- 1Department of Marine Zoology, Senckenberg Research Institute, Frankfurt, Germany
- 2School of Oceanography, University of Washington, Seattle, WA, United States
- 3Departamento de Estratigrafía y Paleontología, Universidad de Granada, Granada, Spain
- 4Departamento de Ecología y Geología, Universidad de Málaga, Málaga, Spain
- 5Monterey Bay Aquarium Research Institute, Moss Landing, CA, United States
The movement patterns of deep-sea bioturbational fauna are believed to be the result of the organism’s interactive response to the perceived spatial distribution of nutritional resources on the seafloor. To address this hypothesis, we examined the movement paths of Echinocrepis rostrata -a common epibenthic bioturbator echinoid in the northeast Pacific Ocean- through fractal analysis in order to characterize how they cover the seafloor during foraging. We used an 18-yr time series photographic record from 4100-m depth at an abyssal site in the eastern North Pacific (Sta. M; 34°50′N, 123°00′W; 4100 m depth). Echinocrepis rostrata paths showed low fractal values (1.09 to 1.39). No positive correlation between particulate organic carbon (POC) flux measured from sediment traps at 600 m and 50 m above bottom and fractal dimension (FD) values was observed. The movement of echinoids was characterized by high-speed periods, followed by slower speed periods and higher turning rates. These slow-speed periods were correlated with higher sinuosity values, slightly wider turning angles, and numerous cross-cuts. Based on visual estimation of seafloor phytodetritus coverage, we hypothesize that its small-scale distribution may be the primary determinant of echinoid feeding movement patterns rather than the bulk amount of nutrients. Finally, this finding reveals new insights into the morphological studies of trace fossils, indicating that trails of past echinoid trace makers could help to evaluate nutrient availability/distribution in the ancient deep-sea and help to decipher past climate-induced changes.
Introduction
Climate-induced changes (e.g., ocean warming, distribution of water masses) influence nutrient availability at the sea surface and subsequent particle export. Climate fluctuations contribute to water stratification, leading to variations in particulate organic carbon (POC) flux, affecting the deep-sea benthic community structure and ultimately the marine carbon cycle (Smith et al., 2013). The organic carbon that reaches the seafloor is the main food source for benthic fauna, controlling the diversity, abundance, size, and ethology of trace makers (i.e., rates of bioturbation) and carbon sequestration dynamics (Levin et al., 2001; Nathan et al., 2008; Vardaro et al., 2009). Epibenthic fauna feeding and movement have also proven to be major factors in incorporating detritus into the benthic food web and redistributing POC (Kaufmann and Smith, 1997).
Biogenic disturbance on and into the seafloor is of interest across many scientific disciplines within Earth and Life sciences due to its crucial influence on geochemical cycles and marine ecology (Smith et al., 2000). Bioturbation promotes multiple ways of priming and total remineralization of sedimentary organic matter (Aller and Cochran, 2019). Accordingly, if bioturbational activities are attenuated or even ceased due to climate-induced changes, then a significant shift in the carbon cycle could follow; for example, greater amounts of carbon could be released back into the water column instead of being sequestered deeper into the sediment (Vardaro et al., 2009). These links between POC flux and bioturbation allow the use of mobile trace maker behaviors and associated biogenic structures (lebensspuren–activity of benthic organisms imprinted on and within the sediment) as a proxy to provide information about the quality, quantity, and distribution of POC deposited on the seafloor over time (Gage and Tyler, 1991; Bell et al., 2013; Durden et al., 2020; Miguez-Salas et al., 2020). The movement patterns of epibenthic megafauna may reflect a number of structuring forces as they search for patchily-distributed food resources (Kaufmann and Smith, 1997). Bioturbators may exhibit variable movement patterns according to food availability and distribution, either as a non-random pattern that enhances their ability to localize patchy food resources or a composite pattern that has both non-random and random movements. Kaufmann and Smith (1997) proposed three qualitative movement patterns: 1) Run and Mill (organism moves relatively large distances in a short period of time, followed by a prolonged period of slower speeds and higher turning rates within a restricted area); 2) Loop (organism describes large loops across the seafloor); and 3) Run (organism runs in a predominantly unidirectional motion with minimal changes of speed and little or no recrossing of previously covered areas). However, the knowledge of how organic matter distribution controls the specific foraging motions (i.e., orientation, complexity, velocity) of mobile trace makers, and thus trace morphology, is still scarce (Koy and Plotnick, 2010).
Under certain environmental and taphonomical conditions, lebensspuren can be preserved in the geological record as trace fossils (Gage and Tyler, 1991; Smith et al., 2005). Thus, comparative analysis between lebensspuren and trace fossils provides a unique opportunity to evaluate deep-sea environmental changes at different time scales through Earth’s history (Miguez-Salas et al., 2020). The identification of diagnostic ichnological features to compare past and present traces and their paleoenvironmental implications are rarely attempted (Wetzel, 2008; Koy and Plotnick, 2010). In deep-sea environments, two major limiting conditions determine trace maker behavior: Rate of oxygenation and nutrient availability (Wetzel, 2008; Buatois and Mángano, 2011; Knaust and Bromley, 2012). The relationship between ichnological features and organic carbon distribution in the deep-sea, mainly focusing on echinoid trails, is comparatively better studied (Wetzel, 2008; Seike et al., 2020). For example, a considerable amount of organic matter (e.g., organic carbon content) is associated with guided meanders of large echinoid trails (Wetzel and Uchman, 2018). Although previous studies reveal that trails left behind by echinoids can represent searching behaviors and nutrient distribution (Sims et al., 2014), this research is still in its early stages. Approaching detailed characterizations of echinoid movement patterns to evaluate the relationship between present nutrient availability and foraging behaviors can be a challenge.
The bioturbation potential of Echinocrepis rostrata – a common deep-sea epibenthic echinoid bioturbator of the northeast Pacific Ocean – has been previously studied over 18-yr time-series photographic record (see Vardaro et al., 2009). This study found no increase in E. rostrata bioturbation over the 18 year study period despite an increase in population size, although there were periodic variations that correlated significantly with changes in POC flux. In more detail, a 1-month lagged relationship between average speed and POC flux (measured using sediment traps in the overlying water column) was observed (Vardaro et al., 2009). Here, we further evaluate that previous research, providing detailed evidence that E. rostrata exhibits a movement pattern controlled by small-scale phytodetritus distribution on the seafloor rather than bulk POC flux settling from overlying waters. Prior studies from the fossil record have revealed that systematic deposit-feeding strategy creates a higher fractal dimension in trace fossils, suggesting the organism feeds from as much of the sediment area as possible (Lehane and Ekdale, 2013; Lehane and Ekdale, 2016). Thus, fractal analysis was used here to examine the movements of E. rostrata and determine likely foraging strategies (e.g., selective feeding, systematic feeding, random pattern). Moreover, foraging strategies were compared with POC fluxes and small-scale nutrient distribution. The aim of the analyses is a precise characterization of echinoid movement, based on fractal analysis (Mandelbrot, 1983), to test if movement patterns can be used as deep-sea proxy of present POC supply to the seafloor and subsequently as a tool for paleoenvironmental applications.
Material and Methods
Study Data
Data were collected from the abyssal time-series site Station M (time-lapse image data sets from 1989 to 2007; Vardaro et al., 2009) in the north-eastern Pacific (34°50 N, 123°06 W, 4100 m water depth; Smith and Druffel, 1998) (Figure 1). The seabed is mainly composed of soft silty clay sediment (Kaufmann and Smith, 1997). Food falls are particulate organic carbon and detrital aggregates comprised primarily of phytodetritus and occasional gelatinous zooplankton (e.g., Smith et al., 2014). POC flux to the seafloor was measured using two Teflon©-coated McLane PARFLUX©-style sediment traps with 0.25-m2 openings moored at 50 m above bottom (mab; 4050 m water depth) and 600 mab (3500 m water depth). A collect time of ten days per sediment trap cup was performed, deployed 50 and 600 meters above bottom (mab). This measure of POC flux (i.e., through sediment traps) provides an estimate of bulk food availability to the seafloor community over time (Smith et al., 1994), and is known to correlate with megafauna abundance (Ruhl and Smith, 2004; Ruhl, 2008) and behavior (Durden et al., 2020). Details of sediment trap data collection and analysis can be found in Baldwin et al. (1998).
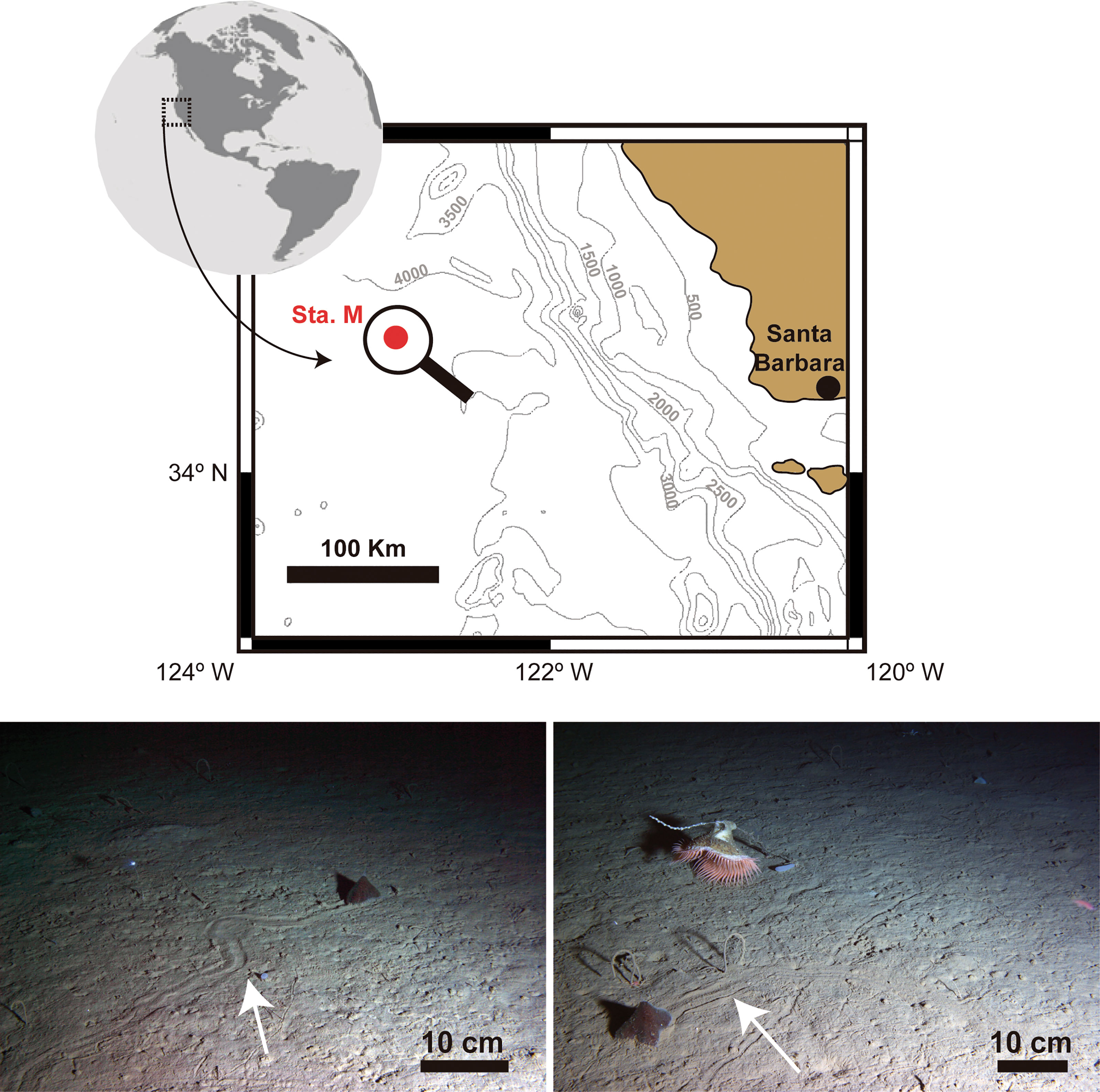
Figure 1 Detailed location of Station M in the Northeast Pacific (4000 m) with 500 m contours (slightly modified from Lampadariou et al., 2020). Image examples (below) of the seafloor at Sta. M with E. rostrata specimens (from Jason ROV and R/V New Horizon). The trail left in the sediment by E. rostrata foraging movement is indicated by white arrows.
A time-lapse camera (TLC) recorded the presence and activity of the Echinocrepis rostrata over a seafloor area of 20 m2 (Vardaro et al., 2009). The TLC consisted of a Benthos 377 camera mounted on a titanium frame at an angle of 31 degrees from horizontal with the lens ~2 m above the seafloor (Vardaro et al., 2009). The camera was equipped with a 28-mm Nikonos lens, providing angular coverage of 50 degrees in the horizontal and 35 degrees in the vertical plane, and held 400 feet of 35-mm color negative film (Fuji, type 8514, 500 ASA). The camera system was set at a 1-hour time-lapse interval and captured 27 image data sets of approximately 4 months each between 1989 and 2007. The still images were analyzed using a perspective-grid method (Wakefield and Genin, 1987). Each image was projected onto a flat surface and instances of individual urchins were digitized with a Science Accessories Corp.® electronic digitizer interfaced with a computer, using each 1 h interval as a step of the organism. Nine echinoid (E. rostrata) trails from 1993 to 2006 were selected based on: 1) variation in POC flux rates (from 0.8 to 16 mg/m2/day); 2) path morphology complexity; and 3) residence time (from 59 to 677 hours). The movements of these nine echinoids were digitally recreated by plotting the recorded positions on X–Y graphs (data from Vardaro et al., 2009).
Methodology
The recreated paths were classified based on their speed; an arbitrary threshold value of 100 mm/h was established to divide high and slow speed periods. Velocity was calculated by the modulus of the velocity vector as a function of the modulus of the position vector. For each individual path left by an echinoid, we calculated the following trail features: residence time (hours), cross-cuts (the result of the trace intersecting with itself), average trail width (mm), average trail speed (mm/h; based on Vardaro et al., 2009 data), total area covered (mm2) and average daily area covered (mm2/d). Additionally, path sinuosity (total path length divided by the minimum distance between the beginning and end of the path) and the turning radius of the angles between steps (degrees) were evaluated. Path tortuosity in this study was determined by calculating the ratio of track length to the actual linear distance traversed by the echinoid. High sinuosity is associated with pathways that have more turns relative to step length, indicating a more complex or twisted pattern of movement.
Fractal analysis (Mandelbrot, 1983) is used to characterize path complexity, quantify path morphologies and measure how completely the E. rostrata traces fill the seafloor area it occupies. The fractal dimensions (FD) of the studied traces were obtained by the box-counting method (e.g., Pérez-Claros et al., 2002; Kaurov, 2014). Although the box-counting method is in some cases less accurate than the Richardson method for low-complexity structures, fractal dimensions obtained by each method are generally similar (see Lutz and Boyajian, 1995). However, Richardson’s method is not suitable for situations where there are double points (the result of the curve intersecting with itself) such as Brownian motion projection on a plane or the traces analyzed here. Thus, fractal dimension estimation was expressly developed in Wolfram Mathematica 10.4.1.0 language, starting from an algorithm published by Kaurov (2014) that was adapted to incorporate the methodological improvements of Pérez-Claros et al. (2002). Then, the possible relationship between FD values and monthly POC flux (1-month lag intervals; up to 12 months in advance) were tested with non-parametric Spearman rank correlation. Trail features (residence time, cross-cuts, average trail width, average trail speed, total area covered, and average daily area covered), FD values, and POC flux (contemporary to the development of the trail) were also tested using non-parametric Spearman rank correlation.
To assess the influence of small-scale food distribution on bioturbation patterns, a visual analysis of E. rostrata movements and organic matter distribution was also conducted. The organic matter distribution visual analysis was developed in still images ranging from the time of trail formation to one month before. This time interval was selected because of the positive correlation between POC flux (lagged 1 month) and average trail speed found by Vardaro et al. (2009). The organic matter distribution analysis was conducted based on the identification of the nearby patches (within a radius of 20 cm from E. rostrata position during trail formation) for each echinoid specimen.
Results
The observed echinoid movement patterns were composed of straight and meandering paths. Straight echinoid movement patterns were characterized by high-speed periods when large distances were covered in a short time interval (green thick path in Figure 2; >100mm/h), followed by a prolonged period of slower movement with higher turning rates within a restricted area (red thin paths in Figure 2; < 100 mm/h). The slow-speed periods correlated with higher sinuosity values, slightly wider turning angles, and numerous cross-cuts (yellow stars in Figure 2). The FD of the nine specimens varied between 1.09 and 1.39. The fractal values were low but reflected a degree of relative complexity for each trace, becoming higher during the slow-speed periods when the echinoid path intersected itself and performed meandering movements.
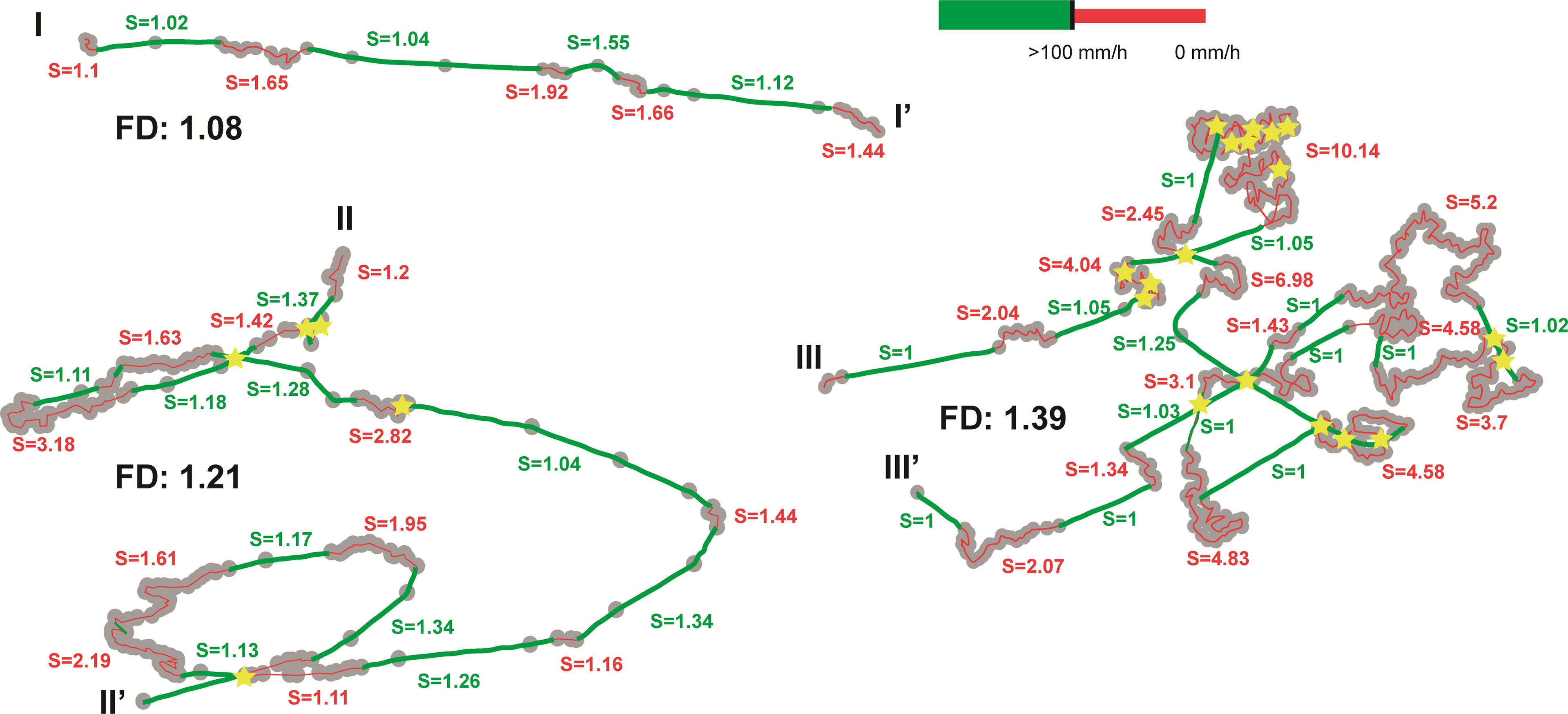
Figure 2 Echinocrepis rostrata digitized trails (I-I’, II-II’, III-III’) with their fractal dimension (FD) values. Red (thin) and green (thick) lines indicate low and high-speed periods respectively. Note that grey dots indicate the hourly position of the organism. Yellow stars indicate cross-cuts. S= tortuosity index.
The Spearman rank correlation between POC flux and FD showed no positive correlation at any time lags between 1 to 12 months (Supplementary Table 1), only a strong negative correlation observed 10 months prior to trail creation (Supplementary Table 1). FD is positively correlated with the number of cross-cuts and negatively correlated with the average width of the trail (Supplementary Table 2). Residence time, average trail speed, total area covered, and average daily area covered showed no correlation with the FD (Supplementary Table 2). A strong negative correlation between residence time and POC flux was observed (Supplementary Table 2). On the other hand, a positive correlation between the average daily area covered and POC flux was recorded (Supplementary Table 2). Number of cross-cuts, average trail speed, total area covered, and average width of the trail showed no correlation with the FD (Supplementary Table 2).
The visual analysis of small-scale organic matter distribution showed no consistent echinoid movement in relation to the patches deposited 1 month in advance. Reviewing the still images from a month prior until the moment in which the nine E. rostrata were examined (i.e., making the trail) produced the same result. There was no apparent relationship between previous organic patch distribution and E. rostrata movement. However, the deposition and distribution of organic matter available contemporaneously with foraging E. rostrata seemed to reflect a link with the echinoid movement pattern (Figure 3). The simplified sequence of movements appeared to unfold as follows (see example in Figure 3): High-speed periods (i.e., Run sensu Kaufmann and Smith, 1997) of E. rostrata movement were related to foraging behaviors (see Figure 3; T = 0h to T = 1h or T = 23h to T = 24h). Then, E. rostrata slower speed periods and higher turning rates were associated with the discovery of organic matter patches (see Figure 3; from T = 3h to T = 7h or from T = 11h to T = 15h). These slow-speed periods were associated with higher sinuosity values, meandering patterns, and feeding stops (i.e., Mill sensu Kaufmann and Smith, 1997).
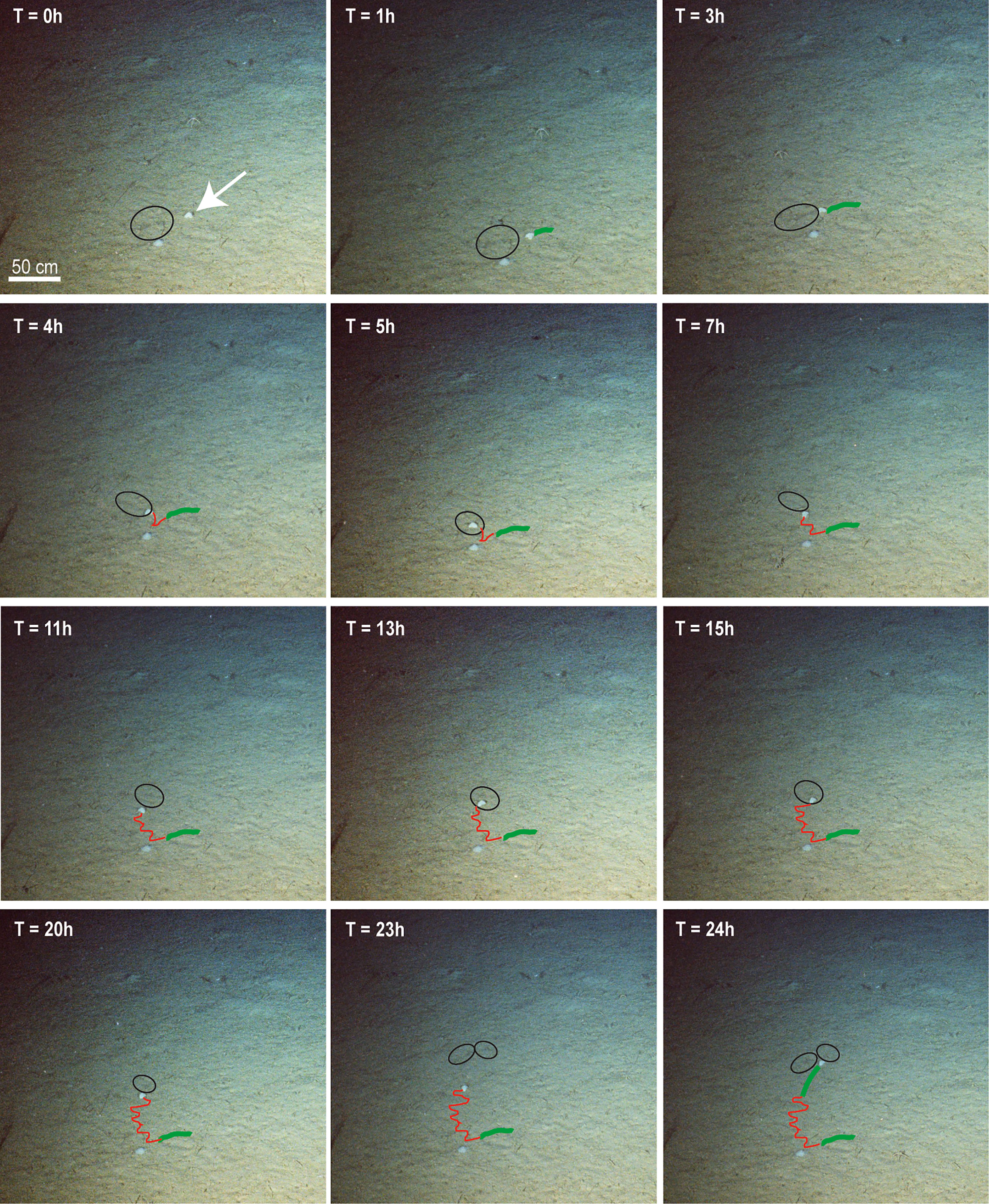
Figure 3 A sequence of images (hourly) that record the movement pattern of E. rostrata (white arrow) and the distribution of organic matter patches consumed by the echinoid (black circles). Note the Run (thick green line; T = 23h to T = 24h) and Mill (thin red line; T = 11h to T = 15h) behaviors of E. rostrata (sensu Kaufmann and Smith, 1997).
Discussion
The movement ecology paradigm of Nathan et al. (2008) and associated trails results from the answer to three main questions: 1) “why move?” 2) “how to move?” and 3) “where to move?”, as well as interactions with external environmental factors (Nathan et al., 2008). In the case of deposit feeders, an optimal foraging strategy implies that the organism ingests as much food-rich sediment as possible with minimum energetic cost, maximizing the coverage of the food-rich deposit (Levinton and Kelaher, 2004). In other words, maximizing the net energy intake while minimizing the time and energy spent foraging. Non-random movement patterns among the mobile epibenthic megafauna have been inferred from sediment traces (Kitchell, 1979), and were corroborated at Station M in the north-eastern Pacific (Kaufmann and Smith, 1997). Accordingly, if we follow Kaufmann and Smith (1997) qualitative movement patterns (Run and Mill, Loop, and Run), selective foraging motions (i.e., exploiting food patches) may be related to movement patterns such as the Run and Mill or Loop vs. continuous Run movement which is likely related to non-selective bulk ingestion (Kaufmann and Smith, 1997; Vardaro et al., 2009). Here, the obtained low FD values (i.e., no systematic deposit-feeding) for E. rostrata movement patterns reveal selective foraging motions. Thus, the studied echinoid seems to develop a Run and Mill strategy.
In the fossil record, the systematic deposit-feeding strategy interpreted for deep-marine trace makers generates trace fossils with a higher fractal dimension (up to 1.85) due to the organism feeding from as much of the sediment as possible (complete coverage of the sediment area) (Lehane and Ekdale, 2013). Thus, tightly spaced geometric attributes of deposit-feeding traces, which yield a high fractal dimension, are interpreted to be the result of a feeding pattern that maximizes the coverage of a food-rich area of the sediment (Lehane and Ekdale, 2013). The fractal values obtained here are considerably lower (1.39 maximum) than those obtained from systematic deposit-feeding strategies in the fossil record, suggesting heterogeneous distribution of benthic food and selective foraging motions while seeking patchy food resources. Accordingly, low fractal values of E. rostrata traces can be attributed to Run and Mill motions (high-speed between-patch movements and low-speed within-patch movements). When a nutritious patch is reached, movement behavior of the echinoid significantly shifts, exhibiting a characteristic sequence of phases: decreased speed, increased sinuosity, and a higher occurrence of cross-cut paths.
Our results are consistent with other experimental studies on mobile benthic fauna in patchy resource environments, where within-patch movements are correlated with smaller step lengths and higher tortuosity values (Koy and Plotnick, 2010). These authors also indicated that a major control on movement and trace morphology is the distribution of organic matter both within a patch and at the larger scale of the landscape. However, the assignment of behavioral significance to the movement patterns must be approached with caution. First, nutritious resources are not always uniformly distributed. For example, detrital aggregates at Sta. M are often patchy and can be randomly distributed on some spatial scales (Smith et al., 1994; Lauerman and Kaufmann, 1998). Also, the exact food quantity and quality of each patch is not possible to evaluate via image analysis; thus, the relative significance of these resources to the nutrition and the movement behavior of E. rostrata is difficult to ascertain. Finally, Run patterns may be segments of Run and Mill or large Loop patterns (Kaufmann and Smith, 1997).
It is unknown whether variation of food distribution at these spatial scales are important to E. rostrata or whether the present-time distribution of detrital aggregates is the primary motivation around which the movement behavior is structured. Durden et al. (2020) found that deposit-feeding megafauna (e.g., echinoids, large holothurians, and asteroids) did not alter their deposit feeding strategy in relation to the seasonality in detrital supply (i.e., changes in the amount of nutrients that reach the seafloor). Also, the guts of abyssal deposit feeders (e.g., E. rostrata) have recently been established as hotspots of organic matter that occupy one trophic level above detritus (Romero-Romero et al., 2021). Our results showed no positive correlation between POC fluxes and FD values. Nevertheless, the inverse correlation between POC flux and residence time indicates that with high POC fluxes, the echinoids spend less time in the area, as presumably there will be more narrowly spaced patches. Also, positive correlation between POC flux and average daily area covered stipulates that during periods of higher POC flux the echinoids will move faster in a straight line from patch to patch.
The visual analysis conducted here favors the interpretation that E. rostrata movement pattern is subject to nearby patch distribution (Figure 3). Our results seem to corroborate that parameters other than high or low prior POC fluxes control E. rostrata foraging movements. Thus, considering previous studies (e.g., Kaufmann and Smith, 1997; Vardaro et al., 2009; Koy and Plotnick, 2010; Durden et al., 2020; Romero-Romero et al., 2021), the obtained data, and that the nutrient content is not related to E. rostrata path complexity, phytodetritus distribution may be the dominant cause of echinoid movement. Analysis of environments with different levels of patch quality and variable travel times between patches must be conducted to resolve these uncertainties and attempt to more generally apply optimal foraging theory to deep-sea echinoids.
Implications for Echinoid Trace Fossils
In the ancient record, burrows in deep-sea environments show more complex patterns than in neritic ones (Seilacher, 2007; Fan et al., 2018). Looping traces have traditionally been interpreted as an uneconomical foraging strategy in which tracemakers inefficiently crossed their own paths (Seilacher, 1997). Instead, Seilacher (2007) proposed meandering traces as a reflection of more efficient foraging behavior, in which trace makers avoided areas they had already exploited. The presence of surfaces fully covered with echinoid trace fossils (e.g., Scolicia) suggests that the exploited area likely contained a considerable amount of organic matter (Wetzel and Uchman, 2018). The occurrence of large echinoid trails with guided meanders is best explained by an optimized exploitation of high-nutrient value benthic food (Kröncke, 2006; Wetzel, 2008). Studies from modern deep-sea sediments of the South China Sea revealed that large echinoid trails and guided meandering patterns were evocative of high-quality benthic food, while small echinoid trails seem to indicate a less favorable trophic situation (Wetzel, 2008). The results obtained at Station M offer new insights into the echinoid trace fossil analysis: 1) Straight “run” patterns likely relate to foraging periods (in search for food patches); 2) meandering patterns may indicate intense deposit-feeding periods; 3) path morphology seems to be more controlled by resource distribution rather than by nutrient abundance; and 4) larger trace width is related to higher nutrient abundance.
Comparison of living animal behaviors to the fossil record is not an easy matter. Any correlation of the present results with the fossil record must consider both the taphonomic bias and the unknown elapsed time required to produce the trace fossils. However, the time-lapse information and fractal analysis could improve paleoecological interpretations characterizing food availability on the seafloor during trace maker feeding activity. The obtained information will open new pathways as a comparison between detailed quantitative trace fossil studies (i.e., fractal analysis), and time-lapse information will offer stronger data on paleoenvironmental changes. Past POC flux fluctuations could be deciphered from changes in trace fossil morphologies through the geological record, enabling a novel approach to studying paleoenvironmental changes such as oxygen variations or water-mass stratifications.
Conclusions
The research conducted on Echinocrepis rostrata specimens -a common epibenthic bioturbator echinoid in the northeast Pacific Ocean- reveals that factors in addition to the flux of POC control their foraging motions. Fractal analysis showed no evidence of systematic deposit feeding strategies. Visual analysis of organic matter distribution revealed that E. rostrata movement patterns are influenced by the patchy distribution of organic detritus. Thus, the obtained results show that when E. rostrata encounter resource patches they increase their turning rates, decrease velocity, and produce meandering trails. Moreover, this finding reveals differences with respect to previous morphological studies of trace fossils, indicating that trails of past echinoid trace makers could be used to evaluate nutrient distribution in the ancient deep-sea.
Data Availability Statement
The original contributions presented in the study are included in the article/Supplementary Material. Further inquiries can be directed to the corresponding author.
Author Contributions
OM-S, CH, and FR-T contributed to the conception and design of the study. MV organized the database. JP-C performed the fractal analysis. All authors contributed to manuscript revision, read, and approved the submitted version.
Funding
The research is funded through a Humboldt Postdoctoral Fellowship from the Humboldt Foundation and PID2019-104625RB-100funded by MCIN/AEI/10.13039/501100011033
Conflict of Interest
The authors declare that the research was conducted in the absence of any commercial or financial relationships that could be construed as a potential conflict of interest.
Publisher’s Note
All claims expressed in this article are solely those of the authors and do not necessarily represent those of their affiliated organizations, or those of the publisher, the editors and the reviewers. Any product that may be evaluated in this article, or claim that may be made by its manufacturer, is not guaranteed or endorsed by the publisher.
Acknowledgments
The research of O. Miguez-Salas is funded through a Humboldt Postdoctoral Fellowship from the Humboldt Foundation. Data collection and involvement of Vardaro and Huffard was provided by the David and Lucile Packard Foundation. This research was supported by Rodríguez-Tovar grant PID2019-104625RB-100 funded by MCIN/AEI/10.13039/501100011033. We thank Dr. Gambi (Associate Editor) and the constructive comments of two anonymous referees who helped us to improve the clarity of the manuscript.
Supplementary Material
The Supplementary Material for this article can be found online at: https://www.frontiersin.org/articles/10.3389/fmars.2022.903864/full#supplementary-material
Supplementary Table 1 | Fractal dimension (FD) of studied Echinocrepis traces and POC flux (mg C m-2 d-1) values from the moment the trail was finished until 12 months before. The last row indicates Spearman’s rank correlation coefficient (statistic value) between FD and POC flux (mg C m-2 d-1) until 12 months before. Note that there is no strong positive correlation between these two parameters at any time lag.
Supplementary Table 2 | The trail features results of studied Echinocrepis traces (bold). The last two rows indicate Spearman’s rank correlation coefficient (statistic value) between trail features and: 1) fractal dimension (FD) of Echinocrepis traces; and 2) POC flux (mg C m-2 d-1) from the moment the trail was finished.
References
Aller R. C., Cochran J. K. (2019). The Critical Role of Bioturbation for Particle Dynamics, Priming Potential, and Organic C Remineralization in Marine Sediments: Local and Basin Scales. Front. Earth Sci. 7. doi: 10.3389/feart.2019.00157
Baldwin R. J., Glatts R. C., Smith K. L. Jr. (1998). “Particulate Matter Fluxes Into the Benthic Boundary Layer at a Long Time-Series Station in the Abyssal NE Pacific: Composition and Fluxes,” in Deep Sea Research Part II: Topical Studies in Oceanography, vol. 45 (United Kingdom: Elsevier Ltd.). 643–665. doi: 10.1016/S0967-0645(97)00097-0
Bell J. B., Jones D. O., Alt C. H. (2013). “Lebensspuren of the Bathyal Mid-Atlantic Ridge,” in Deep Sea Research Part II: Topical Studies in Oceanography, vol. 98. (United Kingdom: Elsevier Ltd.) 341–351. doi: 10.1016/j.dsr2.2012.09.004
Buatois L. A., Mángano M. G. (2011). Ichnology: Organism-Substrate Interactions in Space and Time (Cambridge: University Press).
Durden J. M., Bett B. J., Huffard C. L., Pebody C., Ruhl H. A., Smith K. L. Jr. (2020). “Response of Deep-Sea Deposit-Feeders to Detrital Inputs: A Comparison of Two Abyssal Time-Series Sites,” in Deep Sea Research Part II: Topical Studies in Oceanography, vol. 173. (United Kingdom: Elsevier Ltd.), 104677. doi: 10.1016/j.dsr2.2019.104677
Fan R. Y., Gong Y. M., Uchman A. (2018). Topological Analysis of Graphoglyptid Trace Fossils, a Study of Macrobenthic Solitary and Collective Animal Behaviors in the Deep-Sea Environment. Paleobiology 44 (2), 306–325. doi: 10.1017/pab.2018.1
Gage J. D., Tyler P. A. (1991). Deep-Sea Biology: A Natural History of Organisms at the Deep-Sea Floor (Cambridge: University Press).
Kaufmann R. S., Smith K. L. Jr. (1997). “Activity Patterns of Mobile Epibenthic Megafauna at an Abyssal Site in the Eastern North Pacific: Results From a 17-Month Time-Lapse Photographic Study,” in Deep Sea Research Part I: Oceanographic Research Papers, vol. 44. (United Kingdom: Elsevier Ltd.), 559–579. doi: 10.1016/S0967-0637(97)00005-8
Kaurov V. (2014). “Measuring Fractal Dimension of Natural Object From Digital Images,” in Mathematica Stack Exchange. Available at: https://mathematica.stackexchange.com/questions/13125/measuring-fractal-dimension-of-natural-objects-from-digital-images.
Kitchell J. A. (1979). Deep-Sea Foraging Pathways: An Analysis of Randomness and Resource Exploitation. Paleobiology 5 (2), 107–125. doi: 10.1017/S0094837300006400
Knaust D., Bromley R. (2012). Trace Fossils as Indicators of Sedimentary Environments (Amsterdam: Elsevier).
Koy K. A., Plotnick R. E. (2010). Ichnofossil Morphology as a Response to Resource Distribution: Insights From Modern Invertebrate Foraging. Palaeogeography Palaeoclimatology Palaeoecol. 292 (1-2), 272–281. doi: 10.1016/j.palaeo.2010.03.054
Kröncke I. (2006). Structure and Function of Macrofaunal Communities Influenced by Hydrodynamically Controlled Food Availability in the Wadden Sea, the Open North Sea, and the Deep-Sea. A Synopsis. Senckenbergiana Maritima 36 (2), 123–164. doi: 10.1007/BF03043725
Lampadariou N., Syranidou E., Sevastou K., Tselepides A. (2020). “Meiobenthos From Biogenic Structures of the Abyssal Time-Series Station in the NE Pacific (Station M),” in Deep Sea Research Part II: Topical Studies in Oceanography, vol. 173. (United Kingdom: Elsevier Ltd.), 104720.
Lauerman L. M., Kaufmann R. S. (1998). Deep-Sea Epibenthic Echinoderms and a Temporally Varying Food Supply: Results From a One Year Time Series in the NE Pacific. Deep Sea Res. Part II Top. Stud. Oceanogr. 45 (4-5), 817–842. doi: 10.1016/S0967-0645(98)00004-6
Lehane J. R., Ekdale A. A. (2013). Fractal Analysis of Graphoglyptid Trace Fossils. Palaios 28 (1), 23–32. doi: 10.2110/palo.2012.p12-081r
Lehane J. R., Ekdale A. A. (2016). Morphometric Analysis of Graphoglyptid Trace Fossils in Two Dimensions: Implications for Behavioral Evolution in the Deep Sea. Paleobiology 42 (2), 317–334. doi: 10.1017/pab.2015.52
Levin L. A., Etter R. J., Rex M. A., Gooday A. J., Smith C. R., Pineda J., et al. (2001). Environmental Influences on Regional Deep-Sea Species Diversity. Annu. Rev. Ecol. Systematics 32 (1), 51–93. doi: 10.1146/annurev.ecolsys.32.081501.114002
Levinton J., Kelaher B. (2004). Opposing Organizing Forces of Deposit-Feeding Marine Communities. J. Exp. Marine Biol. Ecol. 300 (1-2), 65–82. doi: 10.1016/j.jembe.2003.12.008
Lutz T. M., Boyajian G. E. (1995). Fractal Geometry of Ammonoid Sutures. Paleobiology 21 (3), 329–342. doi: 10.1017/S0094837300013336
Miguez-Salas O., Huffard C. L., Smith K. L. Jr., McGill P. R., Rodríguez-Tovar F. J. (2020). “Faunal Assemblage Changes, Bioturbation and Benthic Storms at an Abyssal Station in the Northeastern Pacific,” in Deep Sea Research Part I: Oceanographic Research Papers, vol. 160. (United Kingdom: Elsevier Ltd.), 103277. doi: 10.1016/j.dsr.2020.103277
Nathan R., Getz W. M., Revilla E., Holyoak M., Kadmon R., Saltz D., et al. (2008). A Movement Ecology Paradigm for Unifying Organismal Movement Research. Proc. Natl. Acad. Sci. 105 (49), 19052–19059. doi: 10.1073/pnas.0800375105
Pérez-Claros J. A., Palmqvist P., Olóriz F. (2002). First and Second Orders of Suture Complexity in Ammonites: A New Methodological Approach Using Fractal Analysis. Math. Geology 34 (3), 323–343. doi: 10.1023/A:1014847007351
Romero-Romero S., Miller E. C., Black J. A., Popp B. N., Drazen J. C. (2021). Abyssal Deposit Feeders are Secondary Consumers of Detritus and Rely on Nutrition Derived From Microbial Communities in Their Guts. Sci. Rep. 11 (1), 1–11. doi: 10.1038/s41598-021-91927-4
Ruhl H. A. (2008). Community Change in the Variable Resource Habitat of the Abyssal Northeast Pacific. Ecology 89 (4), 991–1000. doi: 10.1890/06-2025.1
Ruhl H. A., Smith K. L. Jr. (2004). Shifts in Deep-Sea Community Structure Linked to Climate and Food Supply. Science 305, 513–515. doi: 10.1126/science.1099759
Seike K., Shirai K., Kubota K., Ota Y., Sassa S. (2020). Does Trace Fossil Size Correspond With Burrower Population Density? An Example From the Modern Counterpart of the Trace Fossil Bichordites. Palaeogeography Palaeoclimatology Palaeoecol. 557, 109946. doi: 10.1016/j.palaeo.2020.109946
Sims D. W., Reynolds A. M., Humphries N. E., Southall E. J., Wearmouth V. J., Metcalfe B., et al. (2014). Hierarchical Random Walks in Trace Fossils and the Origin of Optimal Search Behavior. Proc. Natl. Acad. Sci. 111 (30), 11073–11078. doi: 10.1073/pnas.1405966111
Smith K. L. Jr., Druffel E. R. M. (1998). Long Time-Series Monitoring of an Abyssal Site in the NE Pacific: An Introduction. Deep Sea Res. Part II Top. Stud. Oceanogr. 45 (4-5). 573–586. doi: 10.1016/S0967-0645(97)00094-5
Smith K. L. Jr., Holland N. D., Ruhl H. A. (2005). “Enteropneust Production of Spiral Fecal Trails on the Deep-Sea Floor Observed With Time-Lapse Photography,” in Deep Sea Research Part I: Oceanographic Research Papers, vol. 52. (United Kingdom: Elsevier Ltd.) 1228–1240. doi: 10.1016/j.dsr.2005.02.004
Smith K. L. Jr., Kaufmann R. S., Baldwin R. J. (1994). Coupling of Near-Bottom Pelagic and Benthic Processes at Abyssal Depths in the Eastern North Pacific Ocean. Limnol. Oceanography 39 (5), 1101–1118. doi: 10.4319/lo.1994.39.5.1101
Smith C. R., Levin L. A., Hoover D. J., McMurtry G., Gage J. D. (2000). “Variations in Bioturbation Across the Oxygen Minimum Zone in the Northwest Arabian Sea,” in Deep Sea Research Part II: Topical Studies in Oceanography, vol. 47. (United Kingdom: Elsevier Ltd.), 227–257. doi: 10.1016/S0967-0645(99)00108-3
Smith K. L., Ruhl H. A., Kahru M., Huffard C. L., Sherman A. D. (2013). Deep Ocean Communities Impacted by Changing Climate Over 24 Y in the Abyssal Northeast Pacific Ocean. Proc. Natl. Acad. Sci. 110 (49), 19838–19841. doi: 10.1073/pnas.1315447110
Smith K. L., Sherman A. D., Huffard C. L., McGill P. R., Henthorn R., Von Thun S., et al. (2014). Large Salp Bloom Export From the Upper Ocean and Benthic Community Response in the Abyssal Northeast Pacific: Day to Week Resolution. Limnol. Oceanogr. 59, 745–757. doi: 10.4319/lo.2014.59.3.0745
Vardaro M. F., Ruhl H. A., Smith K. J. L. (2009). Climate Variation, Carbon Flux, and Bioturbation in the Abyssal North Pacific. Limnol. Oceanography 54 (6), 2081–2088. doi: 10.4319/lo.2009.54.6.2081
Wakefield W. W., Genin A. (1987). “The Use of a Canadian (Perspective) Grid in Deep-Sea Photography,” in Deep Sea Research Part A. Oceanographic Research Papers, vol. 34. (United Kingdom: Elsevier Ltd.), 469–478. doi: 10.1016/0198-0149(87)90148-8
Wetzel A. (2008). Recent Bioturbation in the Deep South China Sea: A Uniformitarian Ichnologic Approach. Palaios 23 (9), 601–615. doi: 10.2110/palo.2007.p07-096r
Keywords: deep-sea, bioturbation, echinoid movement, nutrient distribution, Pacific Ocean, station M
Citation: Miguez-Salas O, Vardaro MF, Rodríguez-Tovar FJ, Pérez-Claros JA and Huffard CL (2022) Deep-Sea Echinoid Trails and Seafloor Nutrient Distribution: Present and Past Implications. Front. Mar. Sci. 9:903864. doi: 10.3389/fmars.2022.903864
Received: 24 March 2022; Accepted: 27 April 2022;
Published: 23 May 2022.
Edited by:
Cristina Gambi, Marche Polytechnic University, ItalyReviewed by:
Simone Nunes Brandão, Universidade Estadual de Santa Cruz, BrazilNikolaos Lampadariou, Hellenic Centre for Marine Research (HCMR), Greece
Copyright © 2022 Miguez-Salas, Vardaro, Rodríguez-Tovar, Pérez-Claros and Huffard. This is an open-access article distributed under the terms of the Creative Commons Attribution License (CC BY). The use, distribution or reproduction in other forums is permitted, provided the original author(s) and the copyright owner(s) are credited and that the original publication in this journal is cited, in accordance with accepted academic practice. No use, distribution or reproduction is permitted which does not comply with these terms.
*Correspondence: Olmo Miguez-Salas, b2xtby5taWd1ZXotc2FsYXNAc2VuY2tlbmJlcmcuZGU=