- 1Marine Ecology and Biodiversity, Plymouth Marine Laboratory, Plymouth, United Kingdom
- 2School of Biological and Marine Sciences, University of Plymouth, Plymouth, United Kingdom
- 3Ocean Biogeosciences, National Oceanography Centre, Southampton, United Kingdom
Copepods from the genus Calanus provide an important lipid-rich food source in the Arctic marine foodweb. Despite extensive research on Calanus finmarchicus and Calanus glacialis, accurately identifying adults to species level remains challenging due to similar morphologies. Although these species co-occur in many regions, the distribution of C. finmarchicus and C. glacialis correspond to Atlantic and Arctic water masses respectively and are frequently used as climate indicators. Correct identification is therefore vital for understanding the phenotypic plasticity of these species and the impacts climate change will have on Calanus-dominated marine ecosystems. In this study, prosome length and percentage of red pigmentation (redness) of genital somites, the antennae, and throughout the whole body were determined for 139 females of C. finmarchicus and C. glacialis from the Fram Strait. Molecular analysis of a 16S rDNA barcode confirmed that the best morphological features for resolving the identity of these two species were the redness of the antennae and the redness of the genital somites. Overall accuracy of using antennae redness and genital somite redness to discriminate between the two species were the same, yet each of these explanatory variables had different specificity; C. finmarchicus were more accurately identified by the absence of redness in the genital somites, whereas C. glacialis were more accurately identified using antennae redness. Given the ecological importance of these congeners, these findings contribute to a better understanding of the reliability of using morphological characteristics to identify Calanus to species level, especially when sorting live specimens for climate-related ecological experiments.
Introduction
In the North Atlantic and Arctic marine ecosystems, copepods of the genus Calanus dominate the zooplankton community biomass (Jaschnov, 1970; Fleminger and Hulsemann, 1977). These energy-rich animals play a vital role in food webs consuming primary producers and microzooplankton and passing this energy up to higher trophic levels including ecologically and commercially important species of fish, birds and whales (Varpe et al., 2005; Karnovsky et al., 2008; Falk-Petersen et al., 2009). Calanoid copepods also play a critical role in regulating the Earth’s climate, by removing carbon dioxide from the sea surface through their consumption of phytoplankton and transporting this carbon to depth through vertical migration and respiration, and the production of fast-sinking carbon-rich faecal pellets (Wilson et al., 2008).
Three species of Calanus co-exist in the sub-arctic North Atlantic and Arctic: Calanus hyperboreus, Calanus glacialis and Calanus finmarchicus. The reproductive range of Calanus helgolandicus also overlaps with C. finmarchicus and C. glacialis, but this warm-temperate water species is largely restricted to southern areas of the North Atlantic, the Celtic Sea and North Sea. C. hyperboreus is a deep-water Arctic species, found predominantly in the Arctic Ocean and Greenland Sea (Hirche et al., 1994). C. glacialis is considerably smaller than C. hyperboreus and is found mostly in the Arctic shelf-seas (Frost, 1974; Conover, 1988). C. finmarchicus, overlapping in size with C. glacialis, is a North Atlantic species (Jaschnov, 1970), often coexisting with C. glacialis due to the advection of Atlantic water masses into the sub-Arctic. Considering that the spatial distribution of C. glacialis and C. finmarchicus correspond to the Arctic and Atlantic waters that they respectively originate from, they are regarded as indicator species for those water masses, and indeed climate indicators (Unstad and Tande, 1991; Hirche and Kosobokova, 2007; Falk-Petersen et al., 2009). Model simulations predict that ocean warming and sea ice loss will cause an ‘Atlantification’ of the Arctic Ocean (Wassmann et al., 2006; Slagstad et al., 2011) and hence a northward shift of C. finmarchicus. The model is further supported by analysis of long-term data (Møller and Nielsen, 2020) and experimentally by Kjellerup et al. (2012) who found that a warmer ocean will increase the area in which these sibling species co-exist and reproduce, and likely support a shift in dominance from C. glacialis to C. finmarchicus in the sub-Arctic. Indeed, a recent field campaign has highlighted that not only are C. finmarchicus expatriated to the Arctic but are capable of completing their life-cycle once there, allowing local recruitment (Tarling et al., 2022). Any change in the spatial distributions of these congeners can be used as a tool to monitor climate change effects in northern hemisphere marine ecosystems (Falk-Petersen et al., 2007).
Despite the fundamentally similar role that the different Calanus species play in the marine ecosystem, their size, lipid content, life cycles and phenology all differ, albeit to different extents (Swalethorp et al., 2011; Trudnowska et al., 2020). For example, C. glacialis is recognised as a capital breeder (Hirche and Kattner, 1993) and is more lipid rich than C. finmarchicus, which is considered to be an income breeder, with the potential for capital breeding when food conditions are poor (Plourde and Runge, 1993; Mayor et al., 2009). In addition, C. glacialis is essentially an ice-associated species, whereas C. finmarchicus is predominantly an open ocean species (Wold et al., 2011). As such it has been suggested that C. finmarchicus and C. glacialis support different Arctic food webs (Weslawski et al., 2000; Karnovsky et al., 2008; Falk-Petersen et al., 2009), and therefore a shift in Calanus composition may have major implications on the energy flow through the food web (Falk-Petersen et al., 2009).
To fully understand how climate change and a warmer future will impact Arctic marine food webs, correct identification of these species is essential (Nielsen et al., 2014). C. hyperboreus can reliably be distinguished from both C. glacialis and C. finmarchicus due to its larger size, whereas C. glacialis and C. finmarchicus are morphologically very similar (Frost, 1974) and are often misidentified. Traditional methods for distinguishing between C. finmarchicus and C. glacialis include assessing the curvature of the 5th pair of swimming legs – a method that is not only ambiguous, complex and stressful to the animal, but which has also proven to be unreliable, even when undertaken by skilled taxonomists (Lindeque et al., 1999; Choquet et al., 2018). An easier method of identification is based on prosome length, since C. glacialis are typically larger than C. finmarchicus (Unstad and Tande, 1991; Hirche et al., 1994; Weydmann and Kwasniewski, 2008). However, this approach is also limited because of prosome length overlap of these congeners in regions such as the Greenland Sea, the Irminger Basin, Svalbard and the Norwegian coast (Lindeque et al., 2006; Parent et al., 2011; Gabrielsen et al., 2012; Nielsen et al., 2014; Choquet et al., 2018; Trudnowska et al., 2020). When morphological variation cannot unambiguously identify species, genetic variation may be used for taxonomic discrimination. Calanus species exhibit considerable base-sequence divergence in the mitochondrial 16S rRNA gene (Bucklin et al., 1995). Molecular techniques have been used for the identification of copepods from the genus Calanus including species-specific PCR (Hill et al., 2001); amplification of a region of the 16S rRNA gene using PCR followed by restriction fragment length polymorphism (RFLP) analysis of the amplified product (Lindeque et al., 1999; Lindeque et al., 2004; Lindeque et al., 2006); nuclear microsatellite markers (Provan et al., 2009; Parent et al., 2012; Choquet et al., 2017; Trudnowska et al., 2020) and nuclear insertion/deletion markers (Smolina et al., 2014). The method developed by Lindeque et al. (1999, 2004) has provided a definitive method to unambiguously identify any developmental stage of Calanus to species, and has been successfully used in multiple, large-scale studies (Lindeque et al., 2004; Lindeque et al., 2006; Gabrielsen et al., 2012; Parent et al., 2012; Tarling et al., 2022).
A drawback to all molecular approaches is that they cannot be used to identify animals prior to conducting physiological and ecological experiments that provide insight into the phenological plasticity of different species in response to temperature, food, and other environmental variables. Such experiments are required to fully understand the impacts of climate change on north Atlantic and Arctic food webs, and hence there remains a need to quickly and reliably identify individual live animals with minimal disturbance.
This study builds on the work of Nielsen et al. (2014) to determine the reliability of using prosome length, redness of antennae, somites or the whole animal, to identify live adult females Calanus spp. from the Fram Strait. This region of sympatry for C. finmarchicus and C. glacialis (Von Appen et al., 2015) represents a transition zone between the central Arctic Ocean and the northern North Atlantic where the West Spitsbergen Current transports warm Atlantic Water into the Arctic Ocean and the East Greenland Current carries cold polar water towards the South West (de Steur et al., 2009).
Materials and Methods
Sample Collection
Zooplankton samples were collected in the Fram Strait during late summer 2019 (JR18007, 04/08/2019 to 28/08/2019) aboard RRS James Clark Ross using a motion-compensated Bongo net (200 μm mesh), fitted with a cod end with mesh window, from a depth of 200 m to the surface from ‘Ice Station 2’ (78°20’33.8”N 4°42’04.5”W) and ‘D3’ (79°35’59.6”N 7°19’57.6”E). The collected animals were transferred into a controlled temperature laboratory set at ambient seawater temperature (1°C).
Morphological Characteristics
Live adult female Calanus were carefully picked into 0.2 µm filtered seawater using swan-necked forceps and a dissecting microscope (Wilde M5) under dim light. A total of 139 adult female Calanus were individually photographed in a custom-made Petri dish with visible gradations of known dimensions. Images were captured using an iPhone 7 connected through a microscope adaptor (×12 objective lens, ×10 eyepiece lens), before the photographed individual was transferred into a numbered 1 mL glass vial containing 95% ethanol until molecular analysis. The image analysis software Image J (v. 2.0w; https://imagej.nih.gov/ij/) was used to measure the prosome length by calibrating the software with known distance. Redness of the antennae and genital somite (Figure 1) was measured by cropping the images as tightly as possible around these features, masking any other parts of the organism with white pixels so as not to include them, and measuring red pixels as described in Nielsen et al. (2014); Nielsen et al. (2014b), using thresholding values of: Hue 0-23, Saturation 75-255, Brightness 1-255.
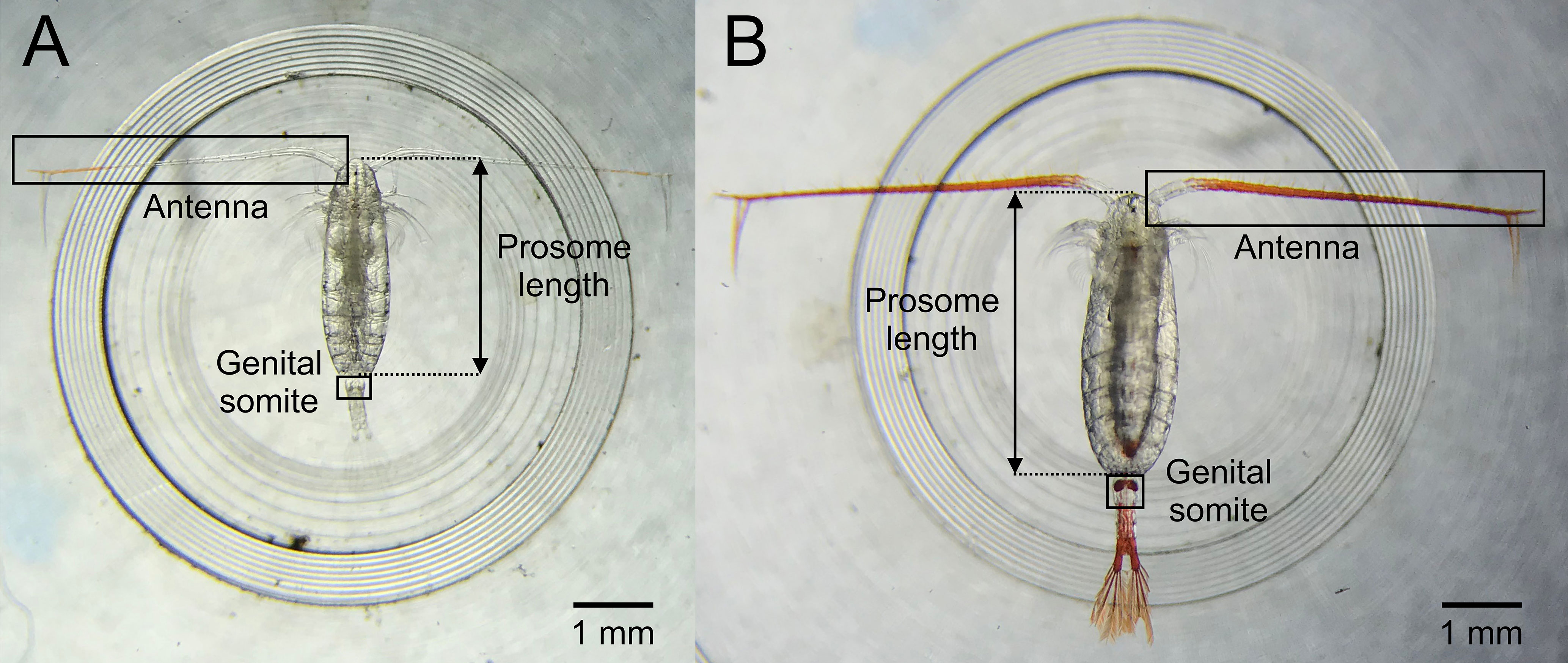
Figure 1 Example images of (A) Calanus finmarchicus and (B) Calanus glacialis showing the diagnostic features used (prosome length and redness criteria of antenna and genital somite) to distinguish between females of the two species.
Molecular Identification
Individual Calanus were genetically characterised to species level based on the Restriction Fragment Length Polymorphism (RFLP) signature of their mitochondrial 16S rDNA using the molecular identification technique described by Lindeque et al. (1999); Lindeque et al. (2006) with modifications detailed below. This was carried out blind, i.e. without reference to the redness and prosome length results.
Individual copepods were removed from sample tubes containing 95% ethanol using a hypodermic needle and were cut in half using a sterile scalpel, half was returned to the ethanol and the other half was processed. Excess ethanol was removed by dabbing on absorbent paper, before rehydration in 150 µL DNA grade water (Fisher Scientific) in a 96-well plate for 6-12 hours at room temperature. After rehydration, the water was removed and replaced with 34.5 µL DNA water and 5µL of 10 x Taq DNA polymerase colourless buffer (Qiagen). The samples were homogenised using a hypodermic needle (19G 11/2) inserted into a pellet pestle hand-held homogeniser (Sigma-Aldrich) and incubated at 4°C overnight. Following incubation, the remaining PCR components were then added: 5 μL 2 mM dNTPS (Qiagen), 2.5 µL of primers 16SB2R (5′-ATTCAACATCGAGGTCACAAAC-3′; Lindeque et al., 1999) and 16SAR (5′-CGCCTGTTTAACAAAACaT-3′; Palumbi and Benzie, 1991) and 0.5 µL of Taq DNA polymerase (Qiagen). Amplifications were performed in a G-Storm, GRI thermocycler. The cycling parameters included an initial denaturation step at 94°C (5 min) followed by 40 cycles of 94°C (1 min), 45°C (2 min), 72°C (1 min) and 94°C (1 min). A final annealing phase at 45°C (2 min) was followed by an extension phase at 72°C (3 min) and storage at 4°C.
To check for amplification efficiency, aliquots (5 µL) of the amplification reaction were analysed by gel electrophoresis (2%). Restriction digests were performed on a 10 µL aliquot of each amplification by the addition of 13 µL DNA water, 3 µL 10 x Tango Buffer and 2 µL of each restriction enzyme (Dde1 and Vsp1, Thermo Scientific). Incubations were performed at 37°C for 4 hours in a PCR machine. 10 µL aliquots of the digestion products were separated by electrophoresis through a 2% metaphor agarose gel (VWR) which had been pre-chilled for 30 minutes at 4°C to improve resolution. The banding patterns were visualised by UV transillumination (SynGene) and the restriction digests analysed.
Data Analysis
The accuracy with which C. finmarchicus and C. glacialis could be discriminated using prosome length, genital somite redness, antennae redness and whole animal redness was examined using logistic regression with binomial errors (Crawley, 2007). The explanatory power of each of these variables was assessed using a variety of metrics: explained deviance (analogous to R2 in a linear regression model); the Akaike Information Criteria (AIC) (a measure of fit after penalizing the model for the number of independent variables); the overall accuracy of the model (its ability to correctly predict the species of Calanus); the area under the receiver operating characteristic (ROC) curve (the higher the value, the better the prediction power of the model). Confusion matrices are presented to illustrate the number of correct/incorrect species identifications generated using each individual explanatory variable. All statistical analyses were conducted in the R programming environment (version 3.6.1; R Core Team, 2019).
Results
Morphological Characteristics
The prosome length distribution of female C. finmarchicus (n = 90) and C. glacialis (n = 49) showed a bimodal pattern, with considerable overlap between the two species (Figure 2). C. glacialis alone also showed a less pronounced but slight bimodal distribution (Figure 2). The mean ± SD prosome lengths of C. finmarchicus and C. glacialis were 2.56 ± 0.24 mm and 3.11 ± 0.38 mm, respectively. The redness (area %) of the genital somites, antennae and whole animal for both species are presented in Figure 3. Respective mean values ± SD were 0.14 ± 1.32, 0.33 ± 0.68 and 0.21 ± 0.35 for C. finmarchicus and 9.06 ± 8.31, 3.05 ± 1.80 and 0.43 ± 0.44 for C. glacialis.
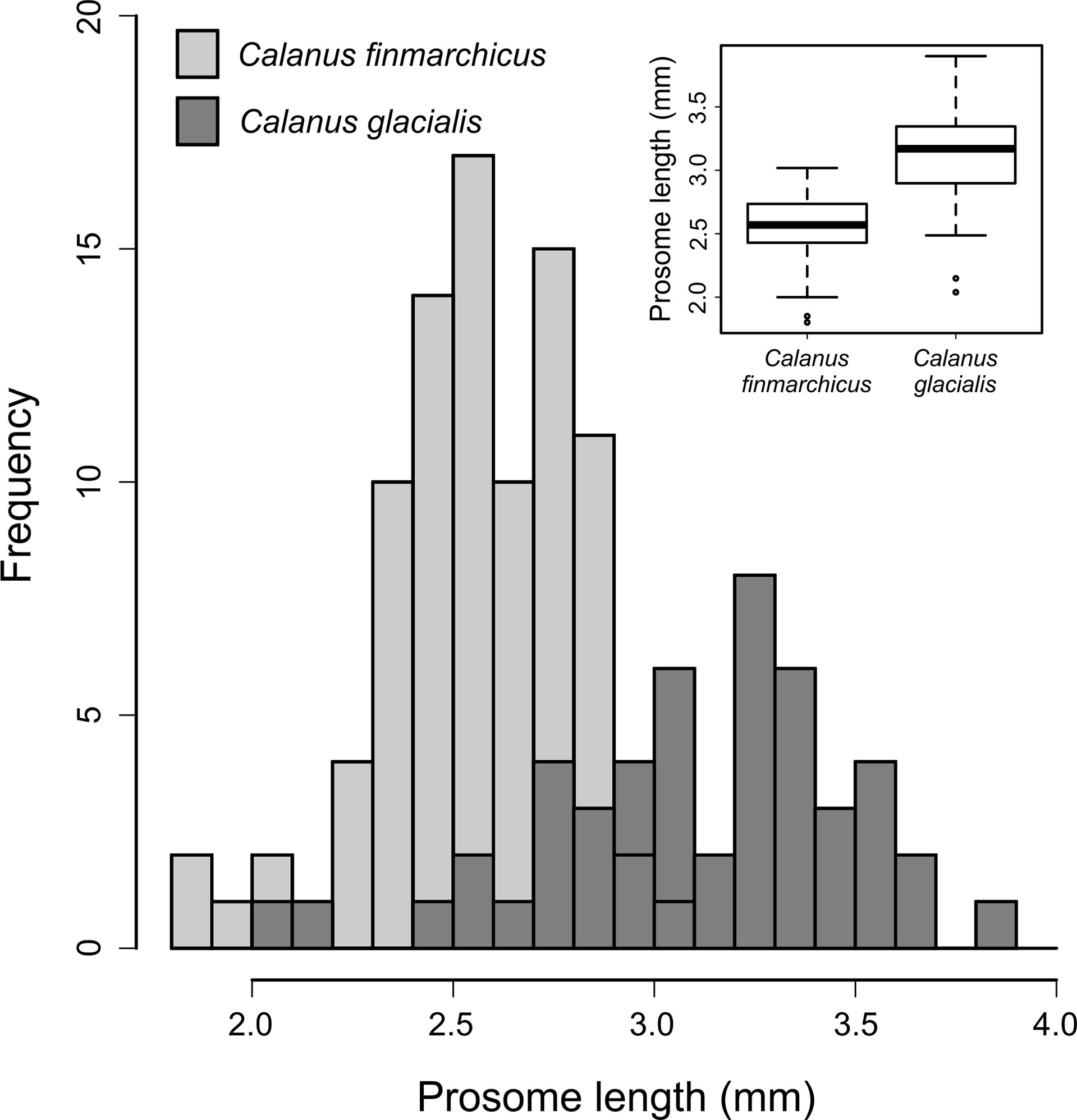
Figure 2 Prosome length distribution of adult females of Calanus finmarchicus and Calanus glacialis from the Fram Strait. Inset boxplot shows the median prosome lengths (thick horizonal lines), their interquartile ranges (top and bottom of boxes = third and first quartile, respectively) and 1.5 times the interquartile ranges (whiskers).
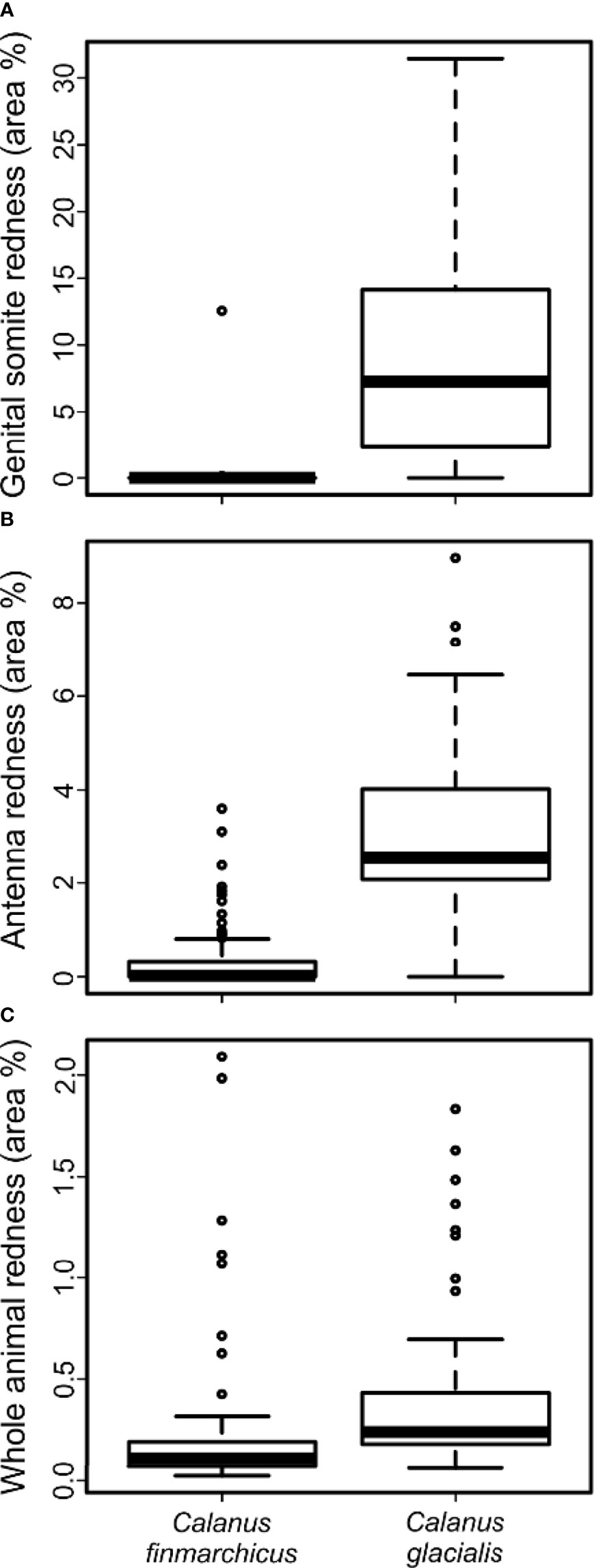
Figure 3 Percentage of red pixels (area %) within selected regions of Calanus finmarchicus and Calanus glacialis: Redness of the genital somite (A), antennae (B) and whole animal (C). Boxplots illustrate the median (thick horizonal lines), interquartile ranges (top and bottom of boxes = third and first quartile, respectively) and 1.5 times the interquartile ranges (whiskers).
Molecular Identification
All 139 individual adult females were identified to species level by RFLP-PCR. Based on the restriction profiles, 49 individuals were assigned to C. glacialis and 90 individuals to C. finmarchicus.
Using Morphological Characteristics to Distinguish Between C. finmarchicus and C. glacialis
Antennae redness was consistently the best at discriminating between C. finmarchicus and C. glacialis across all of the metrics used, and whole animal redness was consistently the worst (Table 1A). The performance of the explanatory variables followed the following rank order: antennae redness > genital somite redness > prosome length > whole animal redness, with the latter explaining only 5% of the observed deviance. Interestingly, the overall accuracy of using antennae redness and genital somite redness to discriminate between the two species were the same (Table 1A), yet each of these explanatory variables had different specificity (Table 1B); C. finmarchicus were more accurately identified using genital somite redness (accuracy = 98.9%), whereas C. glacialis were more accurately identified using antennae redness (accuracy = 85.7%). Prosome length, the third best of the examined response variables, correctly identified C. finmarchicus and C. glacialis in 97% and 76% of the cases, respectively. The high level of accuracy for identifying C. finmarchicus relative to C. glacialis reflects the less variable size range of prosome length in the former (see inset boxplot in Figure 3).
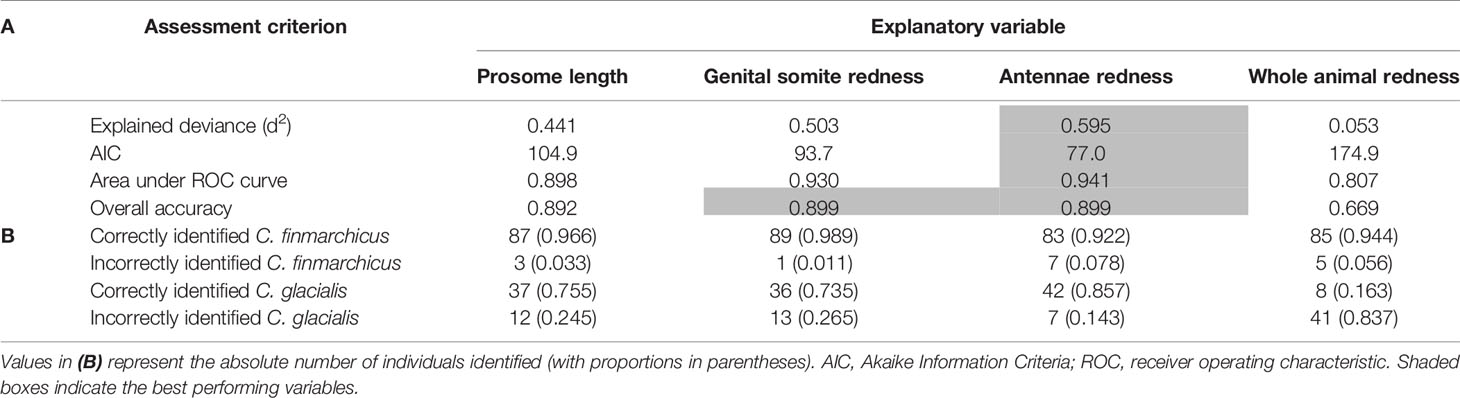
Table 1 Performance metrics (A) and confusion matrices (B) for each of the explanatory variables to discriminate between C. finmarchicus (n = 90) and C. glacialis (n = 49).
Discussion
This study assessed the reliability of identifying C. finmarchicus and C. glacialis in the field using morphological characteristics that are visible by eye. Molecular analysis of a 16S rDNA barcode confirmed that the best overall morphological features for distinguishing between adult females of these two species were the redness of the antennae and the genital somites; both of these criteria generally outperformed prosome length as a distinguishing characteristic, although even prosome length showed good, overall performance. Interestingly, the accuracy of using redness of the antennae and genital somites to discriminate between the two species were the same, yet each of these explanatory variables had different specificity. C. finmarchicus were more accurately identified by the absence of redness in the genital somites (Table 1); of the 90 imaged females, 89 (98.9%) were correctly identified by the level of red pigmentation in this area, whereas only 83 (92.2%) were correctly identified on the basis of their antennae redness. By contrast, C. glacialis were more accurately identified using antennae redness; of the 49 imaged females, 42 (85.7%) were correctly identified by the red pigmentation in the antennae, whereas only 36 (73.5%) could be identified on the basis of the redness of their genital somites.
Previous studies have indicated that live females of C. finmarchicus and C. glacialis can be separated by the red pigmentation of the antennae and genital somites from both East and West Greenlandic waters (Nielsen et al., 2014). Our redness values for the antennae and genital somites for C. glacialis were ~50% lower than those in Nielsen et al. (2014), yet they were sufficient to clearly distinguish between the two species. Choquet et al. (2018) also investigated the reliability of using pigmentation of the antennae and genital somites to identify C. finmarchicus and C. glacialis from the White Sea, Svalbard and three Norwegian fjords. In their study, they found that red pigmentation was variable, with significant differences between the two species, among developmental stages and locations sampled. Nevertheless, the majority of C. finmarchicus tended to have pale antennae, whereas the majority of C. glacialis tended to have red ones. Exceptions to this appear to have been from different developmental stages where males of both species were pale without exception, and C. glacialis CIV were generally less pigmented than C. glacialis CV and CVI. When they compared redness of antennae versus redness of genital somites for both species from distinct locations, in all but two cases, animals with pale antennae and genital somites were C. finmarchicus. All individuals with red antennae and red genital somites were C. glacialis, although some C. finmarchicus from Svalbard and Saltenfjord did display redness in the antennae. Our findings corroborate this: C. finmarchicus were most reliably identified by the absence of redness in the genital somite areas, whereas C. glacialis were most accurately identified by possessing redness in the antennae.
Trudnowska et al. (2020) suggested that the pigmentation of antennae and genital somites in both C. finmarchicus and C. glacialis were highest in copepods inhabiting their preferred water mass, or their ‘comfort zone’. C. finmarchicus CV had high red pigmentation in the antennae when sampled from the Atlantic domain of the Polar Front and C. glacialis CV had high red pigmentation in the antennae and genital somites when sampled from the Arctic domain of the Polar Front. For both species, the amount of redness progressively decreased from the open water stations to the inner fjord and glacial bay stations. If their hypothesis is correct, our observations of poorly pigmented adult female C. finmarchicus in the Fram Strait may indicate that these animals are far less suited to the local conditions than C. glacialis, which were more pigmented. Depth of occurrence may also influence the intensity of red pigmentation in Calanus spp., with deeper-dwelling animals having reduced need for carotenoid pigments (redness) that protect against the harmful effects of ultraviolet irradiance on stored lipids (Trudnowska et al., 2020). Unfortunately, the animals in our study were collected using vertical hauls from 200 m to the surface, and hence variation in red pigmentation with depth could not be assessed.
Previously molecular identification has highlighted how using prosome length as a species discriminator for Calanus has led to erroneous results (Breur, 2003; Lindeque et al., 2004; Parent et al., 2011; Gabrielsen et al., 2012; Nielsen et al., 2014; Choquet et al., 2018). For example, Gabrielsen et al. (2012) found that prosome length systematically overestimated the abundance of C. finmarchicus at the expense of C. glacialis from three Arctic fjords. There is often an overlap between the prosome length of the two species, with the extent of this overlap depending on the location where the species co-occur, the temperature at which development occurred and the likelihood of advection and hence mixing of the species or populations of the same species. In our study, the use of prosome length to distinguish between C. finmarchicus and C. glacialis achieved not dissimilar overall accuracy compared to using the redness of genital somites and antennae (Table 1). Nevertheless, it underperformed relative to the use of genital somites redness when identifying C. finmarchicus, and underperformed relative to the use of antennae redness when identifying C. glacialis. Prosome length correctly identified 87 of the 90 (96.6%) imaged C. finmarchicus, but only 37 of the 49 (75.5%) C. glacialis. The increased accuracy when identifying C. finmarchicus reflects the less variable size range of the individuals of this species in our study, relative to C. glacialis (see inset boxplot in Figure 2). Exactly why the size range of individual C. finmarchicus was less variable than C. glacialis in our study is unknown. It may relate to a more variable temperature range experienced by the copepodites of the latter species during their development as in Parent et al., (2011). Alternatively, it may suggest that the sampled C. glacialis were not from a single generation or even population, and instead, consisted of a local population mixed with at least some larger individuals that have been carried south with the East Greenland Current which carries cold polar water towards the South West of the Fram Strait (de Steur et al., 2009). This interpretation has previously been used to explain why single-species populations of Calanus in the Arctic and Atlantic Oceans have bimodal size distributions (Lindeque et al., 2006; Trudnowska et al., 2020). Regardless, it is clear that the prosome lengths of C. finmarchicus and C. glacialis overlap and cannot be considered as a strong criterion for separating the two species, particularly if the species are being used as representatives of Atlantic versus Arctic water masses and thus climatic indicators.
Our study does, however, suggest, that antennae redness, somite redness and prosome length all achieve a sufficient level of accuracy for identifying adult female Calanus spp. in the Fram Strait for use in experiments. For the purposes of this study, adult female Calanus spp. were picked at random, making no judgement as to whether they were C. finmarchicus, C. glacialis or indistinguishable. However, when picking live animals for use in experiments, we suggest that increased accuracy of species identification may be achieved if only those animals that unambiguously fulfil the pigmentation and/or size criteria were chosen. For example, picking only smaller individuals that showed no redness in the antennae and/or genital somites would likely yield a cohort of individuals dominated by C. finmarchicus, whereas selecting larger animals with increased levels of pigmentation in the antennae and/or genital somites would likely produce a sample dominated by C. glacialis. Accurately determining species identification of live animals in the field using morphological characteristics that are visible by eye greatly facilitates the implementation of experiments to determine how individual species will respond and adapt to climate change in the Arctic. Nevertheless, it is clear that no single morphological characteristic can determine the species of live Calanus with 100% accuracy and we suggest that it is prudent to integrate the use of molecular identification techniques into all future studies working on Calanus spp. in the Arctic.
Data Availability Statement
The original contributions presented in the study are included in the article/supplementary materials. Further inquiries can be directed to the corresponding author.
Author Contributions
Samples were collected, sorted and photographed by DM. AL and IH carried out image analysis to determine antenna and genital somite redness, and prosome length. Molecular analyses were performed by HP and IH. DM undertook data analysis. The manuscript was written by PL, DM and IH with further input from all authors. All authors contributed to the article and approved the submitted version.
Funding
Financial support for authors (PL, DM, HP and KC) was provided by the Changing Arctic Ocean (CAO) Programme DIAPOD, funded by UKRI Natural Environment Research Council (NERC; NE/P006183/1, NE/P006353/1).
Conflict of Interest
The authors declare that the research was conducted in the absence of any commercial or financial relationships that could be construed as a potential conflict of interest.
Publisher’s Note
All claims expressed in this article are solely those of the authors and do not necessarily represent those of their affiliated organizations, or those of the publisher, the editors and the reviewers. Any product that may be evaluated in this article, or claim that may be made by its manufacturer, is not guaranteed or endorsed by the publisher.
Acknowledgments
We are grateful to the officers and crew of the RRS James Clark Ross in supporting field sampling in the Fram Strait. Thanks to David Pond as DIAPOD principal investigator.
References
Breur A. (2003). Influences of Advection on Local Dynamics of Overwintering Zooplankton in an Arctic Sill Fjord. Thesis, Wageningen University, 1–38
Bucklin A., Frost B. W., Kocher T. D. (1995). Molecular Systematics of Six Calanus and Three Metridia Species (Calanoida: Copepoda). Mar. Biol. 121, 655–664. doi: 10.1007/BF00349301
Choquet M., Hatlebakk M., Dhanasiri A., Kosobokova K., Smolina I., Soreide J. E., et al. (2017). Genetic Redraws Pelagic Biogeography of Calanus. Biol. Lett. 13, 20170588. doi: 10.1098/rsbl.2017.0588
Choquet M., Kosobokova K., Kwasniewski S., Hatlebakk M., Dhanasiri A. K. S., Melle W., et al. (2018). Can Morphology Reliably Distinguish Between the Copepods Calanus Finmarchicus and C. Glacialis, or is DNA the Only Way? Limnol. Oceanogr-Meth 16, 209–264. doi: 10.1002/lom3.10240
Conover R.J. (1988). Comparative Life Histories in the Genera Calanus and Neocalanus in High Latitudes of the Northern Hemisphere, In: Boxshall G. A., Schminke H. K. (eds) Biology of Copepods. Developments in Hydrobiology, vol 47. Springer, Dordrecht. doi: 10.1007/978-94-009-3103-9_11
de Steur L., Hansen E., Gerdes R., Karcher M., Fahrbach E., Holfort J. (2009). Freshwater Fluxes in the East Greenland Current: A Decade of Observations. Geophys Res. Lett. 36, L23611. doi: 10.1029/2009GL041278
Falk-Petersen S., Mayzaud P., Kattner G., Sargent J. R. (2009). Lipids and Life Strategy of Arctic Calanus. Mar. Biol. Res. 5, 18–39. doi: 10.1080/17451000802512267
Falk-Petersen S., Pavlov V., Timofeev S., Sargent J. R. (2007). Climate Variability and Possible Effects on Arctic Food Chains: The Role of Calanus. In: Ørbæk J.B., Tombre T., Kallenborn R., Hegseth E., Falk-Petersen S., Hoel A.H., Eds., Arctic Alpine Ecosystems and People in a Changing Environment, Springer, Berlin, 147–166. doi: 10.1007/978-3-540-48514-8_9
Fleminger A., Hulsemann K. (1977). Geographical Range and Taxonomic Divergence in North Atlantic Calanus (C. Helgolandicus, C. Finmarchicus and C. Glacialis). Mar. Biol. 40, 233–248. doi: 10.1007/BF00390879
Frost B. W. (1974). Calanus Marshallae, a New Species of Calanoid Copepod Closely Allied to Sibling Species Calanus Finmarchicus and Calanus Glacialis. Mar. Biol. 26, 77–99. doi: 10.1007/BF00389089
Gabrielsen T. M., Merkel B., Søreide J. E., Johansson-Karlsson E., Bailey A., Vogedes D., et al. (2012). Potential Misidentifications of Two Climate Indicator Species of the Marine Arctic Ecosystem: Calanus Glacialis and C. Finmarchicus. Polar Biol. 35, 1621–1628. doi: 10.1007/s00300-012-1202-7
Hill R., Allen L., Bucklin A. (2001). Multiplexed Species-Specific PCR Protocol to Discriminate Four N. Atlantic Calanus Species, With an mtCOI Gene Tree for Ten Calanus Species. Mar. Biol. 139 (2), 279–287. doi: 10.1007/s002270100548
Hirche H., Hagen W., Mumm N., Richter C. (1994). The Northeast Water Polynya, Greenland Sea. Polar Biol. 14, 491–503. doi: 10.1007/BF00239054
Hirche H. J., Kattner G. (1993). Egg Production and Lipid Content of Calanus Glacialis in Spring: Indication of a Food-Dependent and Food-Independent Reproductive Mode. Mar. Biol. 117 (615), 22. doi: 10.1007/BF00349773
Hirche H. J., Kosobokova K. (2007). Distribution of Calanus Finmarchicus in the Northern North Atlantic and Arctic Ocean- Expatriation and Potential Colonisation. Deep Sea Res. Part II Top. Stud. Oceanogr. 54, 2729–2747. doi: 10.1016/j.dsr2.2007.08.006
Jaschnov W. A. (1970). Distribution of Calanus Species in the Seas of the Northern Hemisphere. Int. Rev. Gesamten Hydrobiol. 55, 197–212. doi: 10.1002/iroh.19700550203
Karnovsky N. J., Hobson K. A., Iverson S., Hunt G. L. (2008). Seasonal Changes in Dies of Seabirds in the North Water Polyna: A Multiple-Indicator Approach. Mar. Ecol. Prog. Ser. 357, 291–299. doi: 10.3354/meps07295
Kjellerup S., Dunweber M., Swalethorp R., Nielsen T. G., Moller E. F., Markager S., et al. (2012). Effects of a Future Warmer Ocean on the Coexisting Copepods Calanus Finmarchicus and C. Glacialis in Disko Bay, Western Greenland. Mar. Ecol. Prog. Ser. 447, 87–108. doi: 10.3354/meps09551
Lindeque P. K., Harris R. P., Jones M. B., Smerdon G. (1999). Simple Molecular Method to Distinguish the Identity of Calanus Species (Copepoda; Calanoida) at Any Developmental Stage. Mar. Biol. 133, 91–96. doi: 10.1007/s002270050446
Lindeque P. K., Harris R. P., Jones M. B., Smerdon G. (2004). Distribution of Calanus Spp. As Determined Using a Genetic Identification System. Sci. Mar. 68, 121–128. doi: 10.3989/scimar.2004.68s1121
Lindeque P. K., Hay S. J., Heath M. R., Ingvarsdottir, Rasmussen J., GR S., et al. (2006). Integrating Conventional Microscopy and Molecular Analysis to Analyse the Abundance Distribution of Four Calanus Congeners in the North Atlantic. J. Plankton Res. 28, 221–238. doi: 10.1093/plankt/fbi115
Møller E. F., Nielsen T. G. (2020). Borealization of Arctic Zooplankton—Smaller and Less Fat Zooplankton Species in Disko Bay, Western Greenland. Limnology Oceanogr. 65, 1175–1188. doi: 10.1002/lno.11380
Mayor D. J., Anderson T. R., Pond D. W., Irigoien X. (2009). Egg Production and Associated Losses of Carbon, Nitrogen and Fatty Acids From Maternal Biomass in Calanus Finmarchicus Before the Spring Bloom. J. Mar. Syst. 78 (4), 505–510. doi: 10.1016/j.jmarsys.2008.12.019
Nielsen T. G., Kjellerup s, Smolina I., Hoarau G., Lindeque P. K. (2014). Live Discrimination of Calanus Glacialis and C. Finmarchicus Females: Can We Trust Phenological Differences? Mar. Biol. 161, 1299–1306. doi: 10.1007/s00227-014-2419-5
Nielsen T. G., Kjellerup s, Smolina I., Hoarau G., Lindeque P. K. (2014b). Erratum to: Mar Biol 161:1299-1306. Mar. Biol. 161, 2697. doi: 10.1007/s00227-014-2419-5
Palumbi S. R., Benzie J. (1991). Large Mitochondrial DNA Differences Between Morphologically Similar Penaeid Shrimp. Molec Mar. Biol. Biotechnol. 1, 27–34. PMID: 1669002.
Parent G. J., Plourde S., Turgeon J. (2011). Overlapping Size Ranges of Calanus Species Off the Canadian Arctic and Atlantic Coasts: Impact on Species Abundances. J. Plankton Res. 33, 1654–1665. doi: 10.1093/plankt/fbr072
Parent G. J., Plourde S., Turgeon J. (2012). Natural Hybridization Between Calanus Finmarchicus and C. Glacialis (Copepoda) in the Arctic and Northwest Atlantic. Limnology oceanogr. 57 (4), 1057–1066. doi: 10.1002/lno.11725
Plourde A., Runge J. A. (1993). Reproduction of the Planktonic Copepod Calanus Finmarchicus in the Lower St. Lawrence Estuary: Relation to the Cycle of Phytoplankton Production and Evidence for a Calanus Pump. Mar. Ecol. Prog. Ser. 102, 217–227. doi: 10.3354/meps102217
Provan J., Beatty G. E., Keating S. L., Maggs C. A., Savidge G. (2009). “High Dispersal Potential has Maintained Long-Term Population Stability in the North Atlantic Copepod Calanus Finmarchicus,” in Proceedings of the Royal Society B: Biological Sciences, Vol. 276 (1655). 301–307. doi: 10.1098/rspb.2008.1062
R Core Team (2019). R: A Language and Environment for Statistical Computing (Vienna, Austria: R Foundation for Statistical Computing). Available at: https://www.R-project.org/.
Slagstad D., Ellingsen I. H., Wassmann P. (2011). Evaluating Primary and Secondary Production in an Arctic Ocean Void of Summer Sea Ice: An Experimental Simulation Approach. Progr. Oceangr. 90, 117–131. doi: 10.1016/j.pocean.2011.02.009
Smolina I., Kollias S., Poortvliet M., Nielsen T. G., Lindeque P., Castellani C., et al. (2014). Genome- and Transcriptome- Assisted Development of Nuclear Insertion/Deletion Markers for Calanus Species (Copepoda: Calanoida) Identification. Mol. Ecol. Resour. 14 (5), 1072–1079. doi: 10.3354/meps11398
Swalethorp R., Kjellerup S., Dünweber M., Nielsen T. G., Møller E. F., Rysgaard S., et al. (2011). Grazing, Egg Production, and Biochemical Evidence of Differences in the Life Strategies of Calanus Finmarchicus, C. Glacialis and C. Hyperboreus in Disko Bay, Western Greenland. Mar. Ecol. Prog. Ser. 429, 125–144. doi: 10.3354/meps09065
Tarling G. A., Freer J. J., Bana N. S., Belcher A., Blackwell M., Castellani C., et al. (2022). Can a Key Boreal Calanus Copepod Species Now Complete its Lifecycle in the Arctic? Evidence and Implications From Arctic Food Webs. Ambio 51, 333–344. doi: 10.1007/s13280-021-01667-y
Trudnowska E., Balazy K., Stoń-Egiert J., Smolina I., Brown T., Gluchowska M. (2020). In a Comfort Zone and Beyond—Ecological Plasticity of Key Marine Mediators. Ecol. Evol. 10:14067–14081. doi: 10.1002/ece3.6997
Unstad K. H., Tande K. S. (1991). Depth Distribution of Calanus Finmarchicus and Calanus Glacialis in Relation to Environmental Conditions in the Barents Sea. Polar Res. 10, 409–420. doi: 10.1111/j.1751-8369.1991.tb00662.x
Varpe Ø, Fiksen Ø, Slotte A. (2005). Meta-Ecosystems and Biological Energy Transport From Ocean to Coast: The Ecological Importance of Herring Migration. Oecologia 146, 443. doi: 10.1007/s00442-005-0219-9
Von Appen W. J., Schauer U., Somavilla R., Bauerfeind E., Beszcyznska-Moeller A. (2015). Exchange of Warming Deep Waters Across Fram Strait. Deep Sea Res. Part I Oceanogr. Res. Pap 103, 86–100. doi: 10.1016/j.dsr.2015.06.003
Wassmann P., Reigstad M., Haug T., Rudels B., Carroll M. L., Hop H., et al. (2006). Food Webs and Carbon Flux in the Barents Sea. Progr. Oceangr. 71, 232–287. doi: 10.1016/j.pocean.2006.10.003
Weslawski J., Hacquebord L., Stempniewicz (2000). Greenland Whales and Walruses in the Svalbard Food Web Before and After Exploitation. Oceanologia 42, 37–56.
Weydmann A., Kwasniewski S. (2008). Distribution of Calanus Populations in a Glaciated Fjord in the Arctic (Hornsund, Spitsbergen) - The Interplay Between Biological and Physical Factors. Polar Biol. 31 (9), 1023–1035. doi: 10.1007/s00300-008-0441-0
Wilson S. E., Steinberg D. K., Buesseler K. O. (2008). Changes in Fecal Pellet Characteristics With Depth as Indicators of Zooplankton Repackaging of Particles in the Mesopelagic Zone of the Subtropical and Subarctic North Pacific Ocean. Deep-Sea Res. Part II Top. Stud. Oceanogr. 55, 1636–1647. doi: 10.1016/j.dsr2.2008.04.019
Keywords: copepod, identification, barcode, molecular systematics, prosome length
Citation: Lindeque PK, Hann I, Parry HE, Cook KB, Lindley AJW and Mayor DJ (2022) Red Pigmentation Can Be Used to Reliably Distinguish Between Live Calanus finmarchicus and Calanus glacialis Females in the Fram Strait. Front. Mar. Sci. 9:906465. doi: 10.3389/fmars.2022.906465
Received: 28 March 2022; Accepted: 24 May 2022;
Published: 30 June 2022.
Edited by:
Sigrun Huld Jonasdottir, Technical University of Denmark, DenmarkCopyright © 2022 Lindeque, Hann, Parry, Cook, Lindley and Mayor. This is an open-access article distributed under the terms of the Creative Commons Attribution License (CC BY). The use, distribution or reproduction in other forums is permitted, provided the original author(s) and the copyright owner(s) are credited and that the original publication in this journal is cited, in accordance with accepted academic practice. No use, distribution or reproduction is permitted which does not comply with these terms.
*Correspondence: Penelope K. Lindeque, cGt3QHBtbC5hYy51aw==