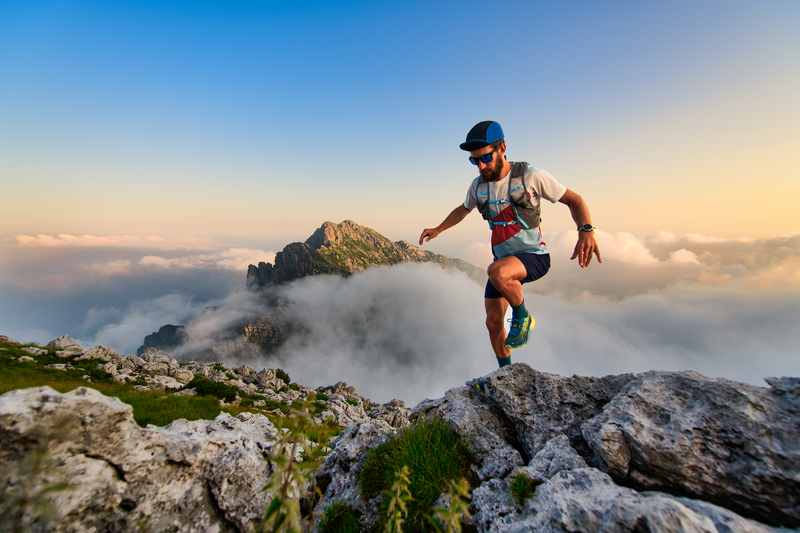
95% of researchers rate our articles as excellent or good
Learn more about the work of our research integrity team to safeguard the quality of each article we publish.
Find out more
ORIGINAL RESEARCH article
Front. Mar. Sci. , 24 June 2022
Sec. Marine Ecosystem Ecology
Volume 9 - 2022 | https://doi.org/10.3389/fmars.2022.900607
This article is part of the Research Topic Coralline Algae: Past, Present, and Future Perspectives View all 10 articles
Coralline algae (Rhodophyta, Florideophyceae) are one of the most abundant organisms in the hard-bottom marine photic zone where they provide settlement substrates, structure and shelter on rocky substrates. Coralline algae also play important roles in tropical reefs, both cementing corals together and producing substantial amounts of calcium carbonate. The ecological roles of coralline algae in the marine environment are related to the biomineralization process that occurs in the cell walls. Currently, this group of algae is receiving renewed attention from researchers from different fields, especially due to possible effects of climate changes over its magnesium calcite skeleton. Despite this renewed attention, we still have poor information regarding the first steps of coralline algae ontogeny and calcification. The aim of this study was to describe the earlier steps of Lithophyllum corallinae development and its calcification process. Algae were collected at Vermelha Beach, Rio de Janeiro city. Adult crusts released spores that settled over microscope slides. The germinating spores were analyzed by Polarizing Light Microscopy, Scanning Electron Microscopy, Energy Dispersive Spectroscopy, Transmission Electron Microscopy and Atomic Force Microscopy. Results showed that cell walls mineralization begins at third spore cell division (8 cells specimen), evidenced by changes in light polarization, elemental composition and hardness, restricted to the cell walls of the innermost part of the developing spore. Nanopores in calcite crystals structure were identified, evidencing macromolecules occlusion, a feature especially important to prevent calcite skeleton cracking in high-energy environments. The beginning of this process could be related to spore size, availability of organic matrix and energy from photosynthesis. All the analysis also confirmed the lack of calcification in the cell walls of the outermost part of the germinating spore, which allows the growth of the individual; but mainly indicates a high level of control in coralline algae mineralization process, representing a relevant information in future studies on coralline algae calcification, including those testing climate changes scenarios.
Coralline algae (Rhodophyta, Florideophyceae) are one of the most abundant organisms in the hard-bottom marine photic zone, where they are noticeable marine calcifiers, growing attached to the substrate (crustose coralline algae – CCA) or as free-living nodules, known as rhodoliths (Steneck, 1986; McCoy and Kamenos, 2015).
These algae are widespread in tropical and temperate intertidal and sub tidal environments, where they provide settlement substrates, structure and shelter for many marine organisms (McCoy and Kamenos, 2015). Coralline algae also play important roles in tropical reefs, both cementing corals together and producing substantial amounts of calcium carbonate (Foster, 2001; Nelson, 2009; Amado-Filho et al., 2012; Foster et al., 2013).
The ecological roles of coralline algae in the marine environment are related to the biomineralization process that occurs within its cell walls, which became heavily calcified (McCoy and Kamenos, 2015). Crystals formed by coralline algae are made of calcium carbonate, normally in the form of high magnesium calcite, Ca(Mg)CO3, which presents a high degree of Ca2+ substitution by Mg2+ at the crystal lattice (more than 4 wt.%), with the majority of species containing Mg substitution higher than 12 wt.% (Smith et al., 2012).
At the nanoscale, these crystals consisted of several single crystallites assembled and associated with the cell wall organic matter, with a high level of spatial organization. In addition, calcification in coralline algae is dependent both on the soluble and insoluble organic matrix, which are directly involved in the control of crystals formation as well as their spatial organization through an organic matrix-mediated process (Carvalho et al., 2017).
Regarding the early stages of coralline algae development, Johansen et al, 1981 observed that coralline algae spores in planktonic phase do not exhibit calcium carbonate crystals within the cell wall, which could help in flotation and dispersion of this spores. Later works suggested that the first steps of calcification occurred in some moment after the spore is attached to the hard substrate (Vesk and Borowitzka, 1984; Cabioch et al., 1986).
Currently, this group of algae is receiving renewed attention from researchers from different fields, especially due to possible effects of climate changes in seawater chemistry (McCoy and Kamenos, 2015). It is important to emphasize that, considering the fact that coralline algae form magnesium calcite crystals in their cell walls, this group of algae is more susceptible to possible skeletal dissolution promoted by changes in seawater chemistry driven by the higher absorption of CO2 (Andersson et al., 2008). Recently, Ordoñez et al., 2017 reported that several spore germination processes (i.e. formation of the germinating disc, initial growth and germiling survival) of coralline algae is negatively impacted by the independent and interactive effects of ocean acidification, increasing seawater temperature and irradiance intensity.
Despite this renewed attention, we still have poor information regarding the first steps of coralline algae calcification. Taking into account the threat against these calcifying organisms, it is important to expand the knowledge about the first steps of calcification in the current seawater pH for future comparisons with in vitro and in vivo experiments.
Thus, the aim of this study was to describe the earlier steps of development and mineralization process in Lithophyllum corallinae.
Lithophyllum coralline was choose for this study because it is one of the main coralline algae found over the Brazilian shelf and is also recognized in Brazil as an important rodolith-forming coralline algae (Bahia, 2014). In addition, it is also common in many sub-tropical and tropical habitats in the Atlantic and Indo-Pacific regions (Henriques et al., 2014). Small crusts of Lithophyllum corallinae were collected at Vermelha Beach localized in the city of Rio de Janeiro (Rio de Janeiro, 22° 95’38”S, 43°16’27”W) over the rocky shore by divers in depths ranging 3 to 5 meters. The adult samples were transferred to Algae Laboratory (from Rio de Janeiro Botanical Garden Research Institute) and maintained in a 400 L aquarium with synthetic seawater prepared with deionized water and synthetic salt (Red Sea Fish Pharm, Houston, USA) until spore release experiments (approx. 2 weeks). The aquarium was set to mimic abiotic in situ features, i.e. from the sampling site (Table 1). Water temperature, pH, total alkalinity, salinity, dissolved oxygen were measured with a YSI 556 Multiparameter (YSI Incorporated, Ohio, USA), light intensity was measured with HOBO Pedant sensors (ONSET, Massachusetts, USA) and Mg/Ca proportion was obtained by Ca and Mg concentration analysis with colorimetric rapid tests (Red Sea Fish Pharm, Houston, USA). To mimic field light conditions (i.e. light intensity, diurnal and nocturnal light cycle, etc) we used AI Vega lights (Aqua Illumination, Pennsylvania, USA). Adult Specimens were identified from morphological and reproductive characters (Supplementary Figure 1) observed in sections prepared using methods detailed in Maneveldt and Van Der Merwe, 2012.
Table 1 Natural and artificial seawater quality parameters measured at Vermelha Beach (Natural seawater) and aquarium.
For spore releasing, we induced the fertile adult crusts adapting a combination of previous methods (Ichiki et al., 2000; Ordoñez et al., 2017). The aquarium was kept under low light intensity (16 – 32 µmol photons m-2 s-1) during the diurnal light cycle and the aquarium temperature was reduced to 18°C for 4 hours. We did not quantify the number of released spores or the reproductive output of the L. corallinae adult crusts.
Microscopy slides (30 mm diameter; n = 80) were previously treated with 2/3 of ethanol (> 99.8%, absolute, Sigma-Aldrich) and 1/3 of hydrochloric acid (> 32% P.A., Sigma-Aldrich) solution to increase roughness and enhance the possibility of spore settlement (Fletcher and Callow, 1992). After the treatment, the slides were washed with distilled water, dried and glued with double-face tape (3M, Scotch, Brazil) over eleven 20 cm wood sticks (n = 7 slides per wood stick). These wood sticks were submerged into the 400 L aquarium where the Lithophyllum corallinae adult crusts were placed. The submersion was done in aquarium regions were the water flow were more intense in order to orientate this settlement substrate direct against the water flow, thus presenting a higher probability of planktonic spores to settle. Settlement of spores of L. corallinae were confirmed by optical microscopy, using previous literature that described the dimension, morphological features and cell division patterns exhibited by coralline algae spores (Chihara, 1974; Ichiki et al., 2000).
The first slides were removed from the aquarium after 4 hours of exposure to the water flow. Later slides were removed in different times (6, 8, 12 and 24 hours). To follow cell wall earlier calcification process in germinating spores of Lithophyllum corallinae, the slides were gently placed over microscope slides where 3 drops of seawater were pipetted. A Zeiss Axioplan-2 Optical Microscope with crossed polarizers and 20x objective lens was used to observe the presence of birefringent material, which could indicate the presence of the calcium carbonate crystals. A phase plate (λ = 551 nm) was also used to indicate the slow and the fast rays (higher and lower effective refractive indexes respectively) of the material. The higher effective refractive index of the phase plate is indicated by the double arrow in Figure 1.
Figure 1 Microscopic images of L. corallinae germinating spores in different development stages. 2, 4, 8, 16 and 32 germinating spores over the slides; Light microscopy (A–E), PLM (F–J) and PLM with phase plate (K–O). PLM showed the presence of polarized material inside the cell walls since the 2 cells spores (Figure 1F). PLM images with the utilization of a phase plate (λ = 551 nm) confirm the presence of calcite crystals with the c axes oriented perpendicular to the cell walls beginning from the 8 cell stage (K–O). Scale bar: 1A – 1C = 10 µm; 1D – 1E = 20 µm.
Ten slides observed by Polarized Light Microscopy (PLM) that presented the highest amount of settle individuals in different development stages were fixed (4% paraformaldehyde; 2.5% glutaraldeheyde; 0.1 M cacodylate; pH = 8.2), dehydrated in increasing concentrations of acetone at room temperature, dried at the CO2 critical point dryer equipment (Bal-Tec CPD030, Leica, Wetzlar, Germany) and coated with a thin carbon layer (108C Auto Carbon Coater, Ted Pella, California, USA). The slides were observed in a FEI Quanta 250 Scanning Electron Microscopy (SEM) and Energy Dispersive Spectroscopy (EDS) was performed with an EDAX Octane silicon drift x-ray detector (New Jersey, USA) operated at 15 kV. Ca and Mg maps from the young individuals were acquired in order to localize and compare the amount of these ions in different development stages.
Samples were prepared as described above. After critical point drying with CO2, coverslips adhered settle individuals were embedded overnight in spurr resin and polymerized for 24h at 70°C. After polymerization, samples were thawed onto liquid nitrogen to separate SPURR embedded settle individuals from glass cover slips. Ultrathin sections (80 nm) were obtained using a Leica EM UC7 ultramicrotome (Leica, Wetzlar, Germany), collected onto 300 mesh lacey carbon grids (Electron Microscopy Sciences-EMS). No staining was performed. Grids were observed on a Tecnai-T20 electron microscope (Thermosfisher) with a LaB6 source operating at 200 kV. Images were recorded using a 1k CCD camera (Veleta, Olympus). Electron diffraction patterns were obtained in the select areas at 1.75m camera lenght.
The production of calcium carbonate crystals in coralline cell walls can affect directly the topography and mechanical properties of these structures and the modification of these parameters could be a good indication of calcification initiation. To identify differences in the cell wall topography and mechanical properties between different development stages, Atomic Force Microscopy (AFM) images of 2 to 8 celled newly germinated individuals (3 hours after settlement) and 16 to 32 celled individuals (12 hours after settlement) were acquired using a Bioscope Catalyst (Bruker, California, USA) operated at the Peak Force Tapping mode (Pittenger et al., 2012). AFM is one of the Scanning Probe Microscopies techniques in which a cantilever with a very sharp tip is used to scan the sample (a few atoms of the tip that actually touch the sample), providing atomic resolution images. Recently, Peak Force Tapping mode (®Bruker, California, USA) was developed, in which the resulting force curve from the interaction between the tip and the sample could provide complementary information, such as elasticity (Young´s modulus), adhesion, dissipation and deformation. In this study, we obtained the elasticity information from the coralline epithallial cells. In this sense, the section of the force curve where the sample and the tip are in contact is fitted using the contact mechanics models. In this case, we used the Derjaguin-Muller-Toporov (DMT) model, which is more realistic because it takes into consideration the adhesion effects (Derjaguin et al., 1975). High resolution maps of topography and elasticity (DMT Modulus) were acquired with Quantitative Nanomechanical Mapping (QNM, Bruker, California, USA). Tip calibration was performed using the absolute method, as recommended by the manufacturer, prior to each experiment. Peak Force Tapping was performed using silicon tips (ScanAsyst-Fluid, Bruker, California, USA, nominal spring constant of 0.7 N/m). The slides were fixed on a Petri dish, and 3 mL of seawater were added for fluid scanning. Images were acquired with 512×512 pixels of resolution at a low scan rate (0.5 Hz), and image processing (line-wise flatten only) was performed using NanoScope Analysis 1.6 (Bruker, Santa Barbara, CA). Statistical analyzes were performed to test differences of elasticity. Force curves (n = 30) from the cell wall area and central area of the 4 and 16 cell stage were acquired. Kolmogorov-Smirnov Test showed that the values had a normal distribution, thus Analysis of variance (Anova) was performed in Statistica software (Statsoft, Hamburg, Germany).
By monitoring spore’s development using polarized light microscopy (PLM), it was possible to follow changes in the structure of young germinating spores (n > 20 settled spores in each microscopic slide) in the different growth stages. Images showed that spores with 2 cells presented numerous possible starch grains inside the cells (Figure 1A), which became less abundant with the growth and subsequent cell divisions. Besides, individuals with 2 cells, presented a transparent and thin cell wall (Figure 1A), while in samples with 4 cells a different cell wall composition seemed evident, with an opaque material present in all the cell wall structure (Figure 1B). PLM observations confirmed the cell wall difference that seems to take place after the second cell division (4 cells). Samples with 2 cells presented a lower birefringence in comparison with 4 cells spores and the spores that are in other development stages. It is interesting to notice that the polarizing material seen in 4 cells samples and in the subsequent stages is concentrated in the cell walls of the innermost part of the growing thallus while the cell walls facing the external medium do not present this polarizing material (Figures 1G–J). With the insertion of the phase plate (pinkish images), the birefringence presented by the walls of 4 cells spores (Figure 1L) had a specific pattern of orientation of the blue and yellow colors, which were inverted relatively to the walls of older germinated spores, with “yellow” cell walls parallel and “blue” cell walls perpendicular to the phase plate direction. As it will be shown in the next section, calcium ions were not found in the innermost cell walls of 2 and 4 cell spores, revealing that macromolecules (such as cellulose fibrils) or shape anisotropy of the walls were responsible for the weak birefringence observed. On the other hand, the cell walls of 8 cells spores were clearly birefringent and that their low ray axis direction were parallel to the low ray axis of the phase plate (double arrow in the Figure 1K) and thus perpendicular to the cell wall contours. This interpretation comes from the observation of blue and yellow colors, corresponding to directions perpendicular and parallel to the cell wall contours relative to the phase plate low ray axis, respectively. This would be in favor of the presence of calcite crystallites inside each cell wall with the c axis (which is the optic axis in calcite) oriented parallel to their contours with an increase of intensity mainly after 8 and 16 cells stages (Figures 1N, O). The c axis of a birefringent crystal (e.g., calcite crystal) is a direction under which the incident light rays, suffer no double refraction when traveling inside the crystal. In this propagation direction, the light passes through the crystal as if it were a non-birefringent material. In other propagation directions, the light is split into two polarized rays that migrate with different velocities associated with specific refractive indices (for details about this subject see Wood, 1977).
The first recovered slides presented settled L. corallinae spores (> 10 spores/slide) with 2 to 4 cells (Figures 2A, B) with an oval to spherical shape and with 43.81 ± 1.52 µm as mean diameter (n = 10). The cell walls were thin in the 2 cells individuals and became more evident in 4 cells germinating spores. The germinating spores presented a network-like material over its structure, probably extracellular matrix used for spore fixation or bacterial EPS. The second cell division occurred in a cruciate morphology, which is typical of the Amphiroa type of growth described by Chihara, 1974, in which Lithophyllum is also a genus that presents this feature in spore growth. The latter slides (After 12 hours of culture) presented germinating spores with 16 to 128 cells with radial or filamentous-like type of growth. The cells presented a different shape from the youngest germinating cell with a rectangular shape. The most evident difference is the increased thickness of the cell walls of the innermost part of the developing thallus (Figures 2C, D). The removal of the superficial cell tissue revealed the resemblance of these call walls with cell walls seen in mature coralline algae. Elemental analysis of the cell walls from L. corallinae germinating spores revealed changes in composition of these structures during the development stages. While in 2 and 4 cells (Figures 2A, B) it is not possible to observe calcium or magnesium signal inside the structure of the cell wall, germinating spores with approx. 16 cells (Figures 2C, D) presented a higher calcium signal in the cell walls that are in the innermost part of the new thallus corroborating the change of cell wall composition observed in the Optical and Polarized Microscopy analyzes. Mg signal also starts to appear in the cell walls of the 16 cells germinating spore.
Figure 2 Scanning Electron Microscopy images and EDS maps (calcium and magnesium) of L. corallinae germinating spores that settled over the microscope slides. 2 cells spore presenting a thin cell wall dividing the cells and a framework material that seems to help with the settlement process (A, scale bar = 10 µm). 4 cells germinating spore with cruciate cell division and with the same framework material covering all the spore and part of the microscope slides (B, scale bar = 10 µm). 12 to 16 cells germinating spore with the superficial tissue removed (C, scale bar = 20 µm). 24 to 32 cells germinating spore with a filamentous growth, showing the small cells from divisions that occurs in the distal regions (D, scale bar = 20 µm).
High resolution transmission electron microscopy of uppermost cells from the epithallus of a 16 cells germinating spore (n = 10) showed the presence of early needle calcite crystals growing in the outermost part of the cell walls (middle lamella) and a thinner cell wall infill with tangential crystals (Figure 3A). Cells that are immediately under the epithallial cells present a thicker cell wall, with the presence of calcite crystals that grew perpendicular to the cell wall (yellow asterisk). Tangential crystals present different sizes and orientations with needle-like and hexagonal shapes (Figure 3B). The calcite crystals from different parts of the cell wall presented nanopores in their structure with a diameter of 4.982 ± 0.77 nm (N = 100) (Figure 3C). Selected area electron diffraction (yellow circle in Figure 3A) indicated that the crystals from the middle lamella are indeed high magnesium calcite, with the (104) peak at 2.964 nm (d-spacing) which represents a magnesium substitution of approx. 25% mol% MgCO3 according to Zhang et al., 2010 (Figure 3D).
Figure 3 Transmission electron microscopy images of 16 cells L. coralline germinating spore showing epithallial cells with thinner cell walls with needle-like and elongated calcite tangential crystals. Perithallial cells with thicker cell walls and perpendicular calcite crystals (A, asterisk). Detail of the tangential crystals with different sizes and orientations (B). Needle-like and elongated calcite nanocrystals with presence of nanopores in the crystalline structure (C). Selected area electron diffraction (SAED) of needle-like calcite nanocrystals evidencing the random orientation and the identification of high magnesium calcite through SAED pattern indexing (D).
Confirming the results of the change in composition of cell walls from 4 cells to later development stages obtained with the other techniques used in this study, Atomic Force Microscopy also detected structural and mechanical differences. Topographical images of 4 cells germinating spores (Figure 4A) showed that the central part of the cells is higher than the cell walls surrounding the cell lumen. The cell walls are thin and almost imperceptible (Figure 4A). In contrast, cell walls from 16 cells samples are more evident, four times thicker than the 4 cells (1.56 ± 0.3 μm x 0.39 ± 0.2 μm). At this stage the central part of the cell is topographically lower in comparison with the surrounding cell wall, as a flattened or compressed shaped epithallial cells (Figure 4B). DMT modulus images (elasticity) showed that 4 cells germinating spores presented a uniform elasticity along the analyzed cells, with regions ranging between 1.5 MPa to 3.7 MPa (Mean = 2.6 ± 0.6 MPa; Figure 4C). The cell wall region and the central part of the 4 cell spores presented similar elasticity (Anova, p = 0.82725; Table 2) Meanwhile, DMT modulus images of germinating spores with 8 to 16 cells showed the decreased elasticity in the cell walls of cells that are in the innermost region of the spore structure (Anova, p = 0.00001; Table 2), with some regions of the cell walls ranging up to 35.8 MPa, which is almost ten times less elastic than the 4 cell wall tissue and the central part of the cell spore with 16 cells (Figure 4D). Although the increase of cell walls inflexibility, the tissue of the central part of the cells in this development stage remained with a similar elasticity (Mean = 3.1 ± 1.2 MPa) of the 4 cells tissues (Anova, p = 0.94775 and p = 0.98949; Table 2).
Figure 4 Topographical and elasticity images of 4 cells (A, B) and 16 cells (C, D) showing the topographical and hardness (DMT Modulus) differences of the cell surfaces between these two developmental stages.
Table 2 Summary of the data from the ANOVA analyzes and Post Hoc Tukey HSD test with DMT Modulus (elasticity).
This study takes over the ontogeny and development of coralline algae ultrastructure and biomineralization process that started over 40 years ago providing the first insights of cell division patterns and the appearance of the first structures inside the cell walls that were related to the early deposition of CaCO3 crystals (Chihara, 1974; Borowitzka and Vesk, 1978; Vesk and Borowitzka, 1984).
Some of the key questions raised up by these studies were how the cell wall develops, what is the composition of the early cell walls and if deposition of the organic matrix precedes the CaCO3 deposition or vice versa.
In this sense, our study with L. corallinae provided new information that can be incorporated with the previous studies and draw a preliminary picture of the early stages of coralline algae germinating spore development and the changes in the cell wall that culminates in the heavy deposition of CaCO3.
The germinating spores of L. corallinae obtained in the slides presented a diameter that is in accordance with the Lithophyllum group mentioned by Chihara, 1974 that ranged between 20 and 45 µm. The cell division was also related to the same pattern seen in this study, with the cruciate division of the 4 cell spore and the subsequent small lateral division and a partially radial growth. The growth patterns exhibited by the germinating spores after 8 cells can be related to several conditions, including water motion, space competition, depth and temperature (McCoy and Kamenos, 2015).
Cell wall formation followed by Polarized Light Microscopy revealed polarizing material in the cell wall from the germinating spores since the first cell division and the observations with the phase plate showed that the birefringence ray axes change from 4 cell spores to higher than 4 cells spores. This fact shows that in early growth stages, the materials that polarizes light are cellulose fibrils (Srivastava, 2002) or the shape anisotropy of the apposed cell walls, while for stages with higher number of cells, birefringence is caused by calcite crystallites (Cheng et al., 1998). Calcite is known as a negative birefringent crystal, which means that in this type of crystals, the refractive index experienced by the extraordinary ray (electric field vibration plane that contains the c axis) is less than that for the ordinary ray (Inoué, 2008).
Taking this information into account, the EDS analysis performed in this work was determinant to point that the material inside the cell wall of the two and four cell individuals is cellulose, while in older germinating spores, in which cell division promotes a confined space between the cells, the detection of calcium and magnesium reveals the biomineralization of high magnesium calcite first on the middle lamella and later within coralline algae cell wall.
High resolution transmission electron microscopy images confirmed the development sequence of coralline algae cell wall proposed by Cabioch and Giraud, 1986, with the production of high magnesium calcite elongated crystallites in the middle lamella, tangential to the cell walls in the outermost part of the cell wall. Finally, perpendicular crystals are formed in subepithallial cells, where the secondary cell wall is well developed. The high magnesium calcite biomineralization of the juvenile L. corallinae is in agreement with the calcification model proposed by Nash et al., 2019, with similarities in the cell wall structure of Amphiroa anceps analyzed by these authors, which is also from the Lithophylloideae subfamily (Aguirre et al., 2010).
Regarding the appearance of the first calcite crystals, the explanation could be related to the size of the germinating spore. In fact, previous evidences point out for a “trans calcification” mechanism proposed by McConnaughey and Whelan (1997) for calcifying macroalgae. In their proposition, calcification is enzymatically controlled, based on the transformation of HCO3-1 in CO2 for photosynthesis by a carbonic anhydrase, which in turn produces CO3-2 and the ion pumps provide the efflux of Ca2+ inside the cell wall for ion supersaturation. One of the most accepted idea is that calcification rate is directly proportional to the photosynthetic rate (Borowitzka and Larkum, 1976; Jensen et al., 1985) and for Halimeda tuna Borowitzka and Larkum have shown that photosynthetic rate must exceed a threshold value of 100 to 200 nmol CO2 fixed g-1 dry weight min.-1 before calcification is stimulated (The carbon dioxide utilization theory). Besides providing the optimal conditions, coralline algae present an organic matrix inside cell walls composed by polysaccharides that presents a high affinity of divalent ions (Bilan and Usov, 2001, Carvalho et al., 2017). This could be the reason for the biomineralization to take place after a minimum growth of the germinating spore, which in fact will present greater photosynthetic surface and more organic matrix production, which can provide the energy and a template necessary for the process.
Another feature that we have noticed in our results is the low concentration of calcium and magnesium in the cell walls that faced the external medium. According to previous studies there are three occasions in which coralline algae cell walls are not calcified, 1) non-calcified segments (intergenicula) of articulated coralline algae (Bilan and Usov, 2001); 2) Regions where some tissue lesion occurred, e.g. fish bites, bacterial infection (Pueschel et al., 2005); 3) Epithallial cells or reproductive structures (Borowitzka and Vesk, 1978). In this sense we can establish that coralline algae exert control over the areas that will be calcified in the thallus. The concentration of ions observed after the 4 cell stage in the EDS analyzes lead us to hypothesize that this process initiates, with the help of photosynthetic energy, in the moment that the germinating spore presents cell walls that are totally isolated of the external medium, corroborating with the intercellular biomineralization hypothesis for coralline algae presented by Mann (2001) and the bioinduced mineralization hypothesis raised by Nash et al., 2019.
The formation of high magnesium calcite crystals after the isolation of the cell walls of the innermost cells in 8 celled germinating spores is in agreement with previous studies which reported the production of crystals in confined spaces in other marine organisms, such as sea urchins (Robach et al., 2005). It is well known that this metastable calcite polymorph can only be precipitated synthetically, without the presence of additives such as proteins and polysaccharides, mixed solvents, organic monolayers or via solid transformation process, at high temperatures and high pressure (Long et al., 2014), so in the case of marine organisms, the isolation from the external media and the presence of specialized macromolecules is essential to produce these highly non-equilibrium crystals.
High resolution images from the tangential crystals produced by the epithallial cells from L. corallinae germinating spores also revealed an interesting feature of the high magnesium calcites, which is the presence of nanopores inside the crystal structure. One of the explanations of this phenomenon could be the presence of macromolecules (proteins and polysaccharides) inside its crystalline structure. During the mineralization process, some proteins and polysaccharides adhere to the growing crystal, then crystal growth rate can be fast enough to avert the macromolecule rejection, which eventually becomes overgrown. In this process, these substances can be occluded inside the crystal structure (Blake and Peacor, 1981; O'Neill, 1981; Berman et al., 1990).
Previous studies stated that one of the advantages of these macromolecules occlusion is the fact that they can enhance the toughness and cause the conchoidal fracture morphology of echinoid calcite skeletal elements, which corresponds to a change in the intrinsic brittleness of synthetic calcite (Berman et al., 1990; Berman et al., 1993).
Carvalho et al., 2017 showed the presence of fibers inside mature coralline algae calcite crystals, which was interpreted as organic matter inside the crystal structure. The combination of these organic fibers (evidenced by the possible occluded macromolecules) together with calcification process, found in this study, could indicate that coralline algae high magnesium calcite exhibits a structure that inhibits cracking of the calcite skeleton in high energy environments, such as coastal areas with strong waves or areas with strong currents.
Up to date, this is the first study that accessed nanomechanical properties of live coralline algae cells. Atomic Force microscopy analyzes revealed the hardening of the cell walls of germinating spores from 4 cells to 16 cells, which became ten times harder, with elasticity similar to other mineralizing tissues like silica stripes found in diatoms that presented 43.7 MPa (Pletikapic´ et al., 2012). The elasticity of the central part of the 16 cells germinating spores remained similar to the 4 cell spore, which suggests that this part of the coralline structure is not calcified and it is probably covered by a soft tissue, confirming the PLM, SEM and EDS results. The lack of calcification in the upper cortical cells is a feature long known for coralline algae (Borowitzka and Vesk, 1978) and this study revealed in which development stage this can be already seen. Mechanical properties (hardness and elastic modulus) from adult crusts were accessed by Nash (2016) with another technique (nanoindentation) and the analyses showed that there is a transition from soft Mg-calcite surface to fracture resistance in the perithallus, which presents dolomite. The modulus recorded for these crusts was in GPa order of magnitude, which differs from what we observed (MPa order of magnitude) because this study was performed in dead polished coralline algae skeleton, with no soft tissue cover. Moreover, nanoindentation uses much more force to indent on the sample surface, causing the deformation of the material, which is different from Atomic Force Microscopy, which uses an atomic scale sharp tip and much less force, only enough to press soft samples without damaging it aiming to provide elasticity measurements of the sample itself, not the substrate or anything else that is beneath the material.
The results herein presented, revealing the formation of first calcite crystals between 8 to 16 cells stages in L. corallinae, may support future studies availing the response of germinating spores and early biomineralization to changes in some environmental factors predicted in future atmospheric and seawater scenarios (IPCC, 2022), like seawater acidification and greenhouse effect. Some of the effects of these changes were already reported by Bradassi et al. (2013) with rising of spore mortality and abnormalities and the decrease of calcification rates. Although the study was performed with only one species (Phymatholithon lenormandii) and germinating spores developed for one week, perhaps these effects could be detected even earlier, in spores with few hours of development and with different intensities regarding different coralline algae species.
Based on the results obtained in this study, the mineralization process in the cell wall of germinating spores of Lithophyllum corallinae begins after 8 to 16 cell of settled germinating spores. This fact was evidenced by the change in the cell wall composition seen with the Polarized Light Microscopy, with the presence of Ca and Mg ions inside the cell walls of the innermost part of the individuals, the identification of high magnesium calcite in the cell wall and the increase of hardness presented by these cell walls. The explanation for the beginning of the calcification in the cell walls could be related with the increase of the spore size together with the increase of the photosynthetic tissue and cell wall organic matrix. The presence of occluded macromolecules in calcite crystals exhibits may be especially important to prevent cracking of the calcite skeleton in high energy environments, such as coastal areas with strong waves or areas with strong currents. All the analysis also confirmed the lack of calcification in the cell walls of the outermost part of the germinating spore, which allows the growth of the individual and indicates some level of control from coralline algae over its biomineralization process.
We thank CENABIO for the Scanning Electron Microscopy facilities; Laboratório de Física Biológica and Dr. Gustavo Miranda Rocha for Atomic Force Microscopy facilities and analyzes. Authors also thank FAPERJ, CNPq, CAPES and PETRORIO/ANP for financial support. M.F. and L.T.S. would like to thank CNPq and FAPERJ Brazilian agencies for research fellowships.
The original contributions presented in the study are included in the article/Supplementary Material. Further inquiries can be directed to the corresponding author.
RC and LS conceived and wrote the paper. MF and LS raised financial support. RB coordinated the field work and identified the coralline algae species. RC, LS and MW designed and executed the laboratory maintenance and sporulation of coralline algae. CW and MF contributed to TEM and electron diffraction data and analyses. RC, LS and MF revised the paper. All authors contributed to the article and approved the submitted version.
Conselho Nacional de Desenvolvimento Científico e Tecnológico (CNPq), Coordenadoria de Aperfeiçoamento de Pessoal deNível Superior (CAPES), Fundação Carlos Chagas Filho de Amparo à Pesquisa do Estado do Rio de Janeiro (FAPERJ), and ANP/Brasoil provided essential funding.
The authors declare that the research was conducted in the absence of any commercial or financial relationships that could be construed as a potential conflict of interest.
All claims expressed in this article are solely those of the authors and do not necessarily represent those of their affiliated organizations, or those of the publisher, the editors and the reviewers. Any product that may be evaluated in this article, or claim that may be made by its manufacturer, is not guaranteed or endorsed by the publisher.
The Supplementary Material for this article can be found online at: https://www.frontiersin.org/articles/10.3389/fmars.2022.900607/full#supplementary-material
Supplementary Figure 1 | Vegetative and reproductive characteristics of L. corallinae from Vermelha Beach, Rio de Janeiro. (A) General aspect of an adult crust adhered to the rocky shore at 4 m depth. (B) Fragment of recovered rock with only L. corallinae encrusting specimens used in this study for spore production. It is noticeable the margin from different individuals (white arrow). (C) Transversal slice of the thallus basal region, showing the dimer thallus construction. (D) Transversal slice of the thallus surface showing rounded epithallial cells and adjacent filaments joined by secondary cell connections (arrows). (E) Superficial view of the uniporate tetrasporangial conceptacles. (F) Longitudinal slice of a uniporate conceptacle. Note the presence of central columella (letter C) and papillated cells the delimits the conceptacle porous canal.
Aguirre J., Perfectti F., Braga J. C. (2010). Integrating Phylogeny, Molecular Clocks, and the Fossil Record in the Evolution of Coralline Algae (Corallinales and Sporolithales, Rhodophyta). Paleobiology 36 (4), 519–533. doi: 10.1666/09041.1
Amado-Filho G. M., Moura R. L., Bastos A. C., Salgado L. T., Sumida P. Y., Guth A. Z., et al. (2012). Rhodolith Beds Are Major CaCO3 Bio-Factories in the Tropical South West Atlantic. PloS One 7 (4), e35171. doi: 10.1371/journal.pone.003517
Andersson A. J., Mackenzie F. T., Bates N. R. (2008). Life on the Margin: Implications of Ocean Acidification on Mg-Calcite, High Latitude and Cold-Water Marine Calcifiers. Mar. Ecol. Prog. Ser. 373, 265–273. doi: 10.3354/meps07639
Bahia R. G. (2014). Algas Coralináceas Formadoras De Rodolitos Da Plataforma Continental Tropical E Ilhas Oceânicas do Brasil: Levantamento Florístico E Taxonomia (Rio de Janeiro: Escola Nacional de Botânica Tropical), 231.
Berman A., Addadi L., Kvick Å., Leiserowitz L., Nelson M., Weiner S. (1990). Intercalation of Sea Urchin Proteins in Calcite: Study of a Crystalline Composite Material. Science 250 (4981), 664–667. doi: 10.1126/science.250.4981.664
Berman A., Hanson J., Leiserowitz L., Koetzle T. F., Weiner S., Addadi L. (1993). Crystal-Protein Interactions: Controlled Anisotropic Changes in Crystal Microtexture. J. Phys. Chem. 97 (19), 5162–5170. doi: 10.1021/j100121a052
Bilan M. I., Usov A. I. (2001). Polysaccharides of Calcareous Algae and Their Effect on Calcification Process. Russian J. Bioorganic Chem. 27, 2–16. doi: 10.1023/A:1009584516443
Blake D. F., Peacor D. R. (1981). Biomineralization on Crinoid Echinoderms. Characterization of Crinoid Skeletal Elements Using TEM and STEM Microanalysis. Scanning Electron Microscopy Pt 3), 321–328.
Borowitzka M. A., Larkum A. W. D. (1976). Calcification in the Green Alga Halimeda III. The Sources of Inorganic Carbon for Photosynthesis and Calcification and a Model of the Mechanism of Calcification. J. Exp. Bot. 27, 879–893. doi: 10.1093/jxb/27.5.879
Borowitzka M. A., Vesk M. (1978). Ultrastructure of the Corallinaceae.I. The Vegetative Ceils of Corallina Officinalis and C. Cuvierii. Mar. Biol. 46, 295–304. doi: 10.1007/BF00391400
Bradassi F., Cumani F., Bressan G., Dupont S. (2013). Early Reproductive Stages in the Crustose Coralline Alga Phymatolithon Lenormandii Are Strongly Affected by Mild Ocean Acidification. Mar. Biol. 160, 2261–2269. doi: 10.1007/s00227-013-2260-2
Cabioch J., Giraud G., Leadbeater B. S. C., Reading R. (1986). “Structural Aspects of Biomineralization in the Coralline Algae (Calcified Rhodophyceae),” in Biomineralization in Lower Plants and Animals (Oxford: Oxford Universirty Press), 141–156.
de Carvalho R.T., et al (2017). Biomineralization of Calcium Carbonate in the Cell Wall of Lithothamnion Crispatum (Hapalidiales, Rhodophyta): Correlation Between the Organic Matrix and the Mineral Phase. Journal of phycology 53 (3), 642–51
Cheng L. L. Y., Howie R. A., Zussman J. (1998). “Rock Forming Minerals – Non-Silicates,” in The Geological Society, 2nd ed., vol. Volume 58. London (UK): The geological Society
Chihara M. (1974). The Significance of Reproductive and Spore Germination Characteristics to the Systematics of the Corallinaceae: Nonarticulated Coralline Algae. J. Phycology 10, 266–274. doi: 10.1111/j.1529-8817.1974.tb02712.x
Derjaguin B. V., Muller V. M., Toporov Y. P. (1975). Effect of Contact Deformations on the Adhesion Particles. J. Colloid Interface 53, 314–326. doi: 10.1016/0021-9797(75)90018-1
Fletcher R. L., Callow M. E. (1992). The Settlement, Attachment and Establishment of Marine Algal Spores. J. Phycology 27, 303–329. doi: 10.1080/00071619200650281
Foster M. S. (2001). Rhodoliths: Between Rocks and Soft Places. J. Phycology 37, 659–667. doi: 10.1046/j.1529-8817.2001.00195.x
Foster M., Amado-Filho G. M., Kamenos N. A., Riosmena-Rodríguez R., Steller D.S.. (2013). “Rhodoliths and Rhodoliths Beds,” in Contribution of SCUBA Diving to Research and Discovery in Marine Environments, 39th ed, vol. vol. 39. (Washington, DC: Smithsonian Institution Scholarly Press), 143–156.
Henriques M. C., Riosmena-Rodriguez R., Coutinho L. M., Figueiredo M. A. (2014). Lithophylloideae and Mastophoroideae (Corallinales, Rhodophyta) From the Brazilian Continental Shelf. Phytotaxa 190 (1), 112–129. doi: 10.11646/phytotaxa.190.1.9
Ichiki S., Hiroyuki M., Hajime Y., Yamamoto Y. (2000). Occurrence of Polynucleate Spores of the Crustose Coralline Alga Lithophyllum Yessoense (Corallinales, Rhdophyceae): Growth and Survival. Phycologia 39 (5), 408–415. doi: 10.2216/i0031-8884-39-5-408.1
Inoué S. (2008). Collected Works of Shinya Inou: Microscopes, Living Cells, and Dynamic Molecules (Singapore: World Scientific).
IPCC (2022). “Climate Change 2022,” in Impacts, Adaptation, and Vulnerability. Contribution of Working Group II to the Sixth Assessment Report of the Intergovernmental Panel on Climate Change. [H.-O. Pörtner, D.C. Roberts, M. Tignor, E.S. Cambridge (UK): Cambridge University Press
Jensen P. R., Gibson R. A., Littler M. M. (1985). Photosynthesis and Calcification in Four Deep-Water Halimeda Species (Chlorophyceae, Caulerpales). Deep-Sea Res. 32, 451–464. doi: 10.1016/0198-0149(85)90091-3
Long X., Ma L., Qi (2014). Biogenic and Synthetic High Magnesium Calcite – A Review. J. Struct. Biol. 185, 1–14. doi: 10.1016/j.jsb.2013.11.004
Maneveldt G. W., Van Der Merwe E. (2012). Heydrichia Cerasina Sp. Nov. (Sporolithales, Corallinophycidae, Rhodophyta) From the Southernmost Tip of Africa. Phycologia 51, 11–21. doi: 10.2216/11-05.1
Mann S. (2001). Biomineralization, Principles and Concepts in Bioinorganic Materials Chemistry (Oxford: Oxford University Press).
McConnaughey T. A., Whelan J. F. (1997). Calcification Generates Protons for Nutrient and Bicarbonate Uptake. Earth Sciences Reviews 42:95–117.
McCoy S. J., Kamenos N. A. (2015). Coralline Algae (Rhodophyta) in a Changing World: Integrating Ecological, Physiological and Geochemical Responses to Global Change. J. Phycology 51 (1), 6–24. doi: 10.1111/jpy.12262
Nash M. C. (2016). “Assessing Ocean Acidification Impacts on the Reef Building Properties of Crustose Coralline Algae,” in PhD Thesis (Canberra, Australia: The Australian National University), 436.
Nash M. C., Diaz-Pulido G., Harvey A. S., Adey W. (2019). Coralline Algal Calcification: A Morphological and Process-Based Understanding. PloS One 14 (9), e0221396. doi: 10.1371/journal.pone.0221396
Nelson W. A. (2009). Calcified Macroalgae – Critical to Coastal Ecosystems and Vulnerable to Change: A Review. Mar. Freshw. Res. 60, 787–801. doi: 10.1071/MF08335
O'Neill P. L. (1981). Polycrystalline Echinoderm Calcite and its Fracture Mechanics. Science 213 (4508), 646–648. doi: 10.1126/science.213.4508.646
Ordoñez A., Kennedy E. V., Diaz-Pulido G. (2017). Reduced Spore Germination Explains Sensitivity of Reef-Building Algae to Climate Stressors. PloS One 12 (2), e0189122. doi: 10.1371/journal.pone.0189122
Pittenger B., Erina N., Su C. (2012). “Quantitative Mechanical Property Mapping at the Nanoscale With PeakForce QNM,” in Bruker Corporation Application Note An128. California (USA): Bruker Surface Division. doi: 10.13140/RG.2.1.4463.8246
Pletikapic´ G., Berquand A., Radic´ T. M., Svetlic˘ic´ V. (2012). Quantitative Nanomechanical Mapping of Marine Diatom in Seawater Using Peak Force Tapping Atomic Force Microscopy. J. Phycology 48, 174–185. doi: 10.1111/j.1529-8817.2011.01093.x
Pueschel C. M., Judson B. L., Wegeberg S. (2005). Decalcification During Epithallial Cell Turnover in Jania Adhaerens (Corallinales, Rhodophyta). Phycologia 44, 156–162. doi: 10.2216/0031-8884(2005)44[156:DDECTI]2.0.CO;2
Robach J. S., Stock S. R., Veis A. (2005). Transmission Electron Microscopy Characterization of Macromolecular Domain Cavities and Microstructure of Single-Crystal Calcite Tooth Plates of the Sea Urchin Lytechinus Variegatus. J. Struct. Biol. 151 (1), 18–29. doi: 10.1016/j.jsb.2005.04.001
Smith A. M., Sutherland J. E., Kregting L., Farr T. J., Winter D. J. (2012). Phylomineralogy of the Coralline Red Algae: Correlation of Skeletal Mineralogy With Molecular Phylogeny. Phytochemistry 81, 97–108. doi: 10.1016/j.phytochem.2012.06.003
Srivastava L. M. (2002). Plant Growth and Development (Hormones and the environment. Oxford: Academic Press).
Steneck R. S. (1986). The Ecology of Coralline Algal Crusts: Convergent Patterns and Adaptive Strategies. Annu. Rev. Ecol. Systematics 17, 273–303. doi: 10.1146/annurev.es.17.110186.001421
Vesk M., Borowitzka M. A. (1984). Ultrastructure of Tetrasporogenesis in the Coralline Alga Halipton Cuvireri (Rhodophyta). J. Phycology 20, 501–515. doi: 10.1111/j.0022-3646.1984.00501.x
Wood E. A. (1977). Crystals and Light. An Introduction to Optic Crystallography (New York: Dover Publications Inc).
Keywords: Coralline algae, biomineralization, germinating spore, cell walls, calcium carbonate
Citation: de Carvalho RT, Wendt CHC, Willemes MJ, Bahia RG, Farina M and Salgado LT (2022) Ontogeny and Early Steps of the Calcification Process in Coralline Algae Lithophyllum corallinae (Florideophyceae, Rhodophyta). Front. Mar. Sci. 9:900607. doi: 10.3389/fmars.2022.900607
Received: 20 March 2022; Accepted: 30 May 2022;
Published: 24 June 2022.
Edited by:
Laurie Carol Hofmann, Alfred Wegener Institute Helmholtz Centre for Polar and Marine Research (AWI), GermanyReviewed by:
Merinda Nash, Australian Government, AustraliaCopyright © 2022 de Carvalho, Wendt, Willemes, Bahia, Farina and Salgado. This is an open-access article distributed under the terms of the Creative Commons Attribution License (CC BY). The use, distribution or reproduction in other forums is permitted, provided the original author(s) and the copyright owner(s) are credited and that the original publication in this journal is cited, in accordance with accepted academic practice. No use, distribution or reproduction is permitted which does not comply with these terms.
*Correspondence: Leonardo Tavares Salgado, bHNhbGdhZG9AamJyai5nb3YuYnI=
Disclaimer: All claims expressed in this article are solely those of the authors and do not necessarily represent those of their affiliated organizations, or those of the publisher, the editors and the reviewers. Any product that may be evaluated in this article or claim that may be made by its manufacturer is not guaranteed or endorsed by the publisher.
Research integrity at Frontiers
Learn more about the work of our research integrity team to safeguard the quality of each article we publish.