen cycle, by which dinitrogen is converted into ammonia and other nitrogenous compounds (Carpenter et al., 2008). Marine diazotrophy may therefore contribute to the regulation of other important biogeochemical processes, which could otherwise be limited by the availability of nitrogen (Gruber, 2016). In nutrient poor waters such as those found in the eastern Mediterranean Sea, diazotrophy offers a mechanism to make fixed (new) nitrogen available to microbial communities. Measurements to date for this area have proved somewhat enigmatic, though the consensus is that rates are generally low and limited by some, as yet, undetermined factor (Zeev et al., 2008). Diazotrophy occurs throughout the Mediterranean and, in reflection of decreasing nutrient levels, shows a similar trend to other conditions and processes (Mermex et al., 2011) with rates decreasing from west to east though there is some degree of variability in the rates that have been reported. Low N2 fixation rates of < 0.15 nmol L-1 d-1 have been recorded in open waters across both basins (e.g (Ibello et al., 2010; Ridame et al., 2011; Rahav et al., 2013). However, higher rates of N2 fixation have been reported for nutrient replete coastal environments in the western Mediterranean such as the plume of the River Rhone (1.80 ± 0.19 nmol L−1 d−1) (Bonnet et al., 2011), station DYFAMED within the Ligurian Sea (between 2 and 7.5 nmol L-1d-1) (Sandroni et al., 2007) and in Corsican coastal waters (2.0 ± 1.2 nmol L-1d-1) (Rees et al., 2017). Mediterranean diazotrophic communities have proved diverse, with studies showing archaea, cyanobacteria and Alpha- and Gamma Proteobacteria to be present (Man-Aharonovich et al., 2007; Ibello et al., 2010; Rahav et al., 2013; Benavides et al., 2016; Rees et al., 2017)
The limiting factor controlling the typically lower rates of nitrogen fixation in the Levantine Basin of the eastern Mediterranean is unclear but may result from phosphorus-deficient conditions affecting this area (Zeev et al., 2008). As a semi-enclosed, nutrient impoverished sea with dense urbanisations close to the coastal areas, the Mediterranean is very susceptible to anthropogenic driven influences (Mermex et al., 2011). Experimental evidence from mesocosm experiments has indicated that the addition of AgNPs can alter the function of some microbial processes (Tsiola et al., 2018) in this region, whilst evidence from in-vitro and non-marine ecosystems indicates that there is some evidence that both AgNPs and Ag+ ions might negatively impact diazotrophy (McGee, 2020). Investigation of the impacts of AgNPs on nitrogen cycling in marine systems appears to be very limited (Beddow et al., 2017) although the indications are that biological nitrogen fixation and the responsible diazotrophs are likely to be disrupted (Beddow et al., 2017; McGee, 2020). There is concern that introduction of Ag+ and AgNPs might further reduce rates of nitrogen fixation in oligotrophic waters such as those characterising the eastern Mediterranean Sea.
This study, which utilized a mesocosm experiment within the coastal waters of Crete in May 2019, aimed to investigate the effect of Ag+ and AgNPs addition on oligotrophic coastal ecosystems, specifically to test the hypothesis that the presence of Ag+ ions and AgNPs would inhibit biological nitrogen fixation activity. The study presented here aims to address the following questions:
1. How are nitrogen fixation rates affected by the addition of AgNPs and Ag+ in oligotrophic coastal ecosystems of the eastern Mediterranean?
2. Which form of silver is more likely to cause toxicity to the local microbial community between dissolved Ag+ and nanosized silver particles (AgNPs)?
3. How are nutrient concentrations relative to phosphate, particulate nitrogen, particulate phosphorus, nitrate and ammonium affected by the addition of AgNPs and Ag+?
4. Which microorganisms characterize the diazotrophic community living in the mesocosms, and how are they impacted by exposure to Ag+ and AgNPs?
Methods
In-situ experimental set-up
The experiment took place during May 2019 at the CRETACOSMOS facility of the Hellenic Centre for Marine Research (HCMR), Crete. Coastal seawater was collected from the Bay of Gournes (Figure 1), situated on the north-eastern coast of the island of Crete (Eastern Mediterranean). In-situ measurements using a Conductivity, Temperature, and Depth sensor (SBE 19plus V2 SeaCAT Profiler CTD) reported a salinity of 39.2 PSU. Seawater from the Bay of Gournes was collected at approximately 7 m depth and transported to the HCMR facilities where it was transferred into 9 polyethylene mesocosm enclosures (Supplementary Figure 1), each reaching a final volume of 3 m3, which were prepared as described in full by (Tsiola et al., 2017a; Tsiola et al., 2017b). Mesocosms were maintained at ambient light and temperature. Conditions in the mesocosms were allowed to stabilise for 24 hours, prior to the experiment starting on 11th May 2019 (day 0). Water samples were collected from each mesocosm prior to the addition of silver. Three mesocosms were left unamended as controls, over the ten day period of the experiment three mesocosm enclosures each received 50 ng Ag+ L-1 per day, reaching a total final concentration of ~500 ng Ag L-1, and the three remaining mesocosm bags each received 50 ng AgNPs L-1 per day. Silver nanoparticles were added in aqueous suspension as branched polyethyleneimine (BPEI) (60 nm diameter size, positive charge, BioPure).
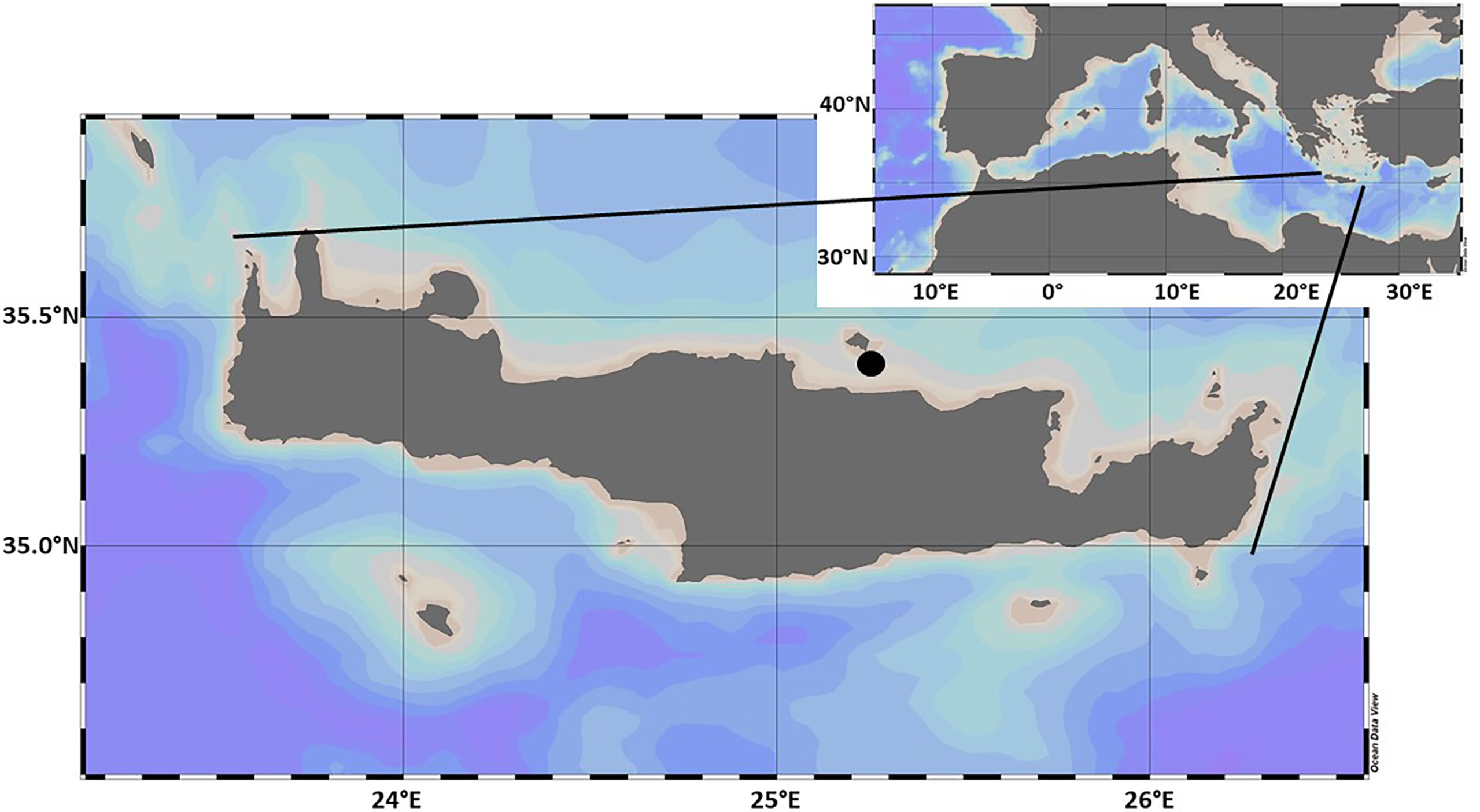
Figure 1 Study area showing the position of Crete in the Mediterranean Sea, and the sampling location (Filled black circle) where seawater was collected for the purpose of the SiNAME experiment (Bay of Gournes). Map created using Ocean Data View.
Nitrogen fixation
Seawater samples were collected every morning after sunrise from each mesocosm into 10 L low-density polyethylene (LDPE) bottles and returned to the HCMR laboratory where water from each was carefully transferred, to exclude air bubbles, into one of nine 2.4 L polycarbonate bottles (3 replicates for each treatment). Following methodological issues relating to addition of 15N-N2 bubble (Mohr et al., 2010; Groszkopf et al., 2012), 15N-N2 additions were made following the procedure of Chang et al. (2019). Bottles were sealed with septa fitted caps and each bottle was injected with 2.4 mL of 15N-N2 (98 atom%, Cambridge Isotope Laboratories Inc., Lot # I-22779IAR0720595) and gently agitated for approximately 20 minutes. Lids were then removed to allow bubble to escape and bottles filled to exclude air bubbles using seawater from the corresponding treatment before being re-sealed. Bottles were then transferred to the mesocosm tanks where they were incubated for 22 hours at approximately 1 m depth close to the mesocosm enclosures. Following incubation, bottles were vacuum filtered onto pre-combusted 25 mm GF-75 glass fibre filters (Advantec, pore size nominally 0.3 µm), which were then transferred into Petri dishes and dried at 50°C for 24 hours. The total volume filtered from each bottle was 2.4 L on day 0 and day 1 and then reduced to 1.9 L for the remaining duration of the experiment due to slow filtration time. Filters were stored at room temperature on silica gel until later analyses at Plymouth Marine Laboratory (PML). Particulate nitrogen (PN) and 15N atom% were measured using continuous-flow stable isotope mass-spectrometry [Sercon Ltd 20-22 and GSL (Owens and Rees, 1989)], with rates determined according to (Montoya et al., 1996). Instrument precision was better than 0.14% CV based on urea standards ((Iso-Analytical Ltd) in the range 0.25 – 2.0 µmol N, which were analysed during three sample runs of the mass spectrometer (mean ± 1 s.d. = 0.3663 atom% ± 0.0005, n=30). The mean particulate N content of samples was 0.66 ± 0.14 µmol L-1. The detection limit for N2 fixation rate was calculated from the determined 15N significant enrichment level and the lowest observed particulate nitrogen concentration (Ridame et al., 2013) and was estimated at 0.07 nmol L-1d-1.
DNA extraction
With the aim of investigating the diazotrophic community composition during the mesocosm experiment, additional seawater from each treatment was collected into 5 L bottles on day 0 and then on alternate days from day 1 until day 11. Seawater from each bottle was filtered onto Sterivex filters (0.22 μm Filter Unit, Millipore Corporation, Billerica, MA, USA) using a peristaltic pump. RNAlater Solution (0.7 mL; Ambion AM7020) was added to each cartridge filter, which was then sealed and stored at -20°C. Nucleic acid extraction was carried out at PML using the Qiagen AllPrep DNA/RNA Mini Kit 50. Although this kit also produces an RNA sample, only DNA samples were studied further. This process resulted in 40 µL of extracted DNA for each Sterivex filter (total of 25 samples), which were frozen at -80°C until subsequent analyses. The presence of DNA was verified using a NanoDrop 1000 v. 3.7.1 spectrophotometer (Thermo Scientific).
Quantitative PCR
To explore the presence and abundance of diazotrophic phylotypes considered likely to characterise this region, a range of previously published PCR primers and probes specific to the following phylotypes were selected for further analysis (Supplementary Table 1): unicellular cyanobacterial (UCYN) groups A1 (Church et al., 2005), A2 (Thompson et al., 2014), and B (Moisander et al., 2010), Trichodesmium sp (Church et al., 2005)., the cyanobacteria Richelia Het-1 and Het-2 (Moisander et al., 2008), and the proteobacterial phylotypes γ-Prot 24774A11 (Moisander et al., 2008), γETSP1, γETSP2 and γETSP3 (Turk-Kubo et al., 2014). Repeated attempts using a range of PCR conditions and protocols were made to amplify a PCR product using each PCR primer pair but this was only successful using the Richelia Het-2 PCR primers. However, when this PCR product was used as a standard in a test qPCR reaction using the probe described within Moisander et al. (2010), no fluorescent signal could be generated, indicating no binding of by the Richelia Het-2 specific probe occurred during the qPCR reaction. Together, this indicated that other unknown diazotrophs were responsible for N2 fixation within the mesocosms.
Gene sequencing
The decision was taken to investigate the wider microbial community identified by 16S and 18S genes rather than simply nifH in order to account for wider impacts on the microbial community that might explain potential changes in nutrients and other variables. High-throughput DNA sequencing was therefore performed by the NUomics sequencing service at Northumbria University (UK). 16S and 18S rRNA gene libraries for prokaryotes and eukaryotes respectively were prepared following protocol (Kozich et al., 2013) in which each fragment was composed by an Illumina adapter, followed by an index sequence (only for forward primer), a 10-nt pad to avoid formation of hairpin structures, linkers and the V4 region-specific forward or reverse primer. For 16S rRNA genes, the primers 515F (GTGYCAGCMGCCGCGGTAA) and 806R (GGACTACHVGGGTWTCTAAT) targeting the prokaryote V4 region were used (Caporaso et al., 2011). For 18S rRNA genes, 1391F (GTACACACCGCCCGTC) and EukBr (TGATCCTTCTGCAGGTTCACCTAC) targeting the microbial eukaryotic lineage V9 region (Thompson et al., 2017). Sequencing was performed on the MiSeq Personal Sequencer (Illumina, San Diego, CA, USA) using the V2 500 reagent kit. Demultiplexed paired end FASTQ files were analysed using QIIME2 (Bolyen et al., 2019) and amplicon sequence variants (ASVs) generated using DADA2 (Callahan et al., 2016). For 16S rRNA gene sequences, taxonomy was classified using the SILVA 132 reference database (Quast et al., 2013). Taxonomy for 18S rRNA gene sequences was assigned by comparison with the NCBI database. Taxonomic abundance data is expressed as percentage abundance enumerated from fractional abundances in sample libraries.
Nutrients
Triplicate seawater samples were taken from the Bay of Gournes prior to water being transported to the mesocosm facility and then on a daily basis from each of the mesocosm enclosures. Concentration of dissolved orthophosphate ( ) was determined using the MAGIC25 method (Rimmelin and Moutin, 2005), nitrate ( ) and silicate ( Si(OH)4 ) according to (Strickland and Parsons, 1972) and ammonium ( ) (Ivancic and Degobbis, 1984). Samples for the analysis of particulate organic phosphorous (POP) were collected onto 25mm GF/F filters and analysed according to (Aspila et al., 1976).
Alkaline phosphatase activity
Alkaline phosphatase activity (APA) was measured in the bulk seawater (total APA) and in the< 0.2 μm fraction (dissolved or cell-free APA). The enzymatic activity was assayed using the fluorogenic substrate analogue (Hoppe et al., 1993; Celussi et al., 2017) 4-methyl-umbelliferone (MUF)-phosphate (Sigma Aldrich®). APA was expressed in terms of MUF production rate, following the substrate’s cleavage. The substrate saturating concentrations were evaluated in samples collected at the sampling point in the Bay of Gournes 1 and 2 days before the beginning of the experiment. Briefly, samples were collected daily from all treatments and triplicate 2 mL subsamples were amended with 25 μM (final concentration) MUF-phosphate and incubated for 2 h in the dark at in situ temperature. Increase in fluorescence due to MUF hydrolysed from the model substrate was measured using a Shimadzu RF-1501 spectrofluorometer (365-nm excitation and 455-nm emission). Triplicate calibration curves were performed on each sampling day using 0.2 μm-filtered seawater from the mesocosms and a 5 μM MUF standard solution.
Statistical analyses
Statistical analyses were performed using RStudio v.1.2.1335. Data were visualised both in RStudio using the “ggplot2” package and using Microsoft Excel. The distribution of data was checked both through visual inspection (histograms and residual plots) and by performing the Shapiro-Wilk Normality Test, followed by the Bartlett Test of Homogeneity of Variances. When high levels of heteroscedasticity were present, non-parametric statistical tests were performed. Molecular data (16S and 18S rRNA gene sequences) were analysed using relative abundances, calculated by dividing the number of gene sequences from each taxon by the total number of sequences from all taxonomic groups. Full p-values have been stated when p>0.001, if smaller, they have been reported as p<0.001. A confidence level of 95% was applied to all statistical tests (α=0.05).
R (Team, 2019) and lme4 (Bates et al., 2014) were used to perform linear mixed effects analyses aiming to explore the combined effect of multiple variables on nitrogen fixation rates, incorporating random effects which might have represented a source of variability in the model. P-values were obtained by likelihood ratio test of the full model containing the effect of interest against a null model without the effect in question, as described by Winter (2013) (Winter, 2013). One-way analysis of variance (ANOVA) or its equivalent non-parametric test (Kruskal-Wallis Test) were performed to test for significant differences between treatments (C, AgNP, Ag+) for the following variables: nitrogen fixation rates, nutrient concentrations and alkaline phosphatase activity., relative 16S and 18S rRNA gene sequence abundance data (using different taxonomic levels). Post hoc Tukey tests (Tukey HSD) or Kruskalmc Multiple Comparison Pairwise Test were applied to determine which treatment significantly differed from the others. The level of correlation between nitrogen fixation activity and nutrient concentrations and alkaline phosphatase activity, and relative sequence abundance data respectively were tested by performing non-parametric Spearman’s rank correlation analyses since the assumptions of linear regression analysis were not met by the data. Further exploratory analyses were conducted using Principal Component Analysis (PCA) to test the level of correlation between nitrogen fixation rates and nutrient concentrations. ANOSIM tests (analysis of similarity) were used to explore the influence of treatment on the composition of bacterial communities throughout the duration of the mesocosm using time as an ordered variable and treatment as unordered variables and PERMANOVA tests were used to explore the influence of sampling day and treatment on the relative sequence abundance of taxa
Results
Nitrogen fixation
Background nitrogen fixation rates derived from measurements taken on samples collected directly from the Bay of Gournes on the 10th of May showed rates of 0.21 ± 0.08 nmol N L-1d-1. Rates within control treatments were lower than ambient rates but remained constant over the duration of the experiment (Figure 2) at 0.13 ± 0.07 nmol N L-1d-1, whilst elevated rates were observed in Ag+ and AgNP treatments. In AgNP treatments the daily mean rate increased after Day 2 to 0.37 ± 0.30 nmol N L-1d-1 and remained at a greater rate than the control (mean rate of 0.29 ± 0.23 nmol N L-1d-1) for the remainder of the experiment. Daily rates determined in the Ag+ treatments were variable, rising to daily mean maxima on days 4 and 6 of 0.35 ± 0.28 nmol N L-1d-1 and 0.33 ± 0.20 nmol N L-1d-1 respectively.
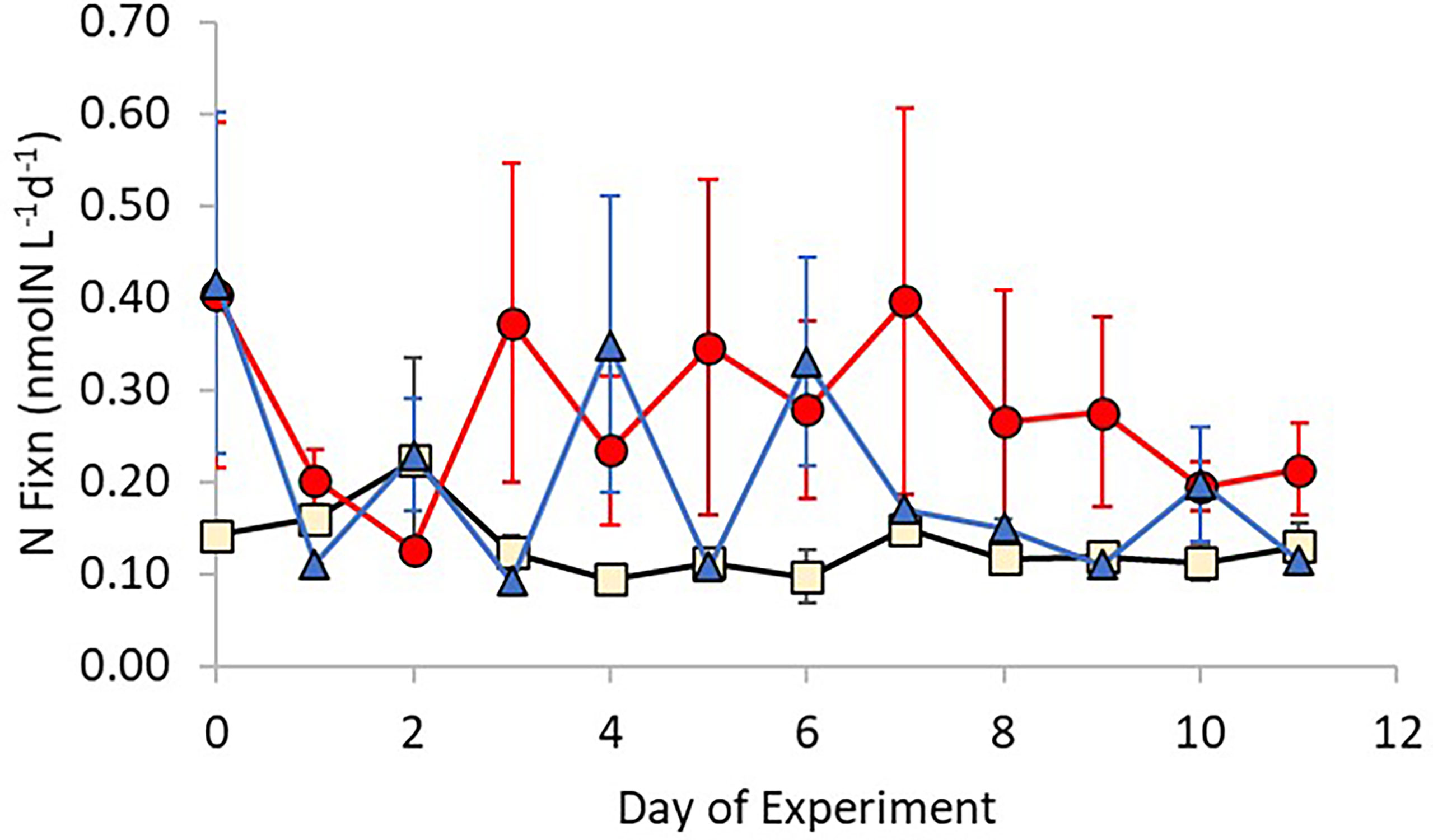
Figure 2 Changes in nitrogen fixation rates over time for each treatment (Control – Beige; +Ag+ – blue; +AgNP – red). Error bars show ± 1 standard deviation of the mean.
Linear mixed effects models were used to test the combined effect of treatment and time on nitrogen fixation activity, using “treatment” (i.e. AgNPs, Ag+, control mesocosms) and “time” (i.e. day of experiment) as fixed effects (both with and without an interaction term) and “mesocosm ID” (corresponding to each mesocosm number) as a random effect to account for potential variability between the nine mesocosms (e.g. possible differences in light or other abiotic factors). A likelihood ratio test of the full model containing the effect of “time” against a null model and a reduced (intercept) model without “time” as fixed effect did not produce significant statistical outputs ( x 2(11)=7.23, n=108, p=0.780). Hence, the model including “treatment” only as the fixed effect explained most of the variation in nitrogen fixation activity. To support this finding, we performed a two-way ANOVA incorporating “treatment” and “day of experiment” as explanatory variables for changes in nitrogen fixation rates. Results showed that with the exception of the first day of experiment, there were no differences between treatments on individual days (two-way ANOVA: F(11,89)=0.72, n=108, p>0.05).
Nitrogen fixation rates were variable between and within treatments and so non-parametric statistical tests were performed to investigate the relationship between rate and treatment. Nitrogen fixation rates were significantly different between treatments (Kruskal-Wallis test: x 2(2)=14.864, n=108, p<0.001), but further Kruskal Multiple Comparison Pairwise Test revealed that this was due to differences between the group of samples treated with AgNPs and the control group (p<0.05) (Figure 3A). Concentrations of PN also showed a significant difference between treatments (one-way ANOVA: F(2,102)=10.43, n=108, p<0.001). The groups treated with AgNP and Ag+ had higher particulate nitrogen concentrations than the control group, as reported by Tukey multiple comparisons of means test (p=0.018, p<0.001, respectively) (Figure 3B).
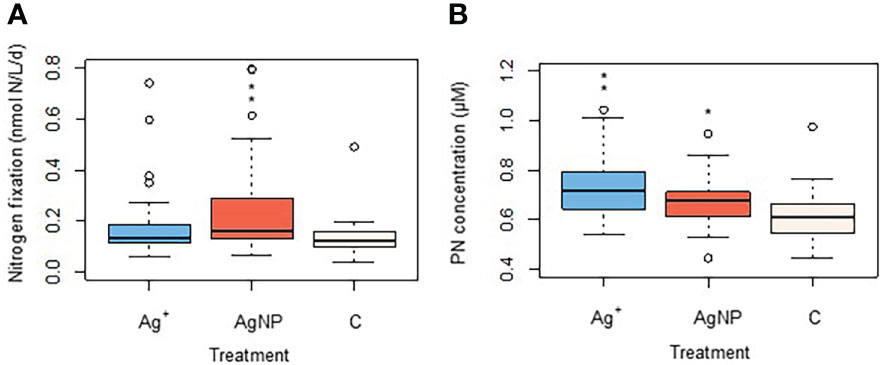
Figure 3 Changes in a) nitrogen fixation rates and b) in particulate nitrogen (PON) as an effect of Ag+ and AgNPs addition. Asterisks above boxplots show levels of significance: *indicates p<0.05, **indicates p<0.001).
Nutrients
Ambient nutrient analyses in the Bay of Gournes on 10th May revealed concentrations of 990 ± 30 nM , 430 ± 17 nM , 240 ± 11 nM and 9.5 ± 2.0 nM indicating a system severely limited by the availability of phosphate: : = 44.5 compared to the Redfield Ratio (Redfield et al., 1963) of ~16.1. Concentrations of decreased between Day 0 to Day 11 in all treatment mesocosms (Figure 4A) from 173 ± 25 nM, 297 ± 118 nM and 233 ± 39 nM to 40 ± 8 nM, 13 ± 13 nM and 37 ± 39 nM in Control, AgNP and Ag+ treatments respectively. Despite some initial variability over days 0 to 2, all treatment mesocosms had similar concentrations and there was no significant difference between them (Kruskal-Wallis test: x 2(2)=0.067,n=108, p=0.967). Ammonium concentrations were stable throughout the experiment in all treatments at 33.0 ± 21.5 nM, 28.2 ± 18.3 nM and 25.5 ± 20.0 nM between Day 1 and Day 11 for Control, AgNP and Ag+ respectively (Supplementary Figure 2), with no significant difference between treatments (Kruskal-Wallis test: x 2(2)=0.415, n=108, p=0.812. Phosphate and silicate concentrations decreased in time between Day 0 and Day 11 in all treatments, though with obvious differences between treatments (Figures 4B, C). Phosphate concentrations on Day 0 were 3.3 ± 0.3 nM, 3.8 ± 0.7 nM and 3.8 ± 0.2 nM in Control, AgNP and Ag+ treatments respectively, falling to 0.7 ± 0.5 nM, 2.7 ± 0.7 nM and 2.1 ± 0.6 nM respectively by Day 11. On Day 6 concentration was below the analytical detection limit in the control treatments. Mean concentrations for the three treatments over the duration of the experiment were 1.1 ± 1.1 nM, 2.3 ± 1.2 nM and 1.7 ± 1.1 nM in Control, AgNP and Ag+ treatments respectively, these differences were significant between treatments (Kruskal-Wallis test: x 2(2)= 18.266, n=108, p<0.001), with the higher phosphate concentrations in the AgNP and Ag+ treatments being significantly higher than the control group (Kruskalmc multiple comparison test; p<0.05) (Figure 5A). Particulate organic phosphorus (POP) concentrations also differed significantly between groups (Kruskal-Wallis test: x 2(2)=8.939, n=108, p=0.011). Kruskalmc multiple comparison tests reported that the group AgNP had higher POP concentrations than the control group (p<0.05) (Figure 5B).
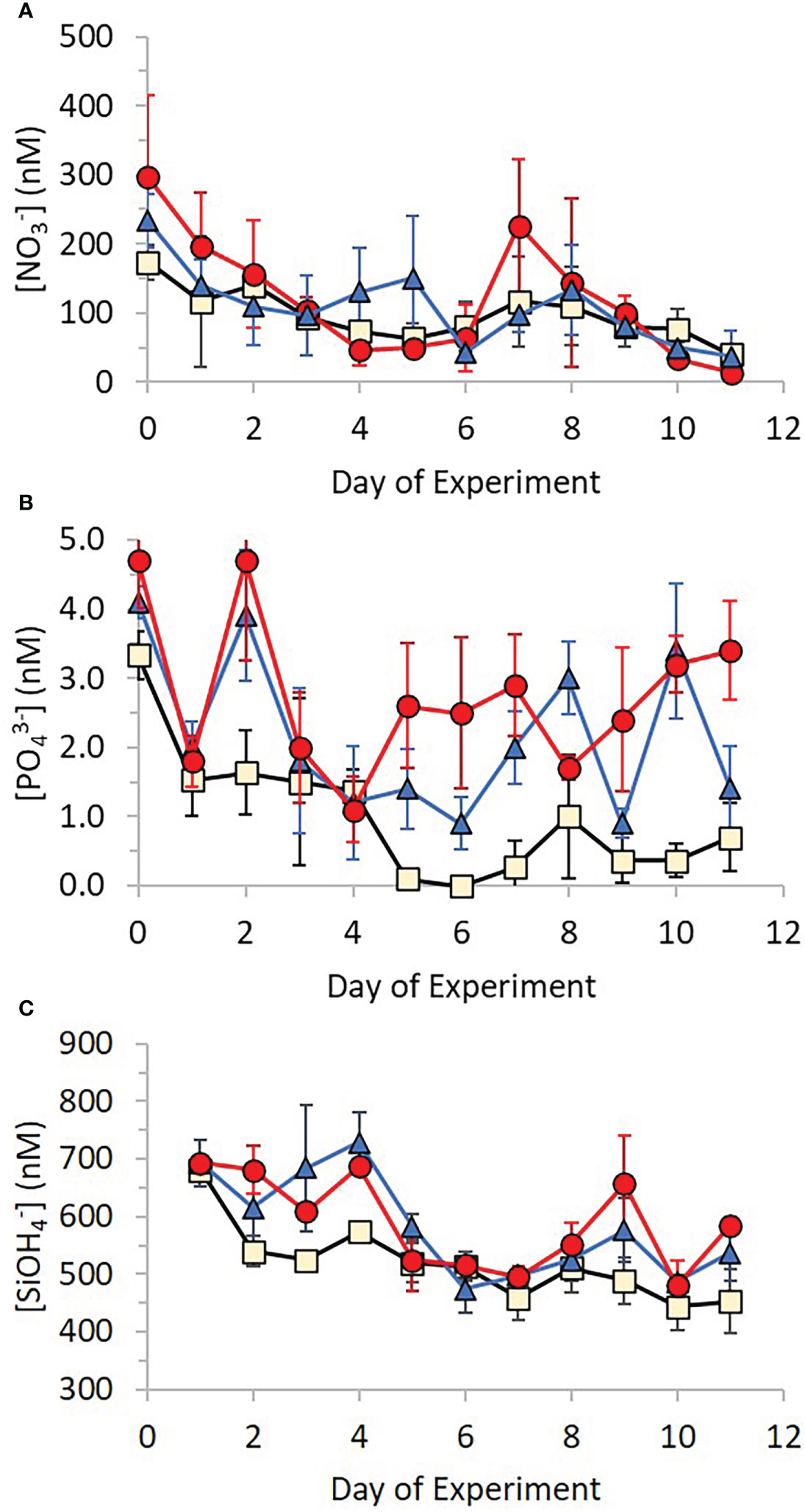
Figure 4 Changes in concentration of (A) nitrate, (B) phosphate and (C) silicate over time for each treatment (Control – beige; +Ag+ – blue; +AgNP – red). Error bars show ± 1 standard deviation of the mean.
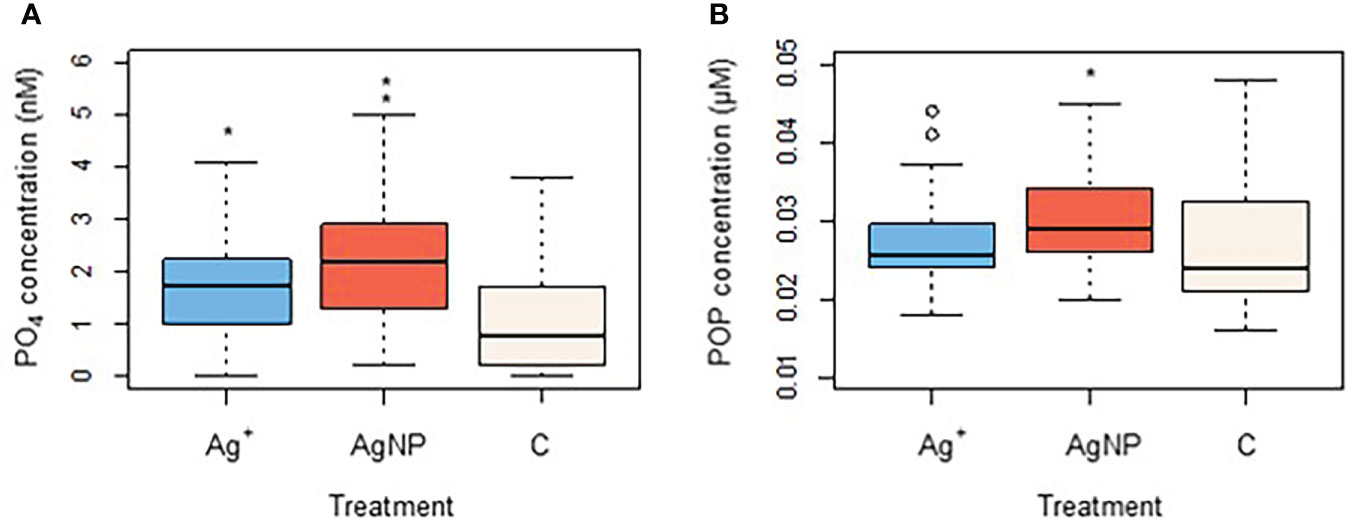
Figure 5 Changes in phosphate (A) and particulate organic phosphorus (B) as an effect of Ag+ and AgNPs addition. Asterisks above boxplots indicate levels of significance (p<0.05).
Similar to the pattern of changes in phosphate concentration, silicate concentrations decreased from 681 ± 17 nM, 696 ± 41 and 711 ± 27 nM in Control, AgNP and Ag+ treatments respectively, falling to 453 ± 56 nM, 504 ± 75 nM and 570 ± 35 nM respectively by Day 11. Overall silicate concentrations varied significantly between treatments (Kruskal-Wallis test: x 2(2)=8.780, n=108, p= 0.012), with higher concentrations recorded in the AgNP treatments compared to control groups (Kruskalmc multiple comparison test: p<0.05), as shown in Figure 6.
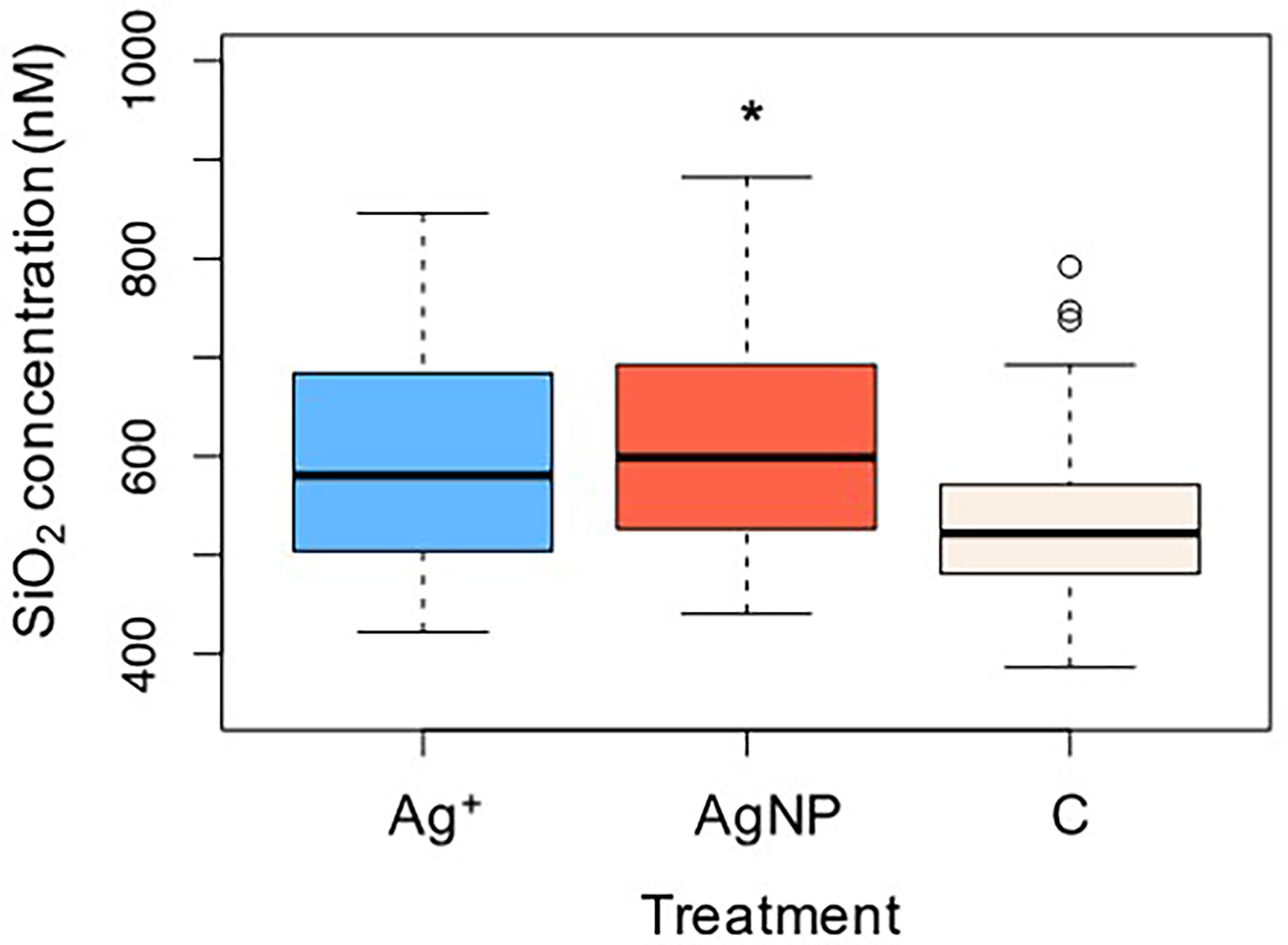
Figure 6 Changes in silicate as an effect of Ag+ and AgNPs addition. Asterisks above boxplots indicate levels of significance (p<0.05).
There were no apparent differences between the rates of APA between treatments for both total and dissolved fractions. Rates of total APA within all treatments decreased from 100.6 ± 4.1 nMh-1 on Day 0 to 27.4 ± 3.6 nMh-1 on Day 11, whilst rates of APA in the dissolved fraction decreased from 40.0 ± 2.1 nMh-1 to 15.3 ± 3.1 nMh-1 over the duration of the experiment. Although the rates of dissolved APA were not significantly affected by the addition of AgNPs and Ag+ when compared to the control group (Kruskal-Wallis test: x 2(2)=2.612, n=108, p=0.271), there was a (non-significant) increase in the % of dissolved over total APA in Ag+ and AgNP treatments (Kruskal-Wallis test: x . 2(2)=3.72, n=108, p=0.195) (Figure 7).
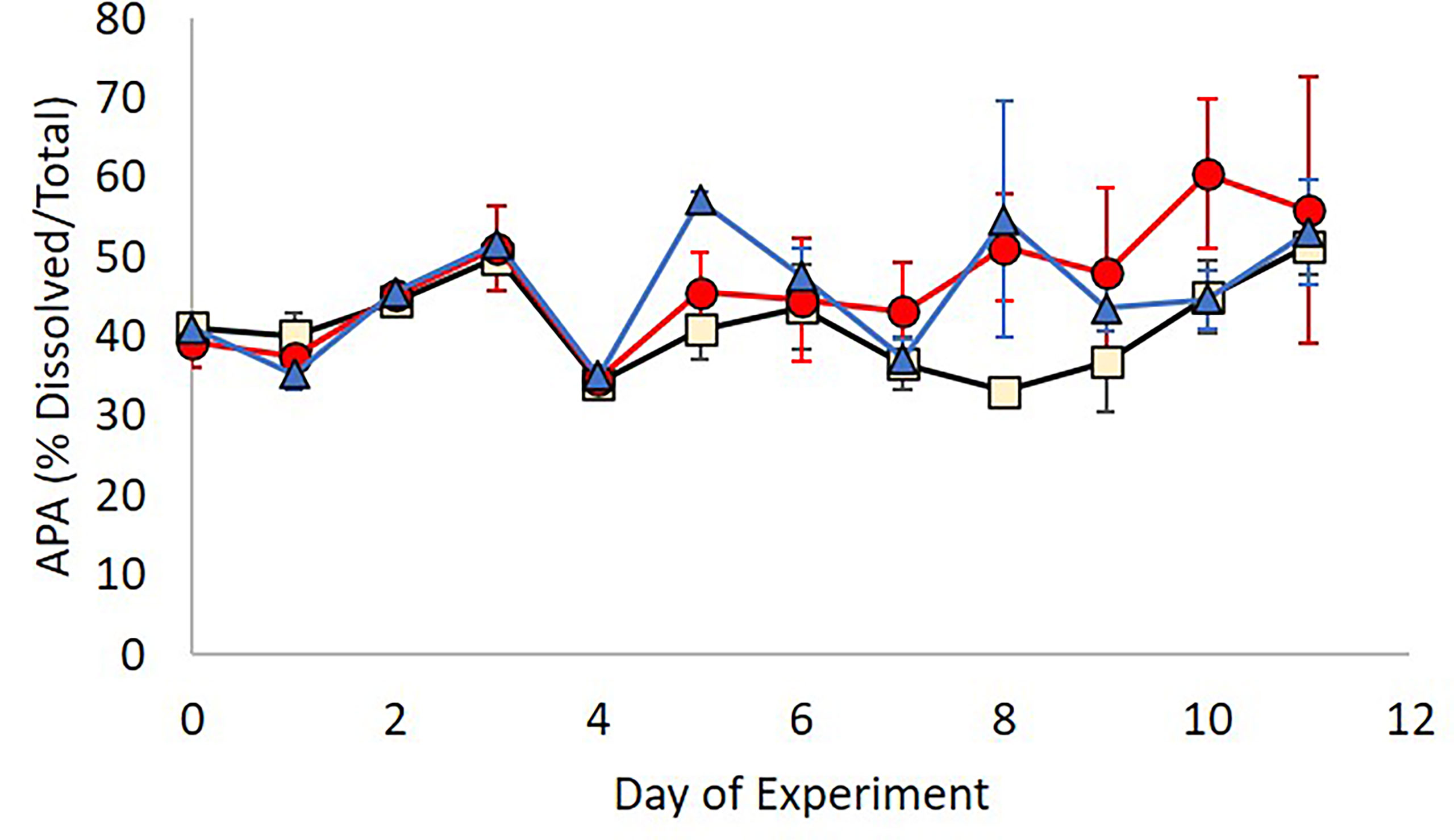
Figure 7 Contribution of cell free alkaline phosphatase activity to total APA in the three treatments; Control (beige), Ag+ (blue), AgNP (red).
The correlation between nitrogen fixation and , POP, and APA was explored further with Spearman’s rank correlation tests. A positive correlation was found between nitrogen fixation and concentration (rs=0.339; n=108; p<0.001) and between nitrogen fixation and POP (rs=0.259; n=108; p=0.008). No significant correlations were detected between nitrogen fixation rates and dissolved APA (rs=0.164; n=108; p=0.097) nor between nitrogen fixation and relative APA (rs=-0.078; n=108; p=0.433).
Principal Component Analysis (PCA) was performed to explore the association between nitrogen fixation rates, nutrient concentrations and APA. 56.4% of the total variance in the dataset were explained by components 1 and 2 (Figure 8).
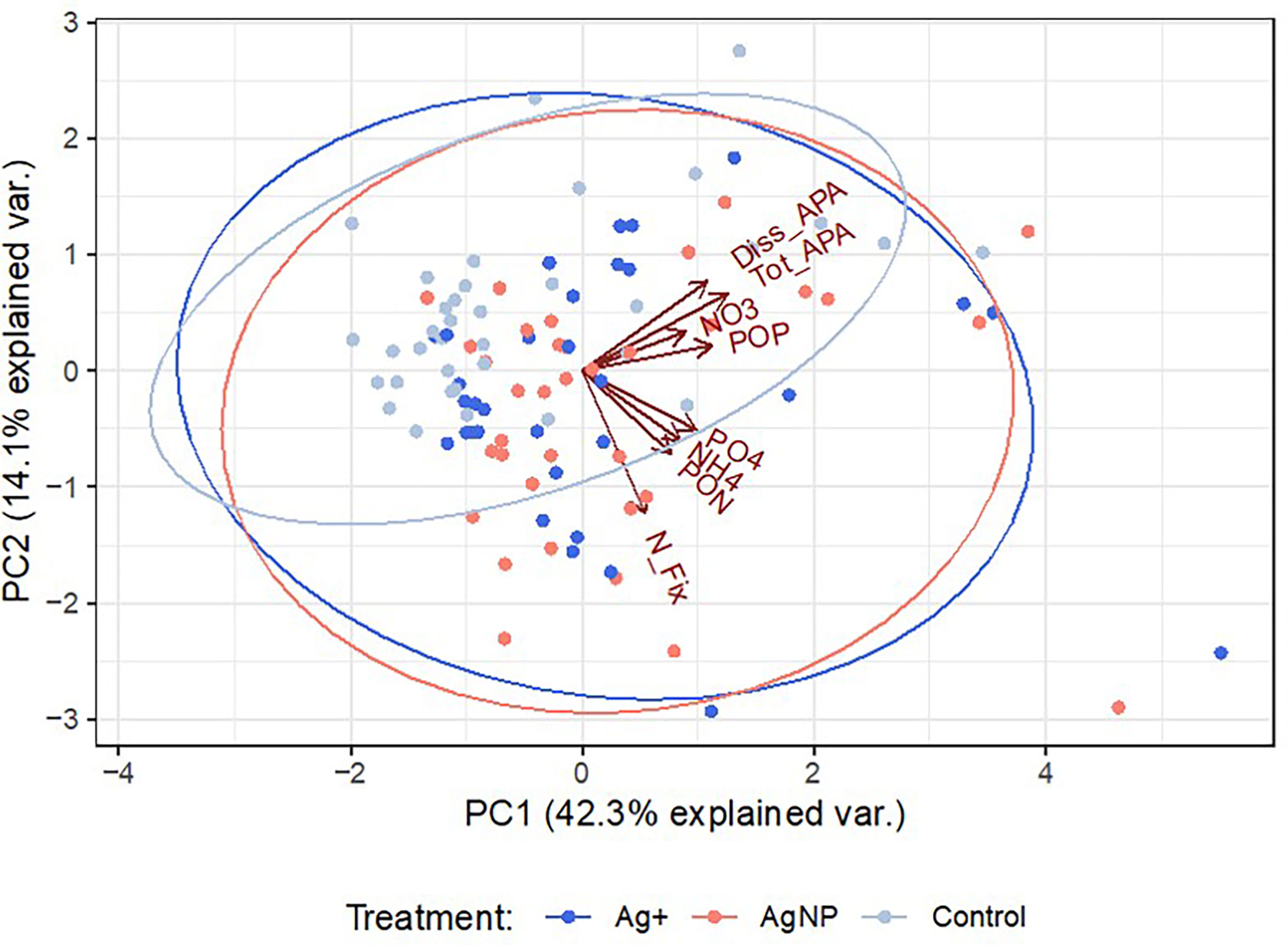
Figure 8 Principal component analysis showing the level of association between the variables analysed. PCA scores (colour-coded dots for each treatment) represent the number of observations used for PCA analysis (n=103).
Microbial community
Targeted qPCR analysis using primers for selected diazotrophs was unsuccessful and we were unable to identify the local community of nitrogen fixing organisms by this approach. Genetic sequencing was performed to investigate the variability of prokaryotic communities by utilising the 16S ribosomal RNA signatures. The overall bacterial community composition considered in this study, which includes diazotrophic groups, showed clear alterations during the time-period of the experiment but there were no significant differences between groups receiving different treatments (ANOSIM tests for difference over time R = 0.745; p = 0.001, and for differences between treatment groups R = 0.143; p = 0.196). The community was dominated by SAR11 (average relative abundance of 29.5% ± 4.19% SD), SAR86 (17.2% ± 4.22% SD) and Puniceispirillales (9.9% ± 2.6% SD; Supplementary Figure 3). The relative sequence abundance of the Parvibaculales (PERMANOVA Pseudo-F = 16.185; p = 0.001) and Oceanospirillales (PERMANOVA Pseudo-F = 13.127; p = 0.001) significantly increased over time whereas the Cellvibrionales (PERMANOVA Pseudo-F = 3.677; p = 0.023) and Opitutales (PERMANOVA Pseudo-F = 4.7856; p = 0.013) decreased over time. Tests to identify taxa influenced by the addition of Ag+ and AgNP showed only two ASVs which had relative sequence abundance lower within the Ag+ and AgNP amended treatments: one closely related to Gammaproteobacteria Ectothiorhodospiraceae (Figure 9A) and the other with Verrucomicrobia Opitutales (Figure 9B). The relative abundance of five ASVs related to taxa that have previously been identified as nitrogen fixers or carriers of nitrogen fixing genes were observed and each found to be significantly correlated to determined nitrogen fixation rates (p< 0.05; Supplementary Table 2). Of these, two were most closely related to Burkholderiaceae, two related to the Oceanospirillales and one related to the Pseudomonadales
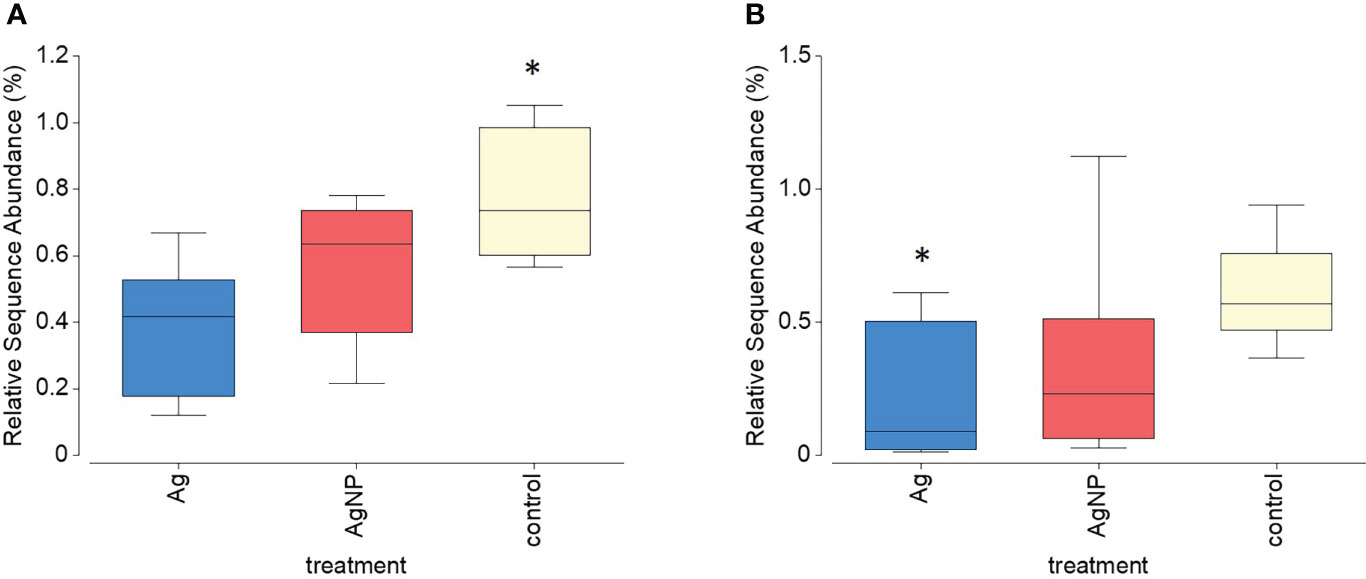
Figure 9 Relative sequence abundance of two ASVs (A) Gammaproteobacteria Ectothiorhodospiraceae and (B) Verrucomicrobia Opitutales) that significantly declined within treatments containing Ag+ and AgNP. Asterisks above boxplots indicate levels of significance (p<0.05).
Similar to the prokaryote 16S rRNA gene data, ANOSIM tests show major changes to the eukaryote community (derived from 18S rRNA gene sequence data) over time (R = 0.737; p = 0.001) but there was no evidence of differences between treatments (R = 0.119; p = 0.236). At the start of the experiment, the community was dominated by Dinophyceae, Bacillariophyceae and Prymnesiophycaea sequences (Supplementary Figure 4). Over time, there was an increase in the relative abundance of sequences identified as grazers: first ciliates (Class Spirotrichea and Oligotichea), followed by Radiozoa Ancantharia and Hexanauplia. The Ag treatments had no significant effect on the abundance of eukaryote taxa. Detailed searches for taxa with higher or lower relative sequence abundance within the Ag+ and AgNP treated mesocosms showed three species of diatoms belonging to the Class Bacillariophyceae (Chaetoceros muelleri, Leptocylindrus aporus, Proboscia alata) which showed changes in relative abundance as an effect of treatments (Figure 10). Control groups exhibited a significantly higher abundance than Ag+ and AgNP treated mesocosms for the species Chaetoceros muelleri (PERMANOVA Pseudo-F = 6.682; p = 0.015), Leptocylindrus aporus (PERMANOVA Pseudo-F = 6.875; p = 0.017) and Proboscia alata (PERMANOVA Pseudo-F = 11.245; p = 0.003). Analyses conducted on other planktonic species and on different taxonomic levels (e.g. orders, classes) showed no significant changes in relative abundance between treatments.
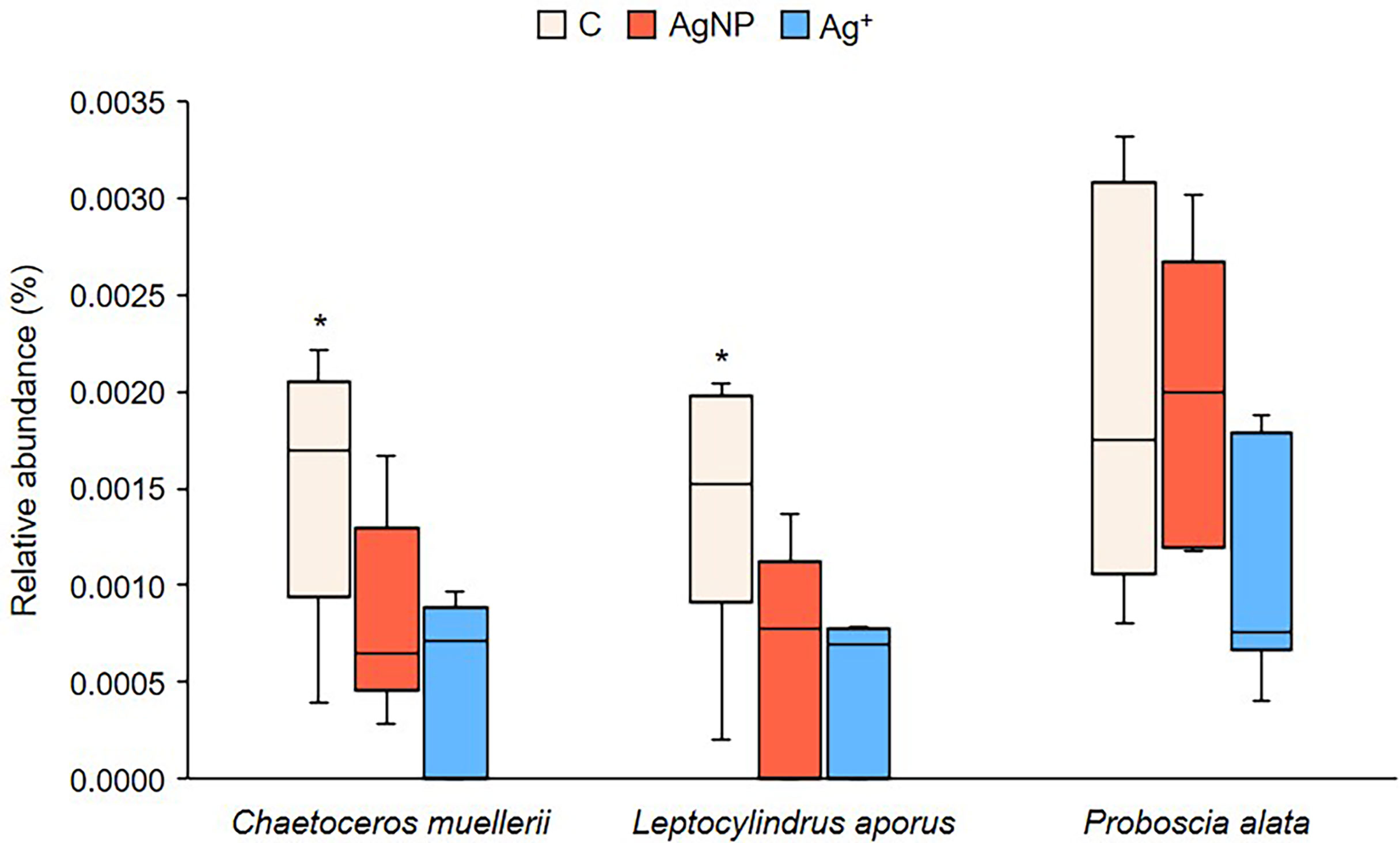
Figure 10 Differences in relative abundance of three diatom species. Asterisks above boxplots show which group significantly differs from the others, as indicated by Tukey multiple comparisons of means test (p<0.05).
Discussion
Background rates of nitrogen fixation determined prior to the experiment of 0.21 ± 0.08 nmol L-1d-1 and those determined during the experiment are comparable to those determined for this region in 2012 (Rahav et al., 2016) and within the range (0.05 to 0.43 nmol L-1d-1) of those published by other authors for the eastern Mediterranean (Ibello et al., 2010; Yogev et al., 2011; Benavides et al., 2016; Rahav et al., 2016). From Figures 2, 3 it is clear that the initial hypothesis that biological nitrogen fixation would be inhibited by the addition of Ag+ and AgNPs was incorrect at the level of addition chosen for this experiment. In contrast there was a significant increase in nitrogen fixation rate and cellular (particulate) N for treatments receiving AgNPs, in treatments receiving Ag+ particulate N was significantly elevated, though nitrogen fixation showed a variable and not significant increase. Compared to mean Control rates over the duration of the experiment of 0.13 ± 0.07 nmol L-1d-1, mean rates in Ag+ and AgNP treatments remained or increased to 0.21 ± 0.19 nmol L-1d-1and 0.28 ± 0.24 nmol L-1d-1respectively. Others have reported that the nitrogen fixation process is unaffected by additions of AgNPs (McGee, 2020), whilst there appear to be no comment on the impact of free Ag+ ions, there is the potential that the free ions may be chelated to some extent rendering them less of a risk to biological communities and processes (Guo et al., 2019). And whilst there is a differential response, it has been suggested that low additions of AgNP might result in a stimulation of biological nitrogen fixation (Guo et al., 2019). As the release of AgNPs in marine environments is expected to increase (Tsiola et al., 2018), the concentrations of AgNPs and Ag+ used in this experiment (50 ngL-1 stepwise addition per day), were purposely kept low in order to represent environmentally relevant concentrations relative to background conditions typically of 0.3 pmolL-1 to ~300 pmolL-1 in oceanic and coastal environments respectively (Barriada et al., 2007). Previous studies report that sublethal concentrations of AgNPs and Ag+ (equivalent to 25 µgL-1 for the diazotrophic bacteria Azotobacter vinelandii) had no significant effects on the expression of nitrogen-fixing genes (nifH genes) and that toxicity was observed at higher silver concentrations (Yang et al., 2013). It has been documented that even at low concentrations, dissolved silver can strongly bioaccumulate in phytoplankton, marine invertebrates and seaweeds, representing a threat to marine life (Reinfelder and Chang, 1999). The increase in nitrogen fixation rate following addition of Ag+ and AgNPs is initially counter intuitive though on reflection suggests that the diazotrophic community have taken an unprecedented benefit from some artefact of the experimental treatments.
Whereas a balanced nutrient supply is essential to maintain growth and productivity of biological systems, in the nutrient impoverished regions of the oceans the relative concentrations of the essential nutrients might be out of balance so that one or other may limit productivity. In the eastern Mediterranean it is widely accepted that microbial productivity is limited by the availability of phosphorus (Krom et al., 2004; Thingstad and Mantoura, 2005; Krom et al., 2010). Marine nitrogen fixation is often also limited by the availability of iron, an essential component of the nitrogenase enzyme complex responsible for diazotrophic activity. The sustained delivery of Saharan derived dust to the waters of this region is thought to maintain sufficiency of iron for this purpose (Ridame et al., 2011; Rahav et al., 2013). The competition for available phosphorus is therefore a particular challenge for diazotrophic communities who are able to satisfy their nitrogen requirements by accessing dissolved N2 and is likely one of the reasons for the low rates of nitrogen fixation experienced in this region (Yogev et al., 2011). Whereas dissolved nitrogenous nutrients showed consistent patterns with no variability between treatments (Figure 4A), concentration of (Figure 4B) significantly decreased in Controls relative to both Ag+ and AgNP treatments (Figure 5). This was reflected in : ratios over the experimental period of 133 ± 199, 76 ± 89 and 77 ± 89 in control, AgNP and Ag+ treatments respectively, indicating some reduction of the severe inherent P limitation following addition of AgNP and Ag+. This may reflect an Ag+ and AgNP inhibition of uptake under experimental treatments, possibly due to the stress/damaging effects of these substances on plankton [e.g. Navarro et al., 2008; Chinnapongse et al., 2011; Das et al., 2012; Das et al., 2014]. Under P starved conditions the microbial community may utilise alkaline phosphatase as a means to access P found within the organic phosphorus pool (Thingstad and Mantoura, 2005; Celussi et al., 2017). Rates of APA are often used as a proxy for P-limitation of planktonic microbial communities (Sala et al., 2001). Initial total APA values (Supplementary Table 4) in our enclosures were approximately 20 times higher than previously reported rates measured in offshore Cretan waters (Luna et al., 2012; Zaccone et al., 2012), thus indicating a phosphate-limited condition and a concomitant hydrolysis stimulation (Celussi and Del Negro, 2012) by POP (ca. 15 times higher than ), as evidenced by the PCA (Figure 8). Total and dissolved APA decreased throughout the experiment, yet a higher proportion of the dissolved activity was detected after day 4 in Ag+ and AgNP treatments (Figure 7). Such phenomenon has previously been observed under different stress conditions (Baltar et al., 2019) and we can speculate that Ag provision might contribute to the alteration of alkaline phosphatase production and release to the environment.
Interrogation of 16S and 18S rRNA gene signatures for prokaryotes and eukaryotes, respectively, showed considerable changes occurred over time but, that at a broad taxonomic level, differences between treatments were less pronounced, possibly due to the great variation showed between different species of the same taxonomic groups and possibly because of the relatively small sample size. Single samples for molecular analyses were collected on alternate days resulting in a smaller sample size (n=21) which therefore limited the strength of statistical analyses. 16S rRNA gene data did indicate that the addition of AgNP and Ag+ caused an alteration to the relative sequence abundance of two ASVs: an Ectothiorhodospiraceae (Figure 9A) and an Opitutales (Figure 9B) bacterium. The Ectothiorhodospiraceae form a separate phylogenetic lineage within the Gammaproteobacteria and include photo- and chemoautotrophic purple sulfur mostly halophilic and haloalkaliphilic bacteria (Tourova et al., 2007). Some also contain nifH genes (Tourova et al., 2007) however nitrogen fixation rates measured within this study did not correlate with the relative sequence abundance of ASVs identified as Ectothiorhodospiraceae bacteria (Supplementary Table 2). The Opitutales taxa were most closely related to Puniceicoccaceae, Coraliomargarita and Lentimonas species, which are thought to be specialist degraders of complex organic matter including the brown algal polysaccharide fucoidan (Sichert et al., 2020).
We were unable to detect known nitrogen fixing bacteria using a range of PCR primers. However, 16S rRNA gene data indicated that taxa previously linked with nitrogen fixation were present, with changes to the relative abundance of several correlating with nitrogen fixation rates (Supplementary Table 2). These included two ASVs identified as being most closely related to Burkholderiaceae two ASVs identified as closely related to Oceanospirillales and a further Pseudomonas group It is known that some of the Burkholderia sp., are capable of nitrogen fixation and have been reported in Mediterranean waters (Man-Aharonovich et al., 2007; Le Moal and Biegala, 2009; Bentzon-Tilia et al., 2015) and examination of TARA Oceans metagenomic data indicated the presence of low numbers of putative nitrogen fixing Oceanospirillales within Mediterranean water (Delmont et al., 2018). Other diazotrophs detected in a previous mesocosm experiment conducted in Crete (Rahav et al., 2016) (Bradyrhizobium sp., Mesorhizobium sp., and Azospirillum sp.) were virtually absent in the dataset analysed.
Our analyses indicated that nitrogen fixation rates in Ag+ and AgNP treated waters were higher than in control experiments (Figures 2, 3) and these observations were in parallel to (Figures 4B, 5) and (Figures 4C, 6) concentrations which were similarly higher in treated mesocosms than controls. Initial speculation led us to suspect that Ag treatments had proved toxic to some microbial groups so that the uptake of these two nutrient species was reduced. The reduced activity of phytoplankton following addition of AgNPs is widely documented (Hutchinson, 1973; Tsiola et al., 2017a; Tsiola et al., 2018). It has also been observed that silver nanoparticles can adversely impact phototrophic communities to a higher degree than the effect of ionic silver (González et al., 2015), which might partly explain why silicate and phosphate concentrations and nitrogen fixation rates were higher in amended mesocosms, where phytoplankton activity might have been more significantly impacted by the toxic effect of Ag+ and AgNPs than were prokaryotic diazotrophs. We did not see any change to chlorophyll-a concentration (data not shown), though other studies have shown detrimental effects of AgNPs on photosynthetic organisms which appear to be linked to damage of photosystems, chloroplast damage and decreased chlorophyll content, whilst impacts on heterotrophic bacteria appear to be associated with membrane integrity and oxidative stress (Guo et al., 2019; McGee, 2020). A limited number of studies have indicated some variability in the response of diazotrophs to AgNPs, though it is indicated that nitrogen fixation may be more resilient than other processes in the nitrogen cycle (e.g. ammonium oxidation and denitrification) whilst the expression of nitrogenase genes was found to be unaffected by concentrations of< 25 μg L-1 AgNP (McGee, 2020). It is quite possible that the size of nanoparticle might prove significant in determining the impact on different groups. During this study we used AgNPs of 60 nm which is well within the range of NPs used for previous microalgal toxicity assays, whilst AgNPs used during previous toxicity assays for heterotrophic microbes were generally smaller and hence, it is possible that larger organisms may have suffered more than smaller ones when exposed to relatively large AgNPs. Chlorophyll-a data (not shown) though proved to be consistent across treatments, that is, there was a decrease over time as with other variables but there were no differences between treatments. Our interrogation of the 18S gene sequence also showed that for most groups there was no indication of a response to Ag additions. Changes in the community composition of diatoms as an effect of AgNP addition has been documented in a previous mesocosm experiment (Tsiola et al., 2018) and in this experiment we observed that the relative sequence abundance of only three diatom taxa (Chaetoceros muelleri, Leptocylindrus aporus and possibly Proboscia alata) were negatively affected by Ag+ and AgNP addition compared to control groups. The small relative contribution of these three groups to the total phytoplankton community though was considered unlikely to account for the reduced demand for available and which was observed in Ag+ and AgNP treatments. Consideration of the mass balance of nutrients indicates that there was disruption of metabolic pathways associated with the uptake of P and Si. As there were no significant changes to the absolute or relative microbial community between treatments, a silver induced physiological disruption of nutrient uptake mechanisms seems likely to have been responsible for anomalies in and observed.
The toxic effect of other metal nanoparticles such as tungsten (W) and titanium (Ti) on nitrogen-fixing bacteria and diazotrophic growth has been reported (Allard et al., 2013). Bacteria and prokaryotes, in general, appear to be sensitive to metal nanoparticles at high concentrations, usually higher than 10 mgL-1 (Niazi and Gu, 2009). The main cause of toxicity of nanoparticles is related to their physical properties and high reactivity, which can lead to membrane disorganisation and oxidative DNA damage in prokaryotes (Syed et al., 2010). Exposure to titanium dioxide nanomaterials can cause inhibition of growth rate in the nitrogen-fixing cyanobacteria Anabaena variabilis, as well as reduced nitrogen fixation activity (Cherchi and Gu, 2010). Previous studies in aquatic ecosystems have reported that the toxicity of AgNPs can vary with phosphorus levels (Das et al., 2014), and that environmental phosphate can act as a natural ligand for silver ions (Norman et al., 2015) and decrease Ag+ accumulation in phytoplankton (McTeer et al., 2014). With the extremely low levels of experienced in this region it is unlikely that this mechanism offers a substantial buffer to Ag toxicity.
Limitations and directions for future studies
Limited studies exploring the impact of silver nanoparticles and silver ions on nitrogen-fixing bacteria, have been conducted to-date. Due to the documented harmfulness of silver, defined as the most toxic trace metal after mercury (Barriada et al., 2007), and the crucial role played by diazotrophs in the marine environment, future studies investigating their relationship will be required. The main restricting factor during this study for an exhaustive evaluation of the mechanisms controlling nitrogen fixation rates in oligotrophic waters exposed to Ag+ and AgNPs contamination is the limited understanding of the incumbent diazotroph community composition. This study was limited to a natural community which were not thoroughly identified, efforts to characterise the mechanistic response of specific diazotroph groups to Ag and their further interactions with environmental variables should be the focus of future research direction.
Measurements of Ag+ concentrations and mean size of AgNPs after silver addition to the mesocosms can be used to determine changes in nitrogen fixation rates knowing the exact concentration of Ag+ and AgNPs, whilst the addition of variable sized AgNPs might enable investigation of impacts on different microbial communities. There was apparent disruption of nutrient uptake pathways during this study and so the targeted investigation of these mechanisms should be incorporated to future investigations. Some limitations that are intrinsic of mesocosms experiments might also be considered, such as the manipulation of enclosed environments used to understand wider ecological processes and the applicability of small-scale aquatic experiments to large-scale marine processes (Spivak et al., 2011). For instance, marine nitrogen fixation is thought to be strongly regulated by variation in temperature and physical forcing (Sohm et al., 2011). Future studies aiming to mimic real conditions at relevant environmental concentrations of Ag+ and AgNPs using small, stepwise addition of silver might consider a longer sampling period than 12 days. Previous research has shown that toxicity of AgNPs becomes more extreme to cyanobacteria, heterotrophic bacteria and diatoms after a period of 25 to 30 days (Wang et al., 2014; Tsiola et al., 2018). The results presented here can serve as preliminary insights on the effect of Ag+ and AgNPs addition on nitrogen fixation activity in oligotrophic marine ecosystems. Future research investigating the diazotrophic community composition of coastal ecosystems should target the impact of silver contamination more specifically and to provide advice for the regulation of silver release and pollution in the marine environment.
Conclusions
This study contributes to the existing knowledge on the impacts of AgNPs and Ag+ on marine biological nitrogen fixation characterising oligotrophic waters of the Eastern Mediterranean. At the level of silver additions made in this study (50 ng Ag+ L-1 per day for ten days) the diazotrophic community proved resilient and indeed benefitted from a relative increase in concentration as indicated by increased rates of nitrogen fixation. The increase in could not be attributed to changes in phytoplankton or bacterial abundance and without direct evidence it is proposed that the Ag additions were sufficient to disrupt nutrient uptake metabolic pathways. It follows that the concentrations or size of AgNPs and Ag+ utilised in this study were apparently not toxic to nitrogen-fixing organisms during the time period of the experiment. Positive correlations between nitrogen fixation activity and phosphate as well as particulate organic phosphorus confirm that phosphorus is a limiting nutrient for biological nitrogen fixation. Ultimately, this study emphasises the lack of understanding of the diversity of nitrogen-fixing organisms in this region.
It remains unclear whether the toxicity exerted by silver to marine life is greater by nanosized silver particles or dissolved Ag+. Due to the expanding nanotechnology industry which will result in increased production and discharge of nanomaterials including AgNPs, the wider implications of their release into the environment need to be carefully addressed not just by scientific research, but also governments and policy-makers. As the effects of climate change increase in the next 30-50 years, with potential for new infectious diseases to arise and social instability to grow, the use of nanosilver as a biocidal agent in medical and healthcare systems is likely to increase significantly (Tsiola et al., 2018) and as a result this is likely to result in increased release into surface and underground waters (Guo et al., 2019). It is of paramount importance that we increase our understanding of the environmental impacts and health risks associated with nanosilver released to the environment in order to fully advise regulatory bodies and to engender responsible control in industrial and medical procedures.
Data availability statement
All sequence data can be accessed from NCBI using the Sequence Read Archive (SRA) unique ID PRJNA750732. Nitrogen fixation rate, alkaline phosphatase activity and particulate and dissolved nutrient data is found Supplementary Table 3.
Author contributions
AR planned the experimental procedures, supervised EF, provided quality control to nitrogen fixation data and wrote the manuscript. EF performed experimental procedures, made nitrogen fixation and qPCR analyses and wrote the manuscript. KT supervised EF, analysed qPCR and gene sequences and contributed to manuscript writing. MC and VM performed alkaline phosphatase experiments and contributed to writing the manuscript. ED and SŽ̌ performed nutrient analyses. AM supervised EF and contributed to manuscript writing. PP secured funding. PP and AT planned and organised the experiment and contributed to the daily routine. All authors contributed to the article and approved the submitted version.
Funding
This work was supported through the Transnational Access Programme of the AQUACOSM project, which has received funding from the European Union’s Horizon 2020 research and innovation programme under grant agreement No. 731065, AQUACOSM project. This work was further supported by UKRI funding to the Plymouth Marine Laboratory and contributes to the outputs of the Marine Biogeochemistry and Observations group and the Marine Ecology and Biodiversity group of PML.
Acknowledgments
We are very grateful to all at HCMR for their warm welcome and expertise in delivering this extremely complex experiment.
Conflict of interest
The authors declare that the research was conducted in the absence of any commercial or financial relationships that could be construed as a potential conflict of interest.
Publisher’s note
All claims expressed in this article are solely those of the authors and do not necessarily represent those of their affiliated organizations, or those of the publisher, the editors and the reviewers. Any product that may be evaluated in this article, or claim that may be made by its manufacturer, is not guaranteed or endorsed by the publisher.
Supplementary material
The Supplementary Material for this article can be found online at: https://www.frontiersin.org/articles/10.3389/fmars.2022.907269/full#supplementary-material
References
Allard P., Darnajoux R., Phalyvong K., Bellenger J.-P. (2013). Effects of tungsten and titanium oxide nanoparticles on the diazotrophic growth and metals acquisition by azotobacter vinelandii under molybdenum limiting condition. Environ. Sci. Technol. 47 (4), 2061–2068. doi: 10.1021/es304544k
Aspila K. I., Agemian H., Chau A. S. Y. (1976). SEMIAUTOMATED METHOD FOR DETERMINATION OF INORGANIC, ORGANIC AND TOTAL PHOSPHATE IN SEDIMENTS. Analyst 101 (1200), 187–197. doi: 10.1039/AN9760100187
Auffan M., Rose J., Bottero J.-Y., Lowry G. V., Jolivet J.-P., Wiesner M. R. (2009). Towards a definition of inorganic nanoparticles from an environmental, health and safety perspective. Nat. nanotechnol 4 (10), 634. doi: 10.1038/nnano.2009.242
Baltar F., De Corte D., Yokokawa T. (2019). Bacterial stress and mortality may be a source of cell-free enzymatic activity in the marine environment. Microbes Environ 34 (1), 83–88. doi: 10.1264/jsme2.ME18123
Barriada J. L., Tappin A. D., Evans e. H., Achterberg E. P. (2007). Dissolved silver measurements in seawater. TrAC Trends Anal Chem. 26 (8), 809–817. doi: 10.1016/j.trac.2007.06.004
Bates D., Mächler M., Bolker B., Walker S.. (2014). Fitting linear mixed-effects models using lme4. doi: 10.48550/arXiv.1406.5823
Beddow J., Stolpe B., Cole P. A., Lead J. R., Sapp M., Lyons B. P., et al. (2017). Nanosilver inhibits nitrification and reduces ammonia-oxidising bacterial but not archaeal amoA gene abundance in estuarine sediments. Environ. Microbiol. 19 (2), 500–510. doi: 10.1111/1462-2920.13441
Benavides M., Bonnet S., Hernandez N., Martinez-Perez A. M., Nieto-Cid M., Alvarez-Salgado X. A., et al. (2016). Basin-wide n-2 fixation in the deep waters of the Mediterranean Sea. Global Biogeochem Cycles 30 (6), 952–961. doi: 10.1002/2015GB005326
Bentzon-Tilia M., Severin I. L., Hansen H., Riemann L., et al. (2015). Genomics and ecophysiology of heterotrophic nitrogen-fixing bacteria isolated from estuarine surface water. Mbio 6 (4):e00929-15. doi: 10.1128/mBio.00929-1
Bolyen E., Rideout J. R., Dillon M. R., Bokulich N. A., Abnet C. C., Al-Ghalith G. A., et al. (2019). Reproducible, interactive, scalable and extensible microbiome data science using QIIME 2. Nat. Biotechnol. 37 (9), 1091–1091. doi: 10.1038/s41587-019-0209-9
Bonnet S., Grosso O., Moutin T. (2011). Planktonic dinitrogen fixation along a longitudinal gradient across the Mediterranean Sea during the stratified period (BOUM cruise). Biogeosciences 8 (8), 2257–2267. doi: 10.5194/bg-8-2257-2011
Callahan B. J., McMurdie P.J., Rosen M.J., Han A.W., Johnson A.J.A., et al. (2016). DADA2: High-resolution sample inference from illumina amplicon data. Nat. Methods 13 (7), 581. doi: 10.1038/nmeth.3869
Caporaso J. G., Lauber C. L., Walters W. A., Berg-Lyons D., Lozupone C. A., Turnbaugh P. J., et al. (2011). Global patterns of 16S rRNA diversity at a depth of millions of sequences per sample. Proc. Natl. Acad. Sci. United States America 108, 4516–4522. doi: 10.1073/pnas.1000080107
Carpenter E. J., Capone D. G. (2008). “Chapter 4 - nitrogen fixation in the marine environment,” in Nitrogen in the marine environment (2nd edition). Ed. Capone D. G. (San Diego: Academic Press), 141–198.
Celussi M., Del Negro P. (2012). Microbial degradation at a shallow coastal site: Long-term spectra and rates of exoenzymatic activities in the NE Adriatic Sea. Estuar. Coast. Shelf Sci. 115, 75–86. doi: 10.1016/j.ecss.2012.02.002
Celussi M., Malfatti F., Franzo A., Gazeau F., Giannakourou A., Pitta P., et al. (2017). Ocean acidification effect on prokaryotic metabolism tested in two diverse trophic regimes in the mediterranean sea. Estuar. Coast. Shelf Sci. 186, 125–138. doi: 10.1016/j.ecss.2015.08.015
Chang B. X., Jayakumar A., Widner B., Bernhardt P., Mordy C. W., Mulholland M. R., et al. (2019). Low rates of dinitrogen fixation in the eastern tropical south pacific. Limnol Oceanogr 64 (5), 1913–1923. doi: 10.1002/lno.11159
Cherchi C., Gu A. Z. (2010). Impact of titanium dioxide nanomaterials on nitrogen fixation rate and intracellular nitrogen storage in anabaena variabilis. Environ. Sci. Technol. 44 (21), 8302–8307. doi: 10.1021/es101658p
Chinnapongse S. L., MacCuspie R. I., Hackley V. A. (2011). Persistence of singly dispersed silver nanoparticles in natural freshwaters, synthetic seawater, and simulated estuarine waters. Sci. Total Environ. 409 (12), 2443–2450. doi: 10.1016/j.scitotenv.2011.03.020
Church M. J., Short C. M., Jenkins B. D., Karl D. M., Zehr J. P., et al. (2005). Temporal patterns of nitrogenase gene (nifH) expression in the oligotrophic north pacific ocean. Appl. Environ. Microbiol. 71 (9), 5362–5370. doi: 10.1128/AEM.71.9.5362-5370.2005
Das P., Williams C. J., Fulthorpe R. R., Hoque M. E., Metcalfe C. D., Xenopoulos M. A., et al. (2012). Changes in bacterial community structure after exposure to silver nanoparticles in natural waters. Environ. Sci. Technol. 46 (16), 9120–9128. doi: 10.1021/es3019918
Das P., Metcalfe C. D., Xenopoulos M. A. (2014). Interactive effects of silver nanoparticles and phosphorus on phytoplankton growth in natural waters. Environ. Sci. Technol. 48 (8), 4573–4580. doi: 10.1021/es405039w
Delmont T. O., Quince C., Shaiber A., Esen O. C., Lee S. T. M., Rappe M. S., et al. (2018). Nitrogen-fixing populations of planctomycetes and proteobacteria are abundant in surface ocean metagenomes. Nat. Microbiol. 3 (7), 804. doi: 10.1038/s41564-018-0176-9
Dobias J., Bernier-Latmani R. (2013). Silver release from silver nanoparticles in natural waters. Environ. Sci. Technol. 47 (9), 4140–4146. doi: 10.1021/es304023p
Faunce T., Watal A. (2010). Nanosilver and global public health: international regulatory issues. Nanomedicine 5 (4), 617–632. doi: 10.2217/nnm.10.33
González A. G., Mombo S., Leflaive J., Lamy A., Pokrovsky O. S., Rols J.–L., et al. (2015). Silver nanoparticles impact phototrophic biofilm communities to a considerably higher degree than ionic silver. Environ. Sci. pollut. Res. 22 (11), 8412–8424. doi: 10.1007/s11356-014-3978-1
Groszkopf T., Mohr W., Baustian T., Schunck H., Gill D., Kuypers M. M. M., et al. (2012). Doubling of marine dinitrogen-fixation rates based on direct measurements. Nature 488 (7411), 361–364. doi: 10.1038/nature11338
Gruber N. (2008). The marine nitrogen cycle: overview and challenges. Nitrogen Mar. Environ. 2, 1–50. doi: 10.1073/pnas.1603646113
Gruber N. (2016). Elusive marine nitrogen fixation. Proc. Natl. Acad. Sci. 113 (16), 4246–4248. doi: 10.1073/pnas.1603646113
Guo Z., Zeng G., Cui K., Chen A.. (2019). Toxicity of environmental nanosilver: mechanism and assessment. Environ. Chem. Lett. 17 (1), 319–333. doi: 10.1007/s10311-018-0800-1
Henryka L.-L., Krystyna P. (2015). Silver nanoparticles – applications and the impact on health and the environment. Medycyna Środowiskowa 18 (3), 7–11.
Hoppe H.-G. (1993). “Use of fluorogenic model substrates for extracellular enzyme activity (EEA) measurement of bacteria,” in Current methods in aquatic microbial ecology. Ed. Kemp P. F. (Boca Raton: CRC Press).
Hutchinson T. C. (1973). Comparative studies of the toxicity of heavy metals to phytoplankton and their synergistic interactions. Water Qual. Res. J. 8 (1), 68–90. doi: 10.2166/wqrj.1973.007
Ibello V., Cantoni C., Cozzi S., Civitarese G. (2010). First basin-wide experimental results on n-2 fixation in the open Mediterranean Sea. Geophys Res. Lett. 37:L03608. doi: 10.1029/2009GL041635
Ivancic I., Degobbis D. (1984). AN OPTIMAL MANUAL PROCEDURE FOR AMMONIA ANALYSIS IN NATURAL-WATERS BY THE INDOPHENOL BLUE METHOD. Water Res. 18 (9), 1143–1147. doi: 10.1016/0043-1354(84)90230-6
Kozich J. J., Westcott S.L., Baxter N. T., Highlander S. K., Schloss P. D., et al. (2013). Development of a dual-index sequencing strategy and curation pipeline for analyzing amplicon sequence data on the MiSeq illumina sequencing platform. Appl. Environ. Microbiol. 79 (17), 5112–5120. doi: 10.1128/AEM.01043-13
Krom M. D., Emeis K. C., Van Cappellen P. (2010). Why is the Eastern Mediterranean phosphorus limited? Prog. Oceanogr 85 (3–4), 236–244. doi: 10.1016/j.pocean.2010.03.003
Krom M. D., Herut B., Mantoura R. F. C. (2004). Nutrient budget for the Eastern Mediterranean: Implications for phosphorus limitation. Limnol Oceanogr 49 (5), 1582–1592. doi: 10.4319/lo.2004.49.5.1582
Le Moal M., Biegala I. C. (2009). Diazotrophic unicellular cyanobacteria in the northwestern Mediterranean Sea: A seasonal cycle. Limnol Oceanogr 54 (3), 845–855. doi: 10.4319/lo.2009.54.3.0845
Levard C., Hotze E. M., Lowry G. V., Brown G. E. Jr, et al. (2012). Environmental transformations of silver nanoparticles: impact on stability and toxicity. Environ. Sci. Technol. 46 (13), 6900–6914. doi: 10.1021/es2037405
Luna G. M., Bianchelli S., Decembrini F., Domenico De E., Danovaro R., Dell'Anno A., et al. (2012). The dark portion of the Mediterranean Sea is a bioreactor of organic matter cycling. Global Biogeochem Cycles 26:GB2017. doi: 10.1029/2011GB004168
Man-Aharonovich D., Kress N., Zeev Bar E., Berman-Frank I., Beja O., et al. (2007). Molecular ecology of nifH genes and transcripts in the eastern Mediterranean Sea. Environ. Microbiol. 9 (9), 2354–2363. doi: 10.1111/j.1462-2920.2007.01353.x
McGee C. F. (2020). The effects of silver nanoparticles on the microbial nitrogen cycle: a review of the known risks. Environ. Sci. pollut. Res. 27 (25), 31061–31073. doi: 10.1007/s11356-020-09548-9
McTeer J., Dean A. P., White K. N., Pittman J. K. (2014). Bioaccumulation of silver nanoparticles into daphnia magna from a freshwater algal diet and the impact of phosphate availability. Nanotoxicology 8 (3), 305–316. doi: 10.3109/17435390.2013.778346
Mermex, Madron D., Guieu C., Sempere R., Conan P., Cossa D., et al. (2011). Marine ecosystems’ responses to climatic and anthropogenic forcings in the Mediterranean. Prog. Oceanogr 91 (2), 97–166. doi: 10.1016/j.pocean.2011.02.003
Mohr W., Grosskopf T., Wallace D. W. R., LaRoche J., et al. (2010). Methodological underestimation of oceanic nitrogen fixation rates. PloS One 5 (9):e12583. doi: 10.1371/journal.pone.0012583
Moisander P. H., Beinart R. A., Voss M., Zehr J. P. (2008). Diversity and abundance of diazotrophic microorganisms in the south China Sea during intermonsoon. ISME J. 2 (9), 954. doi: 10.1038/ismej.2008.5
Moisander P. H., Beinart R. A., Hewson I., White A. E., Johnson K. S., Carlson C. A., et al. (2010). Unicellular cyanobacterial distributions broaden the oceanic N2 fixation domain. Science 327 (5972), 1512–1514. doi: 10.1126/science.1185468
Montoya J. P., Voss M., Kahler P., Capone D. G. (1996). A simple, high-precision, high-sensitivity tracer assay for n-2 fixation. Appl. Environ. Microbiol. 62 (3), 986–993. doi: 10.1128/aem.62.3.986-993.1996
Navarro E., Baun A., Behra R., Hartmann N. B., Filser J., Miao A.-J., et al. (2008). Environmental behavior and ecotoxicity of engineered nanoparticles to algae, plants, and fungi. Ecotoxicology 17 (5), 372–386. doi: 10.1007/s10646-008-0214-0
Niazi J. H., Gu M. B. (2009). “Toxicity of metallic nanoparticles in microorganisms-a review,” in Atmospheric and biological environmental monitoring (Springer), 193–206. doi: 10.1007/978-1-4020-9674-7_12
Norman B. C., Xenopoulos M. A., Braun D., Frost P. C. (2015). Phosphorus availability alters the effects of silver nanoparticles on periphyton growth and stoichiometry. PloS One 10 (6), e0129328. doi: 10.1371/journal.pone.0129328
Nowack B., Krug H. F., Height M. (2011). 120 years of nanosilver history: implications for policy makers (ACS Publications).
Owens N. J. P., Rees A. P. (1989). Determination of n-15 at submicrogram levels of nitrogen using automated continuous-flow isotope ratio mass-spectrometry. Analyst 114 (12), 1655–1657. doi: 10.1039/an9891401655
Quast C., Pruesse E., Yilmaz P., Gerken J., Schweer T., Yarza P., et al. (2013). The SILVA ribosomal RNA gene database project: improved data processing and web-based tools. Nucleic Acids Res. 41 (D1), D590–D596. doi: 10.1093/nar/gks1219
Rahav E., Herut B., Stambler N., Bar-Zeev E., Mulholland M. R., Berman-Frank I., et al. (2013). Uncoupling between dinitrogen fixation and primary productivity in the eastern Mediterranean Sea. J. Geophys Res: Biogeosci 118 (1), 195–202. doi: 10.1002/jgrg.20023
Rahav E., Shun-Yan C., Cui G., Liu H. B., Tsagaraki T. M., Giannakourou A., et al. (2016). Evaluating the impact of atmospheric depositions on springtime dinitrogen fixation in the Cretan Sea (Eastern mediterranean)-a mesocosm approach. Front. Mar. Sci. 3. doi: 10.3389/fmars.2016.00180
Redfield A. C., Ketchum B. H., Richards F. A. (1963). “The influence of organisms on the composition of seawater,” in The Sea. Ed. Hill M. N. (New York: Interscience).
Rees A. P., Turk-Kubo K. A., Al-Moosawi L., Alliouane S., Gazeau F., Hogan M. E., et al. (2017). Ocean acidification impacts on nitrogen fixation in the coastal western Mediterranean Sea. Estuar. Coast. Shelf Sci. 186, 45–57. doi: 10.1016/j.ecss.2016.01.020
Reinfelder J. R., Chang S. I. (1999). Speciation and microalgal bioavailability of inorganic silver. Environ. Sci. Technol. 33 (11), 1860–1863. doi: 10.1021/es980896w
Ridame C., Guieu C., L'Huegen S.. (2011). Nutrient control of n-2 fixation in the oligotrophic Mediterranean Sea and the impact of Saharan dust events. Biogeosciences 8 (9), 2773–2783. doi: 10.5194/bg-8-2773-2011
Ridame C., Guieu C., L’Helguen S. (2013). Strong stimulation of N2 fixation in oligotrophic Mediterranean Sea: results from dust addition in large in situ mesocosms. Biogeosciences 10 (11), 7333–7346. doi: 10.5194/bg-10-7333-2013
Rimmelin P., Moutin T. (2005). Re-examination of the MAGIC method to determine low orthophosphate concentration in seawater. Anal Chimica Acta 548 (1-2), 174–182. doi: 10.1016/j.aca.2005.05.071
Sala M. M., Karner M., Arin L., Marrase C.. (2001). Measurement of ectoenzyme activities as an indication of inorganic nutrient imbalance in microbial communities. Aquat. Microbial Ecol. 23 (3), 301–311. doi: 10.3354/ame023301
Sandroni V., Raimbault P., Migon C., Garcia N., Gouze E., et al. (2007). Dry atmospheric deposition and diazotrophy as sources of new nitrogen to northwestern Mediterranean oligotrophic surface waters. Deep-Sea Res. Part I-Oceanogr Res. Pap 54, 1859–1870. doi: 10.1016/j.dsr.2007.08.004
Sichert A., Corzett C.H., Schechter M.S., Unfried F., Markert S., Becher D., et al. (2020). Verrucomicrobia use hundreds of enzymes to digest the algal polysaccharide fucoidan. Nat. Microbiol. 5 (8), 1026. doi: 10.1038/s41564-020-0720-2
Sohm J. A., Webb E. A., Capone D. G. (2011). Emerging patterns of marine nitrogen fixation. Nat. Rev. Microbiol. 9 (7), 499. doi: 10.1038/nrmicro2594
Spivak A. C., Vanni M. J., Mette E. M. (2011). Moving on up: can results from simple aquatic mesocosm experiments be applied across broad spatial scales? Freshw. Biol. 56 (2), 279–291. doi: 10.1111/j.1365-2427.2010.02495.x
Strickland J. D. H., Parsons T. R. (1972). A practical handbook of seawater analysis. 2nd ed (Ottawa: Fisheries Board of Canada), 167.
Syed M. A., Manzoor U., Shah I., Bukhari S. H. A., et al. (2010). Antibacterial effects of tungsten nanoparticles on the escherichia coli strains isolated from catheterized urinary tract infection (UTI) cases and staphylococcus aureus. New Microbiol 33 (4), 329–335.
Team R. C. (2019). A language and environment for statistical computing (Vienna, Austria: R Foundation for Statistical Computing; 2012). Available at: https://www.R-project.org.
Thingstad T. F., Mantoura R. F. C. (2005). Titrating excess nitrogen content of phosphorous-deficient eastern Mediterranean surface water using alkaline phosphatase activity as a bio-indicator. Limnol Oceanography-Methods 3, 94–100. doi: 10.4319/lom.2005.3.94
Thompson A., Carter B.J., Turk –Kubo K., Malfatti F., Azam F. J., Zehr P., et al, et al. (2014). Genetic diversity of the unicellular nitrogen-fixing cyanobacteria UCYN-a and its prymnesiophyte host. Environ. Microbiol. 16 (10), 3238–3249. doi: 10.1111/1462-2920.12490
Thompson L. R., Sanders J.G., McDonald D., Amir A., Ladau J., Locey K.J., et al. (2017). A communal catalogue reveals earth’s multiscale microbial diversity. Nature 551 (7681), 457. doi: 10.1038/nature24621
Tourova T. P., Spiridonova E.M., Berg I.A., Slobodova N.V., Boulygina E.S., Sorokin D.Y., et al. (2007). Phylogeny and evolution of the family ectothiorhodospiraceae based on comparison of 16S rRNA, cbbL and nifH gene sequences. Int. J. Syst Evol Microbiol. 57, 2387–2398. doi: 10.1099/ijs.0.65041-0
Tsiola A., Pitta P., Callol A. J., Kagiorgi M., Kalantzi I., Mylona K., et al. (2017a). The impact of silver nanoparticles on marine plankton dynamics: Dependence on coating, size and concentration. Sci. Total Environ. 601, 1838–1848. doi: 10.1016/j.scitotenv.2017.06.042
Tsiola A., Tsagaraki T. M., Giannakourou A., Nikolioudakis N., Yucel N., Herut B., et al. (2017b). Bacterial growth and mortality after deposition of Saharan dust and mixed aerosols in the Eastern Mediterranean Sea: A mesocosm experiment (vol 3, 281, 2017). Front. Mar. Sci. 4. doi: 10.3389/fmars.2016.00281
Tsiola A., Toncelli C., Fodelianakis S., Michoud G., Bucheli T.D., Gavriilidou A., et al. (2018). Low-dose addition of silver nanoparticles stresses marine plankton communities. Environ. Sci: Nano 5 (8), 1965–1980. doi: 10.1039/C8EN00195B
Turk-Kubo K. A., Karamchandani M., Capone D.G., Zehr J.P.. (2014). The paradox of marine heterotrophic nitrogen fixation: abundances of heterotrophic diazotrophs do not account for nitrogen fixation rates in the e astern T ropical s outh p acific. Environ. Microbiol. 16 (10), 3095–3114. doi: 10.1111/1462-2920.12346
Voss M., Bange H. W., Dippner J. W., Middelburg J. J., Montoya J. P., Ward B., et al. (2013). The marine nitrogen cycle: recent discoveries, uncertainties and the potential relevance of climate change. Philos. Trans. R. Soc. B: Biol. Sci. 368 (1621), 20130121. doi: 10.1098/rstb.2013.0121
Wang H., Ho K. T., Scheckel K. G., Wu F., Cantwell M. G., Katz D. R., et al. (2014). Toxicity, bioaccumulation, and biotransformation of silver nanoparticles in marine organisms. Environ. Sci. Technol. 48 (23), 13711–13717. doi: 10.1021/es502976y
Winter B. (2013). Linear models and linear mixed effects models in r with linguistic applications. doi: 10.48550/arXiv.1308.5499
Yang Y., Wang J., Xiu Z., Alvarez P. J. J. (2013). Impacts of silver nanoparticles on cellular and transcriptional activity of nitrogen-cycling bacteria. Environ. Toxicol. Chem. 32 (7), 1488–1494. doi: 10.1002/etc.2230
Yogev T., Rahav E., Bar-Zeev E., Man-Aharonovich D., Stambler N., Kress N., et al. (2011). Is dinitrogen fixation significant in the levantine basin, East Mediterranean Sea? Environ. Microbiol. 13 (4), 854–871. doi: 10.1111/j.1462-2920.2010.02402.x
Zaccone R., Boldrin A., Caruso G., Ferla La R., Maimone G., Santinelli C., et al. (2012). Enzymatic activities and prokaryotic abundance in relation to organic matter along a West-East Mediterranean transect (TRANSMED cruise). Microbial Ecol. 64 (1), 54–66. doi: 10.1007/s00248-012-0011-4
Keywords: Diazotroph community, Silver, nanoparticle, Mediterranean sea, Phosphate, Nitrogen Fixation, Phosphorus, alkaline phosphatase
Citation: Rees AP, Faraggiana E, Tait K, Celussi M, Dafnomilli E, Manna V, Manning A, Pitta P, Tsiola A and Živanović S (2022) The presence of silver nanoparticles reduces demand for dissolved phosphorus to the benefit of biological nitrogen fixation in the coastal eastern Mediterranean Sea. Front. Mar. Sci. 9:907269. doi: 10.3389/fmars.2022.907269
Received: 29 March 2022; Accepted: 22 August 2022;
Published: 21 September 2022.
Edited by:
Hongbin Liu, Hong Kong University of Science and Technology, Hong Kong SAR, ChinaReviewed by:
Keisuke Inomura, University of Rhode Island, United StatesShunyan Cheung, Hong Kong University of Science and Technology, Hong Kong SAR, China
Copyright © 2022 Rees, Faraggiana, Tait, Celussi, Dafnomilli, Manna, Manning, Pitta, Tsiola and Živanović. This is an open-access article distributed under the terms of the Creative Commons Attribution License (CC BY). The use, distribution or reproduction in other forums is permitted, provided the original author(s) and the copyright owner(s) are credited and that the original publication in this journal is cited, in accordance with accepted academic practice. No use, distribution or reproduction is permitted which does not comply with these terms.
*Correspondence: Andrew P. Rees, QVBSRUBwbWwuYWMudWs=
†These authors have contributed equally to this work and share first authorship