- 1Department of Physics and William Mong Institute of Nano Science and Technology, The Hong Kong University of Science and Technology, Hong Kong, Hong Kong SAR, China
- 2Southern Marine Science and Engineering Guangdong Laboratory (Guangzhou), Guangzhou, China
- 3Materials Characterization and Preparation Facility (Guangzhou), The Hong Kong University of Science and Technology (Guangzhou), Guangzhou, China
- 4Department of Ocean Science, The Hong Kong University of Science and Technology, Hong Kong, Hong Kong SAR, China
Structural and physiochemical properties contribute to the biological adaptation of deep-sea animals to their harsh living environment but have hardly been investigated systematically. In the present study, we for the first time applied various material characterization techniques including transmission electron microscopy (TEM), scanning electron microscopy (SEM), energy-dispersive X-ray spectroscopy (EDS), X-ray diffraction (XRD), and Fourier-transform infrared (FT-IR) spectroscopy to investigate the shell microstructures and chemical composition of a deep-sea limpet Eulepetopsis crystallina collected from the Tiancheng hydrothermal vent field at a depth of around 2,700 m in the Southwest Indian Ocean. Analyses of shell microstructural morphology and diffraction patterns of E. crystallina explicitly revealed the layered structures, exfoliation characteristics, and crystallographic orientation of each layer’s unit cell which was tilted at a small angle sequentially. In comparison with ordinary shallow-water limpet Cellana toreuma shells, E. crystallina shells showed a unique chemical composition and contained pure calcite of calcium carbonate polymorph and the trace of phosphate originated from regional phosphatic sediments of the Southwest Indian Ocean. The further microscopic analyses indicated that the shell of the deep-sea limpet E. crystallina features integrated and untruncated layer structures with a compressed width, possibly owning to the ultra-high hydrostatic pressure, which confirmed the effects of the living environment on the shell microstructure of deep-sea animals.
Introduction
The microstructure and chemical composition of the deep-sea limpet shells have been investigated, which serves as the first step to investigate their formation mechanism and adaptation to the deep-sea environment (Nakai et al., 2006; Suzuki et al., 2010; Rodolfo-Metalpa et al., 2011; Li et al., 2015). The shell of limpet (Gastropoda class: aquatic snails) features as a composite biomaterial with multifunctional roles in providing mechanical strength and protective support to sustain the life activities of limpet (Denny, 2000; Harley et al., 2009; Johnson et al., 2019). Shallow-water limpets normally gather on the rocky shores where sea waves sweep over them. The flows exert a strong lift or drag force on the shell structures, and thus, shell microstructures need to withstand and adapt to the effects of water velocity in excess of 20 m/s (Denny, 2000). The main chemical composition of limpet shells is composed of calcium carbonate polymorphs and potential inorganic trace elements (Carroll and Romanek, 2008; Agbaje et al., 2018). The morphology, size, color, transparency, and even microstructures of the calcareous shells show remarkable distinction at different depths of the sea (Checa et al., 2006). Accordingly, compared with the common shallow-water limpet shells, the deep-sea limpet shells are expected to be distinctive in their microstructures and physicochemical characteristics because the animals usually live in an environment with relatively slow water velocity—but totally dark—and high hydrostatic pressure. Information on the shell microstructure and physiochemistry will provide insights into their functional properties and biomimetic applications that allow selection as prospective biomaterials.
The formation of limpet shell has been determined as a typical process of biomineralization (Weiner and Addadi, 2011; Oliveira et al., 2020), in which calcium carbonate evolves in different crystalline polycrystalline forms, such as calcite, aragonite, and spheraragonite, under natural conditions (Song et al., 2019). The various crystalline polycrystals of calcium carbonate are arranged in layers with unique patterns to form complex biomineral microstructures in limpet shells. In previous studies, the shallow-water limpet Patella pellucida (depth<27 m) featuring the blue-rayed translucent shell was proven to be an embedded hierarchical photonic architecture which displayed the light reflection from the exterior shell (Li et al., 2015). Besides, the shallow-water limpet Patella vulgate shell showed strong impact resistance, which depends on the two geometric parameters: apex thickness and the ratio of apex height to rim diameter (Harford et al., 2020). Up to now, the relevant research on limpet shells has mainly focused on the shallow-water species, and thus, there has been limited information on shell microstructures and chemical composition of highly structured biomineralized deep-sea animal shells.
In this study, we aimed to reveal the shell microstructures and chemical composition of the deep-sea limpet E. crystallina living at a depth of about 2,700 m of the Southwest Indian Ocean Ridge using various material characterization techniques including transmission electron microscopy (TEM), scanning electron microscopy (SEM), energy-dispersive X-ray spectroscopy (EDS), X-ray diffraction (XRD), and Fourier-transform infrared (FT-IR) spectroscopy. The TEM characterization is a proficient tool that allows scientists to investigate microstructural images and crystallographic details of nanomaterials (Suzuki et al., 2011; Suzuki et al., 2017; Wang et al., 2018; Ying et al., 2020). The width difference in layered structure between E. crystallina and C. toreuma shells due to their living environment was determined by TEM images. The SEM which scans a focused electron beam on the material surface can provide information about the surface morphology and layered microstructures of shells. Besides, the chemical composition of deep-sea and shallow-water limpet shells was explicitly determined. EDS is feasible for elemental analysis which analyzes the characteristic X-ray emission spectra from the individual element (Ying et al., 2021b). FT-IR detects the infrared spectrum of absorption or emission of samples and determines the bonding characteristics of inorganic materials. Non-destructive XRD offers detailed information of crystallographic structures, lattice parameters, and chemical composition. By EDS and FT-IR, the trace of phosphate was detected in shells of deep-sea limpet E. crystallina, but not in shallow-water limpet C. toreuma. Therefore, these exhaustive investigations on E. crystallina shells will give insight into shell microstructures and chemical compositions of deep-sea limpets, providing a wide range of possibilities for limpet living environments, shell formation mechanism, origin of invertebrate marine biomaterials, forced biomineralization, and extreme biomimetics (Li et al., 2015; Ehrlich and Nikolaev, 2017; Ehrlich, 2019; Lomovasky et al., 2020; Ehrlich et al., 2021).
Materials and methods
Collection of deep-sea limpets from the Southwest Indian Ocean and shallow-water limpets from Hong Kong
Deep-sea limpets Eulepetopsis crystallina were collected using the ROV Sea Dragon III onboard the R/V Dayangyihao from the Tiancheng hydrothermal vent field in the Southwest Indian Ocean during the COMRA DY52nd cruise in April 2019 (see details in Sun et al., 2020) (Sun et al., 2020). The deep-sea limpet E. crystallina samples were stored at –80°C after collections. Samples were directly fixed in pure ethanol immediately after arrival onboard. Soft tissues were removed from the shells with tweezers in the laboratory; the remaining shells were immersed in 0.2% of bleach and gently shaken at a rotation speed of 250 rpm at room temperature for 2 h to further remove the residual of soft tissues, and the shaking process was repeated with 0.1% of bleach and rinsed with Milli-Q waters.
Shallow-water limpets Cellana toreuma were manually collected from the shore of Clear Water Bay in The Hong Kong University of Science and Technology, Hong Kong, in October 2019. The shallow-water limpet C. toreuma samples were stored at -80°C after collections. After arrival onboard, samples were rinsed with water and fixed in pure ethanol immediately on the collection site. Soft tissues were removed from the shells with tweezers in the laboratory, the remaining shells were immersed in 0.2% of bleach and gently shaken at a rotation speed of 250 rpm at room temperature for 2 h to further remove the residual of soft tissues, and the shaking process was repeated with 0.1% of bleach and rinsed with Milli-Q water.
Besides, the limpet E. crystallina and C. toreuma samples were stored at -80°C and then conducted for microstructure characterization and chemical composition analysis after the ROV Sea Dragon III arrived at the home port and treatments in the laboratory (approximately 2 months later).
The microstructure characterization
For the samples characterized by TEM and SEM, the ultra-thin lamella samples of shells of both the deep-sea limpet E. crystallina and shallow-water limpet C. toreuma were prepared through the milling and fabrication processes using the FEI dual-beam FIB/FESEM system (Helios G4 UX, Waltham, United States), which enabled cross-sectional milling and imaging to reveal microstructures below the surfaces. The equipped drift compensated frame integration (DCFI) and Thermo Scientific SmartScan™ modes can realize integrated sample cleanliness management and dedicated imaging modes, which offered artifact-free imaging. The low-dose condition was applied in the FIB process to eliminate the potential Ga+ beam-induced damage. The selected area electron diffraction (SAED) and high-resolution TEM (HRTEM) images that disclosed the crystallographic information and layered microstructures of the deep-sea and shallow-water limpet shells were captured by a JEOL 2010F field emission gun transmission electron microscope working at 200 kV with the point-to-point resolution of 1.2 Å. All TEM images were captured under electron beam fux with a current density of less than 20 pA/cm2, in order to protect the sample from electron irradiation damage such as knock-on damage and thermal effect (Ying et al., 2021c). The morphology images were provided by the JEOL-6390 SEM and FEI dual-beam FIB/FESEM system (Helios G4 UX, Waltham, United States) working at 20 kV.
The chemical composition analysis
The EDS spectra of the chemical compositions of deep-sea and shallow-water limpet shells were characterized using the EDS system and using a 65-mm2 Oxford Silicon Drift Detector (SDD, Abingdon, UK) – X-Max with the resolution of 133 eV which was directly connected with HRTEM. The XRD spectra of deep-sea and shallow-water shells were characterized using a Powder X-ray PANalytical diffractometer (X’Pert Pro, Malvern, UK) equipped with a Cu Kα1 X-ray emitting source, λ = 1.5406 Å. The infrared spectra of deep-sea and shallow-water shells were characterized using the Bruker Vertex 70 Hyperion 1000 FT-IR spectrometer over a range of 4000 cm−1 to 400 cm−1 at a 2-cm−1 resolution and averaging 64 scans.
Results
Optical microscope
The appearance, morphology, and exfoliation properties of E. crystallina and C. toreuma shells were first investigated under the optical microscope (Figure 1). The appearances of the E. crystallina shell and the C. toreuma shell are shown in Figures 1A, B where the E. crystallina shell exhibited a semitransparent shell structure while the C. toreuma shell featured a brown and opaque structure. Under the optical microscope, the E. crystallina shell exhibited an uneven and semitransparent lamellar structure (Figure 1C) while the C. toreuma shell showed a smooth and dense surface (Figure 1D). The interference in Figure 1C results from the overlapped and uneven layer structures of E. crystallina shells. The interference fringes shown in the optical image of E. crystallina shell surface (Figure 1C) indicated that its distinct layered microstructure has endowed it with this light reflection property. In Figure 1D, the complete triangular shape is attributed to cleavage which is the tendency preference of crystalline materials to split along defined crystallographic structural planes. The observed smooth repeating surfaces under the optical microscope resulted from the regular locations of atoms and ions in crystalline materials. When the bonds in certain directions are weaker than those in others, the crystal will tend to split along these weakly bonded planes, which leads to the complete and smooth triangular shape of shallow-water limpet C. toreuma shell. These flat breakages are termed “cleavage.” Due to the resolution limit, the more detailed microscopic structure cannot be observed by optical microscopy; thus, further observation will be required with electron microscopy. Besides, several-layer or even single-layer lamella of the E. crystallina shell can be readily prepared by mechanical exfoliation (pointed out by arrows in Figure 1E) in which the fresh surface of layered shells was torn off using an adhesive tape. This exfoliation phenomenon was attributed to the very weak van der Waals interaction between the layers of the deep-sea limpet E. crystallina shell. However, this unique exfoliation availability was not achieved in C. toreuma shells (pointed out by arrows in Figure 1F).
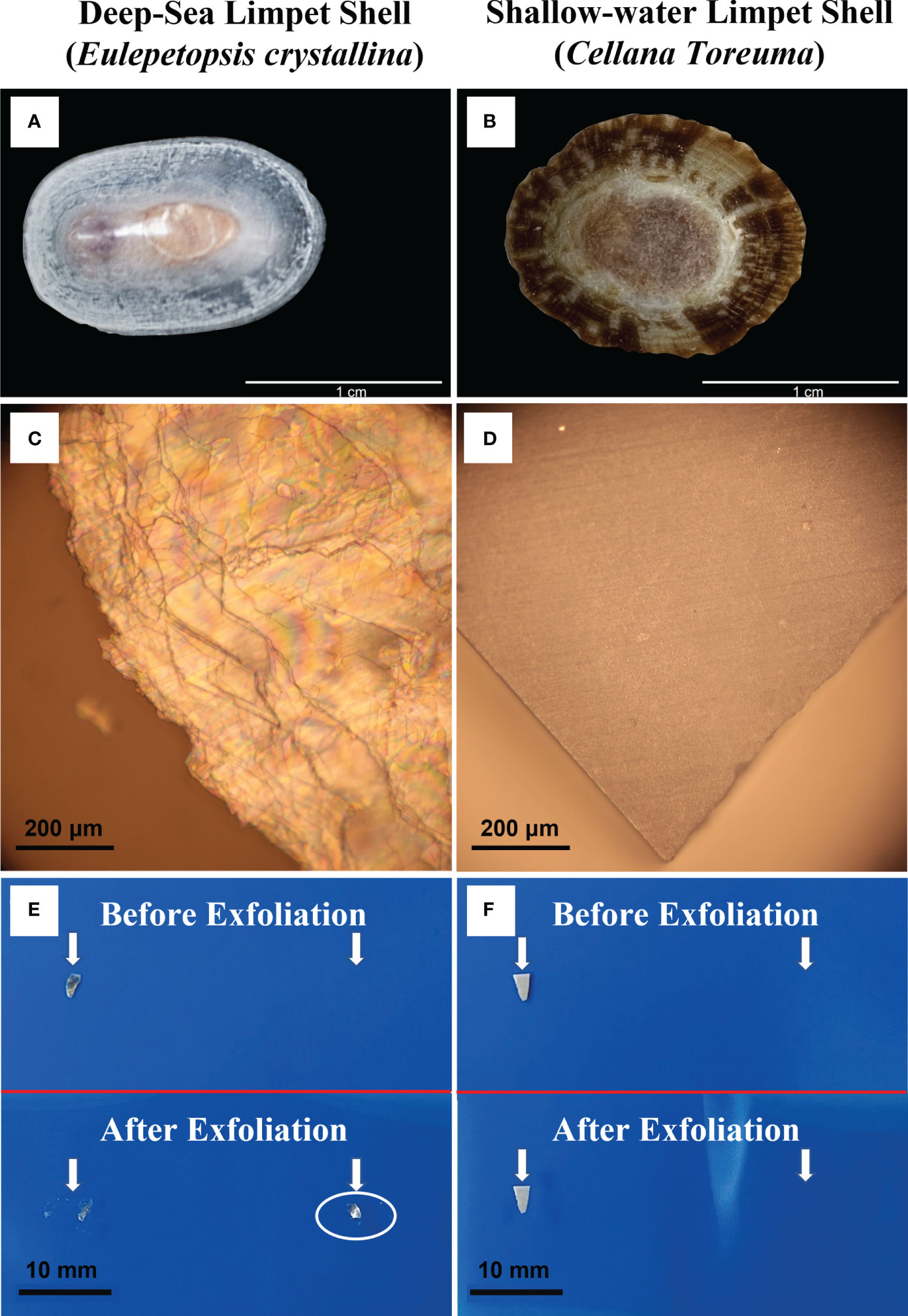
Figure 1 Optical images of (A) deep-sea limpet E. crystallina shell; (B) shallow-water limpet C. toreuma shell; (C) E. crystallina shell surface showing evident interference fringes; (D) C. toreuma shell dense and smooth surface; (E) visible exfoliation property of deep-sea limpet E. crystallina shell structure before and after exfoliation, and (F) shallow-water limpet C. toreuma shell structure before and after exfoliation. [Photos Figure 1A courtesy of Dr. Chenggang Liu].
TEM
The high-magnification morphology and microstructures of E. crystallina shells shown in the TEM images at different magnifications (Figure 2) explicitly revealed the microstructural details and exfoliation availability of layered structures. In the plane-view TEM image (Figure 2A), a thin layer can be exfoliated from the base and subsequently folded on the top layer (outlined by yellow dot line), which can be confirmed by the Moiré patterns at the overlaying area. The Moiré patterns are the interference patterns that are produced when the crystalline nanosheet pattern is overlaid on the adjacent similar pattern, and thus they are the most direct features for exfoliation properties under microscopic imaging (Jiang et al., 2014). In the cross-sectional TEM images (Figures 2B, C), alternate inorganic–organic layers protected by the outermost organic periostracum were clearly observed in the E. crystallina shell. Due to mass-thickness contrast, the inorganic crystal parts were observed as thicker and darker layers and separated by the organic parts as thinner and brighter layers. In the TEM image of the cross-sectional areas of the C. toreuma limpet shell (Figure S1), the multilayered microstructure was clearly revealed, and the average thickness () of organic matrices of the C. toreuma limpet shell was more inconspicuous and much thinner than those of deep-sea limpet E. crystallina shells ) shown in Figure 2C, Figure S1. In the high-resolution TEM inset image (Figure 2D), some lattice fringes were found in interfacial organic layers, indicating the presence of some inorganic nanometric crystals in the organic layer of E. crystallina shells. However, the lattice fringes of nanometric crystals were not found in the thin organic matrices of shallow-sea limpet C. toreuma shells.
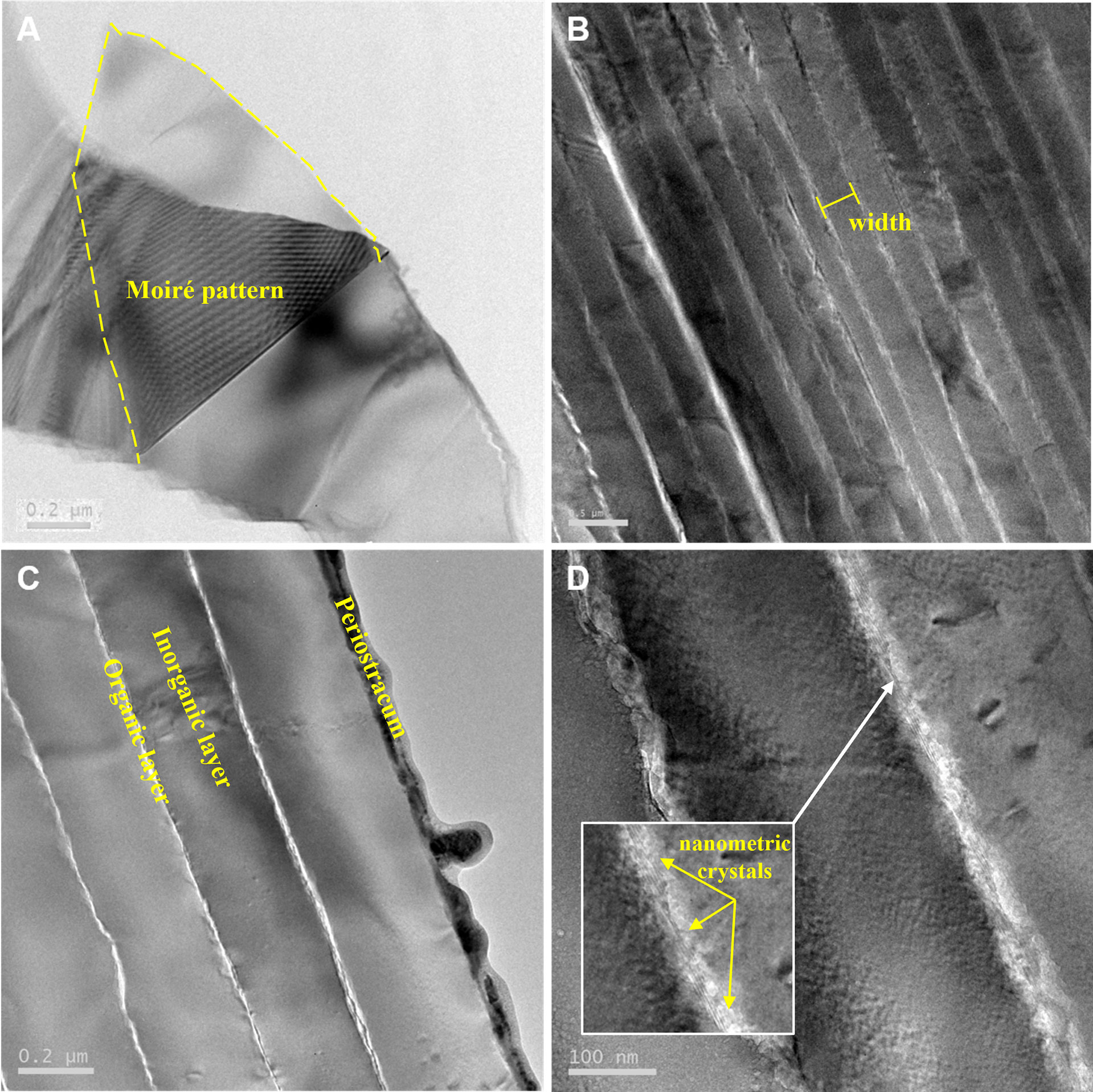
Figure 2 TEM images of deep-sea limpet E. crystallina shells at a depth of 2,700 m in the Southwest Indian Ocean. (A) Plane-view image showing the exfoliation property of the E. crystallina shell; (B–D) cross-sectional images showing multilayered microstructures of E. crystallina shells at different magnifications.
SEM
The cross-sectional view of the C. toreuma shell (Figures 3A, B) exhibits a trilayered structure: the outermost thin organic matrix (periostracum) and two calcified layers (the outer prismatic layer and the inner nacreous layer). Compared with the E. crystallina shell, the C. toreuma shell features the different microstructures of prismatic layers. The crystal structures of the prismatic layers in the E. crystallina shell (Figure 3E) exhibit the integrated and untruncated layer structures without any cracks. In comparison, the prismatic layers of C. toreuma shells are made up of elongated calcified crystals, organized in the brick wall-like structures which feature interstitial cracks between adjacent crystal blocks (Figure 3D) (Marie et al., 2012). In Figures 3C, G, it is evident that the average width of inorganic crystal structures in the prismatic layer of E. crystallina shells () is far smaller than those of the C. toreuma shell (), which is attributed to the ultra-high compressive force exerted by the hydrostatic pressure in the deep-sea environment at a depth of 2,700 m.
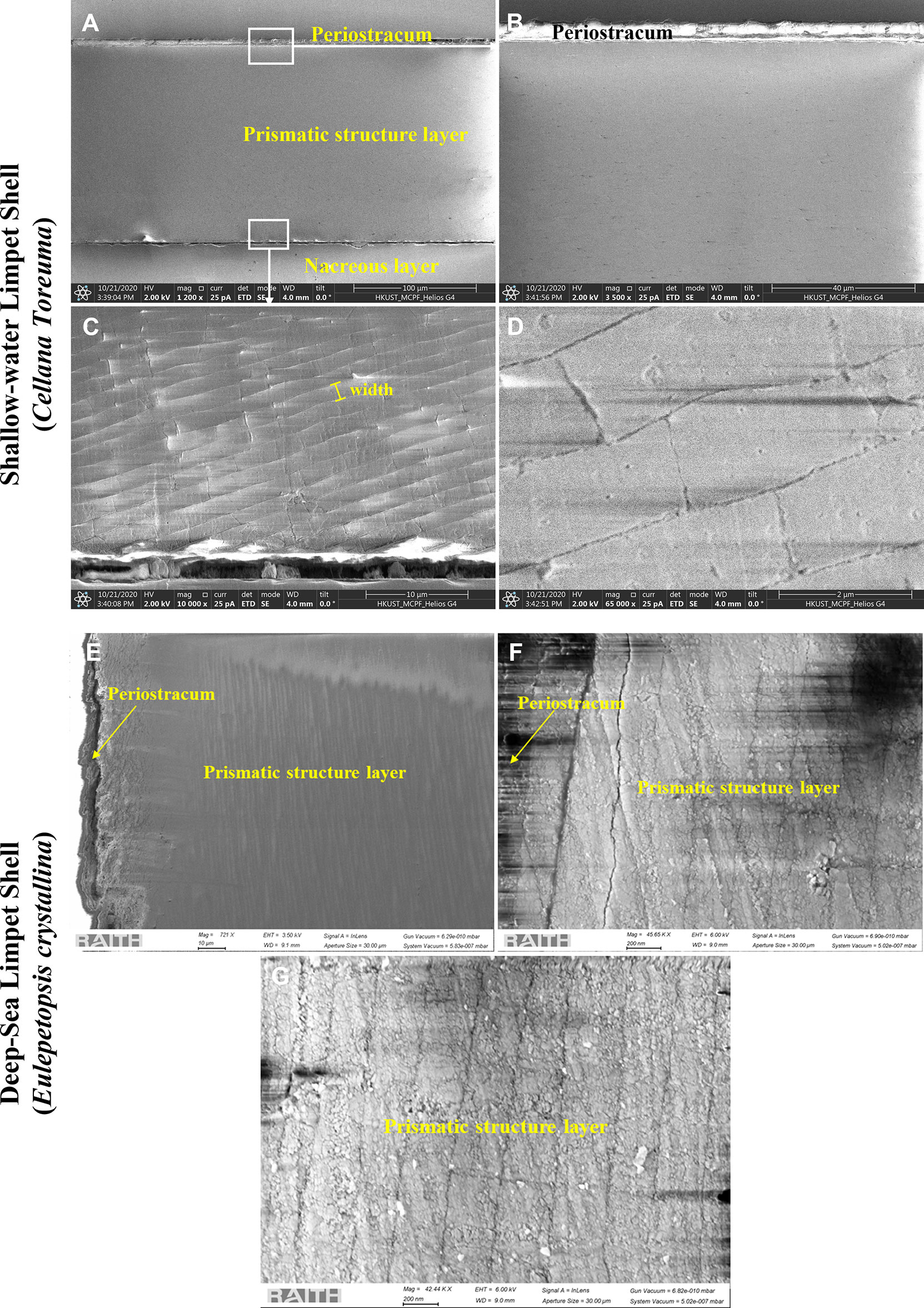
Figure 3 SEM images of the cross-sectional areas of shallow-water limpet C. toreuma shells prepared by FIB: (A) morphology of the cross-sectional layered structures. (B) The enlarged details of the relative area pointed by white rectangular frame (upper) in panel (A). (C) The enlarged details of the relative area pointed by white rectangular frame (bottom) in panel (A). (D) The high-magnification details of the prismatic structure layer in panel (C); SEM images of cross-sectional areas of deep-sea limpet E. crystallina shells prepared by FIB: (E) morphology of the cross-sectional layered structures. (F) The enlarged details of the interface between the periostracum and prismatic structure layer. (G) The enlarged details of the prismatic structure layer.
SAED
The selected-area electron diffraction (SAED) pattern was performed by TEM to determine the crystal structure and crystallographic orientation of the layered structure of E. crystallina shells. Figure 4A exhibits a typical cross-sectional TEM image of the inorganic phase of E. crystallina shell, and the outermost inorganic structure of the prismatic layer outlined by yellow lines is selected for SAED characterization. Figure 4B shows the corresponding SAED pattern, where the diffraction spots confirm that the prismatic layer of E. crystallina shell is crystalline. The indexed SAED pattern verifies that the outermost inorganic layer has a trigonal calcite-CaCO3 structure where the spacing of reciprocal lattice in Figure 4B matches with the calcite-CaCO3 structure and the longitudinal orientation of the elongated outermost layer labeled by the white arrow is along the [0 0 0 6] direction. The unit cells of calcite are aligned in a texturized arrangement in the way that the c-axes are perpendicular to the lamella surface and others are horizontal to the lamella surface. Besides, the resulted lattice parameters are a = 4.98 Å, c = 17.07 Å, which are consistent with literature values, and the calcite crystals orient along the [] zone axis (shown by the schematic model in the inset of Figure 4A) (Aizenberg et al., 1997). In Figure 5, in addition to the outermost calcite layer (labeled 1), four more SAED patterns of the inner layers (labeled 2–5) are recorded as shown in Figures 5C–F to investigate crystal orientation in these layers. Each diffraction spot in TEM images corresponded to a specifically satisfied diffraction condition of the sample’s crystal structure. If the crystal is tilted, different diffraction conditions will be satisfied and diffraction spots will change. Here, for the layered structures 1–5, they exhibit different SAED patterns, which indicated that the crystal orientations of adjacent layered structures 1–5 are not aligned and tilted at a small angle sequentially. In addition, the information of SAED patterns of the shallow-water limpet C. toreuma shell (Figures 5G–J) was added and further compared with deep-sea limpet E. crystallina shells. In Figures 5H–J, SAED patterns verified that the crystal orientations of adjacent layered structures 1–3 were not aligned in a small tilt angle. For example, the diffraction patterns of layer 1 and neighboring layer 2 have no observable orientation relationship because C. toreuma limpet shells consist of two calcium carbonate crystal polymorphs and SAED patterns are no longer single crystal diffraction patterns, which will be discussed later together with the results of chemical composition.
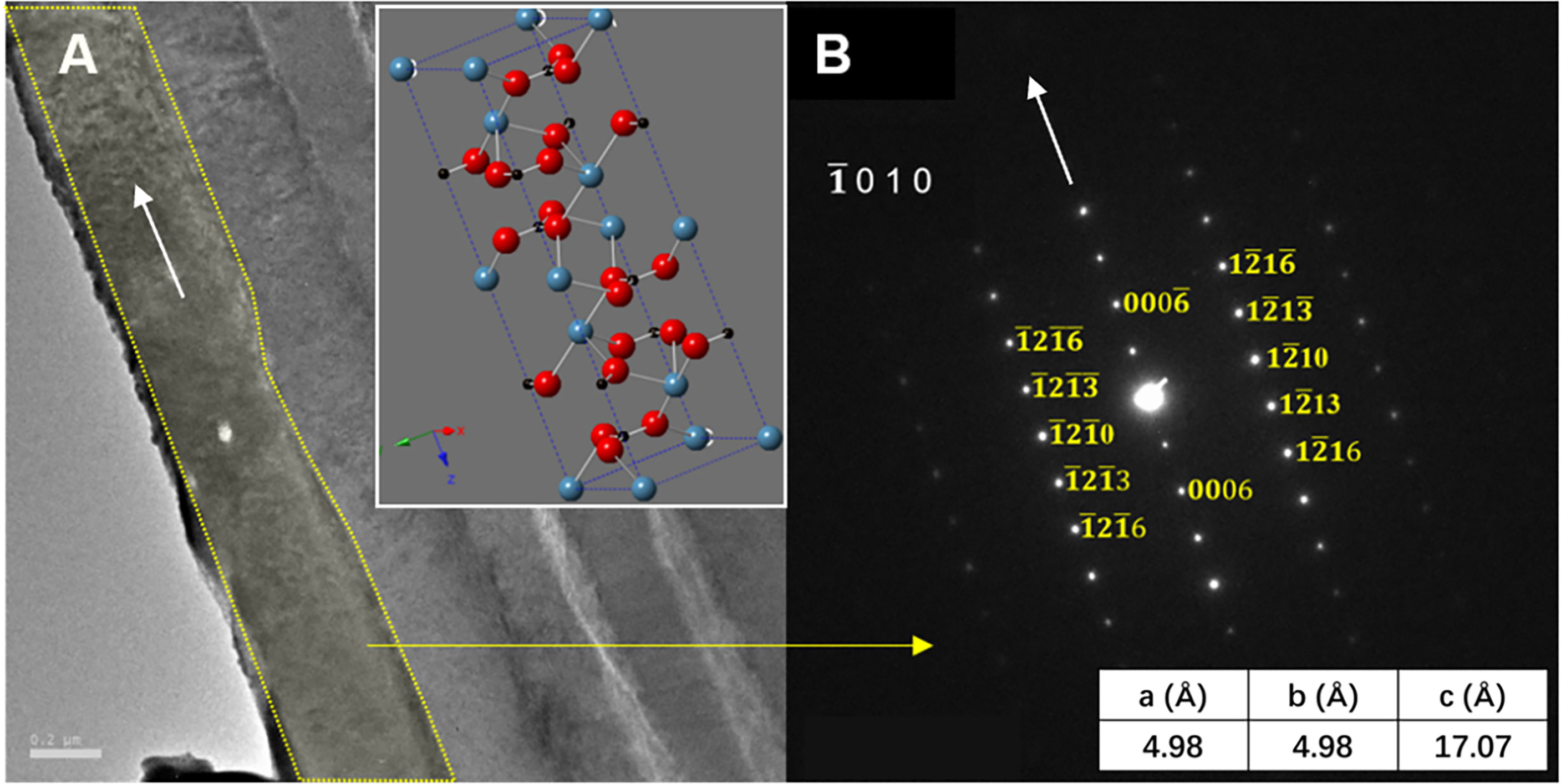
Figure 4 (A) The TEM image showing the crystallography of the inorganic phase of the deep-sea limpet E. crystallina shell and (B) SAED pattern of the marked area of the inorganic phase of the deep-sea limpet E. crystallina shell.
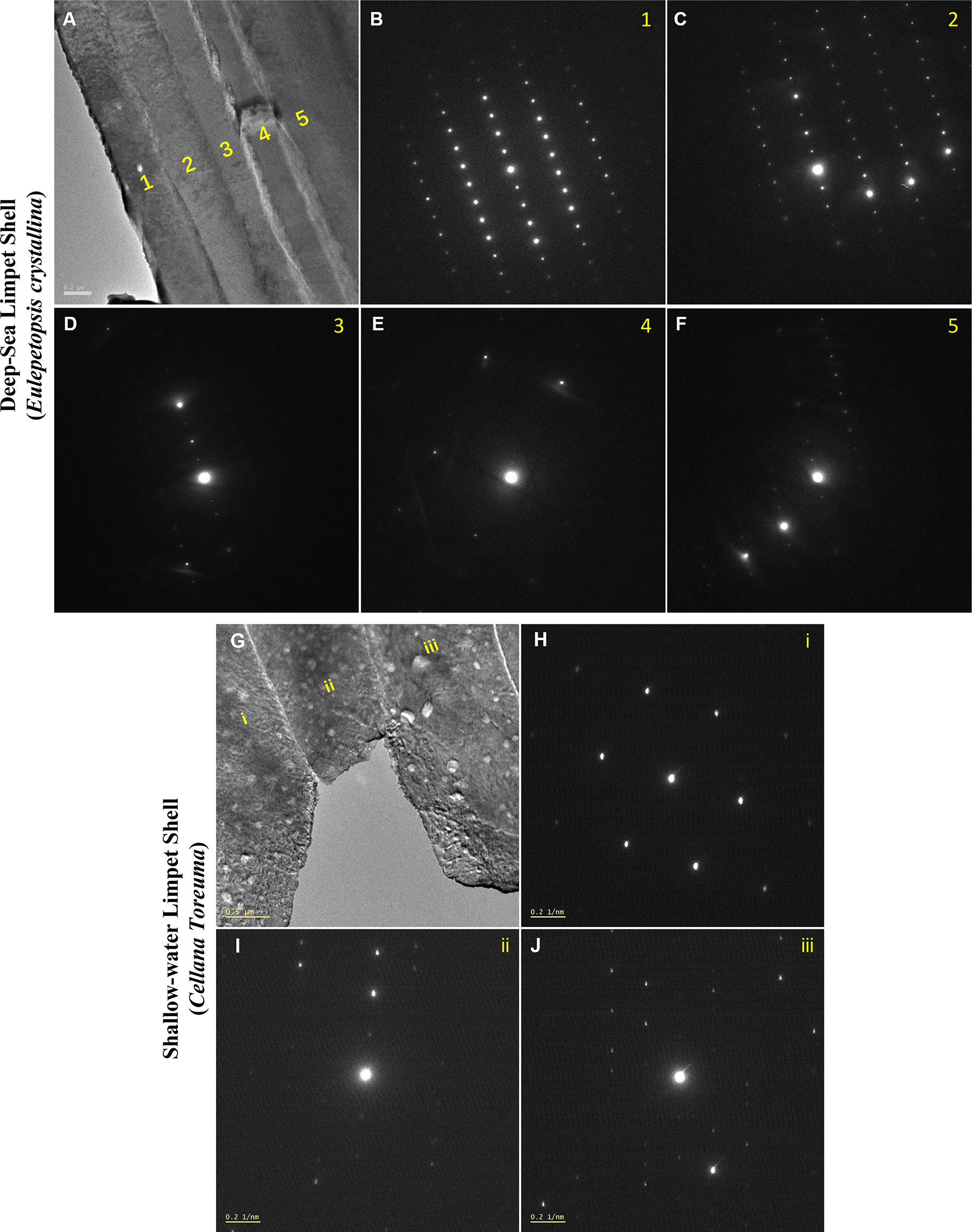
Figure 5 (A) The TEM image of the cross-sectional layered microstructures of deep-sea limpet E. crystallina shell. (B–F) Selected-area electron diffraction (SAED) patterns of the corresponding numbered layers 1–5 of deep-sea limpet E. crystallina shell in panel (A). (G) The TEM image of the cross-sectional layered microstructures of shallow-water limpet C. toreuma shell. (H–J) SAED patterns of the corresponding numbered layers i–iii of shallow-water limpet C. toreuma shells in panel (G).
EDS
The chemical composition refers to the chemical elements that make up the target material, which would clarify the marine living environment, cross-sectional layered structures, and crystalline arrangements. In general, the main chemical composition of marine animal shells is composed of carbonate minerals and some inorganic trace elements (Islam et al., 2011; Hamester et al., 2012; Wu et al., 2017). Regarding EDS characterization, the characteristic energy distribution spectra of X-rays can be obtained when samples are irradiated by electron beam, and thus EDS can determine most of elements in the sample qualitatively (Ying et al., 2021a). The elemental analysis of E. crystallina and C. toreuma shells was detected by energy-dispersive X-ray spectroscopy, and the EDS spectra (Figure 6) confirmed the presence of carbon (C), oxygen (O), magnesium (Mg), and calcium (Ca) in both E. crystallina and C. toreuma shells. Compared with C. toreuma shells (Figure 6B), E. crystallina shells uniquely obtained the trace of phosphorous (P) which originated from the potential phosphorite and phosphatic sediments in phosphorus-rich deep-sea vent ecosystems of the Southwest Indian Ocean (Figure 6A) (Baturin, 1982; Martin et al., 2018). Besides, the copper (Cu) signal originated from the TEM copper grid that was used for EDS measurements.
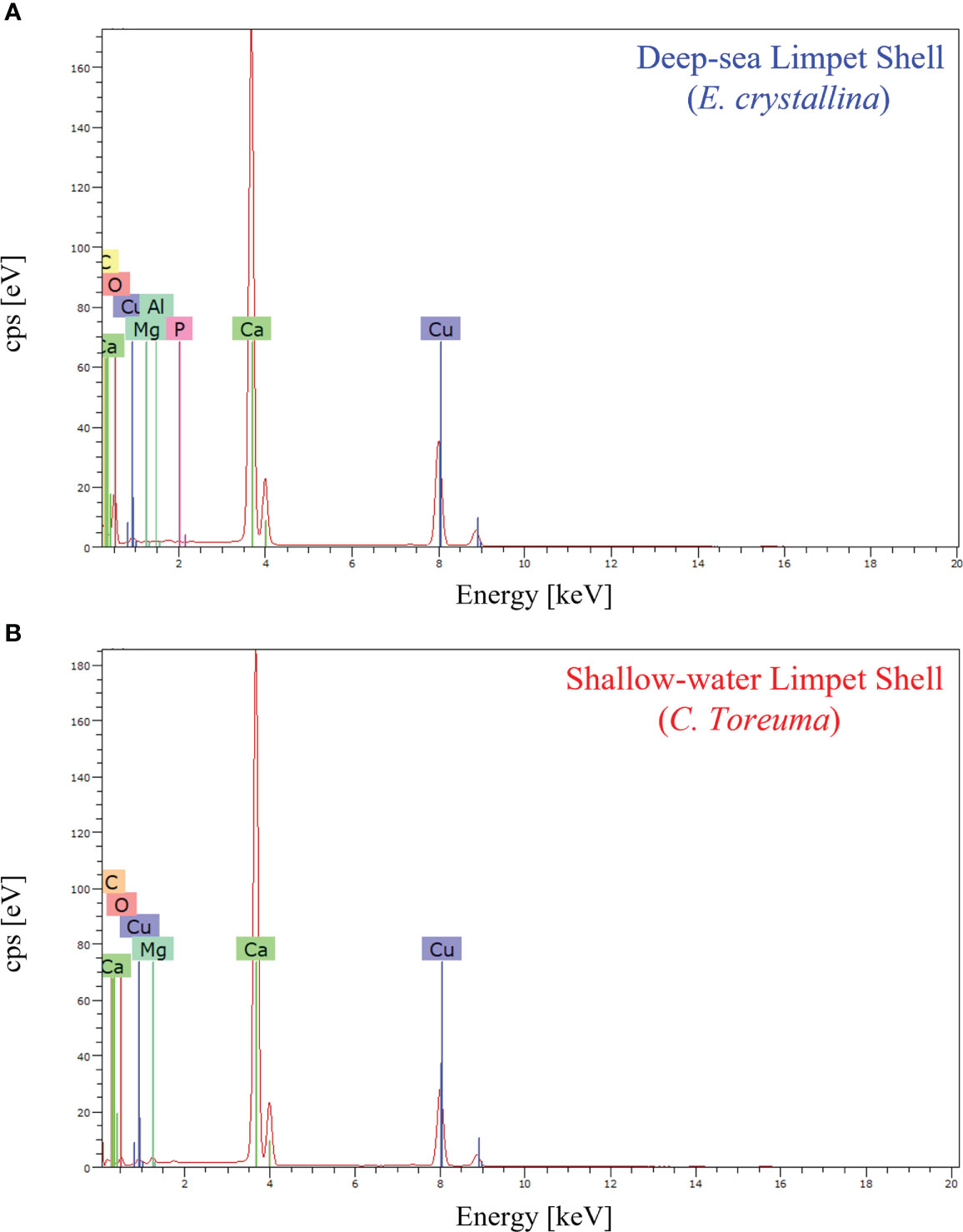
Figure 6 The EDS spectra of (A) deep-sea limpet E. crystallina shells containing Ca, C, and O as the main components and P and Al as unique trace elements and (B) shallow-water limpet C. toreuma shells containing Ca C, and O as the main components, where the Cu signal generates from the TEM copper grid used for EDS measurements.
FT-IR
The FT-IR spectra verified that E. crystallina shell and C. toreuma shell powder were composed of both organic substances and mineral compounds with characteristic bands from 4,000 cm−1 to 500 cm−1 (Kamalanathan et al., 2014). The prominent absorption peaks attributed to the of calcite-CaCO3 were observed at 1,045 cm−1, 873 cm−1, and 712 cm−1 in both E. crystallina and C. toreuma shells (Figure 7) (Cahya and Marfuah, 2014). Besides, the organic functional groups represented by the weaker absorption peaks were amide (2,520 cm−1) and carboxylic acid (1,794 cm−1) (Islam et al., 2011). E. crystallina shells contained unique phosphate () which was assigned to the broad IR absorption band at 1,100–1,200 cm−1 (Figure 7A) (Berzina-Cimdina and Borodajenko, 2012). In addition, the characteristic IR absorption peak of aragonite-CaCO3 was observed at 1,082 cm−1 of in C. toreuma shells (Figure 7B), which meant that the shallow-water limpet shell contained two type polymorphs of calcium carbonate (Lee et al., 2011). Besides, the amorphous calcium carbonate (ACC) was not found in TEM studies of both E. crystallina and C. toreuma, and this result can be further verified by the obtained FT-IR and XRD results of limpet powders (Figures 7, 8). In FT-IR spectra, the ACC is expected to show an evident shoulder peak on the main asymmetric ν3 CO3 band at 1,460 cm−1 (Wang et al., 2009), while this characteristic peak was not found in FT-IR spectra of E. crystallina and C. toreuma shells (Figure 7). In XRD spectra, the ACC is expected to feature two broad humps at approximately 30° and 45° 2θ (Rodriguez-Blanco et al., 2012), while these broad characteristic peaks were not shown in the XRD spectra of E. crystallina and C. toreuma shells (Figure 8).
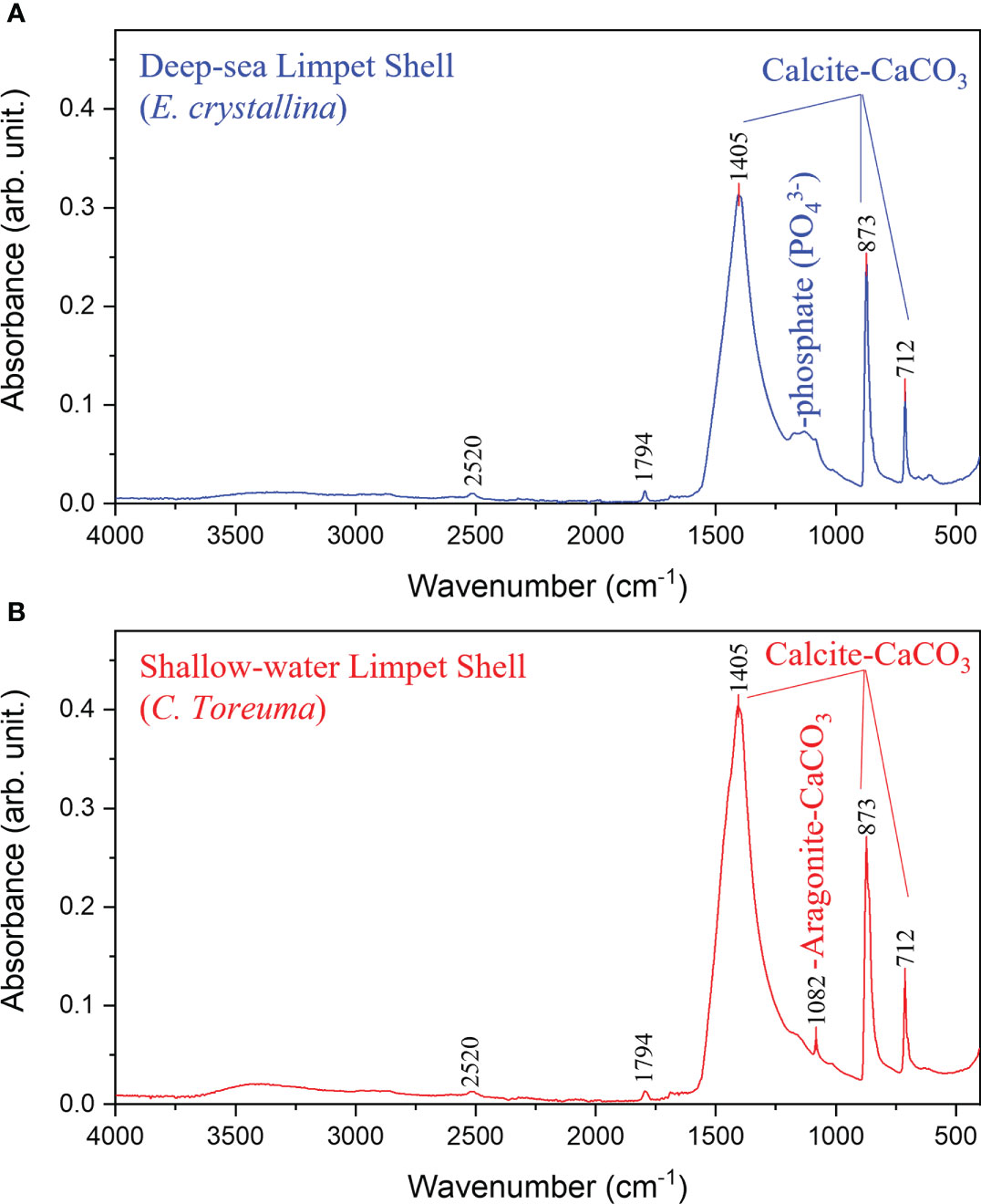
Figure 7 The FT-IR spectra of (A) deep-sea limpet E. crystallina shells containing calcite-CaCO3 and unique phosphate () and (B) shallow-water limpet C. toreuma shells containing two CaCO3 polymorphs, calcite-CaCO3 and aragonite-CaCO3.
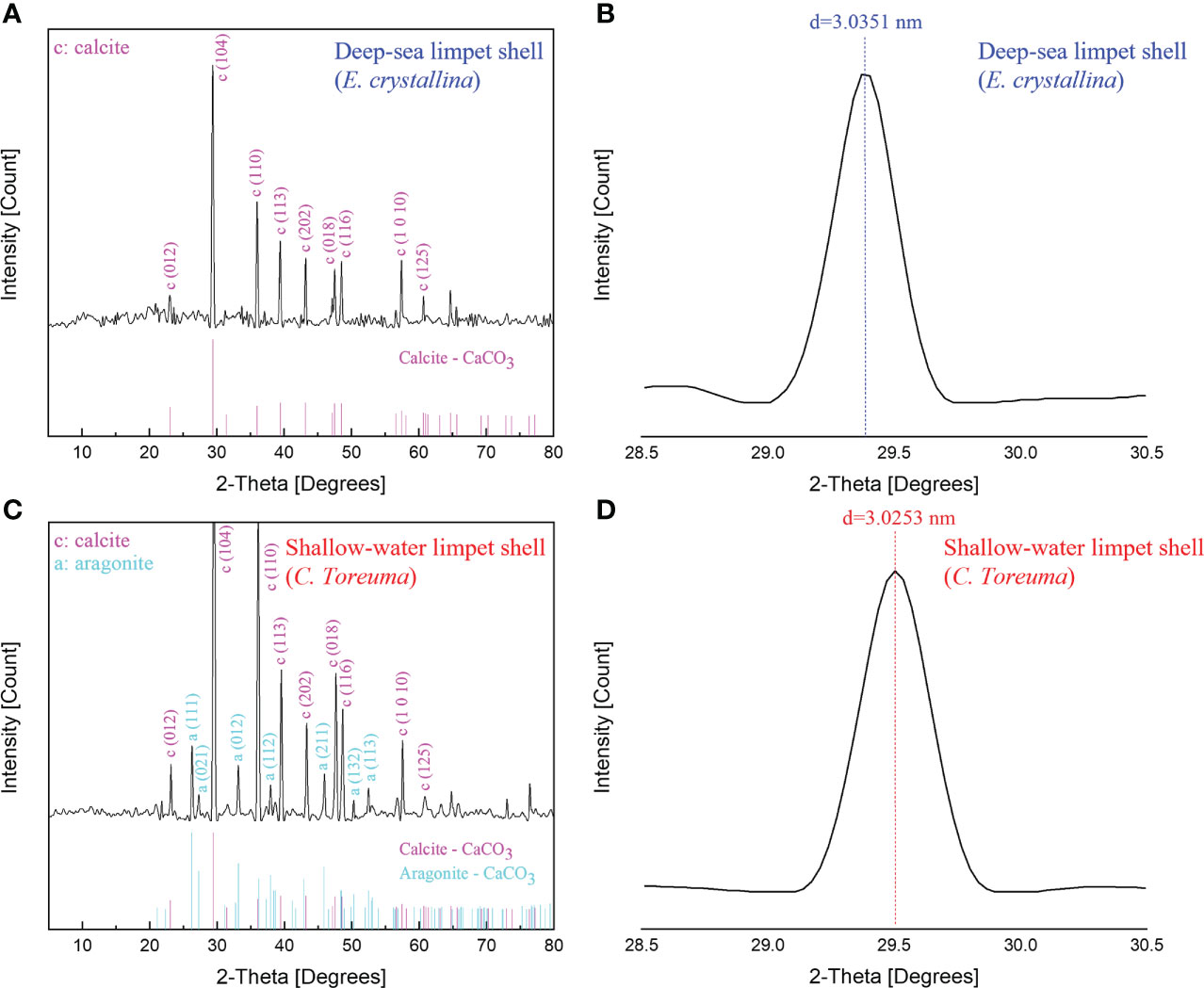
Figure 8 The XRD spectra of (A) deep-sea limpet E. crystallina shells containing pure calcite-CaCO3. (B) The detailed diffraction peak information of deep-sea limpet E. crystallina shells which is consistent with the literature value. (C) Shallow-water limpet C. toreuma shells containing two types of CaCO3 polymorphs, i.e., calcite and aragonite. (D) The detailed diffraction peak information of shallow-water limpet C. toreuma shells deviated from the literature value.
XRD
The further powder XRD analysis (Figure 8) was conducted to determine all possible crystalline phases of CaCO3 in the whole deep-sea and shallow-water limpet shells. XRD is generally used for phase identification of a crystalline material and can provide information on unit cell dimensions, characterization of crystalline, and measurement of sample purity. According to the XRD results shown in Figure 8A, the phase of the powders obtained from E. crystallina shells was indexed to be a rhombohedral calcite–CaCO3 structure which was consistent with the previous study (Duquette et al., 2017). The calcite–CaCO3 phase features a rhombohedral structure with the calculated parameters of a = 4.98 Å, c = 17.07 Å, catalogized in space group R-3c (JCPDS card no. 01-080-9776) (Wu et al., 2017). The diffraction peaks of calcite were intense, and thus the powders were proven to be highly crystalline. The characteristic calcite-CaCO3 peak of E. crystallina shells at 2θ of 29.4 ± 0.01° (Figure 8B) corresponded to the (1 0 4) crystallographic plane with the interplanar spacing d(E. crystallina-calcite) = 3.0351 ± 0.0003 nm which was consistent with the literature value of calcite crystal, d(calcite) = 3.0350 nm (Yuan et al., 2016). Notably, no diffraction peaks of other CaCO3 polymorphs are detected, which confirmed the only high pure phase of calcite-CaCO3 as the main composition in E. crystallina shells. In XRD spectra of C. toreuma limpet shells (Figure 8C), two polymorphs of calcium carbonate, i.e., calcite, aragonite, are detected as the crystalline phases. There are some small peaks confirming the existence of orthorhombic aragonite as a minor phase in C. toreuma shells with the lattice parameters a = 4.97 Å, b = 7.96 Å, c = 5.75 Å, catalogized in space group Pmcn (JCPDS card no. 01-075-9984) (Wu et al., 2017). However, the (1 0 4) calcite-CaCO3 peak of C. toreuma shells at 2θ of 29.5 ± 0.01° (Figure 8D) corresponded to the interplanar spacing d(C. toreuma-calcite) = 3.0253 ± 0.0003 nm which is lower than the literature value of calcite (Yuan et al., 2016). The deviation of diffraction peaks resulted from the mixture of different CaCO3 crystal polymorphs. The results from EDS, FT-IR, and XRD will provide more detailed chemical composition information on the demineralized isolation of molluscan conchixes and their potential multifunctional applications in pharmacy, cosmetics, and feed additives (Ehrlich et al., 2020).
Discussion
By using the TEM, SEM, FIB, EDS, and XRD techniques, the microstructure, crystallography, and chemical composition of deep-sea limpet E. crystallina shells living at a depth of 2,700 m of the Southwest Indian Ocean Ridge and shallow-water limpet C. toreuma shell were investigated and compared, which revealed effects of the living environment of ultra-high hydrostatic pressure on shell microstructures of deep-sea animals. Besides, the distinctive layered structures and pure calcite component of deep-sea limpet E. crystallina shells provided potential explanation of the origin of invertebrate marine biomaterials and further offered prospective applications in forced biomineralization and extreme biomimetics.
Conclusions
In the present study, we conducted TEM, SEM, EDS, XRD, and FT-IR for the first time to investigate the shell microstructural features and chemical composition of the deep-sea limpet E. crystallina collected at a depth of around 2,700 m in the Southwest Indian Ocean Ridge. The results revealed the detailed layered microstructures, the exfoliation characteristics, and the tilted crystallographic orientation of each layer’s unit cell at a small angle sequentially. The microscopic results further revealed that the E. crystallina shell exhibited untruncated and width-compressed layered structures due to the ultra-high hydrostatic force in the 2,700 m deep-sea condition, which disclosed potential effects of the living environment on the microstructure of deep-sea marine animal shells. Compared with shells of shallow-water limpet C. toreuma, E. crystallina shells were unique in containing pure calcite as the only calcium carbonate composition and the trace of phosphate originated from the phosphorus-rich deep-sea vent of the Southwest Indian Ocean, which verified that the deep-sea living environment potentially affected not only microstructures but also the chemical composition of marine animal shells.
Data availability statement
The raw data supporting the conclusions of this article will be made available by the authors, without undue reservation.
Ethics statement
The animal study was reviewed and approved by The Ethics Committee of the Hong Kong University of Science and Technology.
Author contributions
NW and PQ conceived and designed the project. ZY, SW, XC, and SX performed microstructure experiments of shells. ZY and XF performed the chemical composition experiments of shells. WW collected samples. ZY and WW drafted the manuscript. ZY, SW, SX, and YC participated in data analysis. All authors contributed to the manuscript revision and approved it for submission and publication.
Funding
This work was supported by grants from the Southern Marine Science and Engineering Guangdong Laboratory (Guangzhou) (SMSEGL20SC01).
Acknowledgments
The authors acknowledge the financial support from the Southern Marine Science and Engineering Guangdong Laboratory (Guangzhou) (SMSEGL20SC01) and the technical support at the Materials Characterization and Preparation Facility of The Hong Kong University of Science and Technology.
Conflict of interest
The authors declare that the research was conducted in the absence of any commercial or financial relationships that could be construed as a potential conflict of interest.
Publisher’s note
All claims expressed in this article are solely those of the authors and do not necessarily represent those of their affiliated organizations, or those of the publisher, the editors and the reviewers. Any product that may be evaluated in this article, or claim that may be made by its manufacturer, is not guaranteed or endorsed by the publisher.
Supplementary material
The Supplementary Material for this article can be found online at: https://www.frontiersin.org/articles/10.3389/fmars.2022.902815/full#supplementary-material
References
Agbaje O. B. A., Shir I. B., Zax D. B., Schmidt A., Jacob D. E. (2018). Biomacromolecules within bivalve shells: Is chitin abundant? Acta Biomater. 80, 176–187. doi: 10.1016/j.actbio.2018.09.009
Aizenberg J., Hanson J., Koetzle T., Weiner S., Addadi L. (1997). Control of macromolecule distribution within synthetic and biogenic single calcite crystals. J. Am. Chem. Soc. 119 (5), 881–886. doi: 10.1021/ja9628821
Berzina-Cimdina L., Borodajenko N. (2012). Research of calcium phosphates using Fourier transform infrared spectroscopy. (London: IntechOpen).
Cahya M., Marfuah N. (2014). “Identification of Calcium carbonate (CaCO3) characteristics from different kinds of poultry eggshells using X-ray diffraction (XRD) and Fourier transformation infra-red (FTIR)” in 2014 international conference on physics and its applications (ICOPIA-14) (Dordrecht, Netherlands: Atlantis Press).
Carroll M., Romanek C. S. (2008). Shell layer variation in trace element concentration for the freshwater bivalve Elliptio complanata. Geo Mar. Lett. 28 (5-6), 369–381. doi: 10.1007/s00367-008-0117-3
Checa A. G., Okamoto T., Ramírez J. (2006). Organization pattern of nacre in pteriidae (Bivalvia: Mollusca) explained by crystal competition. Proc. R. Soc B 273 (1592), 1329–1337. doi: 10.1098/rspb.2005.3460
Denny M. W. (2000). Limits to optimization: fluid dynamics, adhesive strength and the evolution of shape in limpet shells. J. Exp. Biol. 203 (17), 2603–2622. doi: 10.1242/jeb.203.17.2603
Duquette A., McClintock J. B., Amsler C. D., Pérez-Huerta A., Milazzo M., Hall-Spencer J. M. (2017). Effects of ocean acidification on the shells of four Mediterranean gastropod species near a CO2 seep. Mar. pollut. Bull. 124 (2), 917–928. doi: 10.1016/j.marpolbul.2017.08.007
Ehrlich H. (2019). Marine biological materials of invertebrate origin (Cham, Switzerland: Springer).
Ehrlich H., Bailey E., Wysokowski M., Jesionowski T. (2021). Forced biomineralization: A review. Biomimetics 6 (3), 46. doi: 10.3390/biomimetics6030046
Ehrlich H., Martinović R., Joksimović D., Petrenko I., Schiaparelli S., Wysokowski M., et al. (2020). Conchixes: Organic scaffolds which resemble the size and shapes of mollusks shells, their isolation and potential multifunctional applications. Appl. Phys. A 126 (7), 1–13. doi: 10.1007/s00339-020-03728-7
Ehrlich H., Nikolaev A. (2017). “\Psychrophiles as sources for bioinspiration in biomineralization and biological materials science” in Extreme Biomimetics (Cham, Switzerland: Springer), 1–51.
Hamester M. R. R., Balzer P. S., Becker D. (2012). Characterization of calcium carbonate obtained from oyster and mussel shells and incorporation in polypropylene. Mater. Res. 15, 204–208. doi: 10.1590/S1516-14392012005000014
Harford N., O’Connor N., Taylor D. (2020). Impact resistance of limpet shells: a study of local adaptations. Appl. Phys. A: Mater. Sci. Process. 126 (9), 1–9. doi: 10.1007/s00339-020-03941-4
Harley C. D., Denny M. W., Mach K. J., Miller L. P. (2009). Thermal stress and morphological adaptations in limpets. Funct. Ecol. 23 (2), 292–301. doi: 10.1111/j.1365-2435.2008.01496.x
Islam K. N., Bakar M. Z. B. A., Noordin M. M., Hussein M. Z. B., Abd Rahman N. S. B., Ali M. E. (2011). Characterisation of calcium carbonate and its polymorphs from cockle shells (Anadara granosa). Powder Technol. 213 (1-3), 188–191. doi: 10.1016/j.powtec.2011.07.031
Jiang T., Liu H., Huang D., Zhang S., Li Y., Gong X., et al. (2014). Valley and band structure engineering of folded MoS2 bilayers. Nat. Nanotechnol. 9 (10), 825–829. doi: 10.1038/nnano.2014.176
Johnson A. B., Fogel N. S., Lambert J. D. (2019). Growth and morphogenesis of the gastropod shell. Proc. Natl. Acad. Sci. 116 (14), 6878–6883. doi: 10.1073/pnas.1816089116
Kamalanathan P., Ramesh S., Bang L., Niakan A., Tan C., Purbolaksono J., et al. (2014). Synthesis and sintering of hydroxyapatite derived from eggshells as a calcium precursor. Ceram. Int. 40 (10), 16349–16359. doi: 10.1016/j.ceramint.2014.07.074
Lee S. W., Jang Y. N., Kim J. C. (2011). Characteristics of the aragonitic layer in adult oyster shells, Crassostrea gigas: structural study of myostracum including the adductor muscle scar. Evid. Based Complement. Altern. Med. 2011, 10. doi: 10.1155/2011/742963
Li L., Kolle S., Weaver J. C., Ortiz C., Aizenberg J., Kolle M. (2015). A highly conspicuous mineralized composite photonic architecture in the translucent shell of the blue-rayed limpet. Nat. Commun. 6 (1), 1–11. doi: 10.1038/ncomms7322
Lomovasky B. J., de Aranzamendi M. C., Abele D. (2020). Shorter but thicker: analysis of internal growth bands in shells of intertidal vs. subtidal Antarctic limpets, nacella concinna, reflects their environmental adaptation. Polar Biol. 43 (2), 131–141. doi: 10.1007/s00300-019-02615-z
Marie B., Joubert C., Tayalé A., Zanella-Cléon I., Belliard C., Piquemal D., et al. (2012). Different secretory repertoires control the biomineralization processes of prism and nacre deposition of the pearl oyster shell. Proc. Natl. Acad. Sci. 109 (51), 20986–20991. doi: 10.1073/pnas.1210552109
Martin P., Lauro F. M., Sarkar A., Goodkin N., Prakash S., Vinayachandran P. (2018). Particulate polyphosphate and alkaline phosphatase activity across a latitudinal transect in the tropical Indian ocean. Limnol. Oceanogr. 63 (3), 1395–1406. doi: 10.1002/lno.10780
Nakai S., Miura O., Maki M., Chiba S. (2006). Morphological and habitat divergence in the intertidal limpet Patelloida pygmaea. Mar. Biol. 149 (3), 515–523. doi: 10.1007/s00227-005-0241-9
Oliveira C. R. M., De Castro L. M., Da Cruz Nazareth M. A., Harayashiki C. A. Y., Castro Í. B. (2020). Shell structure and composition alterations in the limpet Lottia subrugosa along a contamination gradient in the santos estuary, Brazil. Ecol. Indic. 115, 106417. doi: 10.1016/j.ecolind.2020.106417
Rodolfo-Metalpa R., Houlbrèque F., Tambutté É., Boisson F., Baggini C., Patti F. P., et al. (2011). Coral and mollusc resistance to ocean acidification adversely affected by warming. Nat. Clim. Change 1 (6), 308–312. doi: 10.1038/nclimate1200
Rodriguez-Blanco J., Shaw S., Bots P., Roncal-Herrero T., Benning L. G. (2012). The role of pH and mg on the stability and crystallization of amorphous calcium carbonate. J. Alloys Compd. 536, S477–S479. doi: 10.1016/j.jallcom.2011.11.057
Song X., Liu Z., Wang L., Song L. (2019). Recent advances of shell matrix proteins and cellular orchestration in marine molluscan shell biomineralization. Front. Mar. Sci. 6, 41. doi: 10.3389/fmars.2019.00041
Sun J., Zhou Y., Chen C., Kwan Y. H., Sun Y., Wang X., et al. (2020). Nearest vent, dearest friend: biodiversity of tiancheng vent field reveals cross-ridge similarities in the Indian ocean. R. Soc Open Sci. 7 (3), 200110. doi: 10.1098/rsos.200110
Suzuki M., Kameda J., Sasaki T., Saruwatari K., Nagasawa H., Kogure T. (2010). Characterization of the multilayered shell of a limpet, Lottia kogamogai (Mollusca: Patellogastropoda), using SEM–EBSD and FIB–TEM techniques. J. Struct. Biol. 171 (2), 223–230. doi: 10.1016/j.jsb.2010.04.008
Suzuki M., Kogure T., Nagasawa H. (2017). Studies on the chemical structures of organic matrices and their functions in the biomineralization processes of molluscan shells. AGri Biosci. Monogr. 7, 25–39. doi: 10.5047/agbm.2017.00702.0025
Suzuki M., Kogure T., Weiner S., Addadi L. (2011). Formation of aragonite crystals in the crossed lamellar microstructure of limpet shells. Cryst. Growth Des. 11 (11), 4850–4859. doi: 10.1021/cg2010997
Wang X., Kong R., Pan X., Xu H., Xia D., Shan H., et al. (2009). Role of ovalbumin in the stabilization of metastable vaterite in calcium carbonate biomineralization. J. Phys. Chem. B 113 (26), 8975–8982. doi: 10.1021/jp810281f
Wang Y., Liu C., Du J., Huang J., Zhang S., Zhang R. (2018). The microstructure, proteomics and crystallization of the limpet teeth. Proteomics 18 (19), 1800194. doi: 10.1002/pmic.201800194
Weiner S., Addadi L. (2011). Crystallization pathways in biomineralization. Annu. Rev. Mater. Res. 41, 21–40. doi: 10.1146/annurev-matsci-062910-095803
Wu S., Chiang C., Zhou W. (2017). Formation mechanism of CaCO3 spherulites in the myostracum layer of limpet shells. Crystals 7 (10), 319. doi: 10.3390/cryst7100319
Ying Z., Diao J., Wang S., Cai X., Cai Y., Liu H., et al. (2020). Revealing high temperature stability of platinum nanocatalysts deposited on graphene oxide by in-situ TEM. Mater. Charact. 170, 110706. doi: 10.1016/j.matchar.2020.110706
Ying Z., Diao J., Wang S., Cai X., Cai Y., Liu H., et al. (2021a). In situ atomic-scale studies of thermal stability and surface reconstruction of ZnO nanowires based pd nanocatalysts. Mater. Des. 209, 109947. doi: 10.1016/j.matdes.2021.109947
Ying Z., Diao J., Wang S., Cai X., Cai Y., Liu H., et al. (2021b). Thermal stability, ripening dynamics and coalescing microstructures of reduced graphene oxide-based platinum nanocatalysts: An in-situ TEM study. Diam. Relat. Mater. 120, 108690. doi: 10.1016/j.diamond.2021.108690
Ying Z., Diao J., Wang S., Cai X., Liu H., Wang N. (2021c). In-situ transmission electron microscopy: electron beam effects in carbon-based nanomaterials. Microsc. Microanal. 27 (S1), 2110–2113. doi: 10.1017/S1431927621007625
Keywords: Deep-sea, limpet, microstructure, composition, crystallography
Citation: Ying Z, Wang S, Wong WC, Cai X, Feng X, Xiang S, Cai Y, Qian P-Y and Wang N (2022) An insight into the microstructures and composition of 2,700 m-depth deep-sea limpet shells. Front. Mar. Sci. 9:902815. doi: 10.3389/fmars.2022.902815
Received: 23 March 2022; Accepted: 25 July 2022;
Published: 17 August 2022.
Edited by:
Hermann Ehrlich, Freiberg University of Mining and Technology, GermanyReviewed by:
Jose Luis Arias, University of Chile, ChileMarcin Wysokowski, Poznań University of Technology, Poland
Copyright © 2022 Ying, Wang, Wong, Cai, Feng, Xiang, Cai, Qian and Wang. This is an open-access article distributed under the terms of the Creative Commons Attribution License (CC BY). The use, distribution or reproduction in other forums is permitted, provided the original author(s) and the copyright owner(s) are credited and that the original publication in this journal is cited, in accordance with accepted academic practice. No use, distribution or reproduction is permitted which does not comply with these terms.
*Correspondence: Pei-Yuan Qian, boqianpy@ust.hk; Ning Wang, phwang@ust.hk