- 1Department of Food Science, Cornell University, Ithaca, NY, United States
- 2Department of Microbiology and Immunology, Cornell University, Ithaca, NY, United States
- 3Division of Nutritional Sciences, Cornell University, Ithaca, NY, United States
- 4Department of Animal Science, Cornell University, Ithaca, NY, United States
The aquaculture industry faces growing pressure to reduce the use of antibiotics for control of bacterial diseases. In this study we tested the effectiveness of dietary cecropin A, an insect-derived antimicrobial peptide, at preventing mortality and reducing incidence of carrier status in rainbow trout (Oncorhynchus mykiss) challenged by immersion with Yersinia ruckeri. Additionally, we conducted longitudinal analyses of microbiome changes to elucidate effects of both cecropin A and bacterial infection. An in vitro experiment indicated that Y. ruckeri is susceptible to cecropin A. However, dietary cecropin A did not improve the survival of fish challenged with Y. ruckeri, nor did it decrease the persistence of Y. ruckeri in the intestine of fish that survived infection. Moreover, levels of intestinal Y. ruckeri as measured by qPCR suggested that cecropin A may have negatively impacted the ability of fish to resist colonization by this bacterial pathogen. Concomitantly with the survival experiments, the microbiomes of challenged and mock-challenged fish were sampled at days 0, 3, 8, and 30. The microbiomes were in general dominated by Mycoplasma sp. at days 0, 3 and 8, independent of diet, and whether fish had been challenged or mock-challenged. At day 30, the microbiomes of mock-challenged fish fed the +cecropin diet were characterized by lower internal (alpha) diversity (p<.01), greater relative abundance of Mycoplasma sp., and a decrease in gram-negative taxa, when compared to the microbiomes of fish fed the control diet. The opposite was observed in the microbiome of challenged fish. Lastly, correlation analysis of amplicon sequence variants (ASVs) revealed a negative correlation between the presence of Y. ruckeri and seven ASVs, including Mycoplasma sp., suggesting possible beneficial effects of these taxa. In addition, six ASVs were positively correlated to Y. ruckeri, including Flavobacterium succinicans – a known opportunistic fish pathogen. In conclusion, this study revealed that dietary cecropin A was bioactive and exerted significant effects on the microbiome but did not improve fish resistance to infection by Y. ruckeri. Based on our observations and other published results, it appears that high relative abundance of Mycoplasma sp. correlates with higher resistance to intestinal colonization by bacterial pathogens.
Introduction
Historical use of antibiotics is not well-documented for aquaculture (Schar et al., 2020), but evidence from regional studies where data is available supports the claim that the rapid growth and intensification of aquaculture production in the past fifty years have been fueled in many cases by heavy antimicrobial use (Millanao et al., 2011; Rico et al., 2013; Cabello et al., 2016; Chuah et al., 2016). As international momentum coalesces around tighter regulation of antibiotic use, it is essential that the aquaculture industry continue to develop alternative strategies for control of infectious disease (Stentiford et al., 2017).
The primary focus of most research on fish disease has been has rightfully been on prevention rather than treatment of outbreaks. Development of vaccines, better nutrition, increasingly rapid and accurate diagnostic services, improvements in farm siting and biosecurity, and selective breeding for disease resistance have all been instrumental breakthroughs in maintaining fish health (Austin, 2012). In recent years, the role of the fish microbiome has also been increasingly investigated in aquaculture contexts (de Bruijn et al., 2018; Legrand et al., 2020; Infante-Villamil et al., 2021). The microbiome of fish is influenced by diet (Ringø et al., 2016), and this has been shown to mediate changes in the immune response to infection (Ingerslev et al., 2014). Changes in fish microbiome are associated with infection states of various pathogens, including bacteria (Li et al., 2016; Bozzi et al., 2021), viruses (Reid et al., 2017; She et al., 2017) and parasites (Llewellyn et al., 2017; Vasemägi et al., 2017). Furthermore, a number of studies have shown that modification of the microbiome via probiotic bacteria can improve immune parameters and disease resistance in fish (Yi et al., 2018; Chen et al., 2019; Gauthier et al., 2019). Despite these findings, however, current understanding of what constitutes a healthy or natural microbiome for most fish species is limited (Legrand et al., 2020).
In addition to preventive strategies, it is also worth considering whether it will be possible to one day replace antibiotics for disease intervention. Therapeutic antibiotic treatments remain an important backstop for fish welfare in the event of disease outbreaks, even though resistance has led to diminishing utility in some regions (Cabello and Godfrey, 2019). One proposed alternative is to replace or augment the use of conventional antibiotics with natural or synthetic antimicrobial peptides (AMPs), also referred to as host-defense peptides. AMPs are a part of the innate immune response in animals, fungi, and plants, and are also produced by bacteria. They are typically small (10-50 amino acid) cationic peptides with the ability to inhibit bacterial growth in vitro, though many are now known to have additional functions in immune signaling. Structurally, AMPs are characterized by a combination of positively charged and hydrophobic residues which facilitate their interaction with bacterial cell membranes (Fjell et al., 2012). Bacterial resistance to AMPs can develop via selective pressure (Joo et al., 2016), but there are a number of reasons why AMPs are likely to be refractory to recapitulating the current AMR crisis (Lazzaro et al., 2020).
AMPs have been tested as dietary supplements in feeds for a variety of terrestrial animal production systems, including swine, poultry, and cattle, and a number of positive effects including mitigation of disease symptoms and modest increases in growth and/or feed efficiency have been reported (Silveira et al., 2021). A growing body of research in recent years has also begun to investigate the effects of AMPs in fish, examining their effects on growth (Zhou et al., 2008; Dong et al., 2015; Ge et al., 2020; Hu et al., 2021), immune status (Wang et al., 2021), and disease resistance (Lygren et al., 1999; Liu et al., 2014; Chettri et al., 2017; Ting et al., 2018; Lee et al., 2019; de Sousa et al., 2021; Rashidian et al., 2021; Simora et al., 2021). For the most part, these studies have not reported adverse effects of AMPs, and about half have shown protective effects in the context of infection. Of the studies listed above, only Ge et al. looked at the effect of AMPs on the microbiome, finding that microbial diversity was increased relative to the control group in fish fed the highest tested dose of AMP (Ge et al., 2020). Furthermore, in studies that have tested AMPs in the context of fish infection, their application has nearly always been prophylactic rather than therapeutic.
There remains a knowledge gap in the understanding of how microbiome-based approaches to fish health management interact with other strategies, and in the effectiveness of AMPs administered close to or after the onset of infection. Conventional antibiotics have been shown in mammalian systems to negatively impact resistance to some diseases by disrupting the microbiome (Theriot et al., 2014; Langdon et al., 2016; Gutierrez et al., 2020). While the body of literature is much smaller, similar effects have been shown in fish: treatment with streptomycin sulfate prior to challenge with Vibrio anguillarum increased mortality in black molly (Poecilia sphenops) (Schmidt et al., 2017), and pre-treatment with olaquindox increased mortality in zebrafish (Danio rerio) challenged with Aeromonas hydrophila (He et al., 2017). In both of these studies, the authors attributed the observed changes in disease resistance to disruption of the microbiome by antibiotic treatment. These findings, and evidence for the importance of the microbiome in general, would appear to be in tension with the fact that historically a significant portion of antibiotic use in aquaculture has been prophylactic (Ibrahim et al., 2020): presumably this is the case because farmers have concluded that the health benefits of prophylactic antibiotic use outweigh the costs. Understanding the effect that antimicrobials – either conventional antibiotics or AMPs – exert on the microbiome, and the consequences of those effects on fish health, is key to integrating the findings of these developing fields.
In this study, we examined the effect of a canonical insect-derived AMP – Hyalaphora cecropia cecropin A – on the microbiome, disease resistance, and carrier status of Rainbow trout (Oncorhynchus mykiss) in the context of bacterial infection. The cecropin family of AMPs is highly expressed in insects when exposed to infection by gram-negative bacteria (Lemaitre et al., 1997) and has strong in vitro activity against this group of bacteria. Our hypothesis was that introduction of cecropin A in the diet would protect trout against the gram-negative bacterium Yersinia ruckeri, the causative agent of enteric redmouth disease (ERM) and would reduce the prevalence of detectable load of Y. ruckeri in the intestine over the course of infection and recovery. The timing of when to introduce cecropin to the fish was an important consideration. In most previous studies of AMPs involving a disease challenge, fish spend at least a month on experimental feeds before being exposed to pathogens. In the context of this project, we aimed to better evaluate the potential effectiveness of dietary cecropin as a tool for disease intervention. Therefore, we opted to begin feeding 3 days prior to the challenge. This gave a sufficient window to ensure that fish would accept the experimental feed while minimizing changes to the underlying host biology. We also sought to understand how the fish microbiome responds over the course of infection and how, if at all, dietary cecropin might modify this response. Our findings reveal that in this experimental context, cecropin A did not improve resistance to infection or clearance of Y. ruckeri from the intestine. Analyses of intestinal microbiomes indicated that fish fed a control diet displayed a lower alpha-diversity following a challenge with Y. ruckeri compared to those fed the +cecropin diet, whereas in mock-challenged fish an increase in alpha diversity was observed in both diet groups.
Materials and Methods
In vitro Activity of Cecropin A Against Yersinia ruckeri
Recombinant cecropin A peptide (Abbexa, Ltd., Houston, TX) was used for this project. Its activity against Y. ruckeri was first tested in vitro in three replicate experiments. Log-phase broth culture of Y. ruckeri strain CSF007-82 (Nelson et al., 2015) was diluted to a density of 1.5–5 x106 CFU/ml and incubated at 16°C with serial dilutions of cecropin A in triplicate wells of a 96 well plate. OD600 was recorded for 48 hours using an Elx808 absorbance microplate reader (BioTek). Samples with no change in OD600 after 48 hours were deemed fully inhibited; the minimum inhibitory concentration (MIC) was defined as the lowest tested concentration of cecropin A for which no change in OD600 was observed. To differentiate between bacteriostatic and bactericidal concentrations, samples with no apparent bacterial growth were plated on tryptic soy agar. The bactericidal concentration was defined as the lowest concentration of cecropin A that killed 99.9% of the initial inoculum.
Diet Production and Analysis
Two diets, hereafter referred to as the control and +cecropin diets, were created for this experiment using BioClarks Fry 1.2-mm pellets (Bio-Oregon, Longview, WA) as a basal diet. This diet was selected because, in contrast to many commercial diets for fry, it does not contain immune-stimulating ingredients. The +cecropin diet was generated by vacuum-spray coating the basal diet with aqueous cecropin A: 110 mg of cecropin A per kilogram of basal diet, which is within the range used in previous studies of this AMP in fish diets (ZiRui et al., 2014; Lin et al., 2015). The control diet was generated by vacuum spray-coating the basal diet with sterile water. Both diets were lyophilized and stored at -20°C. Proximate analysis of remaining diet samples was performed by Exact Scientific Services (Ferndale, WA) at the conclusion of the study (Table S1).
Fish Care and Handling
Rainbow trout (Oncorhynchus mykiss) fry were provided by the New York State fish hatchery at Bath, NY where they were kept outdoors in flowthrough concrete raceways. All animals were cared for in compliance with the Guide for the Care and Use of Laboratory Animals and American Association of Laboratory Animal Science Position Statements, and all experimental protocols were approved by the Cornell University Institutional Care and Use Committee (IACUC). Upon arrival at the Cornell aquatic facility, fish were kept in a 700 liter fiberglass tank under flowthrough conditions at 12 ± 1°C and fed BioClarks Fry 1.2-mm pellets at a rate of 3% of total bodyweight per day. Over a third week of acclimation, temperature was gradually increased from 12 ± 1°C to 15 ± 1°C. For the duration of the experiment, effluent from fish tanks was collected and decontaminated with a chlorine injection system prior to being released into the sewer system.
For the first trial, 172 fish (average body weight = 2.5g) were randomly assigned to one of four treatments: control diet mock-infection (28 fish), +cecropin diet mock-infection (28), control diet infection (58), or +cecropin diet infection (58). Three days after commencement of feeding the experimental diets, fish were either challenged with Y. ruckeri or mock-challenged as described below. Six fish were sampled at each of the first three timepoints and all remaining fish were sampled at day 30. Upon completion of this trial, all tanks were sterilized and flushed with water for 48 hours in preparation for the second trial.
The second trial focused on repeating the infection treatments with larger sample sizes: 138 fish (avg. = 4.0g) were randomly assigned to each diet. Fish were fed the experimental diet for three days before being challenged with Y. ruckeri. Eight fish from each diet group were sampled at each of the first three timepoints and 10 fish per diet group were sampled at day 30.
Bacterial Challenge Design and Sampling
Fish were challenged by immersion with Y. ruckeri strain CSF007-82 at a concentration of ~8x108 CFU/ml. The mock-challenge group was immersed in water with a corresponding amount of sterile tryptic soy broth (TSB). Challenges took place at a target stocking density of 7.5g/L in randomly assigned 10-L tanks within a zebrafish rack modified to operate as a flowthrough system with supplemental aeration. Water flow was paused during the challenge. Bacterial concentration was verified by plating of water samples from each tank. After 1 hour, flow was restored to rapidly flush out the system; after 24 hours, fish were transferred back to 700-L flowthrough tanks and maintained on the experimental diets for the remainder of the experiment. Mortalities and morbidities were monitored for 30 days. Appetite was notably depressed in the challenged group from day 3 to day 14 post-infection; during this period feeding was reduced to 1% of total estimated bodyweight per day. For all other days, feeding was 3% of total estimated bodyweight.
Samples were collected at 4 timepoints: immediately prior to the challenge (day 0), and then on days 3, 8, and 30 post-challenge. Sampled fish were euthanized via overdose with buffered MS-222 and all intestinal tissue (including contents) posterior to the pyloric caeca were aseptically collected, flash frozen, and stored at -80°C. In addition, posterior kidney samples from challenged fish were streaked on TSA plates for confirmation of infection. At 30 days post-challenge all remaining fish were euthanized with buffered MS-222.
DNA Extraction From Fish Intestines
DNA was extracted as previously described (Sibinga and Marquis, 2021). Briefly, whole intestines were weighed before an initial homogenization step using 1.3mm chrome steel beads and DNA extraction buffer (200 mM NaCl, 200 mM Tris– HCl pH 7.5, 20 mM EDTA, 5% SDS). A volume of the primary homogenate corresponding to 20 mg of intestine was subsequently subjected to a secondary homogenization step with 0.1-mm zirconia/silica beads. DNA was isolated via phenol:chloroform extraction and isopropanol precipitation before resuspension in Tris-EDTA buffer.
qPCR Detection of the Y. ruckeri 16S rRNA Gene
Quantitative polymerase chain reaction (qPCR) was performed using a 7500 real-time PCR instrument (Applied Biosystems) for detection of Y. ruckeri in intestinal DNA extracts. We used a previously published hydrolysis (Taqman) primer/probe set targeting the Y. ruckeri 16S rRNA gene (Ghosh et al., 2018). The primers target the V2 and V3 regions, while the probe targets the conserved region in between. Reactions of 10μl were performed in triplicate wells of a 96-well plate using 5μl 2X PrimeTime Gene Expression Master Mix (Integrated DNA Technologies, Coralville, IA), primers (400nM each), probe (100nM), and DNA template (1μl). Samples for which the 16S gene was detected in at least two of three wells were considered positive for Y. ruckeri.
PCR Amplification of 16S rRNA Gene for DNA Sequencing
Intestinal DNA extracts were diluted 1:10 before being amplified by PCR targeting the 16S rRNA gene sequence (region V4) using the universal primers 515F and GoLay-barcoded 806R and the PCR program as previously described (Caporaso et al., 2011). Samples were amplified in duplicate with the following thermocycler protocol: hold at 94°C for 3 min; 30 cycles of 94°C for 45 s, 50°C for 1 min, 72°C for 1.5 min; and hold at 72°C for 10 min, and the duplicate final amplified products were pooled. Amplicons were further purified using Mag-Bind® RxnPure Plus (Omega Bio-tek, Inc., GA) and quantified with NanoDrop 2000 spectrophotometer (Thermo Fisher Scientific, Waltham, MA, USA), and 150 ng of amplicons from each sample were pooled and paired-end sequenced (2x250bp) on an Illumina MiSeq instrument in the Genomic Facility at Cornell Institute of Biotechnology.
Analysis of 16S rRNA Gene Sequences
Sequence data processing was performed using the QIIME 2 (v 2020.2) pipeline (Caporaso et al., 2010). The Divisive Amplicon Denoising Algorithm 2 (DADA2) (Callahan et al., 2016) method was applied to quality-filter sequences and categorize amplicon sequence variants (ASVs). Taxonomic alignment of all ASVs was performed using Wasabi (Veidenberg et al., 2016).
The resulting ASVs were assigned taxonomy by mapping with the Greengenes 16S rRNA Gene Database (McDonald et al., 2012). Feature tables were generated collapsed at various taxonomic levels including phylum, class, order, family, genus, and species. The QIIME2 diversity plugin was used to compute Shannon’s diversity index and Bray-Curtis distances for alpha and beta diversity evaluation (Bolyen et al., 2019). Beta diversity distances were calculated between all samples to facilitate comparisons between and within each group. The QIIME output data was then imported to Rstudio (Version 1.0.136) with several packages including phyloseq (McMurdie and Holmes, 2013), microbiome (Lahti and Shetty, 2012; Lahti and Shetty, 2019), tidyverse (Wickham et al., 2019), EnhancedVolcano (Blighe et al., 2021) and ggplot2 (Wickham, 2016) for normalizing and plotting of the input data.
Statistical Analysis
Fish survival was plotted using a Kaplan-Meier curve; sampled fish and those surviving to the end of the experiment were censored at the appropriate time points. Analysis of survival data used JMP Pro 14 software to conduct a Gehan-Breslow-Wilcoxon test and a Cox proportional hazard model accounting for diet and trial effects. Detection data was analyzed using Fisher’s exact test for each timepoint with Bonferroni correction for multiple comparisons. QIIME 2 (v 2020.2) and Rstudio (v 1.0.136) were used to analyze sequence data. In the QIIME workflow, samples with poor quality (as evaluated by over 60% chimeric reads) were ignored in subsequent analysis and graphing (Table S2). Shannon and Bray-Curtis diversity metrics were tested against sample metadata factors using QIIME2 diveristy plugin (Bolyen et al., 2019). Shannon index was compared with pairwise Kruskal-Wallis tests. Bray-Curtis distances were compared with pairwise permutational multivariate analysis of variance (PERMANOVA) tests using 999 permutations and Benjamini/Hochberg FDR p-value adjustment for pairwise comparisons. Volcano plots for identifying differentially abundant taxa between two selected groups were calculated via DESeq2 (Love et al., 2014). The correlation coefficients plot between Y. ruckeri and taxonomic variables was generated by calculating the Pearson correlation between centered log-ratio transformation of the abundance of taxonomic variables and the Cq value of Y. ruckeri level in the intestine.
Results
Cecropin A Inhibits the Growth of Y. ruckeri In Vitro
We first tested the in vitro activity of cecropin A, an insect AMP, against Y. ruckeri, a fish bacterial pathogen. The minimum inhibitory concentration (MIC) of cecropin A against Y. ruckeri was 3.75 μM under the parameters tested in this study, while partial inhibition of growth was observed as low as 0.47μM (Figure 1). Plating revealed that 3.75 μM is bacteriostatic: while there was no change in OD600 after 48 hours, by plate count the bacterial population actually doubled in this time period. By contrast, both the 7.5 μM and 15 μM concentrations were bactericidal over the same timeframe. The 3.75 μM MIC in this study corresponds to approximately 6.6x10-16 moles (4x108 molecules) of cecropin A for each bacterial cell. Assuming equal distribution of peptide among feed particles and equal consumption of feed by each fish, the feed in this study would have supplied each fish with approximately 3.4x10-9 moles (2.1x1015 molecules) of cecropin A per day.
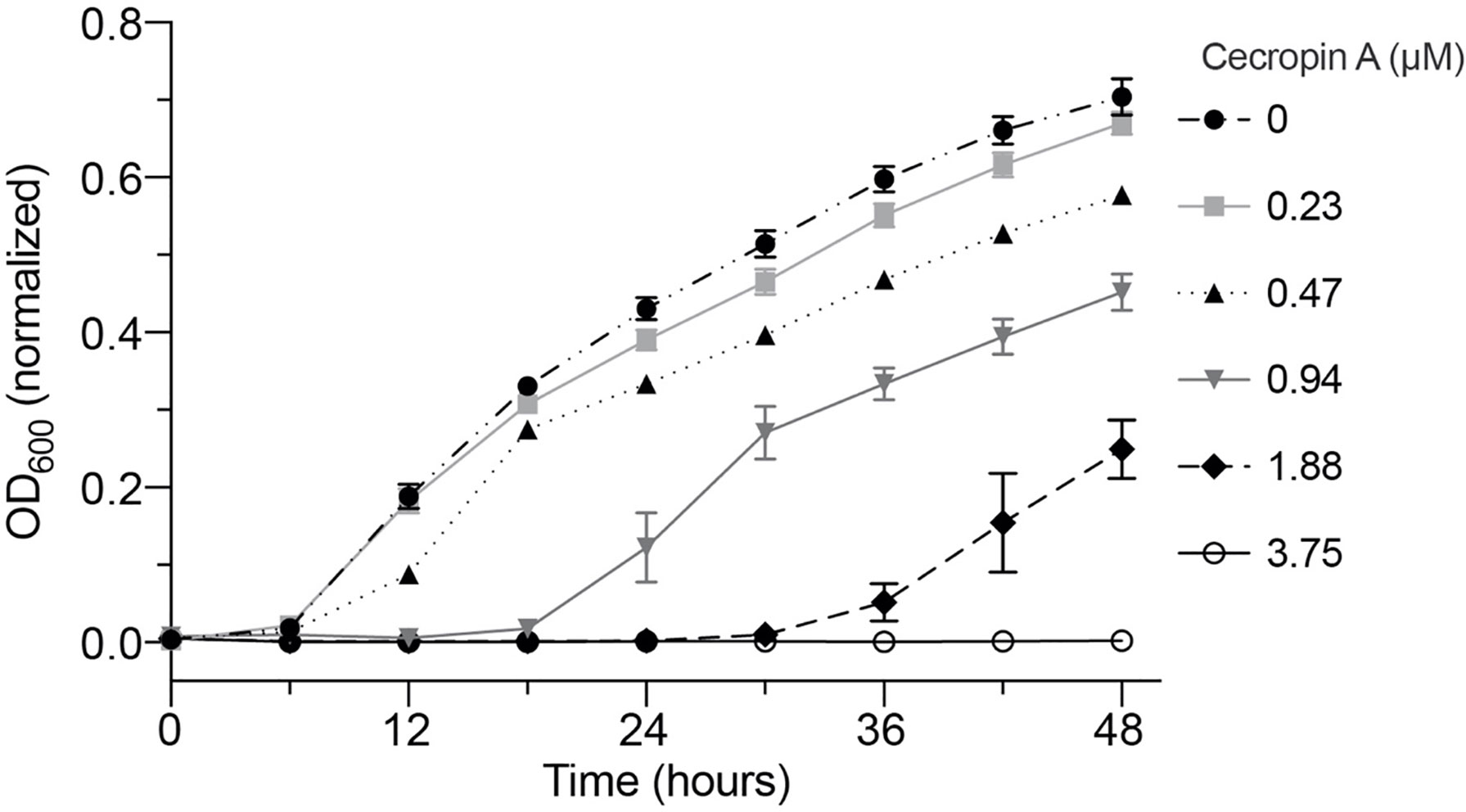
Figure 1 Dose-dependent inhibition of Y. ruckeri by cecropin A in vitro. Y. ruckeri was incubated at 16°C in broth supplemented with serial dilutions of cecropin A. The experiment was performed in a 96-well plate and OD600 was recorded in triplicate wells per sample for a period of 48 hours. Plotted values are the average of three independent experiments. No change in OD600 was observed in wells containing 3.75, 7.5, and 15 µM cecropin A (latter two concentrations not shown).
Cecropin A Did Not Protect Fish Against Y. ruckeri
Considering that cecropin A inhibits the growth of Y. ruckeri in vitro, we aimed to assess whether adding cecropin A to diet could improve fish resistance to a challenge with this bacterial pathogen. Fish were fed a diet supplemented or not with cecropin A and challenged with Y. ruckeri by immersion. The infection challenge was performed twice, with the second trial immediately following the first. Fish were monitored for a period of 30 days post-challenge (Figure 2). In the first trial, mortalities were observed between day 5 and 9 for the control diet group, and between day 4 and 9 for the +cecropin diet group. Survival rates were 31% and 20% for the control and +cecropin diet groups, respectively. In the second trial, mortalities were observed between day 5 and 14 for the control diet group, and between day 4 and 22 for the +cecropin diet group. Survival rates were 43% and 32% for the control and +cecropin diet groups, respectively. No mortality was observed in mock-challenged fish for either diet group. A Cox proportional hazards model combining data from both trials revealed a statistically significant difference between the trials (p<0.001) but not the diets (p=0.059); the infection trials were therefore considered separately for analysis. Differences in survival between diet groups were not statistically significant for either trial.
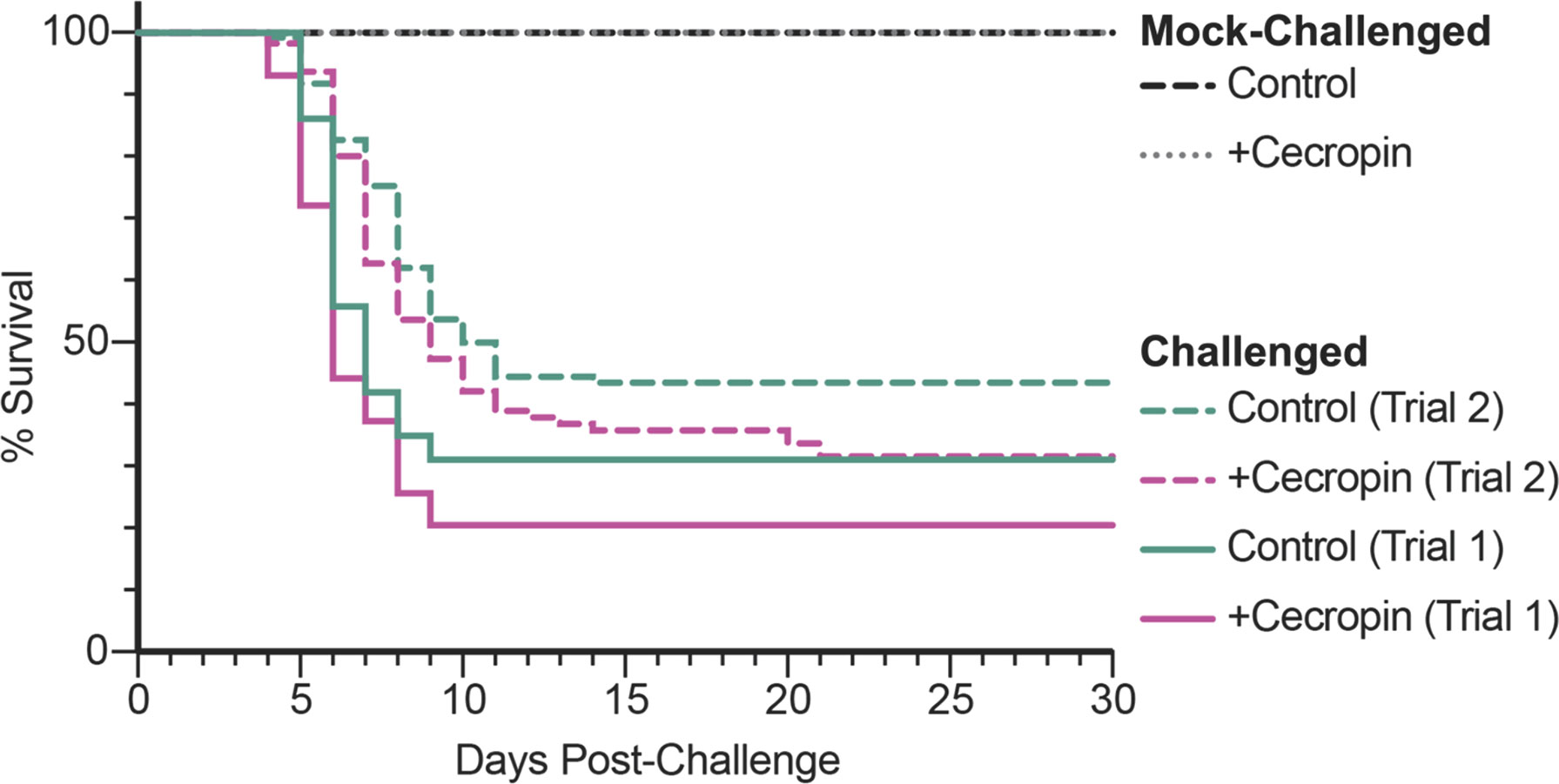
Figure 2 Kaplan-Meier curve showing survival of fish fed either the control or the diet supplemented with cecropin A following an immersion challenge with Y. ruckeri. Each trial was analyzed independently. There were no statistical differences between the two diet groups for either trial according to the Gehan-Breslow-Wilcoxon test: p=0.15 for Trial 1, p=0.16 for Trial 2. No mortality was observed in mock-challenged fish fed either diet.
Cecropin A Did Not Reduce the Prevalence of Y. ruckeri in Intestines of Fish
While cecropin A did not significantly impact survival, we next assessed whether it had on an effect on the ability of fish to clear Y. ruckeri from the intestine following infection challenge. The presence of Y. ruckeri in the intestines of fish was monitored at 3, 8, and 30 days post-challenge using a qPCR assay described in a previous study (Sibinga and Marquis, 2021). In the first trial, Y. ruckeri was detected in 83% and 100% of fish at day 3 and 8, respectively, independent of the diet (Table 1). At 30 days post-challenge, 88% of fish in the control diet group and 100% of fish in the +cecropin diet group were positive for Y. ruckeri; this difference was not statistically significant. In the second trial, Y. ruckeri was detected in 88% of fish at day 3 in both the control and +cecropin diets, and in 100% of fish at day 8 independent of the diet. At 30 days post-challenge, 80% of fish in the control diet group and 100% of fish in the +cecropin diet group were positive for Y. ruckeri. No statistically significant differences in prevalence of carrier status were observed between diet groups for any of the time points sampled. There were signs, however, that the bacterial load of carrier fish in the +cecropin diet may have been elevated. 10 of the 11 samples with the lowest cq values (which correspond to high abundance of Y. ruckeri) across both trials were found in the +cecropin diet group (Figure S1). Based on a standard curve of purified Y. ruckeri DNA, these fish were all estimated to possess >1.5x104 bacterial cells per 20 mg intestinal tissue (data not shown).
Cecropin A Modifies the Intestinal Microbiome of Mock-Challenged Fish
We began our examination of the microbiomes in this study by comparing the differences between the control and +cecropin diet groups in mock-challenged fish. At the start of trial 1, the microbiomes were dominated by a single amplicon sequence variant (ASV) from the genus Mycoplasma (Figure 3A). Over time, the internal (alpha) diversity and richness of both microbial communities increased, as measured by Shannon index, though this increase was more modest in fish fed the +cecropin diet: at day 30 the control diet had significantly higher alpha diversity (Figure 3B). Given the initial high relative abundance of Mycoplasma sp., increases in alpha diversity roughly corresponded to decreases in Mycoplasma sp.; accordingly, control diet group fish had lower average relative abundance of Mycoplasma sp. The rapid increase in alpha diversity observed in the control diet group led to significantly different microbiomes between diet groups at day 30, as evaluated by PERMANOVA (Figure 3C). Using DESeq2, we identified a number of ASVs significantly enriched at day 30 in the microbiomes of mock-challenged fish fed the control diet: 28 of the 31 ASVs identified in this screen were putatively matched by 16S sequence to gram-negative phyla (Figure S2 and Table S3).
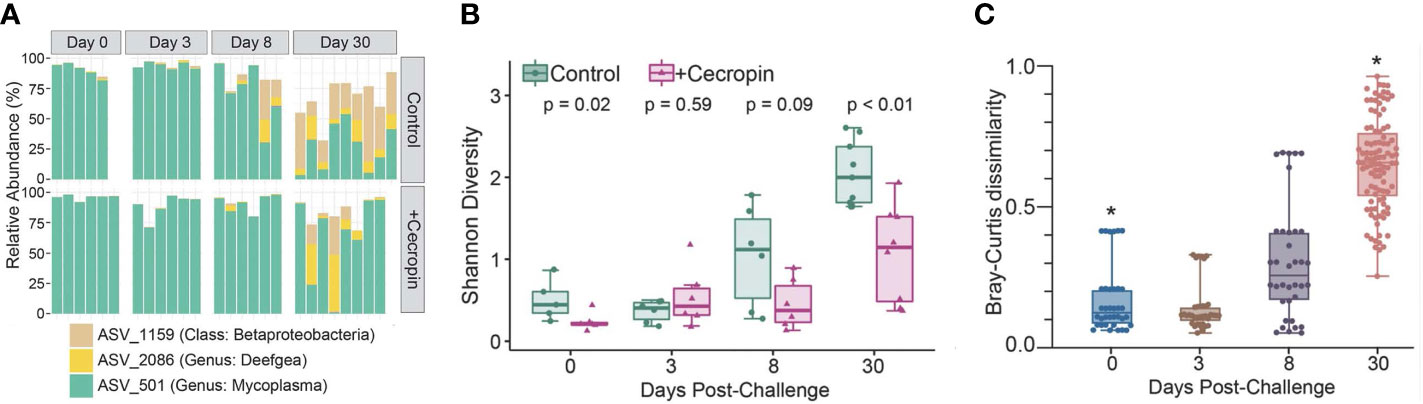
Figure 3 Microbiome comparison between mock-challenged fish fed either control or +cecropin diet. (A, B) Relative abundance of the core ASVs shifted over time. Microbiomes started out dominated by a single species of Mycoplasma. Alpha diversity increased in both diet groups over time, though by day 30 alpha diversity was significantly higher in fish fed the control diet. (C) Individual microbiomes of fish fed the control diet were compared to microbiomes of fish fed the +cecropin for each time point. Each point on the graph represents a pairwise Bray-Curtis distance between individual microbiomes from the two diet groups. Lower distance values represent more similar microbiomes, while higher distances represent more divergent microbiomes. Statistical significance was evaluated by PERMANOVA, initially for all timepoints and both diets, and then for post-hoc pairwise comparisons between diets for each timepoint. Statistical significance on the graph refers to the difference between diet groups for an individual timepoint, where q is the p-value after correction for multiple comparisons (*q<.05).
Cecropin A Influences the Alpha-Diversity of the Intestinal Microbiome of Fish Challenged by Bacterial Infection
We performed two challenge trials to investigate the effect of dietary cecropin on the fish microbiome in the context of infection. Fish in trial 2 were from the same origin and age cohort as those in trial 1 but had been maintained in a separate room in the Cornell aquatic facility for the duration of trial 1. Initial microbial community structure differed between the two trials, with fish at the start of trial 1 displaying higher average relative abundance of Mycoplasma sp. than those at the start of trial 2 (Figure 4A). The relative abundance of Mycoplasma sp. appeared to increase following the infection challenge in both trials but this effect was more pronounced in trial 2, owing to lower initial relative abundance (Figure 4A). Reflecting the observed patterns in Mycoplasma sp. relative abundance, alpha diversity was higher for most fish in the second trial than the first, but the same pattern was observed in both cases (Figure 4B). In fish challenged with Y. ruckeri, a drop in alpha diversity was observed from day 0 to day 3 independent of diet (Figure 4B). Alpha diversity subsequently appeared to increase in both groups at day 8 and again at day 30 post-challenge. The magnitude of these increases, however, was notably smaller for fish fed the control diet than for those fed the +cecropin diet. At day 30 post-challenge, alpha diversity was higher for fish fed the +cecropin diet in trial 1 (p=0.012); the same trend was apparent in trial 2, though in this case the difference between diet groups was not statistically significant (p=0.054) (Figure 4B). This suggests that dietary supplementation of cecropin A can improve the recovery of alpha diversity of the microbiome following Y. ruckeri infection.
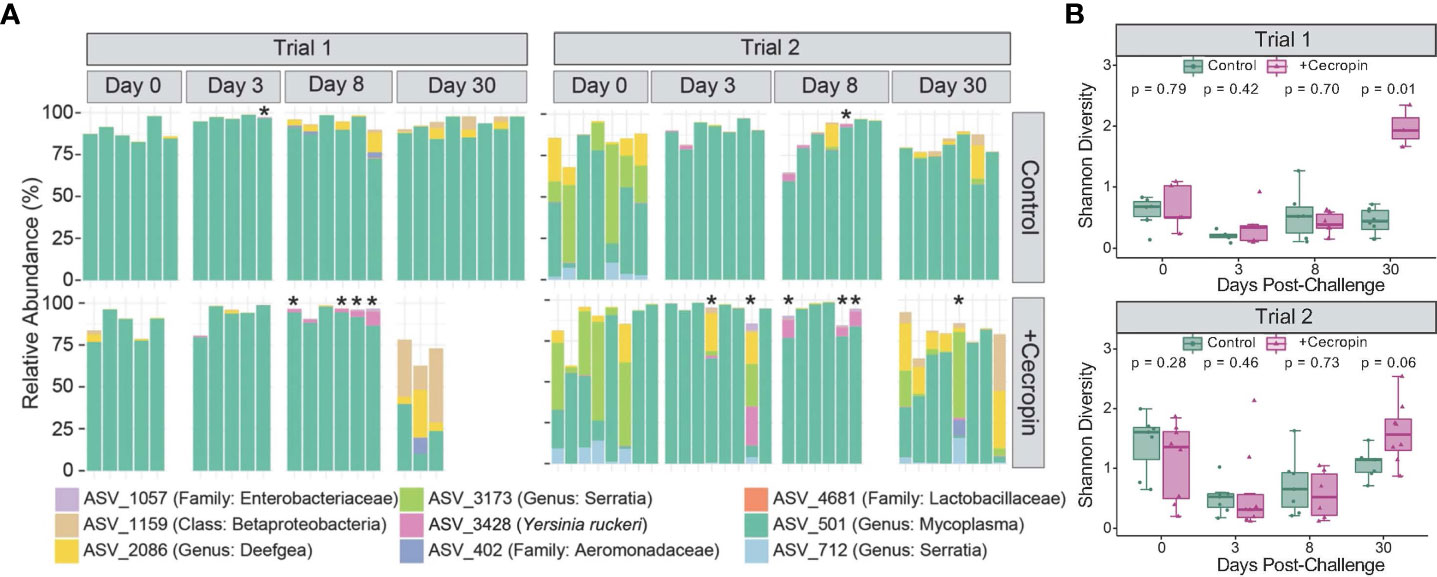
Figure 4 Microbiome comparison between fish challenged with Y. ruckeri fed either control or +cecropin diet. (A) Relative abundance of the 9 most prevalent taxa are shown for individual fish sampled before challenge (day 0) and post challenge (days 3, 8, and 30). Taxonomic assignments represent the most specific level of resolution assigned by Greengenes, with the exception of ASV_3428 which was identified by multiple methods (see text for details). Fish marked with an * had high absolute abundance of Y. ruckeri as measured by qPCR (detection after 25 or fewer cycles, corresponding to ~1.5x104 bacteria/20 mg of intestinal tissue). Baseline microbiomes at the start of trial 1 were markedly different from those at the start of trial 2. (B) Alpha diversity as measured by Shannon index for trial 1 and trial 2; p-values are for comparisons of diet groups at each timepoint, as assessed by Wilcoxon signed rank test with correction for multiple comparisons.
Identification of ASVs Corresponding to Y. ruckeri and Correlation Analysis
We next aimed to determine what microbiome states were associated with detection of Y. ruckeri. There was a strong correlation between Y. ruckeri qPCR threshold count, which depends on the abundance of copies of the target sequence at the beginning of the reaction, and the relative abundance of ASV_3428 and ASV_1057 (Figure S1). Using BLAST, both candidate ASVs’ sequences aligned to the published 16S sequence of the Y. ruckeri CSF007-82 strain used in this study (Nelson et al., 2015). Both sequence variants, which differed by one base pair, appeared in each challenge trial, suggesting that the frozen stock of Y. ruckeri used in this study may have contained both genotypes. Phylogenetic alignment of ASVs identified a total of 5 additional ASVs with at least 99.5% sequence identity to Y. ruckeri CSF007-82. All of these additional ASVs were extremely rare, however, occurring in fewer than 2% of fish sampled and only at very low abundance. A comparison of qPCR and sequencing data confirmed that while identification of carriers was possible using sequence data, this method was less sensitive than qPCR: only 30% of the fish identified as carriers by qPCR had sequence reads corresponding to Y. ruckeri after quality control and filtering.
ASV_3428 and ASV_1057 were then used to conduct a global correlation analysis in the microbiomes of challenged fish. Statistically significant correlations were identified for 14 ASVs (Figure 5). Six ASVs were found to have positive correlations to Y. ruckeri, including ASVs from the genera Flavobacterium, Achromobacter, and Sphingomonas. Seven ASVs were negatively correlated with Y. ruckeri, suggesting possible benefits for fish health. These ASVs included two strains identified as belonging to the family Lactobacillaceae, as well as ASV_1159, which was characterized only to the class level as a Betaproteobacteria in the GreenGenes database. ASV_1159 was detected in all but one fish sampled in this study, though the average relative abundance was less than 4%. Also negatively correlated with Y. ruckeri was the Mycoplasma sp. species that was dominant in most fish. The negative correlation of Y. ruckeri and Mycoplasma sp. at the individual level contrasts with the observation that at the fish population level exposure to Y. ruckeri led to an increase in Mycoplasma sp. relative abundance at (Figure 4A).
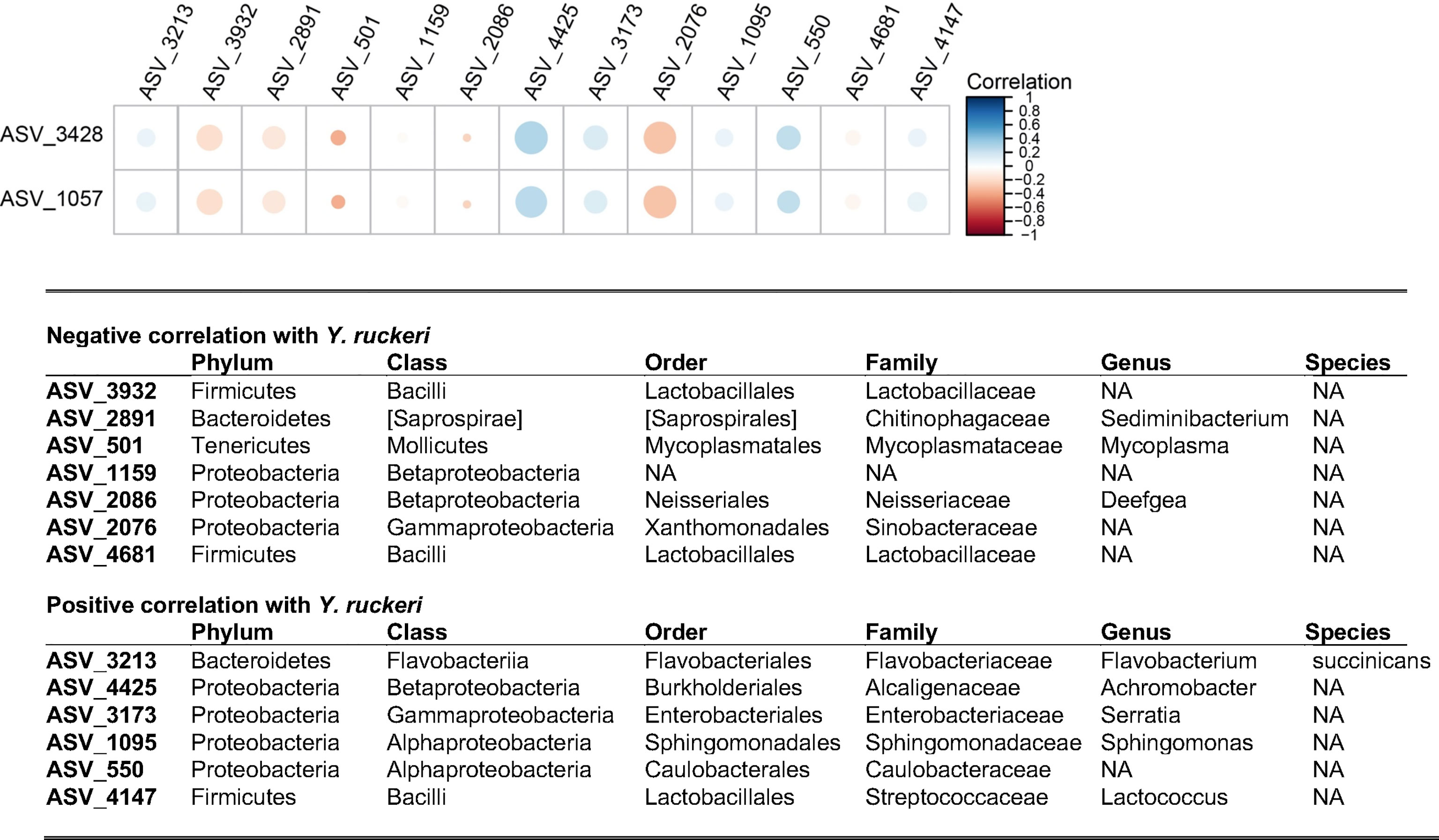
Figure 5 Correlation analysis between ASV_3428 and ASV_1057 identified as Y. ruckeri and elements of the microbiome. All taxa shown have a statistically significant correlation (p < 0.05) to Y. ruckeri after correction. The size of the circles represents the significance of the correlation (larger circles have lower p-values) and the color denotes the direction and strength of the correlation. For example, ASV_4425 is strongly positively correlated to ASV_3428 and ASV_1057 with high statistical significance. Taxonomic assignments for each ASV were generated by comparison to the Greengenes database. NA, Not Assigned.
Discussion
This project aimed to evaluate the impact of dietary cecropin A, an insect-derived AMP, on fish health. Specifically, this project aimed (i) to determine if dietary cecropin A aids in fighting colonization of the fish intestines by Y. ruckeri, the cause of enteric redmouth disease, and (ii) to evaluate the effects of dietary AMPs, such as cecropin A, on the integrity of the intestinal microbiota. First, we observed that dietary cecropin A had a slight negative effect on fish health, with trends towards decreased survival and increased pathogen load in fish that survived infection at the 30-day time point. Second, we observed that Mycoplasma sp. dominated for the most part the intestinal microbiome of fish independent of diet in the early stages of the experiment. By day 30, the microbiome of mock-challenged fish showed an increase in alpha diversity independent of diet. However, fish fed the +cecropin diet had higher relative abundance of the dominant strain of Mycoplasma sp. compared to fish on control diet. On the contrary, following a challenge with Y. ruckeri, the microbiome of surviving fish fed the +cecropin diet group showed higher alpha diversity and lower relative abundance of Mycoplasma sp. than the control diet group at the 30-day time point. Overall, these observations indicated that cecropin A was biologically active, although not beneficial to fish health when given as a feed ingredient. Nevertheless, the observed changes in intestinal microbiota of challenged and mock-challenged fish over a period of 30 days should contribute to a better understanding of the relationship between AMPs, microbiome composition, and fish health.
As expected, cecropin A proved to be inhibitory of Y. ruckeri in vitro. This supported our experimental approach, though it was of limited use in determining an effective oral dose for in vivo experiments. To set our experimental dose of cecropin A, we relied on previously published studies using cecropin as a feed additive in nile tilapia [75, 150, 225 mg/kg (Lin et al., 2015)] and crucian carp [100, 150, 200, 250 mg/kg (ZiRui et al., 2014)]. Both of these studies reported increased growth and survival in the context of an infection challenge, as well as measurable changes to physiological parameters including immune status and tissue composition at higher doses. A study in turbot using a higher dose range (250, 500, 750, 1000 mg/kg) reported negative effects on the microbiome diversity and disease resistance at 1000 mg/kg (Dai et al., 2020). Accordingly, we set our experimental dose at 110 mg of cecropin A per kg of feed. The moisture content of the +cecropin diet was 9.2% compared to 6.2% for the control diet. This could be the result of suboptimal storage – the diets were kept at -20°C and daily opening of the cold container in the humid conditions of the aquatic facility may have resulted in accumulation of condensation; because there was only a small amount of +cecropin feed remaining at the end of the study, this effect would have been more pronounced for this diet. While we cannot rule out the possibility that this had an effect on the nutritional quality of the +cecropin feed, both diets were comfortably above the published requirement for rainbow trout of this size (National Research Council, 2011).
We observed a trend towards lower survival in the +cecropin diet following a challenge with Y. ruckeri, though this difference was not statistically significant. Overall survival was lower than anticipated, resulting in fewer samples for microbiome analysis. We subsequently repeated the challenge in a second trial using fish from the same cohort and larger sample sizes. In the second trial, survival in both groups was somewhat improved relative to the first trial, which could be explained by the fact that younger fish are more susceptible to infection with Y. ruckeri (Ohtani et al., 2019). The trend, however, was similar to the first trial with a lower survival rate in the group of fish fed a diet supplemented with cecropin, though again not statistically significant.
Since dietary cecropin did not improve survival, we next tried to determine if it could reduce the prevalence of Y. ruckeri in the trout intestine over the course of infection and recovery. We found no significant differences in the likelihood of detection between diet groups for any timepoint, suggesting that cecropin did not help eliminate Y. ruckeri from the gut. We did, however, observe that most of the fish with high absolute abundance of Y. ruckeri as measured by qPCR of intestinal DNA extracts came from the +cecropin diet group, possibly suggesting that this group experienced more severe infections.
The microbiomes of fish in this study started out, and to some extent remained, overwhelmingly dominated by a single taxon identified as belonging to the genus Mycoplasma. At the beginning of the first trial, Mycoplasma sp. accounted for at least 75% of the sequence reads in all sampled fish. For fish not exposed to the infection challenge, however, the proportion of Mycoplasma sp. tended to decrease over time, especially in fish fed the control diet (Figure 3A). Though the larger fish in the second trial began with lower mean relative abundances of Mycoplasma sp. compared to those in the first trial, in both trials we observed that exposure to Y. ruckeri was linked to higher mean Mycoplasma sp. relative abundance by day three post-challenge (Figure 4A). It should be noted that in challenged fish, decreased feed intake from day 3-14 introduces an additional variable. We extracted DNA from whole intestinal samples, so lower feed intake would be expected to alter the ratio of digesta to mucosa in these samples. In fish, digesta samples tend to have higher microbial diversity than mucosa samples (Gajardo et al., 2016); decreased feed intake post-challenge could therefore help explain the observed decrease in alpha diversity in this period. Interestingly, while exposure to Y. ruckeri led to higher Mycoplasma sp. levels at the population level, for individual fish higher detected levels of Y. ruckeri were associated with lower relative abundance of Mycoplasma sp. (Figure 5 and Figure S1). This suggests that relative abundance of Mycoplasma sp. may be an indicator of the ability of the fish to maintain low pathogen loads.
In addition to Mycoplasma sp., negative correlations were observed between Y. ruckeri and six other ASVs, including two from the family Lactobacillaceae (Figure 5). These are strong candidates to be considered health-promoting members of the microbiome as certain Lactobacillus spp. are well-established probiotics, and members of this family have been shown to improve growth and resistance to bacterial infection in rainbow trout (Mohammadian et al., 2019). On the other hand, positive correlations to Y. ruckeri included Flavobacterium succinicans, which has previously been identified as a likely opportunistic pathogen in the context of bacterial gill disease (Good et al., 2015). Additional positive correlations included genus-level identifications that are consistent with previously reported fish pathogens: Serratia (McIntosh and Austin, 1990; Baya et al., 1992) and Lactococcus (Karami et al., 2019). Furthermore, a member of the Sphingomonas genus has recently been reported as an opportunistic pathogen of rainbow trout (Çanak et al., 2021). This suggests that Y. ruckeri infection created a niche for the opportunistic expansion of other potential pathogens.
Mycoplasma sp. relative abundance in the intestine has recently been proposed as a biomarker of health in rainbow trout (Rasmussen et al., 2022). One of the earliest sequence-based analyses of the salmonid gut revealed that Mycoplasma sp. comprised more than 95% of the microbiome in wild Atlantic salmon (Salmo salar) (Holben et al., 2002). More recently, Mycoplasma sp. has been recognized as a natural and likely beneficial component of the salmonid microbiome (Llewellyn et al., 2016; Huyben et al., 2018; Heys et al., 2020; Rasmussen et al., 2021). Increased relative abundance of Mycoplasma sp. in the intestine has been shown to correlate with greater resistance to disease (Li et al., 2016; Karlsen et al., 2017; Brown et al., 2019; Bozzi et al., 2021). Furthermore, in systems where Mycoplasma sp. is present, it frequently comprises a majority of all sequence reads; this is consistent with the observation that for salmonids, lower alpha diversity is at least in some cases associated with microbiome health rather than dysbiosis (Karlsen et al., 2017; Llewellyn et al., 2017; Vasemägi et al., 2017; Lavoie et al., 2018; Zhang et al., 2018).
Our study offers a rare longitudinal view of Mycoplasma sp. abundance in the trout gut before, during, and after an acute infection challenge. Our data show that average Mycoplasma sp. relative abundance was elevated in DNA extracted from whole intestines in the time points immediately following the bacterial challenge. This is in contrast to the findings of Rasmussen et al. who saw marked decreases in average Mycoplasma sp. relative abundance in the digesta of trout fed a control diet following Y. ruckeri infection (Rasmussen et al., 2022). However, in keeping with that study, we also observed that during infection, high Mycoplasma sp. relative abundance at the individual fish level was associated with lower relative abundance of Y. ruckeri (Figure 5).
A recent survey of wild and hatchery-reared Atlantic salmon from the United Kingdom and France identified Mycoplasma sp. as more abundant in hatchery fish than wild fish and as the sole core element of the microbiome across three separate hatcheries (present in >80% of individuals), though the relative abundance of Mycoplasma sp. varied substantially by hatchery (Webster et al., 2018). The fish in this study were received from a hatchery where they were kept at high stocking density. Over the experimental period, we observed a trend towards reduction in Mycoplasma sp. relative abundance, and an increase in alpha diversity (Figure 3A). Brown et al. observed differences in Mycoplasma sp. relative abundance and alpha diversity of rainbow trout held at different densities (Brown et al., 2019), suggesting that the lower stocking density in our facility may have played a role in this shift. This highlights a potential larger issue in the design of microbiome-oriented studies of fish disease: research settings are unlikely to replicate the stocking density and immune stress experienced by fish in farm and hatchery settings. It should be noted that Mycoplasma sp. has not been found in all studies of the salmonid microbiome, including two high-profile papers that sought to define reference microbiomes for Atlantic salmon (Gajardo et al., 2016) and rainbow trout (Wong et al., 2013). The factors that favor proliferation of Mycoplasma sp. in the microbiome are a topic worthy of further study.
Interestingly, the population-level decline in Mycoplasma sp. relative abundance over time that took place in mock-challenged fish was less pronounced in this study for fish fed the +cecropin diet (Figure 3A), though the mechanism underlying this difference remains unclear. The difference in microbiomes between the two diet groups could potentially help explain the reported effectiveness of longer-term prophylactic treatment of fish with AMPs prior to infection challenge (Lee et al., 2019; Rashidian et al., 2021; Simora et al., 2021). However, when fish had been challenged by exposure to a bacterial pathogen, the opposite was true: infection challenged fish fed the +cecropin diet had a more pronounced drop off in Mycoplasma sp. relative abundance at day 30 post-challenge than fish fed the control diet, suggesting that cecropin A may have interfered with innate resistance mechanisms to infection.
This study highlights the complexity of the interactions between AMPs, the microbiome, and the host response to infection. Cecropin did not prove to be effective at preventing mortality, and if anything appeared to exacerbate the infectious burden in this context. That said, there are many variables that we did not test that could affect this outcome – for example, the dose of cecropin and the timing of the challenge relative to the introduction of the AMP. Examination of the fish microbiome over the course of infection supports the idea that Mycoplasma sp. is intimately involved in the fish resistance to colonization by Y. ruckeri. Any future use of AMP-based therapeutics in aquaculture settings will likely have to take into account the effects of AMPs on the host microbiome and immune system.
Data Availability Statement
The datasets presented in this study can be found in online repositories. The names of the repository/repositories and accession number(s) can be found below: NCBI [accession:PRJNA818995].
Ethics Statement
The animal study was reviewed and approved by Cornell University Institutional Animal Care and Use Committee.
Author Contributions
NS, VS, and HM contributed to conception and design of the study. NS performed the experiments. M-TL and EJ performed the sequencing and organized the database. M-TL and NS analyzed the data. NS drafted the manuscript. M-TL and HM wrote sections of the manuscript. All authors contributed to manuscript revision, read, and approved the submitted version.
Funding
This work is supported by AFRI NIFA Fellowships Grant Program, Award Number: 12680741 from the USDA National Institute of Food and Agriculture.
Conflict of Interest
The authors declare that the research was conducted in the absence of any commercial or financial relationships that could be construed as a potential conflict of interest.
Publisher’s Note
All claims expressed in this article are solely those of the authors and do not necessarily represent those of their affiliated organizations, or those of the publisher, the editors and the reviewers. Any product that may be evaluated in this article, or claim that may be made by its manufacturer, is not guaranteed or endorsed by the publisher.
Acknowledgments
From Cornell University, we are grateful to Kevin Besler for technical assistance with sample preparation for sequencing, Dr. Alina Demeter for analysis of histopathology samples, and Lynn Johnson from the Cornell Statistical Consulting Unit for help with interpretation and visualization of data. Ken Osika of the New York State Department of Environmental Conservation, Bath Hatchery, kindly contributed the fish used in this study.
Supplementary Material
The Supplementary Material for this article can be found online at: https://www.frontiersin.org/articles/10.3389/fmars.2022.901389/full#supplementary-material
References
Austin B. (2012). Infectious Disease in Aquaculture Prevention and Control (Oxford; Philadelphia: Woodhead Pub. Ltd).
Baya A. M., Toranzo A. E., Lupiani B., Santos Y., Hetrick F. M. (1992). Serratia Marcescens: a Potential Pathogen for Fish. J. Fish Dis. 15, 15–26. doi: 10.1111/j.1365-2761.1992.tb00632.x
Blighe K., Rana S., Lewis M. (2022). EnhancedVolcano: Publication-Ready Volcano Plots With Enhanced Colouring and Labeling R package version 1.14.0. Available at: https://github.com/kevinblighe/EnhancedVolcano.
Bolyen E., Rideout J. R., Dillon M. R., Bokulich N. A., Abnet C. C., Al-Ghalith G. A., et al. (2019). Reproducible, Interactive, Scalable and Extensible Microbiome Data Science Using QIIME 2. Nat. Biotechnol. 37, 852–857. doi: 10.1038/s41587-019-0209-9
Bozzi D., Rasmussen J. A., Carøe C., Sveier H., Nordøy K., Gilbert M. T. P., et al. (2021). Salmon Gut Microbiota Correlates With Disease Infection Status: Potential for Monitoring Health in Farmed Animals. Anim. Microbiome 3, 30. doi: 10.1186/s42523-021-00096-2
Brown R. M., Wiens G. D., Salinas I. (2019). Analysis of the Gut and Gill Microbiome of Resistant and Susceptible Lines of Rainbow Trout (Oncorhynchus Mykiss). Fish Shellfish Immunol. 86, 497–506. doi: 10.1016/j.fsi.2018.11.079
Cabello F. C., Godfrey H. P. (2019). Salmon Aquaculture, Piscirickettsia Salmonis Virulence, and One Health: Dealing With Harmful Synergies Between Heavy Antimicrobial Use and Piscine and Human Health. Aquaculture 507, 451–456. doi: 10.1016/j.aquaculture.2019.04.048
Cabello F. C., Godfrey H. P., Buschmann A. H., Dölz H. J. (2016). Aquaculture as Yet Another Environmental Gateway to the Development and Globalisation of Antimicrobial Resistance. Lancet Infect. Dis. 16, e127–e133. doi: 10.1016/S1473-3099(16)00100-6
Callahan B. J., McMurdie P. J., Rosen M. J., Han A. W., Johnson A. J. A., Holmes S. P. (2016). DADA2: High-Resolution Sample Inference From Illumina Amplicon Data. Nat. Methods 13, 581–583. doi: 10.1038/nmeth.3869
Çanak Ö., Akayli T., Ürkü Ç. (2021). A Mixed Bacillus Gibsonii and Sphingomonas Echinoides Infection in Cultured Rainbow Trout (Oncorhynchus Mykiss). Mar. Life Sci. 3, 71–79. doi: 10.51756/marlife.999539
Caporaso J. G., Kuczynski J., Stombaugh J., Bittinger K., Bushman F. D., Costello E. K., et al. (2010). QIIME Allows Analysis of High-Throughput Community Sequencing Data. Nat. Methods 7, 335–336. doi: 10.1038/nmeth.f.303
Caporaso J. G., Lauber C. L., Walters W. A., Berg-Lyons D., Lozupone C. A., Turnbaugh P. J., et al. (2011). Global Patterns of 16S rRNA Diversity at a Depth of Millions of Sequences Per Sample. Proc. Natl. Acad. Sci. 108, 4516–4522. doi: 10.1073/pnas.1000080107
Chen S.-W., Liu C.-H., Hu S.-Y. (2019). Dietary Administration of Probiotic Paenibacillus Ehimensis NPUST1 With Bacteriocin-Like Activity Improves Growth Performance and Immunity Against Aeromonas Hydrophila and Streptococcus Iniae in Nile Tilapia (Oreochromis Niloticus). Fish Shellfish Immunol. 84, 695–703. doi: 10.1016/j.fsi.2018.10.059
Chettri J. K., Mehrdana F., Hansen E. B., Ebbensgaard A., Overgaard M. T., Lauritsen A. H., et al. (2017). Antimicrobial Peptide CAP18 and its Effect on Yersinia Ruckeri Infections in Rainbow Trout Oncorhynchus Mykiss (Walbaum): Comparing Administration by Injection and Oral Routes. J. Fish Dis. 40, 97–104. doi: 10.1111/jfd.12497
Chuah L.-O., Effarizah M. E., Goni A. M., Rusul G. (2016). Antibiotic Application and Emergence of Multiple Antibiotic Resistance (MAR) in Global Catfish Aquaculture. Curr. Envir. Health Rpt. 3, 118–127. doi: 10.1007/s40572-016-0091-2
Dai J., Zheng J., Ou W., Xu W., Ai Q., Zhang W., et al. (2020). The Effect of Dietary Cecropin AD on Intestinal Health, Immune Response and Disease Resistance of Juvenile Turbot (Scophthalmus Maximus L.). Fish Shellfish Immunol. 100, 117–125. doi: 10.1016/j.fsi.2020.02.052
de Bruijn I., Liu Y., Wiegertjes G. F., Raaijmakers J. M. (2018). Exploring Fish Microbial Communities to Mitigate Emerging Diseases in Aquaculture. FEMS Microbiol. Ecol. 94. doi: 10.1093/femsec/fix161
de Sousa E. L., Assane I. M., Santos-Filho N. A., Cilli E. M., de Jesus R. B., Pilarski F. (2021). Haematological, Biochemical and Immunological Biomarkers, Antibacterial Activity, and Survival in Nile Tilapia Oreochromis Niloticus After Treatment Using Antimicrobial Peptide LL-37 Against Streptococcus Agalactiae. Aquaculture 533, 736181. doi: 10.1016/j.aquaculture.2020.736181
Dong X.-Q., Zhang D.-M., Chen Y.-K., Wang Q.-J., Yang Y.-Y. (2015). Effects of Antimicrobial Peptides (AMPs) on Blood Biochemical Parameters, Antioxidase Activity, and Immune Function in the Common Carp (Cyprinus Carpio). Fish Shellfish Immunol. 47, 429–434. doi: 10.1016/j.fsi.2015.09.030
Fjell C. D., Hiss J. A., Hancock R. E. W., Schneider G. (2012). Designing Antimicrobial Peptides: Form Follows Function. Nat. Rev. Drug Discov. 11, 37–51. doi: 10.1038/nrd3591
Gajardo K., Rodiles A., Kortner T. M., Krogdahl Å., Bakke A. M., Merrifield D. L., et al. (2016). A High-Resolution Map of the Gut Microbiota in Atlantic Salmon (Salmo Salar): A Basis for Comparative Gut Microbial Research. Sci. Rep. 6, 30893. doi: 10.1038/srep30893
Gauthier J., Rouleau-Breton S., J. Charette S., Derome N. (2019). Stimulated Growth and Innate Immunity in Brook Charr (Salvelinus Fontinalis) Treated With a General Probiotic (Bactocell®) and Two Endogenous Probiotics That Inhibit Aeromonas Salmonicida In Vitro. Microorganisms 7, 193. doi: 10.3390/microorganisms7070193
Ge H., Wang Q., Chen H., Liu G., Pan Y., Chen J., et al. (2020). Effects of Antimicrobial Peptide APSH-07 on the Growth Performance, Anti-Oxidation Responses, Stress Resistance and Intestine Microbiota in Large Yellow Croaker Larimichthys Crocea. Aquacult. Nutr. 26, 715–726. doi: 10.1111/anu.13031
Ghosh B., Crosbie P. B. B., Nowak B. F., Bridle A. R. (2018). A Highly Sensitive, non-Invasive qPCR-Based Strategy for Direct Quantification of Yersinia Ruckeri in Fish Faeces. J. Fish Dis. 41, 1421–1428. doi: 10.1111/jfd.12839
Good C., Davidson J., Wiens G. D., Welch T. J., Summerfelt S. (2015). Flavobacterium Branchiophilum and F. Succinicans Associated With Bacterial Gill Disease in Rainbow Trout Oncorhynchus Mykiss (Walbaum) in Water Recirculation Aquaculture Systems. J. Fish Dis. 38, 409–413. doi: 10.1111/jfd.12249
Gutierrez D., Weinstock A., Antharam V. C., Gu H., Jasbi P., Shi X., et al. (2020). Antibiotic-Induced Gut Metabolome and Microbiome Alterations Increase the Susceptibility to Candida Albicans Colonization in the Gastrointestinal Tract. FEMS Microbiol. Ecol. 96, fiz187. doi: 10.1093/femsec/fiz187
He S., Wang Q., Li S., Ran C., Guo X., Zhang Z., et al. (2017). Antibiotic Growth Promoter Olaquindox Increases Pathogen Susceptibility in Fish by Inducing Gut Microbiota Dysbiosis. Sci. China Life Sci. 60, 1260–1270. doi: 10.1007/s11427-016-9072-6
Heys C., Cheaib B., Busetti A., Kazlauskaite R., Maier L., Sloan W. T., et al. (2020). Neutral Processes Dominate Microbial Community Assembly in Atlantic Salmon, Salmo Salar. Appl. Environ. Microbiol. 86, e02283–e02219. doi: 10.1128/AEM.02283-19
Holben W. E., Williams P., Saarinen M., Särkilahti L. K., Apajalahti J. H. A. (2002). Phylogenetic Analysis of Intestinal Microflora Indicates a Novel Mycoplasma Phylotype in Farmed and Wild Salmon. Microbial. Ecol. 44, 175–185. doi: 10.1007/s00248-002-1011-6
Hu Q.-Y., Wu P., Feng L., Jiang W.-D., Liu Y., Kuang S.-Y., et al. (2021). Antimicrobial Peptide Isalo Scorpion Cytotoxic Peptide (IsCT) Enhanced Growth Performance and Improved Intestinal Immune Function Associated With Janus Kinases (JAKs)/signal Transducers and Activators of Transcription (STATs) Signalling Pathways in on-Growing Grass Carp (Ctenopharyngodon Idella). Aquaculture 539, 736585. doi: 10.1016/j.aquaculture.2021.736585
Huyben D., Sun L., Moccia R., Kiessling A., Dicksved J., Lundh T. (2018). Dietary Live Yeast and Increased Water Temperature Influence the Gut Microbiota of Rainbow Trout. J. Appl. Microbiol. 124, 1377–1392. doi: 10.1111/jam.13738
Ibrahim M., Ahmad F., Yaqub B., Ramzan A., Imran A., Afzaal M., et al. (2020). “Chapter 4 - Current Trends of Antimicrobials Used in Food Animals and Aquaculture” in Antibiotics and Antimicrobial Resistance Genes in the Environment Advances in Environmental Pollution Research Series. Ed. Hashmi M. Z. (Elsevier), 39–69. doi: 10.1016/B978-0-12-818882-8.00004-8
Infante-Villamil S., Huerlimann R., Jerry D. R. (2021). Microbiome Diversity and Dysbiosis in Aquaculture. Rev. Aquacult. 13, 1077–1096. doi: 10.1111/raq.12513
Ingerslev H.-C., Strube M. L., Jørgensen L., von G., Dalsgaard I., Boye M., et al. (2014). Diet Type Dictates the Gut Microbiota and the Immune Response Against Yersinia Ruckeri in Rainbow Trout (Oncorhynchus Mykiss). Fish Shellfish Immunol. 40, 624–633. doi: 10.1016/j.fsi.2014.08.021
Joo H.-S., Fu C.-I., Otto M. (2016). Bacterial Strategies of Resistance to Antimicrobial Peptides. Philos. Trans. R Soc. Lond. B Biol. Sci. 371, 20150292. doi: 10.1098/rstb.2015.0292
Karami E., Alishahi M., Molayemraftar T., Ghorbanpour M., Tabandeh M. R., Mohammadian T. (2019). Study of Pathogenicity and Severity of Lactococcus Garvieae Isolated From Rainbow Trout (Oncorhynchus Mykiss) Farms in Kohkilooieh and Boyerahmad Province. Fish. Aquat. Sci. 22, 21. doi: 10.1186/s41240-019-0135-2
Karlsen C., Ottem K. F., Brevik Ø.J., Davey M., Sørum H., Winther-Larsen H. C. (2017). The Environmental and Host-Associated Bacterial Microbiota of Arctic Seawater-Farmed Atlantic Salmon With Ulcerative Disorders. J. Fish Dis. 40, 1645–1663. doi: 10.1111/jfd.12632
Lahti L., Shetty S. (2012) Microbiome R Package. In: Microbiome. Available at: https://github.com/microbiome/microbiome/blob/1db2fdb9c1f27e01deb158f72eb89548bddc710f/inst/CITATION (Accessed March 18, 2022).
Lahti L., Shetty S. (2019) Microbiome R Package. Available at: http://microbiome.github.io.
Langdon A., Crook N., Dantas G. (2016). The Effects of Antibiotics on the Microbiome Throughout Development and Alternative Approaches for Therapeutic Modulation. Genome Med. 8, 1–16. doi: 10.1186/s13073-016-0294-z
Lavoie C., Courcelle M., Redivo B., Derome N. (2018). Structural and Compositional Mismatch Between Captive and Wild Atlantic Salmon (Salmo Salar) Parrs’ Gut Microbiota Highlights the Relevance of Integrating Molecular Ecology for Management and Conservation Methods. Evol. Appl. 11, 1671–1685. doi: 10.1111/eva.12658
Lazzaro B. P., Zasloff M., Rolff J. (2020). Antimicrobial Peptides: Application Informed by Evolution. Science 368, eaau5480. doi: 10.1126/science.aau5480
Lee B.-C., Hung C.-W., Lin C.-Y., Shih C.-H., Tsai H.-J. (2019). Oral Administration of Transgenic Biosafe Microorganism Containing Antimicrobial Peptide Enhances the Survival of Tilapia Fry Infected Bacterial Pathogen. Fish Shellfish Immunol. 95, 606–616. doi: 10.1016/j.fsi.2019.10.052
Legrand T. P. R. A., Wynne J. W., Weyrich L. S., Oxley A. P. A. (2020). A Microbial Sea of Possibilities: Current Knowledge and Prospects for an Improved Understanding of the Fish Microbiome. Rev. Aquacult. 12, 1101–1134. doi: 10.1111/raq.12375
Lemaitre B., Reichhart J.-M., Hoffmann J. A. (1997). Drosophila Host Defense: Differential Induction of Antimicrobial Peptide Genes After Infection by Various Classes of Microorganisms. Proc. Natl. Acad. Sci. 94, 14614–14619. doi: 10.1073/pnas.94.26.14614
Li T., Long M., Ji C., Shen Z., Gatesoupe F.-J., Zhang X., et al. (2016). Alterations of the Gut Microbiome of Largemouth Bronze Gudgeon (Coreius Guichenoti) Suffering From Furunculosis. Sci. Rep. 6, 30606. doi: 10.1038/srep30606
Lin X., Chen W., Lin S., Luo L. (2015). Effects of Dietary Cecropin on Growth, non-Specific Immunity and Disease Resistance of Tilapia (Oreochromis Niloticus × O. Aureus). Aquacult. Res. 46, 2999–3007. doi: 10.1111/are.12457
Liu M., Wang B., Jiang K., Gong K., Sun S., Wang L., et al. (2014). Rice Bran Expressing a Shrimp Antimicrobial Peptide Confers Delayed Spoilage of Fish Feed and Resistance of Tilapia to Aeromonas Hydrophila. J. World Aquacult. Soc. 45, 269–278. doi: 10.1111/jwas.12121
Llewellyn M. S., Leadbeater S., Garcia C., Sylvain F.-E., Custodio M., Ang K. P., et al. (2017). Parasitism Perturbs the Mucosal Microbiome of Atlantic Salmon. Sci. Rep. 7, 43465. doi: 10.1038/srep43465
Llewellyn M. S., McGinnity P., Dionne M., Letourneau J., Thonier F., Carvalho G. R., et al. (2016). The Biogeography of the Atlantic Salmon (Salmo Salar) Gut Microbiome. ISME J. 10, 1280–1284. doi: 10.1038/ismej.2015.189
Love M. I., Huber W., Anders S. (2014). Moderated Estimation of Fold Change and Dispersion for RNA-Seq Data With Deseq2. Genome Biol. 15, 550. doi: 10.1186/s13059-014-0550-8
Lygren B., Sveier H., Hjeltnes B., Waagbø R. (1999). Examination of the Immunomodulatory Properties and the Effect on Disease Resistance of Dietary Bovine Lactoferrin and Vitamin C Fed to Atlantic Salmon (Salmo Salar) for a Short-Term Period. Fish Shellfish Immunol. 9, 95–107. doi: 10.1006/fsim.1998.0179
McDonald D., Price M. N., Goodrich J., Nawrocki E. P., DeSantis T. Z., Probst A., et al. (2012). An Improved Greengenes Taxonomy With Explicit Ranks for Ecological and Evolutionary Analyses of Bacteria and Archaea. ISME J. 6, 610–618. doi: 10.1038/ismej.2011.139
McIntosh D., Austin B. (1990). Recovery of an Extremely Proteolytic Form of Serratia Liquefaciens as a Pathogen of Atlantic Salmon, Salmo Solar, in Scotland. J. Fish Biol. 36, 765–772. doi: 10.1111/j.1095-8649.1990.tb04330.x
McMurdie P. J., Holmes S. (2013). Phyloseq: An R Package for Reproducible Interactive Analysis and Graphics of Microbiome Census Data. PLoS One 8, e61217. doi: 10.1371/journal.pone.0061217
Millanao A. B., Barrientos M. H., Gómez C. C., Tomova A., Buschmann A., Dölz H., et al. (2011). Injudicious and Excessive Use of Antibiotics: Public Health and Salmon Aquaculture in Chile. Rev. Med. Chil 139, 107–118.
Mohammadian T., Nasirpour M., Tabandeh M. R., Heidary A. A., Ghanei-Motlagh R., Hosseini S. S. (2019). Administrations of Autochthonous Probiotics Altered Juvenile Rainbow Trout Oncorhynchus Mykiss Health Status, Growth Performance and Resistance to Lactococcus Garvieae, an Experimental Infection. Fish Shellfish Immunol. 86, 269–279. doi: 10.1016/j.fsi.2018.11.052
National Research Council (2011) Nutrient Requirements of Fish and Shrimp. Available at: https://www.nap.edu/catalog/13039/nutrient-requirements-of-fish-and-shrimp (Accessed May 2, 2017).
Nelson M. C., LaPatra S. E., Welch T. J., Graf J. (2015). Complete Genome Sequence of Yersinia Ruckeri Strain CSF007-82, Etiologic Agent of Red Mouth Disease in Salmonid Fish. Genome Announc. 3, e01491–e01414. doi: 10.1128/genomeA.01491-14
Ohtani M., Villumsen K. R., Strøm H. K., Lauritsen A. H., Aalbæk B., Dalsgaard I., et al. (2019). Effects of Fish Size and Route of Infection on Virulence of a Danish Yersinia Ruckeri O1 Biotype 2 Strain in Rainbow Trout (Oncorhynchus Mykiss). Aquaculture 503, 519–526. doi: 10.1016/j.aquaculture.2019.01.041
Rashidian G., Moosazadeh Moghaddam M., Mirnejad R., Mohammadi Azad Z. (2021). Supplementation of Zebrafish (Danio Rerio) Diet Using a Short Antimicrobial Peptide: Evaluation of Growth Performance, Immunomodulatory Function, Antioxidant Activity, and Disease Resistance. Fish Shellfish Immunol. 119, 42–50. doi: 10.1016/j.fsi.2021.09.035
Rasmussen J. A., Villumsen K. R., Duchêne D. A., Puetz L. C., Delmont T. O., Sveier H., et al. (2021). Genome-Resolved Metagenomics Suggests a Mutualistic Relationship Between Mycoplasma and Salmonid Hosts. Commun. Biol. 4, 1–10. doi: 10.1038/s42003-021-02105-1
Rasmussen J. A., Villumsen K. R., von Gersdorff Jørgensen L., Forberg T., Zuo S., Kania P. W., et al. (2022). Integrative Analyses of Probiotics, Pathogenic Infections and Host Immune Response Highlight the Importance of Gut Microbiota in Understanding Disease Recovery in Rainbow Trout (Oncorhynchus Mykiss). J. Appl. Microbiol. 134, 3201–3216 doi: 10.1111/jam.15433
Reid K. M., Patel S., Robinson A. J., Bu L., Jarungsriapisit J., Moore L. J., et al. (2017). Salmonid Alphavirus Infection Causes Skin Dysbiosis in Atlantic Salmon (Salmo Salar L.) Post-Smolts. PLoS One 12, e0172856. doi: 10.1371/journal.pone.0172856
Rico A., Phu T. M., Satapornvanit K., Min J., Shahabuddin A. M., Henriksson P. J. G., et al. (2013). Use of Veterinary Medicines, Feed Additives and Probiotics in Four Major Internationally Traded Aquaculture Species Farmed in Asia. Aquaculture 412–413, 231–243. doi: 10.1016/j.aquaculture.2013.07.028
Ringø E., Zhou Z., Vecino J. L. G., Wadsworth S., Romero J., Krogdahl Å., et al. (2016). Effect of Dietary Components on the Gut Microbiota of Aquatic Animals. A Never-Ending Story? Aquacult. Nutr. 22, 219–282. doi: 10.1111/anu.12346
Schar D., Klein E. Y., Laxminarayan R., Gilbert M., Van Boeckel T. P. (2020). Global Trends in Antimicrobial Use in Aquaculture. Sci. Rep. 10, 21878. doi: 10.1038/s41598-020-78849-3
Schmidt V., Gomez-Chiarri M., Roy C., Smith K., Amaral-Zettler L. (2017). Subtle Microbiome Manipulation Using Probiotics Reduces Antibiotic-Associated Mortality in Fish. mSystems 2, e00133–e00117. doi: 10.1128/mSystems.00133-17
She R., Li T.-T., Luo D., Li J.-B., Yin L.-Y., Li H., et al. (2017). Changes in the Intestinal Microbiota of Gibel Carp (Carassius Gibelio) Associated With Cyprinid Herpesvirus 2 (CyHV-2) Infection. Curr. Microbiol. 74, 1130–1136. doi: 10.1007/s00284-017-1294-y
Sibinga N. A., Marquis H. (2021). Tissue-Specific Differences in Detection of Yersinia Ruckeri Carrier Status in Rainbow Trout (Oncorhynchus Mykiss ). J. Fish Dis. 44, 2013–2020. doi: 10.1111/jfd.13515
Silveira R. F., Roque-Borda C. A., Vicente E. F. (2021). Antimicrobial Peptides as a Feed Additive Alternative to Animal Production, Food Safety and Public Health Implications: An Overview. Anim. Nutr. 7, 896–904. doi: 10.1016/j.aninu.2021.01.004
Simora R. M. C., Wang W., Coogan M., El Husseini N., Terhune J. S., Dunham R. A. (2021). Effectiveness of Cathelicidin Antimicrobial Peptide Against Ictalurid Catfish Bacterial Pathogens. J. Aquat. Anim. Health 33, 178–189. doi: 10.1002/aah.10131
Stentiford G. D., Sritunyalucksana K., Flegel T. W., Williams B. A. P., Withyachumnarnkul B., Itsathitphaisarn O., et al. (2017). New Paradigms to Help Solve the Global Aquaculture Disease Crisis. PoS Pathog. 13, e1006160. doi: 10.1371/journal.ppat.1006160
Theriot C. M., Koenigsknecht M. J., Carlson P. E., Hatton G. E., Nelson A. M., Li B., et al. (2014). Antibiotic-Induced Shifts in the Mouse Gut Microbiome and Metabolome Increase Susceptibility to Clostridium Difficile Infection. Nat. Commun. 5, 3114. doi: 10.1038/ncomms4114
Ting C.-H., Chen Y.-C., Chen J.-Y. (2018). Nile Tilapia Fry Fed on Antimicrobial Peptide Epinecidin-1-Expressing Artemia Cyst Exhibit Enhanced Immunity Against Acute Bacterial Infection. Fish Shellfish Immunol. 81, 37–48. doi: 10.1016/j.fsi.2018.07.008
Vasemägi A., Visse M., Kisand V. (2017). Effect of Environmental Factors and an Emerging Parasitic Disease on Gut Microbiome of Wild Salmonid Fish. mSphere 2, e00418–e00417. doi: 10.1128/mSphere.00418-17
Veidenberg A., Medlar A., Löytynoja A. (2016). Wasabi: An Integrated Platform for Evolutionary Sequence Analysis and Data Visualization. Mol. Biol. Evol. 33, 1126–1130. doi: 10.1093/molbev/msv333
Wang S., Xie S., Zhou A., Zhang C., Wen L., Xu G., et al. (2021). Effects of Mixed Antimicrobial Peptide on the Growth Performance, Antioxidant and Immune Responses and Disease Resistance of Pengze Crucian Carp (Carassius Auratus Var. Pengze). Fish Shellfish Immunol. 114, 112–118. doi: 10.1016/j.fsi.2021.04.017
Webster T. M. U., Consuegra S., Hitchings M., de Leaniz C. G. (2018). Interpopulation Variation in the Atlantic Salmon Microbiome Reflects Environmental and Genetic Diversity. Appl. Environ. Microbiol. 84, e00691-18 doi: 10.1128/AEM.00691-18
Wickham H., Averick M., Bryan J., Chang W., McGowan L. D., François R., et al. (2019). Welcome to the Tidyverse. J. Open Source Softw. 4, 1686. doi: 10.21105/joss.01686
Wong S., Waldrop T., Summerfelt S., Davidson J., Barrows F., Kenney P. B., et al. (2013)Aquacultured Rainbow Trout (Oncorhynchus Mykiss) Possess a Large Core Intestinal Microbiota That Is Resistant to Variation in Diet and Rearing Density (Accessed January 14, 2022).
Yi Y., Zhang Z., Zhao F., Liu H., Yu L., Zha J., et al. (2018). Probiotic Potential of Bacillus Velezensis JW: Antimicrobial Activity Against Fish Pathogenic Bacteria and Immune Enhancement Effects on Carassius Auratus. Fish Shellfish Immunol. 78, 322–330. doi: 10.1016/j.fsi.2018.04.055
Zhang X., Ding L., Yu Y., Kong W., Yin Y., Huang Z., et al. (2018)The Change of Teleost Skin Commensal Microbiota Is Associated With Skin Mucosal Transcriptomic Responses During Parasitic Infection by Ichthyophthirius Multifillis (Accessed March 16, 2022).
Zhou X., Wang Y., Li W. (2008). Effect of Feeding Apidaecin on Common Carp (Cyprinus Carpio) Growth Performances and Immune Function. Aquaculture 279, 108–112. doi: 10.1016/j.aquaculture.2008.04.024
Keywords: antimicrobial, AMP (antimicrobial peptides), infection challenge, aquaculture, Mycoplasma sp., one health
Citation: Sibinga NA, Lee M-T, Johnson EL, Selvaraj V and Marquis H (2022) Longitudinal Sampling of the Rainbow Trout (Oncorhynchus mykiss) Microbiome Reveals Effects of Dietary Cecropin A and Yersinia ruckeri Infection. Front. Mar. Sci. 9:901389. doi: 10.3389/fmars.2022.901389
Received: 21 March 2022; Accepted: 22 April 2022;
Published: 30 May 2022.
Edited by:
Yanjiao Zhang, Ocean University of China, ChinaReviewed by:
Yun-Zhang Sun, Jimei University, ChinaMonica Fengsrud Brinchmann, Nord University, Norway
Copyright © 2022 Sibinga, Lee, Johnson, Selvaraj and Marquis. This is an open-access article distributed under the terms of the Creative Commons Attribution License (CC BY). The use, distribution or reproduction in other forums is permitted, provided the original author(s) and the copyright owner(s) are credited and that the original publication in this journal is cited, in accordance with accepted academic practice. No use, distribution or reproduction is permitted which does not comply with these terms.
*Correspondence: Hélène Marquis, aG03MkBjb3JuZWxsLmVkdQ==