- 1Applied Plant Ecology, Institute of Plant Science and Microbiology, University of Hamburg, Hamburg, Germany
- 2Alfred Wegener Institute, Helmholtz Centre for Polar and Marine Research, Wadden Sea Research Station, List, Germany
- 3Department of Geography, School of Natural Sciences, Trinity College Dublin, Dublin, Ireland
- 4British Antarctic Survey, Cambridge, United Kingdom
- 5Ecosphere, University of Antwerp, Antwerp, Belgium
- 6Landscape Ecology and Environmental Systems Analysis, Institute of Geoecology, Technische Universität Braunschweig, Braunschweig, Germany
- 7Leibniz University Hannover, Ludwig Franzius Institute of Hydraulic, Estuarine and Coastal Engineering, Hannover, Germany
- 8Department of Estuarine and Delta Systems, Royal Netherlands Institute for Sea Research (NIOZ), Yerseke, Netherlands
- 9Faculty of Geosciences, Department of Physical Geography, Utrecht University, Utrecht, Netherlands
- 10BioConsult SH GmbH & Co. KG, Husum, Germany
- 11Forschungszentrum Küste (FZK), Hannover, Germany
- 12School of Environmental Sciences, University of East Anglia, Norwich, United Kingdom
- 13Center for Environment, Fisheries and Aquaculture Science (Cefas), Lowestoft, United Kingdom
The coastal protection function provided by the vegetation of tidal wetlands (e.g. salt marshes) will play an important role in defending coastlines against storm surges in the future and depend on how these systems respond to such forcing. Extreme wave events may induce vegetation failure and thereby risking loss of functionality in coastal protection. However, crucial knowledge on how hydrodynamic forces affect salt-marsh vegetation and whether plant properties might influence plant resistance is missing. In a true-to-scale flume experiment, we exposed two salt-marsh species to extreme hydrodynamic conditions and quantified wave-induced changes in plant frontal area, which was used to estimate plant damage. Moreover, half of the plants were artificially weakened to induce senescence, thus allowing us to examine potential seasonal effects on plant resistance. Morphological, biomechanical as well as biochemical plant properties were assessed to better explain potential differences in wave-induced plant damage. Our results indicate that the plants were more robust than expected, with pioneer species Spartina anglica showing a higher resistance than the high-marsh species Elymus athericus. Furthermore, wave-induced plant damage mostly occurred in the upper part of the vegetation canopy and thus higher canopies (i.e. Elymus athericus) were more vulnerable to damage. Besides a taller canopy, Elymus athericus had weaker stems than Spartina anglica, suggesting that biomechanical properties (flexural stiffness) also played a role in defining plant resistance. Under the highest wave conditions, we also found seasonal differences in the vulnerability to plant damage but only for Elymus athericus. Although we found higher concentrations of a strengthening compound (biogenic silica) in the plant material of the weakened plants, the flexibility of the plant material was not affected indicating that the treatment might not has been applied long enough. Nevertheless, this study yields important implications since we demonstrate a high robustness of the salt-marsh vegetation as well as species-specific and seasonal differences in the vulnerability to plant damage.
Introduction
Salt marshes are intertidal wetlands that are widely distributed along the coastlines from middle to high latitudes. They are increasingly recognized as contributing to the provision of valuable ecosystem services such as climate change mitigation through long-term carbon sequestration (Mcleod et al., 2011) or coastal protection through wave attenuation (Möller et al., 2014). To a great extent, the provision of these ecosystem services is determined by the vegetation of salt marshes which mainly consists of highly specialized grasses, herbs and shrubs that are adapted to high salinities and regular flooding (Adam, 2002). Despite their value, the persistence of salt marshes is threatened by anthropogenic pressures like their embankment, land-use change, eutrophication and climate change, leading to a salt marsh loss of 1-2% of the global area per year (Duarte et al., 2013). From all climate change factors, so far the impact of accelerated sea level rise on marshes has been most widely studied (Spencer et al., 2016; Schuerch et al., 2018), while other climate change effects such as the projected changes in storm intensities and thus hydrodynamic forcing has still received relatively less attention (Leonardi et al., 2018; Armitage et al., 2020).
Previous research examining the interaction of hydrodynamic forces and salt-marsh vegetation mainly focused on the wave attenuation capacity of salt marshes, i.e. the effect of vegetation on waves. These studies compared the wave attenuation capacity of different plant species as well as whole ecosystems, or aimed to find determinants of effective reduction in wave energy (Möller et al., 2003; Pinsky et al., 2013; Anderson and Smith, 2014). In a true-to-scale flume experiment, Möller et al. (2014) showed that the vegetation of NW European salt marshes is able to reduce significant wave height by between 15% and 60% over a 40 m distance under storm surge conditions. Additionally, the authors observed that the behavior of the tall grass Elymus athericus under the action of waves differed from that of the much shorter Puccinellia maritima, suggesting that plant-wave interactions and thereby wave attenuation capacity is dependent on species-specific traits (Rupprecht et al., 2017). These species traits include e.g. length, width, number or rigidity of plant stems and leaves, all influencing vegetation properties known to affect wave attenuation such as aboveground biomass (Bouma et al., 2010; Ysebaert et al., 2011), plant frontal area (Zhang and Nepf, 2021), stem density (Bouma et al., 2005; Anderson and Smith, 2014) and biomechanical properties (Riffe et al., 2011; Paul et al., 2016). However, the vegetation’s ability to reduce wave energy is also depending on hydrodynamic conditions like inundation depth and wave orbital velocity (Koch et al., 2009; Gedan et al., 2011; Garzon et al., 2019). For example, above certain values of orbital velocity, stem breakage and biomass loss may lead to a decrease in wave attenuation (Rupprecht et al., 2017; Vuik et al., 2018). Yet, studies investigating these limits, i.e. defining hydrodynamic conditions above which salt-marsh vegetation experiences severe physical damage, as well as factors that influence plants resistance, are rare.
The few studies that focused on physical damage induced by waves all suggest that the plant damage is species-specific (Coops and van der Velde, 1996; Heuner et al., 2015; Vuik et al., 2018; Schoutens et al., 2021). Furthermore, the observed differences between species have been attributed to differences in stem flexibility (Coops and van der Velde, 1996; Heuner et al., 2015; Vuik et al., 2018) and plant height (Vuik et al., 2018) and, in the case of seedling survival, stem diameter (Schoutens et al., 2021). A theoretical approach by Duan et al. (2002) showed that the position of stem breakage was dependent on the ratio of the top to base stem diameter. With increasing ratio, the position of steam breakage moved further up the stem, a finding that was in line with field observations by Groeneveld and French (1995). Moreover, a stem breakage model developed by Vuik et al. (2018) combined plant morphology (stem height and diameter), flexural strength of the stems and wave-induced bending stress to determine hydrodynamic forces above which stems break or fold. Vuik et al. (2018) found that shorter species were more flexible than taller ones and have a lower probability of stem breakage. However, a species-specific validation remains indispensable.
Apart from species-specific differences, seasonality in vegetation properties needs to be considered when evaluating the resistance of salt-marsh vegetation and their role for wave attenuation capacity (Schoutens et al., 2019; Zhang et al., 2022). In temperate and boreal regions, aboveground parts of many salt-marsh species die-off in autumn, but remain as dead standing biomass in winter until storms (and thus wave action) induce breakage of stems or leaves and eventually carry the litter away. While transforming from a vital to senescent or even dead state, the plant material undergoes major physiological changes that affect biomechanical properties like stem flexibility (Coops and van der Velde, 1996; Schulze et al., 2019; Zhu et al., 2020). The seasonal vegetation response was also confirmed by the stem breakage model of Vuik et al. (2018), which suggested that the salt marsh grass Spartina anglica is more vulnerable to stem breakage in November compared to December or April. During the storm surge season in temperate regions (from autumn until early spring), the coastal protection provided by salt marshes is most needed. However, the protection capacity in winter might be hampered due to changes in plant resistance and wave-induced damage. To our knowledge, it has never been experimentally tested whether seasonal changes in biomechanical or even morphological vegetation properties directly affect plant resistance to extreme wave conditions.
To improve our understanding on how plant’s vulnerability to wave-induced damage differs between species and seasonal conditions, we thus conducted a controlled flume experiment. We exposed two NW European salt marsh grass species (Spartina anglica, Elymus athericus) to progressively increased wave energies and measured plant response as well as biomechanical, biochemical and morphological plant properties. Since Spartina anglica usually grows in the pioneer zone of the marsh (low elevations, at the marsh edge), we expected a higher resistance to hydrodynamic forcing and thus lower vulnerability to wave-induced plant damage than for Elymus athericus, which typically grows at higher elevations that are less frequently flooded. Furthermore, prior to wave exposure, we induced senescence in half of the plants to create a weakened ‘autumn treatment’. We hypothesized that the plant’s resistance to hydrodynamic forcing is negatively affected by the autumn treatment due to changes in their biomechanical properties.
Material & methods
This flume experiment was conducted in the Large Wave Flume (Großer Wellenkanal (GWK) of the Forschungszentrum Küste (FZK), Hanover, Germany) in which salt-marsh plants, that have been previously collected in the field, were exposed to extreme hydrodynamic conditions. In addition to recordings of plant response to wave exposure (i.e. wave-induced plant damage), plant properties were assessed.
Plant material
For this study we used Spartina anglica (C. E. Hubb) and Elymus athericus (Link) Kerguélen, two clonal grass species that are widely distributed in NW European salt marshes. In March 2018, plants were collected in salt marshes of Paulinaschor (Spartina anglica, 51°20’56.2”N 3°43’37.4”E) and Zuidgors (Elymus athericus, 51°23’13.6”N 3°49’18.5”E) along the Western Scheldt estuary in the Netherlands. They were excavated in sods (20 cm x 20 cm) and transported to the Royal Netherlands Institute for Sea Research (NIOZ) in Yerseke, the Netherlands, where they were kept in the greenhouse until they were planted. Before planting the sods, boxes (120 cm x 80 cm x 40 cm, hereafter referred to as pallets) were lined with an impermeable foil and filled with sediment taken from a marsh of the Scheldt estuary (see Schoutens et al. (2021) for soil properties). Ten holes (2 mm diameter) on each side of the pallet were drilled to allow for drainage after inundation. In April, six sods per pallet were arranged together to create a dense canopy, leading to stem densities of 741 ± 123 stems/m2 for Spartina anglica and 1108 ± 176 stems/m2 for Elymus athericus (mean ± SD). The sods were planted at one end of the pallet to allow the waves to run up over an unvegetated strip before reaching the vegetation (see Figure 1 and Supplementary Material Figures 1A, B).
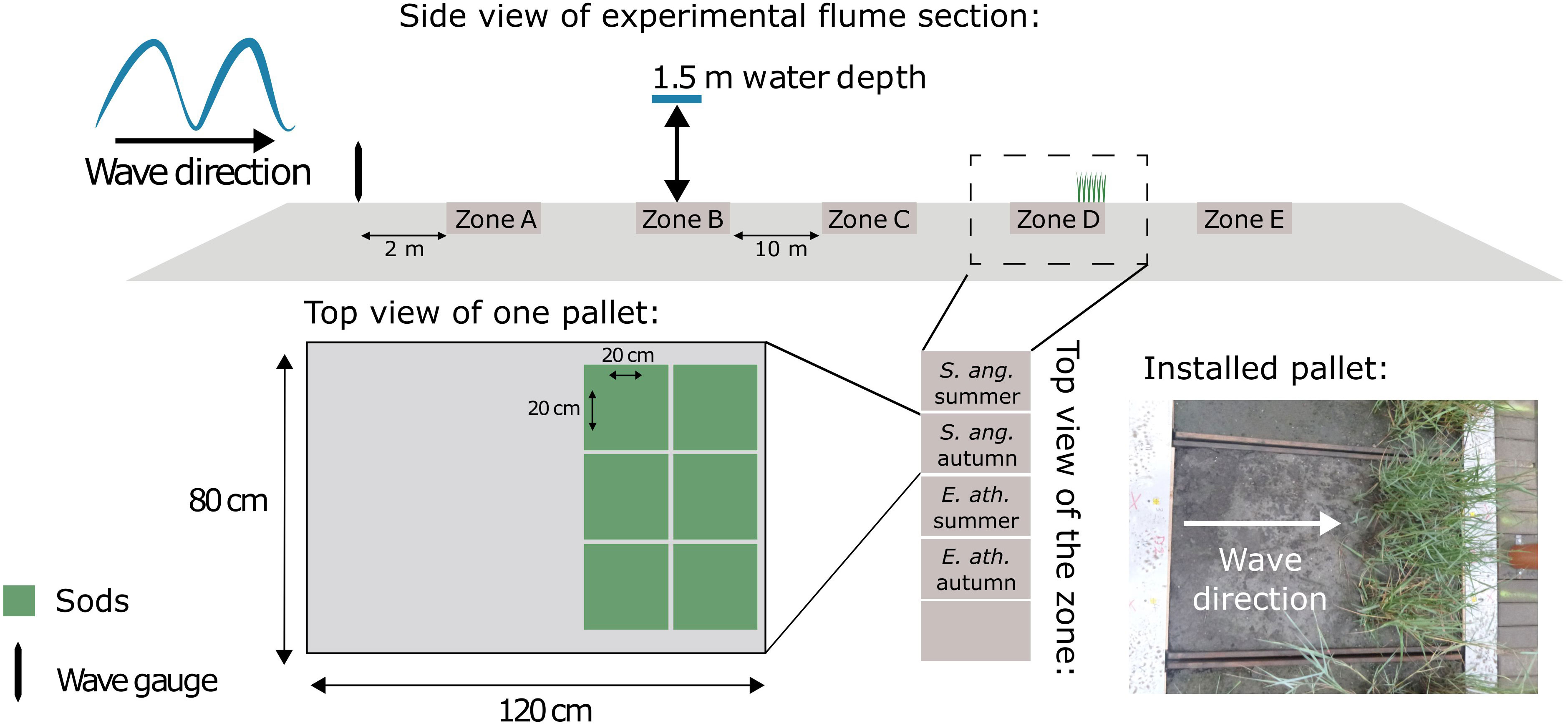
Figure 1 Schematic figure illustrating the experimental set up in the flume. The side view of the experimental platform shows the positions of the zones and wave gauges. The top views below visualize the arrangement of the pallets within zone D as well as the sods within a pallet (S. ang. = Spartina anglica, E. ath. = Elymus athericus). The figure was adapted from Schoutens et al. (2021).
To assess seasonal differences in plant response to hydrodynamic forces, half of the plants were subjected to an ‘autumn treatment’ while the others remained as ‘summer treatment’. The ‘autumn’ treatment was achieved by creating a drought, since the plant stress response (e.g. to drought) shares many similarities with the process of natural plant senescence at the end of the growing season (Gepstein and Glick, 2013; Sade et al., 2018). Furthermore, it has been reported that several plant species showed a significant accelerated maturity in response to water limitation (Desclaux and Roumet, 1996; Talukdar, 2013; Cseresnyés et al., 2020). To create a drought, pallets of the ‘autumn treatment’ were covered for six weeks prior to the flume experiment to exclude rainfall while the ‘summer treatment’ pallets were kept irrigated with freshwater (Schoutens et al., 2021). Hereafter, the term treatment is used to refer to the condition of the vegetation (summer/autumn).
Flume set up
The experiment in the Large Wave Flume (300 m x 5 m x 7 m) was carried out over three weeks in August 2018. For addressing different research questions within the RESIST project, five zones in total (10 m apart, Figure 1) were used to investigate the effect of increased hydrodynamic forces on different types of marsh vegetation as well as their soil surface. For instance, in Schoutens et al. (2021), we reported the results for one specific zone where we tested the resistance of seedlings to strong waves. Within each zone five pallets were placed next to each other over the flume width and between concrete blocks which had the same height as the pallets (see Figure 1 and Supplementary Material Figure 1C). The present study only focusses on one other zone, where we specifically tested the resistance of mature shoots of Spartina anglica and Elymus athericus, both in summer and autumn treatment, resulting in four pallets within this zone, while one pallet was left empty. Each Monday for the three weeks of the experiment, we replaced all pallets with a new set and carried out baseline measurements.
Hydrodynamic conditions
Hydrodynamic conditions were the same as reported in Schoutens et al. (2021). Each day from Tuesday to Friday, we created a sequence of waves that are hereafter referred to as ‘wave runs’. Wave runs consisted of 1000 randomly generated waves (JONSWAP spectrum, Hasselmann et al., 1973) that were monitored with wave gauges and from which we calculated significant wave height (Hs) and significant wave period (Ts). The wave gauge array was installed on the flume wall, 2 m in front of the first zone (zone A, Figure 1). The still water level was 1.5 m above the sediment surface for all wave runs. We increased bed orbital velocity by increasing wave height and/or wave period in subsequent wave runs over the course of days and weeks (Table 1), which also implies a cumulative wave exposure experienced by the vegetation and sediment per week. In the third week, pallets were moved to zone A and thus closer to the wave paddle (Figure 1) to further increase hydrodynamic forces. The wave conditions generated in this flume experiment were comparable to natural storm surge conditions in temperate regions (this study: Hs = 0.78 m, h = 1.5 m; field: Hs = 0.58-1.0 m, h = 1.39-3.85 m; Hs = significant wave height, h = inundation depth, Schoutens et al., 2021). After each wave run, we slowly drained the flume to record potential impacts of hydrodynamic forcing on the vegetation (details in next section).
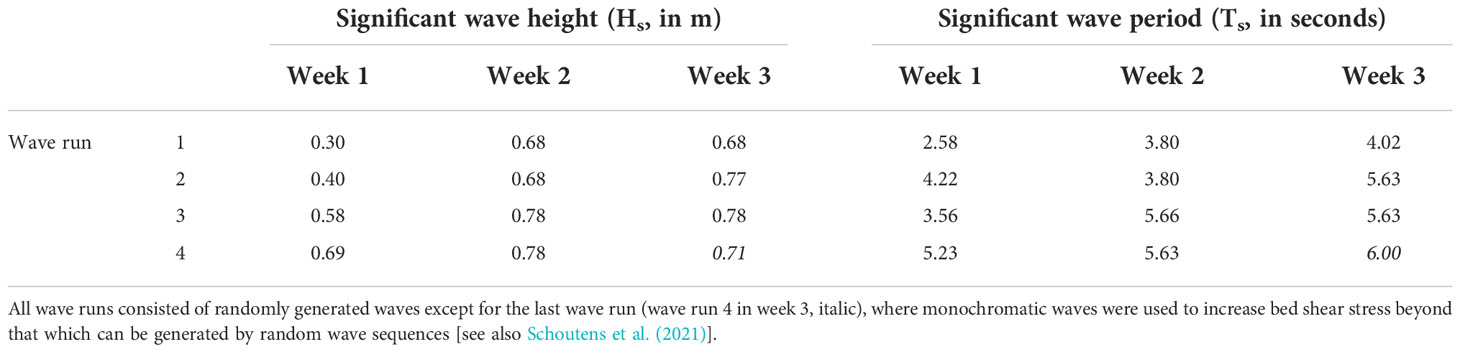
Table 1 Wave conditions measured in front of the first zone generated in this flume experiment and shown per week and wave run.
Vegetation response: Δ frontal area and mean vegetation height
To measure plant damage non-destructively, we used the photo-method described by Möller (2006). Every day after the wave run and draining of the flume, a red screen with a scale attached was placed behind the vegetation to increase contrast between vegetation and background (see Supplementary Material Figure 2A). Side-on photographs (Supplementary Material Figure 2B) were taken of the vegetation with a calibrated camera and from the back of the pallet (i.e. facing the back of the flume) always at the same position, height and distance to the screen. Photographs were cropped and corrected for distortion in MATLAB (The MathWorks Inc., 2019) before pixels were classified into either vegetation or background pixel using an unsupervised classification tool and followed by manual class allocation in ArcGIS (ESRI, 2020). Similarly to Rupprecht et al. (2015), these obtained binary images (Supplementary Material Figure 2C) were used to perform further program routines in MATLAB to generate information on vegetation structure, adapting the protocol developed by Möller (2006, MATLAB program available on request). Vegetation structure parameters included projected plant frontal area (projected area of vegetation pixel (mm2) normalized by the horizontal extent of the image (mm)) as well as the vegetation profile (highest vegetation pixel per pixel column). In this study, plant frontal area is given per pixel column and per vertical subsections (0-20 cm, 20-40 cm and >40 cm from soil surface). While projected plant frontal area per pixel column was further processed as described below, plant frontal area per vertical subsections was used to illustrate potential changes in the vertical distribution of the vegetation in each photograph.
Projected plant frontal area per pixel column was spatially referenced and converted into 1 cm wide columns (starting from the bottom left of the image). The data was averaged over 5 cm wide vertical subsections. Every second subsection, as well as the left and right outer edges (6 cm each), were removed to reduce potential impacts from adjacent subsections and pallets, respectively. To detect potential changes in plant frontal area due to wave exposure (indicating plant damage), we calculated the difference between projected plant frontal area prior to (Monday) and after wave exposure (Friday) per week (designated as Δ plant frontal area). It should be noted that changes in plant surface area might result from plant damage but also from reconfiguration of shoots and leaves. Estimations for vegetation height were obtained from vegetation profile data of the photographs taken prior to wave exposure. That data was averaged over the same 5 cm wide vertical subsections as for Δ plant frontal area and designated as mean vegetation height.
Biomechanical plant properties
Three-point bending tests were performed to measure stem flexibility as potential explanatory factor of plant damage under hydrodynamic forcing. Each week prior to and after wave exposure, five stems per species-treatment combination were cut at random at the soil surface. The bottom part of the stem was shortened to a length that was not exceeding a stem diameter-to-length ratio of 1:15 to avoid shear stress while testing (Niklas, 1992). Bending tests were performed with a universal test machine, including flexure fixture and a 5 kN load cell (Instron Corporation, Canton, MA, USA). Following Rupprecht et al. (2015) and Schulze et al. (2019), a force-displacement curve was created to determine the linear slope indicating elastic behavior that was then used to calculate flexural stiffness. Flexural stiffness or flexural rigidity describes the ability of the stem to resist bending, i.e. high values indicate high stem stiffness and thus low flexibility. It combines the information on the stem’s material property expressed by the Young’s bending modulus and the stem’s morphology expressed by the second moment of area which both contribute to overall flexural stiffness. The second moment of area is a term to include stem morphology (i.e. shape and diameter). Since the second moment of area can be derived from the stem’s dimensions, we were able to calculate Young’s bending modulus, which is only describing the flexibility of the material but without taking stem morphology into account. Equations for calculating flexural stiffness, second moment of area and Young’s bending modulus can be found in the Supplementary Material.
Biochemical plant properties
Biogenic silica, lignin and cellulose are known to affect the rigidity of plant tissue (Turner et al., 2001; Schoelynck et al., 2010; Schoelynck et al., 2012) and thus Young’s bending modulus. Potential differences in concentrations of these strengthening compounds could provide additional explanations for differences in stem flexibility and therefore plant resistance to hydrodynamic forcing. Plant material that was harvested for flexibility measurements was dried for 72 hours at 70°C and afterwards ground with a mixer mill (MM400, Retsch, Germany). For analyzing lignin and cellulose content, we used the Van Soest method (Van Soest 1963) whereas the biogenic silica content was determined by applying the DeMaster alkaline extraction method (DeMaster 1981).
Statistical analyses
To test whether Δ plant frontal area (difference between projected plant frontal area prior to and after wave exposure), flexibility parameters (Young’s bending modulus, flexural stiffness) and concentration of strengthening compounds (cellulose, lignin, biogenic silica) differed between species, treatments and weeks, factorial ANOVAs were applied. Accordingly, species, treatment, and week, as well as their interactions, were set as explanatory variables. After visually checking ANOVA assumptions following Zuur et al. (2010), flexibility parameters (Young’s bending modulus, flexural stiffness) were log transformed to meet normality assumptions. Plant material that was harvested for flexibility measurements and biochemical analyses was tested for differences between the two harvesting days (Monday and Friday). Since we did not find any differences, we merged the data per week. To detect significant differences between species, treatments, and weeks, post hoc tests (Tukey’s HSD, honest significant difference) were applied. A linear regression was performed to examine the relationship between Δ plant frontal area and mean vegetation height for the entire data set and separately for each species-treatment combination. To test whether biogenic silica concentration affect the flexibility of the plant material (Young’s bending modulus) another linear regression with these two variables was conducted. For this regression, Young’s bending modulus needed to be averaged over the five stems measured per day since there was not enough plant material of the single samples for the chemical analyses. All statistical analyses were performed using R version 4.1.1. (R Core Team, 2021).
Results
Plant damage under wave exposure
In this study, we used Δ plant frontal area to indicate wave-induced plant damage. Although reconfiguration of plant material might have influenced plant frontal area, we did observe plant damage in the form of torn-off material in the flume after draining (see Supplementary Material Figures 3, 4) which consisted of plant parts (e.g. leaves or inflorescences) as well as whole plants which broke off at soil surface level. Moreover, the quantity of the torn-off plant material reflected the overall trends we found for Δ plant frontal area. However, allocation of torn-off plant material to single pallets or zones was not possible. Δ plant frontal area differed significantly between species, treatments, and weeks (Table 2). Additionally, the three-way-interaction of these factors had a significant effect on Δ plant frontal area as well. In general, Elymus athericus showed a stronger reduction in frontal area compared to Spartina anglica (Elymus: -985.31 ± 950.66 mm2/mm, Spartina: -6.44 ± 499.91 mm2/mm, Figure 2, mean ± SD), implying that Elymus athericus lost more biomass due to wave exposure than Spartina anglica. Summer and autumn treatments of Spartina anglica showed similar response in Δ plant frontal area and decreased from week to week. This decrease was also found for Elymus athericus in autumn condition, but in comparison with Spartina anglica it was much more pronounced in Elymus athericus. In the third week, the difference in Δ plant frontal area between the summer and autumn treatment of Elymus athericus was highly significant (Figure 2) while differences between summer and autumn treatment were not significant for all other weeks for Elymus athericus and were never significant for Spartina anglica.
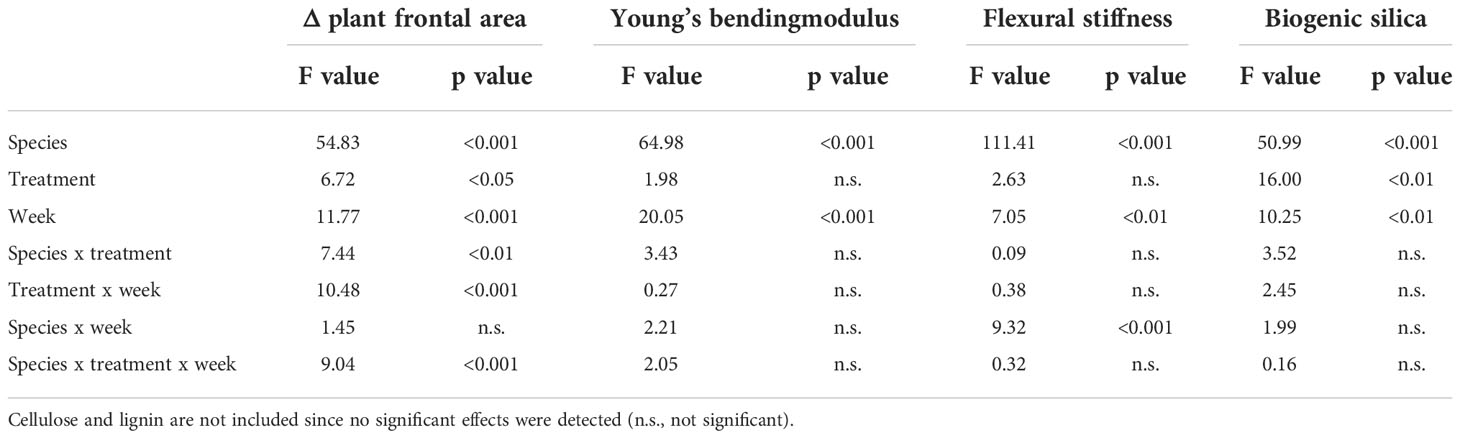
Table 2 Summary statistics of factorial ANOVAs for testing the effect of species, treatment and week (and their interaction) on Δ plant frontal area, Young’s bending modulus (MPa), flexural stiffness (Nm2) and biogenic silica concentration (mg/g dry weight).
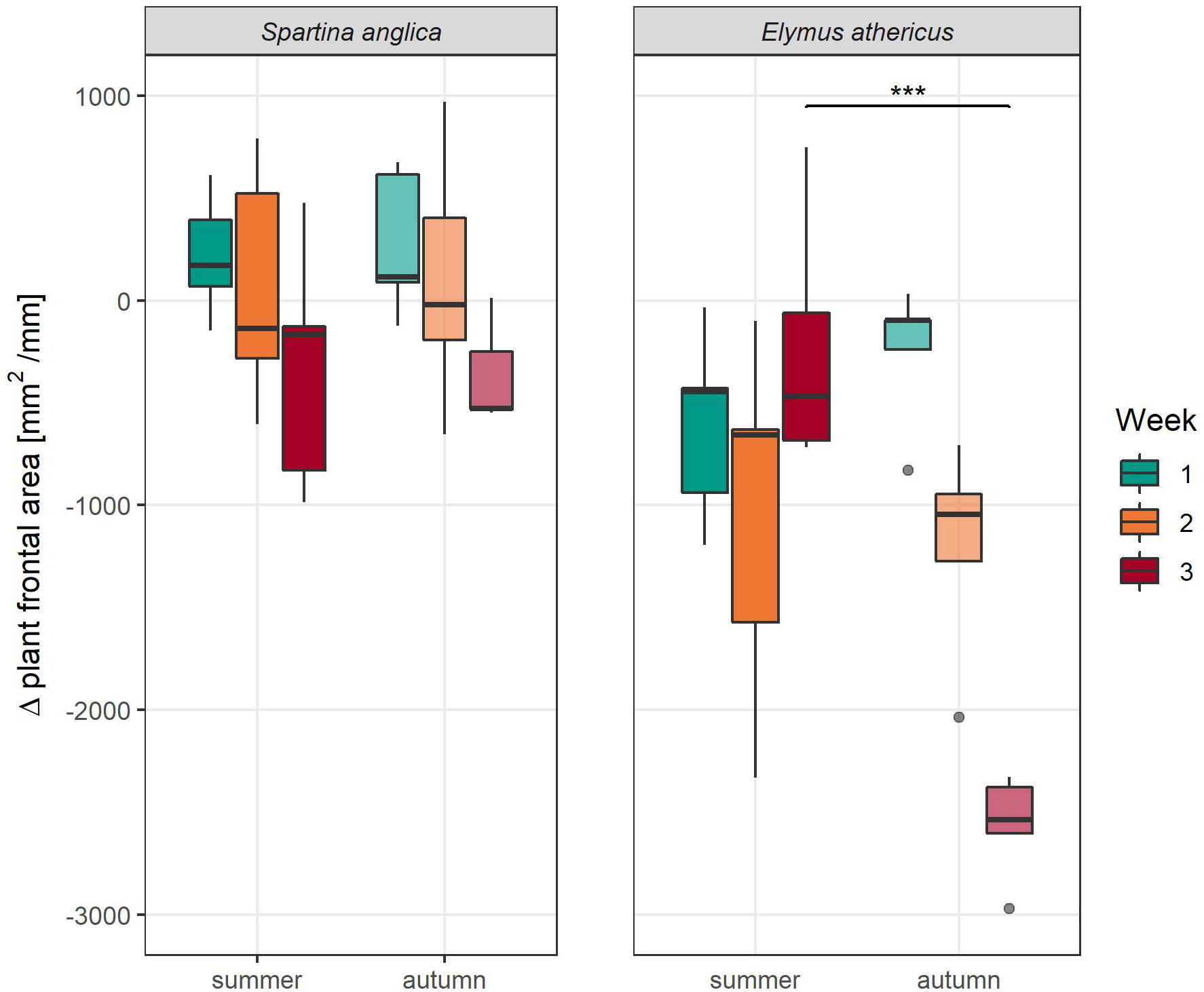
Figure 2 Δ plant frontal area (difference between prior to and after wave exposure) per week of Spartina anglica and Elymus athericus in summer and autumn condition. Asterisks show significant differences between the summer and autumn treatment within the same species and week based on Tukey’s HSD post hoc test (***p < 0.001).
Considering the spatial distribution of the vegetation in each photograph (Figure 3), the area from the bottom of the photograph to 20 cm height was completely saturated with vegetation for both species. For Elymus athericus, the area from 20 to 40 cm was likewise saturated, while wave-induced changes in frontal area became visible in this section on photographs of Spartina anglica. However, most changes in frontal area were detected in the upper section (>40 cm) for all species-treatment combinations. Here, frontal area tended to decrease over the course of days and weeks, which was most pronounced in the autumn treatment of Elymus athericus, indicating that plant damage was restricted to biomass removal from the top of the vegetation.
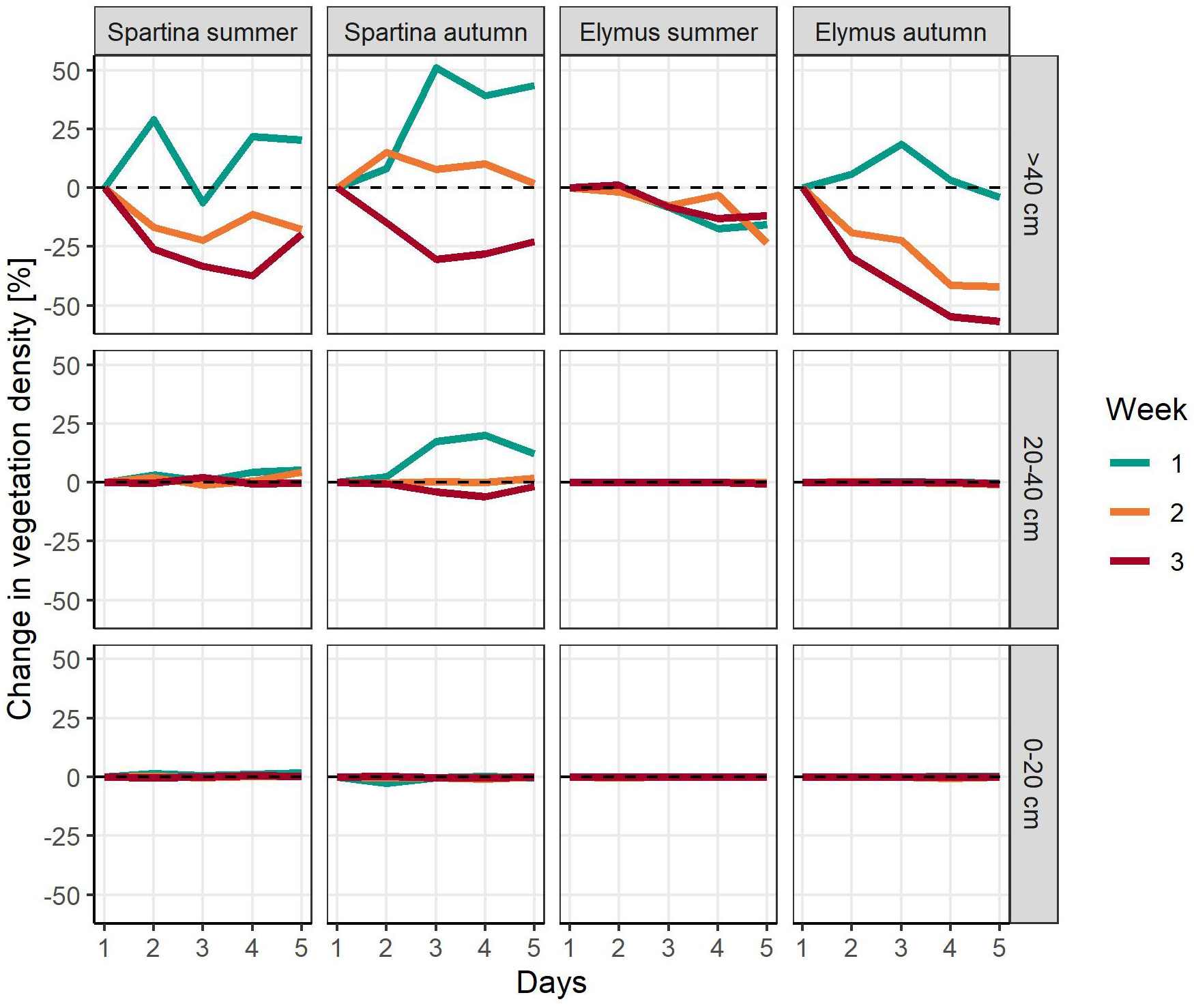
Figure 3 Change in projected plant frontal area in percent (relative to the condition prior to wave exposure on day 1) of three different vertical subsections shown per day, week and species-treatment combination. Dashed lines indicate a plant frontal area equivalent to the initial state on day 1 of the experimental week (i.e. 100%).
Morphological properties and plant damage
The linear regression between mean vegetation height (in mm) and Δ plant frontal area showed a negative relationship (R2 = 0.36, p <0.001, Table 3) indicating an increase in plant damage (as Δ plant frontal area decreased) with increasing vegetation height (Figure 4). Additionally, mean vegetation height clearly differed between both species, i.e. Elymus athericus being taller than Spartina anglica (Figure 4). For both species, autumn treatments were slightly taller than summer treatments (Spartina anglica: 624.1 ± 98.5 mm (summer) and 658.9 ± 145.6 mm (autumn), Elymus athericus: 832.7 ± 121.3 mm (summer) and 929.9 ± 92.4 mm (autumn)). However, no significant linear relationships between mean vegetation height and Δ plant frontal area were found for single species-treatment combinations except for the summer treatment of Elymus athericus (Table 3).

Table 3 Results of linear regression analyses testing the relationship between mean vegetation height and Δ plant frontal area for the different species-treatment combinations as well as the entire data set (n.s. = not significant).
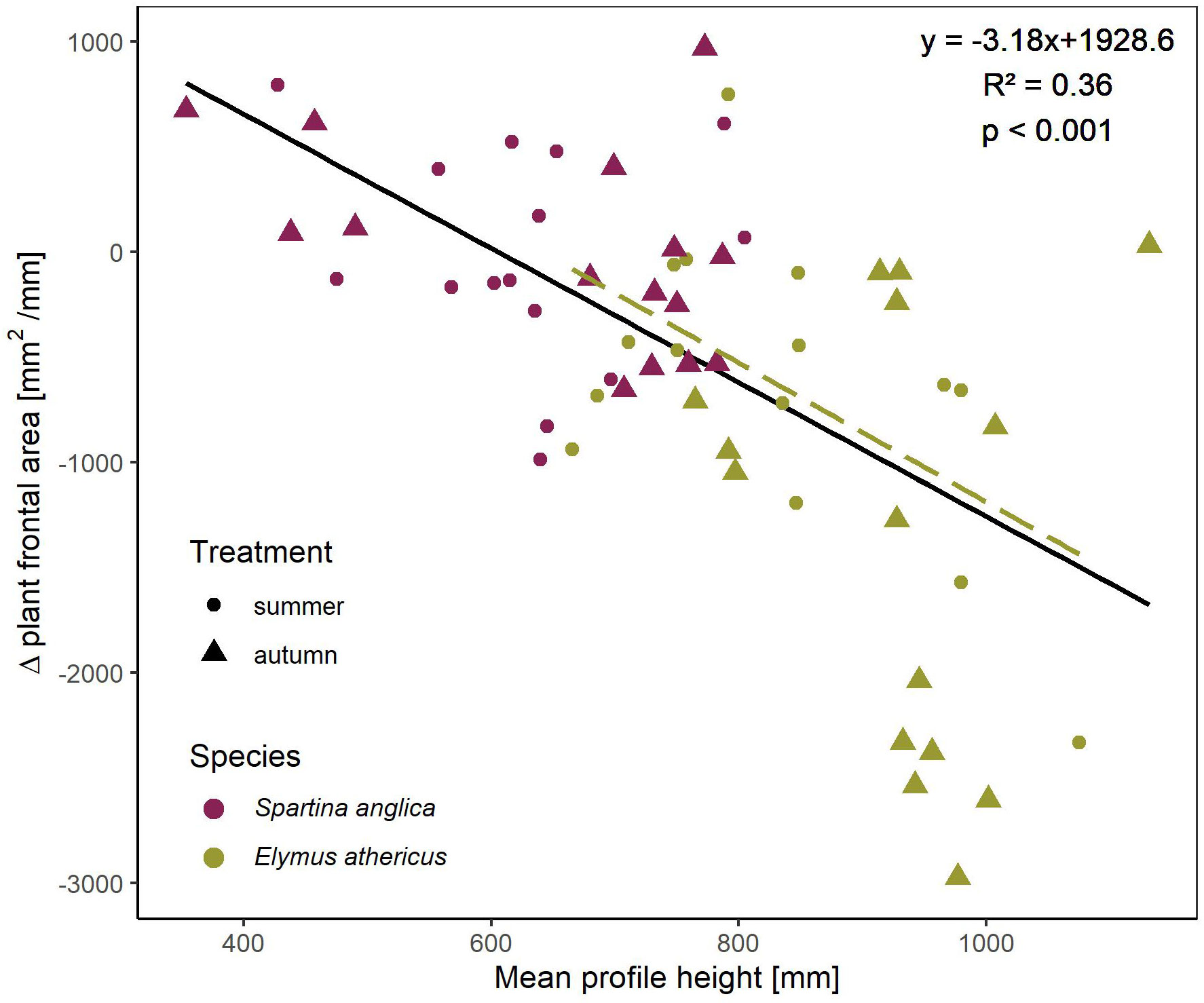
Figure 4 Linear regression showing the significant negative relationship between mean vegetation height (in mm, measured prior to wave exposure) and Δ plant frontal area of the entire data set (black line, Spartina anglica and Elymus athericus including both summer and autumn treatment). The dashed line indicates the significant linear relationship between mean vegetation height and Δ plant frontal area of the summer treatment of Elymus athericus only, while the ones of the other species-treatment combinations were not significant (Table 3) and therefore not shown here.
Biomechanical properties and plant resistance
Young’s bending modulus differed significantly between species and weeks, but no significant effects of treatment and the interactions between factors were found (Table 2). In general, Young’s bending modulus of Elymus athericus was twice as high as that of Spartina anglica (Elymus: 1169.82 ± 646.86 MPa, Spartina: 553.74 ± 315.04 MPa, Figure 5), indicating a higher stiffness of the plant material of Elymus athericus. From week to week, Young’s bending modulus increased, a trend that was more pronounced for Elymus athericus.
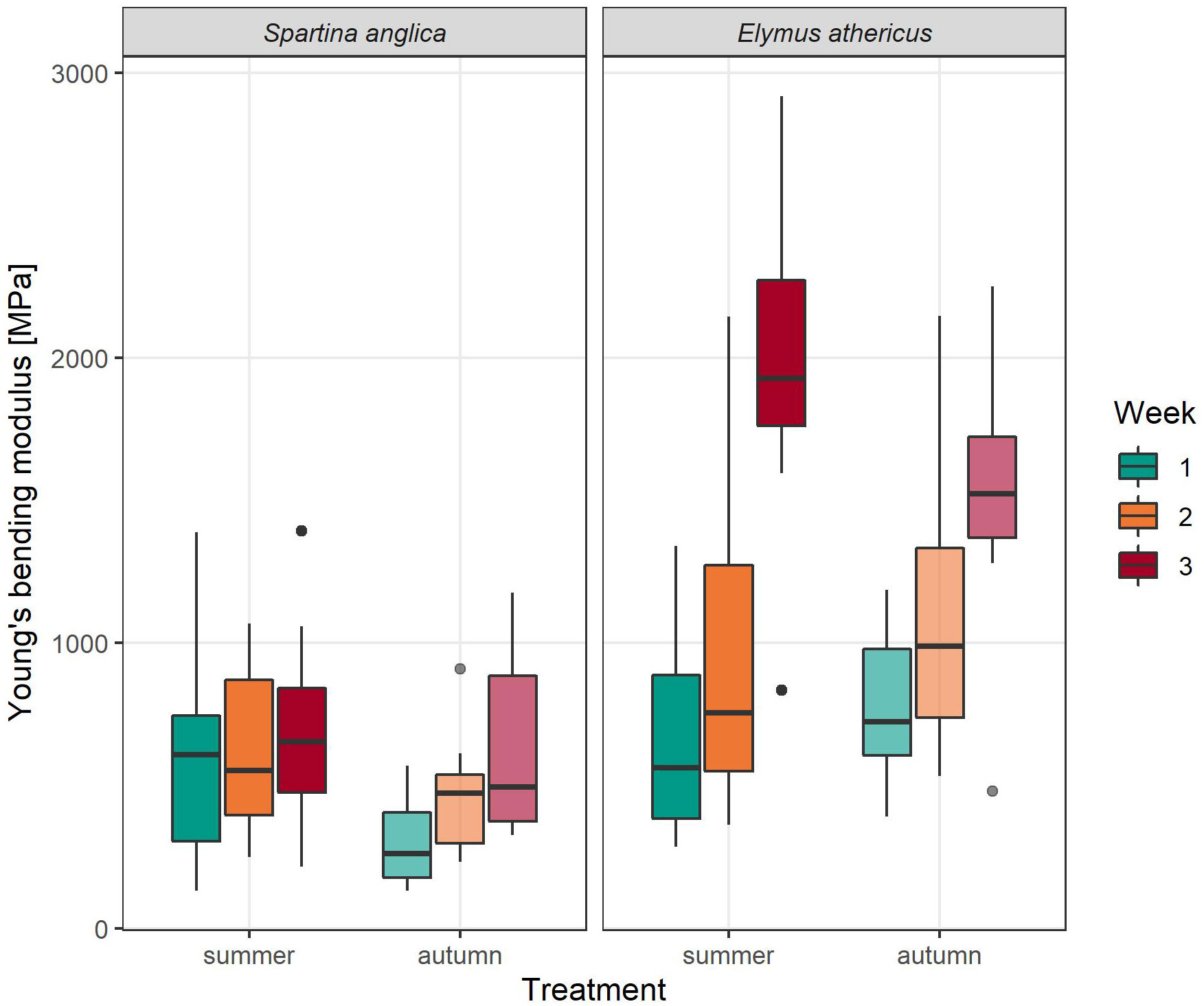
Figure 5 Young’s bending modulus (MPa) per week of Spartina anglica and Elymus athericus in summer and autumn condition.
Similarly, flexural stiffness, which describes the absolute strength of plant stems (material stiffness + stem morphology), differed significantly between species and weeks (Table 2). Furthermore, the interaction of species and week had a significant effect on flexural stiffness of the vegetation. Flexural stiffness of Spartina anglica was four times higher than of Elymus athericus (Spartina: 6.56 ± 4.39 Nm2*10-3, Elymus: 1.61 ± 1.59 Nm2*10-3), indicating that Spartina anglica stems had a higher resistance to bending than the ones of Elymus athericus. Yet no clear trend was recognizable for Spartina anglica, we found a slight increase in flexural stiffness for Elymus athericus from week to week (Figure 6).
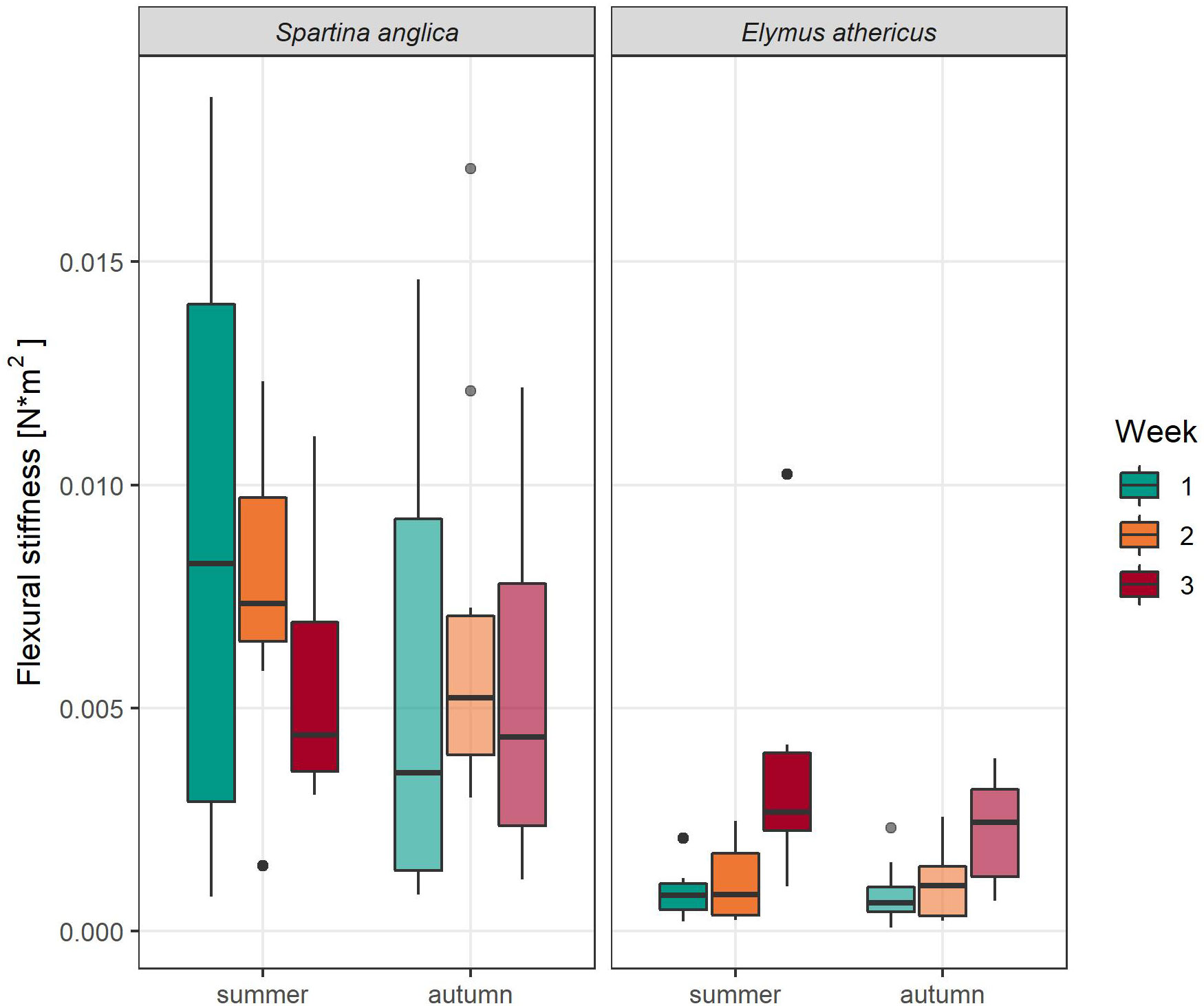
Figure 6 Flexural stiffness (Nm2) per week of Spartina anglica and Elymus athericus in summer and autumn condition.
Biochemical properties and plant resistance
We found no significant effects of species, treatment or week on the lignin and cellulose concentration of the plant material harvested in our flume experiment. Biogenic silica concentration, however, was significantly affected by species, treatment, and week but no significant interaction was found (Table 2). Biogenic silica concentration in the plant material of Elymus athericus was almost twice as high as of Spartina anglica (Elymus: 7.14 ± 1.66 mg/g dry weight, Spartina: 4.84 ± 0.85 mg/g dry weight). Plants that had been exposed to the autumn treatment had higher biogenic silica concentrations than those of the summer treatment, but this was only significant in Elymus athericus (Table 4). Regardless of the species-treatment combinations, biogenic silica concentrations of the third week were significantly higher than those measured in the first and second week (1: 5.50 ± 1.99 mg/g dry weight, 2: 5.45 ± 1.07 mg/g dry weight, 3: 7.02 ± 1.75 mg/g dry weight). Highest concentrations for biogenic silica were detected in the autumn treatment of Elymus athericus (Table 4). The biogenic silica concentration and Young’s bending modulus showed a significant positive relationship, indicating an increase in the stiffness of the plant material with increasing biogenic silica concentration (Figure 7).

Table 4 Strengthening compound concentration of plant material of two salt-marsh species in summer and autumn condition averaged over weeks (mean ± SD in mg/g dry weight).
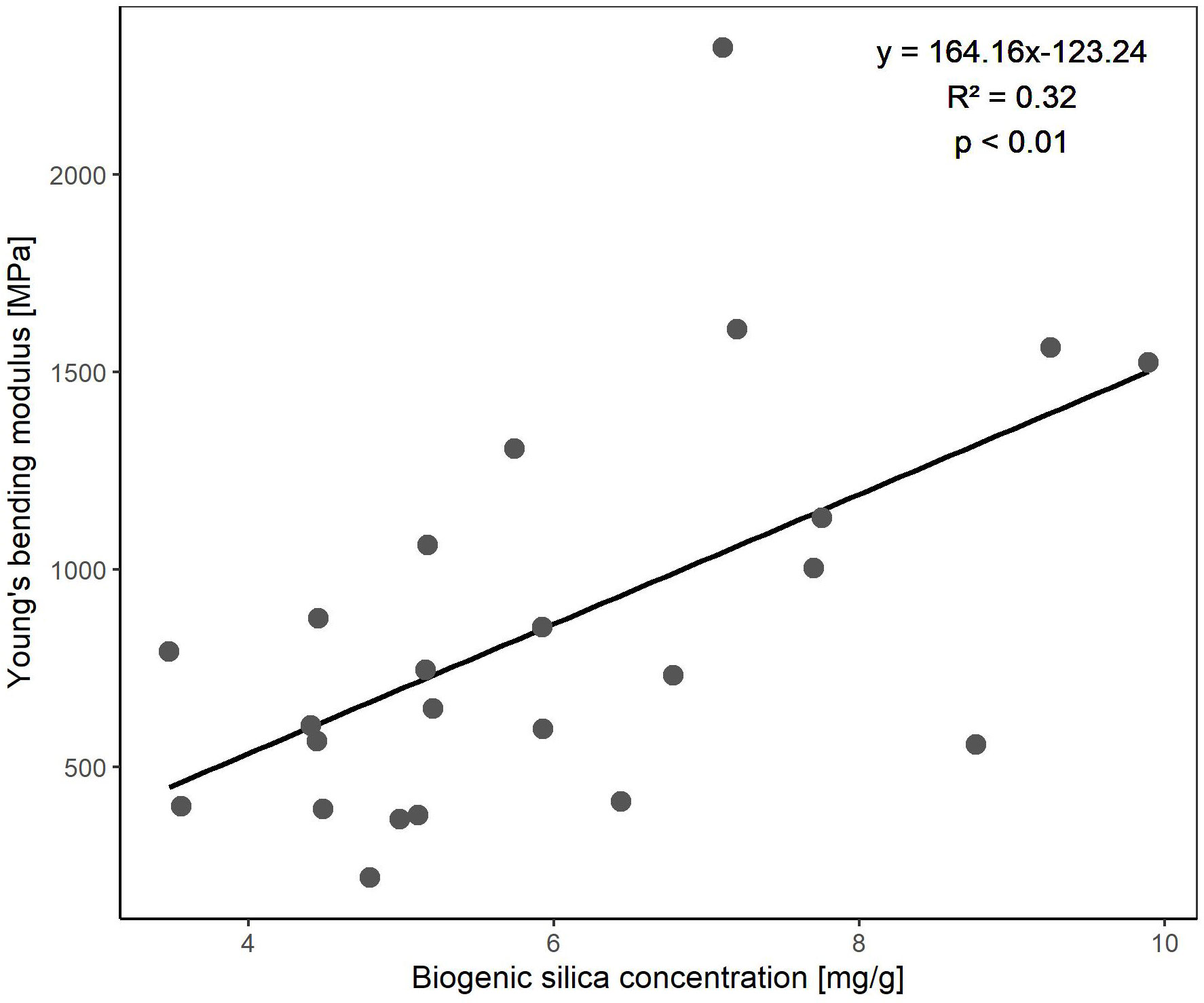
Figure 7 Linear regression showing the significant positive relationship between Young’s bending modulus and biogenic silica concentration. The data includes both species, treatments and all three weeks.
Discussion
Our study investigated the resistance of salt-marsh vegetation to wave-induced plant damage, which is a relevant question as climate change may induce changes in wave forcing on marshes, and as damage to marsh vegetation may imply loss of valuable ecosystem services. So far, studies investigating how hydrodynamic forces affect marsh vegetation focused exclusively on vital vegetation (Heuner et al., 2015; Rupprecht et al., 2017). Seasonal differences in vegetation condition were only accounted for in modelling studies (Vuik et al., 2018) and in field studies (Schulze et al., 2019; Schoutens et al., 2019; Zhu et al., 2020) not directly quantifying plant damage. This flume experiment was designed to compare wave-induced plant damage of two salt-marsh species under both summer (vital) and autumn (weakened) conditions. Furthermore, we aimed to assess whether species-specific or seasonal differences in plant damage are caused by potential differences in biomechanical, biochemical and/or morphological plant properties. In accordance with our first hypothesis, the wave forcing caused significantly more damage (measured as Δ plant frontal area) to Elymus athericus, than to Spartina anglica. Moreover, we found indications of higher plant damage in the autumn treatment compared to the summer treatment in Elymus athericus, but not in Spartina anglica, only partly supporting our second hypothesis. In the following paragraphs, we will discuss factors that may have affected our results, focusing on measured variables (canopy height, biomechanical and biochemical plant properties) and whether they are suitable determinants of plant resistance to wave-induce plant damage.
In comparison with Elymus athericus, the plant damage of Spartina anglica was relatively low. Spartina anglica is typically growing in pioneer marsh zones and is therefore regularly exposed to higher wave intensities, which may lead to a higher resistance, as compared to Elymus athericus, which grows typically in high, wave-sheltered marsh zones (Suchrow and Jensen, 2010). This adaptation mechanism has also been suggested as a possible explanation for thicker reed (Phragmites spp) stems at an exposed site on the southern shore of the Baltic Sea (Möller et al., 2011) and has been shown to exist for other marsh pioneer species (Silinski et al., 2018). Interestingly, for other species it has been shown that the opposite mechanism (i.e. a reduction in rigidity) is beneficial as it allows plants to bend under the flow, thereby reducing drag forces acting on plants (Schoutens et al., 2020). Even seedlings display different levels of plant resistance to hydrodynamic forcing depending on their site of origin, with salt-marsh species being less damaged by hydrodynamic forcing than brackish marsh species (Schoutens et al., 2021).
Vegetation height
Our results indicate that wave-induced plant damage mostly occurs in the upper part of the vegetation canopy (Figure 3) and that higher vegetation canopies (e.g. Elymus athericus) are more vulnerable to damage (Figure 4). An increase in plant damage with increasing plant height was also described in modelling studies by Vuik et al. (2018) and Duan et al. (2002). Taller plants might be more affected by wave impact because wave orbital motion and thereby wave energy is greatest at the water surface and decreases with water depth (Anderson and Smith, 2014; Möller and Christie, 2019). As Duan et al. (2002) found that the ratio of the top to base stem diameter can affect the position of plant damage along the stem this ratio might have given another possible explanation for our observation but unfortunately this has not been assessed in this study. Although plants under the autumn treatment were slightly taller than under the summer treatment, these differences might not have been big enough to affect the plants vulnerability to plant damage since we have not found significant relationships through our linear regression analyses (except for Elymus athericus in summer condition, Table 3). This suggest that vegetation height alone did not entirely explain observed pattern e.g. the higher plant damage in the autumn treatment compared to the summer treatment of Elymus athericus. However, it should be noted that one value of Elymus athericus under the autumn treatment stands out (mean vegetation height = 1131.68 mm, Δ plant frontal area = 31.89 mm2/mm, Figure 4) which might have affected the insignificance of the relationship.
Biomechanical plant properties and plant resistance
Canopy height may correlate with biomechanical plant properties because taller species tend to have stiffer stems than shorter ones (Zhu et al., 2020). In our study, this difference between taller and shorter species was only true for Young’s bending modulus, which describes the stiffness of plant material itself without taking stem morphology into account. Here the plant material of the taller species (Elymus athericus) was indeed stiffer. However, when considering the absolute strength of the stems by integrating stem morphology (i.e. flexural stiffness), the taller species Elymus athericus had weaker stems than the shorter Spartina anglica (Figure 6), which might have contributed to the higher vulnerability to wave-induced plant damage found for Elymus athericus. Flexural stiffness of Elymus athericus (1.61 ± 1.59 Nm2*10-3) was similar to values Rupprecht et al. (2015) reported for salt marshes in the Dengie Peninsula, England, (1.23 ± 0.64 Nm2*10-3). Nevertheless, flexural stiffness of Spartina anglica (6.56 ± 4.39 Nm2*10-3) was much higher in our study compared to Rupprecht et al. (2015) (3.51 ± 0.58 Nm2*10-3). Interestingly, flexural stiffness increased from week to week but only for Elymus athericus, while we see a decreasing tendency for Spartina anglica. This significant interaction effect of weeks and species on flexural stiffness might reflect different aging pattern as the plants of week two and three had more time to mature before they placed in the flume. Consequently, an adaptation to wave exposure can be neglected because we used new sets of plants every week. Neither Young’s bending modulus nor flexural stiffness were affected by the summer and autumn treatments (Table 2). Since the increase in Young’s bending modulus over the weeks was also present in the autumn treatments, it might suggest that the peak in plant stiffness with further maturation was not reached and the drought treatment was not long enough.
Biochemical plant properties and plant resistance
We have not found any significant differences between species nor treatments in strengthening compounds except for biogenic silica. Biogenic silica increases rigidity and, in contrast to cellulose and lignin, its incorporation is at lower energetic costs (Schoelynck et al., 2010). In comparison with Spartina anglica, biogenic silica concentrations were much higher for Elymus athericus, which might have resulted in the higher stiffness of the plant material (Young’s bending modulus) since a positive relationship between these two variables has been shown to exist for the entire data set (Figure 7). We also found significant higher biogenic silica concentrations in the plant material of the autumn treatment in comparison with the summer treatment (Tables 2 and 4). However, these differences between the treatments cannot directly be translated into a higher stiffness of the plant material since we have not found an effect of the treatment on Young’s bending modulus. The seasonal differences in biogenic silica concentration might have contributed to a higher stiffness of the plant material, if the drought treatment were to have been applied for longer.
Methodological considerations
Autumn treatment – Applying a drought to create an autumn treatment seems to have worked well for Elymus athericus since biomechanical properties of the autumn treatment correspond well with data gathered in the field in early spring i.e. before the onset of plant growth (flexural stiffness, our study: 1.40 ± 1.09 Nm2*10-3, field: 1.54 ± 0.58 Nm2*10-3, Schulze et al., 2019). In contrast, Spartina anglica seemed to be barely affected by the drought treatment. This could be explained by Spartina anglica being a C4 plant, which generally results in higher water use efficiency and tolerance against drought (Taylor et al., 2014). Considering the circumstances of our experimental set up, the drought treatment turned out as a good method to create the weakened autumn condition. However, for future studies, we suggest to conduct a flume study with vegetation collected in autumn to support our findings. Alternatively, and if available, climate rooms could be used to induce an autumn condition by reducing light availability and temperature.
Photo method – For our study, using the photo method for detecting plant damage fast and non-destructively generated relatively good results. This is especially true for higher wave intensities resulting in major changes in plant frontal area which was also noticeable in the amount of torn-off plant material after wave exposure (Supplementary Material Figure 3). Nevertheless, reconfiguration, which is likely to be height-dependent, cannot be excluded as contributing factor to changes in plant frontal area. In the first week, this became visible when wave intensities were not high enough to cause distinguishable damage but rather caused a rearrangement and straightening of the vegetation under inundation, which eventually led to positive values in Δ plant frontal area. Since the bottom sections of the vegetation photographs were saturated with vegetation pixels and plant parts were overlapping, changes in plant frontal area might not be as recognizable there as at the top (where vegetation was less dense). For future research on wave-induced plant damage, we recommend to use less dense vegetation if it is not possible/desirable to create extreme wave conditions. Additionally, more investigations should be conducted on the flexibility as well as concentration of strengthening compounds of the plant leaves since they contribute to plant frontal area (Zhang and Nepf, 2021) and have not been determined in this study.
Effect of weeks – Lastly, plant damage of both species increased (i.e. Δ plant frontal area decreased) from week to week (Figure 2). This can be explained by the hydrodynamic forces, which were set to increase successively. Moving of the pallets in the direction of the wave paddle in the third week further increased hydrodynamic forces potentially resulting in an amplification of plant damage, which was observed for Elymus athericus (in autumn condition) and perhaps even implies a threshold effect for this particular species-treatment combination. However, we noticed upward trends from week to week also in other variables that were independent of wave conditions such as Young’s bending modulus, flexural stiffness and biogenic silica concentration. These trends might be due to natural development as plants matured outside the wave flume prior to the placement on the flume test section, which was inevitable but should be born in mind when interpreting our results.
Although we created storm surge conditions that are typical for the NW European salt marshes, the vegetation was more robust than expected. This finding is of great importance as it provides additional support for the high resilience of salt marshes to storm impact. A species-specific characterization with respect to the vegetation’s vulnerability to wave-induced damage is important to faster assess the status and predict future responses of a salt marsh, and of course adapt management if necessary. Furthermore, we need to investigate how climate change (e.g. increased warming) affects biomechanical and morphological plant properties, which have been shown to clearly affect plant’s vulnerability to wave-induced damage. It is interesting to note here that recent studies report that Elymus athericus has formed a new genotype that is growing at lower elevations and appears to be better adapted to higher flooding frequencies (Veeneklaas et al., 2013; Reents et al., 2021). Elymus athericus is highly competitive and has, due to the new genotype, the potential to outcompete other species and further establish in lower parts of the marsh. Investigations on whether this genotype exhibits a higher resistance against increased hydrodynamic forces, is required to improve predictions on potential shifts in species composition and thereby marsh resilience in the future.
However, it is worth noting that, over longer (decadal) time scales, the susceptibility of aboveground biomass to breakage or loss may in fact reduce the risk for uprooting and hence allow the belowground biomass to remain intact during severe storm impact, as noted by Schoutens et al. (2021). Without removal of the aboveground parts of the plant, wave-forces can translate into stresses at the water/sediment interface around the plant stem that may produce scour, associated loss of sediment, and ultimately the potential uprooting of the plant itself. Following this, another focus for future studies should be to investigate whether wave-induced plant damage and thus aboveground biomass loss ultimately have a positive or negative effect on the longer-term survival chances of the vegetation and thereby provision of wave attenuation under altered future environmental conditions.
Data availability statement
The raw data supporting the conclusions of this article will be made available by the authors, without undue reservation.
Author contributions
SR conducted vegetation measurements, analyzed the data and wrote the first draft. JL conducted the three-point bending tests. BE wrote the MATLAB program for the image analysis. All authors designed the study, contributed to the article and approved the submitted version.
Funding
The work described in this publication was supported by the European Community’s Horizon 2020 Research and Innovation Programme through the grant to HYDRALAB-PLUS (contract no. 654110). SR was funded by the German Research Foundation (DFG, Deutsche Forschungsgemeinschaft, project no. 401564364) and KS by the Research Foundation Flanders, Belgium (FWO, PhD fellowship for fundamental research, 1116319 N). Additional support was provided by the RESIST-UK project (UKRI Natural Environment Research Council grant no. NE/R01082X/1). We acknowledge support by the Open Access Publication Funds of Alfred-Wegener-Institut Helmholtz-Zentrum für Polar- und Meeresforschung.
Acknowledgments
We would like to thank the team from the Forschungszentrum Küste (FZK) as well as Meline Brendel, Helen Brooks, Haobing Cao, Elizabeth Christie, Rachael Dennis, Anke van Eggermond, Grazia Doronzo and Lennart van IJzerloo.
Conflict of interest
Author JL is employed by BioConsult SH GmbH & Co. KG. The remaining authors declare that the research was conducted in the absence of any commercial or financial relationships that could be construed as a potential conflict of interest.
Publisher’s note
All claims expressed in this article are solely those of the authors and do not necessarily represent those of their affiliated organizations, or those of the publisher, the editors and the reviewers. Any product that may be evaluated in this article, or claim that may be made by its manufacturer, is not guaranteed or endorsed by the publisher.
Supplementary material
The Supplementary Material for this article can be found online at: https://www.frontiersin.org/articles/10.3389/fmars.2022.898080/full#supplementary-material
References
Adam P. (2002). Saltmarshes in a time of change. Envir. Conserv. 29, 39–61. doi: 10.1017/S0376892902000048
Anderson M. E., Smith J. M. (2014). Wave attenuation by flexible, idealized salt marsh vegetation. Coast. Eng. 83, 82–92. doi: 10.1016/j.coastaleng.2013.10.004
Armitage A. R., Weaver C. A., Kominoski J. S., Pennings S. C. (2020). Resistance to hurricane effects varies among wetland vegetation types in the marsh–mangrove ecotone. Estuaries. Coasts. 43, 960–970. doi: 10.1007/s12237-019-00577-3
Bouma T. J., Vries M.B. de, Herman P. M. J. (2010). Comparing ecosystem engineering efficiency of two plant species with contrasting growth strategies. Ecology 91, 2696–2704. doi: 10.1890/09-0690.1
Bouma T. J., Vries M.B.de, Low E., Peralta G., Tánczos I. C., van de Koppel J., et al. (2005). Trade-offs related to ecosystem engineering: A case study on stiffness of emerging macrophytes. Ecology 86, 2187–2199. doi: 10.1890/04-1588
Coops H., van der Velde G. (1996). Effects of waves on helophyte stands: mechanical characteristics of stems of Phragmites australis and Scirpus lacustris. Aquat. Bot. 53, 175–185. doi: 10.1016/0304-3770(96)01026-1
Cseresnyés I., Rajkai K., Szitár K., Radimszky L., Ónodi G., Kröel-Dulay G. (2020). Root capacitance measurements allow non-intrusive in-situ monitoring of the seasonal dynamics and drought response of root activity in two grassland species. Plant Soil 449, 423–437. doi: 10.1007/s11104-020-04505-4
Desclaux D., Roumet P. (1996). Impact of drought stress on the phenology of two soybean (Glycine max L. Merr) cultivars. Field Crops Res. 46, 61–70. doi: 10.1016/0378-4290(95)00086-0
Duan J. G., French R. H., Miller J. (2002). The lodging velocity for emergent aquatic plants in open channels. J. Am. Water Resour. Assoc. 38, 255–263. doi: 10.1111/j.1752-1688.2002.tb01549.x
Duarte C. M., Losada I. J., Hendriks I. E., Mazarrasa I., Marbà N. (2013). The role of coastal plant communities for climate change mitigation and adaptation. Nat. Clim. Change 3, 961–968. doi: 10.1038/nclimate1970
Garzon J. L., Maza M., Ferreira C. M., Lara J. L., Losada I. J. (2019). Wave attenuation by Spartina saltmarshes in the Chesapeake bay under storm surge conditions. J. Geophys. Res. Oceans. 124, 5220–5243. doi: 10.1029/2018JC014865
Gedan K. B., Kirwan M. L., Wolanski E., Barbier E. B., Silliman B. R. (2011). The present and future role of coastal wetland vegetation in protecting shorelines: answering recent challenges to the paradigm. Climatic. Change 106, 7–29. doi: 10.1007/s10584-010-0003-7
Gepstein S., Glick B. R. (2013). Strategies to ameliorate abiotic stress-induced plant senescence. Plant Mol. Biol. 82, 623–633. doi: 10.1007/s11103-013-0038-z
Groeneveld D. P., French R. H. (1995). Hydrodynamic control of an emergent aquatic plant (Scirpus acutus) in open channels. J. Am. Water Resour. Assoc. 31, 505–514. doi: 10.1111/j.1752-1688.1995.tb04037.x
Hasselmann K., Barnett T. P., Bouws E., Carlson H., Cartwright D. E., Enke K., et al. (1973). Measurements of wind-wave growth and swell decay during the joint north Sea wave project (JONSWAP). Ergänzungsheft. zur Deutschen Hydrographischen. Zeitschrift. Reihe. A Nr. 12, 1–95.
Heuner M., Silinski A., Schoelynck J., Bouma T. J., Puijalon S., Troch P., et al. (2015). Ecosystem engineering by plants on wave-exposed intertidal flats is governed by relationships between effect and response traits. PloS One 10, e0138086. doi: 10.1371/journal.pone.0138086
Koch E. W., Barbier E. B., Silliman B. R., Reed D. J., Perillo G. M. E., Hacker S. D., et al. (2009). Non-linearity in ecosystem services: temporal and spatial variability in coastal protection. Front. Ecol. Environ. 7, 29–37. doi: 10.1890/080126
Leonardi N., Carnacina I., Donatelli C., Ganju N. K., Plater A. J., Schuerch M., et al. (2018). Dynamic interactions between coastal storms and salt marshes: A review. Geomorphology 301, 92–107. doi: 10.1016/j.geomorph.2017.11.001
Mcleod E., Chmura G. L., Bouillon S., Salm R., Björk M., Duarte C. M., et al. (2011). A blueprint for blue carbon: Toward an improved understanding of the role of vegetated coastal habitats in sequestering CO 2. Front. Ecol. Environ. 9, 552–560. doi: 10.1890/110004
Möller I. (2006). Quantifying saltmarsh vegetation and its effect on wave height dissipation: Results from a UK East coast saltmarsh. Estuarine. Coast. Shelf. Sci. 69, 337–351. doi: 10.1016/j.ecss.2006.05.003
Möller I., Christie E. (2019). “Hydrodynamics and modeling of water flow in coastal wetlands,” in Coastal wetlands (Amsterdam (NL), Oxford (UK), Cambridge (USA): Elsevier), 289–323.
Möller I., Kudella M., Rupprecht F., Spencer T., Paul M., van Wesenbeeck B. K., et al. (2014). Wave attenuation over coastal salt marshes under storm surge conditions. Nat. Geosci. 7, 727–731. doi: 10.1038/ngeo2251
Möller I., Mantilla-Contreras J., Spencer T., Hayes A. (2011). Micro-tidal coastal reed beds: Hydro-morphological insights and observations on wave transformation from the southern Baltic Sea. Estuarine. Coast. Shelf. Sci. 92, 424–436. doi: 10.1016/j.ecss.2011.01.016
Möller I., Spencer T., Rawson J. (2003). “Spatial and temporal variability of wave attenuation over a UK saltmarsh,” in Coastal Engineering 2002: Proceedings of the 28th international conference - Coastal Engineering. (Singapore (SGP), New Jersey (USA), London (UK)) World Scientific. 651–663.
Niklas K. J. (1992). Plant biomechanics: An engineering approach to plant form and function (Chicago & London: The University of Chicago Press).
Paul M., Rupprecht F., Möller I., Bouma T. J., Spencer T., Kudella M., et al. (2016). Plant stiffness and biomass as drivers for drag forces under extreme wave loading: A flume study on mimics. Coast. Eng. 117, 70–78. doi: 10.1016/j.coastaleng.2016.07.004
Pinsky M. L., Guannel G., Arkema K. K. (2013). Quantifying wave attenuation to inform coastal habitat conservation. Ecosphere 4, 1–16. doi: 10.1890/ES13-00080.1
R Core Team (2021). R: A language and environment for statistical (Vienna, Austria: R Foundation for Statistical Computing).
Reents S., Mueller P., Tang H., Jensen K., Nolte S. (2021). Plant genotype determines biomass response to flooding frequency in tidal wetlands. Biogeosciences 18, 403–411. doi: 10.5194/bg-18-403-2021
Riffe K. C., Henderson S. M., Mullarney J. C. (2011). Wave dissipation by flexible vegetation. Geophys. Res. Lett. 38, 1–5. doi: 10.1029/2011GL048773
Rupprecht F., Möller I., Evans B., Spencer T., Jensen K. (2015). Biophysical properties of salt marsh canopies — Quantifying plant stem flexibility and above ground biomass. Coast. Eng. 100, 48–57. doi: 10.1016/j.coastaleng.2015.03.009
Rupprecht F., Möller I., Paul M., Kudella M., Spencer T., van Wesenbeeck B. K., et al. (2017). Vegetation-wave interactions in salt marshes under storm surge conditions. Ecol. Eng. 100, 301–315. doi: 10.1016/j.ecoleng.2016.12.030
Sade N., Del Mar Rubio-Wilhelmi M., Umnajkitikorn K., Blumwald E. (2018). Stress-induced senescence and plant tolerance to abiotic stress. J. Exp. Bot. 69, 845–853. doi: 10.1093/jxb/erx235
Schoelynck J., Bal K., Backx H., Okruszko T., Meire P., Struyf E. (2010). Silica uptake in aquatic and wetland macrophytes: A strategic choice between silica, lignin and cellulose? New Phytol. 186, 385–391. doi: 10.1111/j.1469-8137.2009.03176.x
Schoelynck J., Bal K., Puijalon S., Meire P., Struyf E. (2012). Hydrodynamically mediated macrophyte silica dynamics. Plant Biol. (Stuttg) 14, 997–1005. doi: 10.1111/j.1438-8677.2012.00583.x
Schoutens K., Heuner M., Fuchs E., Minden V., Schulte-Ostermann T., Belliard J.-P., et al. (2020). Nature-based shoreline protection by tidal marsh plants depends on trade-offs between avoidance and attenuation of hydrodynamic forces. Estuarine. Coast. Shelf. Sci. 236, 106645. doi: 10.1016/j.ecss.2020.106645
Schoutens K., Heuner M., Minden V., Schulte Ostermann T., Silinski A., Belliard J.-P., et al. (2019). How effective are tidal marshes as nature-based shoreline protection throughout seasons? Limnol. Oceanogr. 64, 1750–1762. doi: 10.1002/lno.11149
Schoutens K., Reents S., Nolte S., Evans B., Paul M., Kudella M., et al. (2021). Survival of the thickest? impacts of extreme wave-forcing on marsh seedlings are mediated by species morphology. Limnol. Oceanogr. 66, 2936–2951. doi: 10.1002/lno.11850
Schuerch M., Spencer T., Temmerman S., Kirwan M. L., Wolff C., Lincke D., et al. (2018). Future response of global coastal wetlands to sea-level rise. Nature 561, 231–234. doi: 10.1038/s41586-018-0476-5
Schulze D., Rupprecht F., Nolte S., Jensen K. (2019). Seasonal and spatial within-marsh differences of biophysical plant properties: Implications for wave attenuation capacity of salt marshes. Aquat. Sci. 81, 1–11. doi: 10.1007/s00027-019-0660-1
Silinski A., Schoutens K., Puijalon S., Schoelynck J., Luyckx D., Troch P., et al. (2018). Coping with waves: Plasticity in tidal marsh plants as self-adapting coastal ecosystem engineers. Limnol. Oceanogr. 63, 799–815. doi: 10.1002/lno.10671
Spencer T., Schuerch M., Nicholls R. J., Hinkel J., Lincke D., Vafeidis A. T., et al. (2016). Global coastal wetland change under sea-level rise and related stresses: The DIVA wetland change model. Global Planetary. Change 139, 15–30. doi: 10.1016/j.gloplacha.2015.12.018
Suchrow S., Jensen K. (2010). Plant species responses to an elevational gradient in German North Sea salt marshes. Wetlands 30, 735–746. doi: 10.1007/s13157-010-0073-3
Talukdar D. (2013). Comparative morpho-physiological and biochemical responses of lentil and grass pea genotypes under water stress. J. Nat. Sci. Biol. Med. 4, 396–402. doi: 10.4103/0976-9668.116983
Taylor S. H., Ripley B. S., Martin T., De-Wet L.-A., Woodward F. I., Osborne C. P. (2014). Physiological advantages of C4 grasses in the field: A comparative experiment demonstrating the importance of drought. Glob. Chang. Biol. 20, 1992–2003. doi: 10.1111/gcb.12498
Turner S. R., Taylor N., Jones L. (2001). “Mutations of the secondary cell wall,” in Plant cell walls. Eds. Carpita N. C., Campbell M., Tierney M. (Dordrecht: Springer Netherlands), 209–219.
Veeneklaas R. M., Dijkema K. S., Hecker N., Bakker J. P. (2013). Spatio-temporal dynamics of the invasive plant species Elytrigia atherica on natural salt marshes. Appl. Veg. Sci. 16, 205–216. doi: 10.1111/j.1654-109X.2012.01228.x
Vuik V., Suh Heo H. Y., Zhu Z., Borsje B. W., Jonkman S. N. (2018). Stem breakage of salt marsh vegetation under wave forcing: A field and model study. Estuarine. Coast. Shelf. Sci. 200, 41–58. doi: 10.1016/j.ecss.2017.09.028
Ysebaert T., Yang S.-L., Zhang L., He Q., Bouma T. J., Herman P. M. J. (2011). Wave attenuation by two contrasting ecosystem engineering salt marsh macrophytes in the intertidal pioneer zone. Wetlands 31, 1043–1054. doi: 10.1007/s13157-011-0240-1
Zhang W., Ge Z.-M., Li S.-H., Tan L.-S., Zhou K., Li Y.-L., et al. (2022). The role of seasonal vegetation properties in determining the wave attenuation capacity of coastal marshes: Implications for building natural defenses. Ecol. Eng. 175, 106494. doi: 10.1016/j.ecoleng.2021.106494
Zhang X., Nepf H. (2021). Wave-induced reconfiguration of and drag on marsh plants. J. Fluids. Structures 100, 103192. doi: 10.1016/j.jfluidstructs.2020.103192
Zhu Z., Yang Z., Bouma T. J. (2020). Biomechanical properties of marsh vegetation in space and time: Effects of salinity, inundation and seasonality. Ann. Bot. 125, 277–290. doi: 10.1093/aob/mcz063
Keywords: salt marshes, flume experiment, wave-induced damage, plant properties, seasonality
Citation: Reents S, Möller I, Evans BR, Schoutens K, Jensen K, Paul M, Bouma TJ, Temmerman S, Lustig J, Kudella M and Nolte S (2022) Species-specific and seasonal differences in the resistance of salt-marsh vegetation to wave impact. Front. Mar. Sci. 9:898080. doi: 10.3389/fmars.2022.898080
Received: 16 March 2022; Accepted: 21 November 2022;
Published: 14 December 2022.
Edited by:
Juan Jose Munoz-Perez, University of Cádiz, SpainReviewed by:
Xiaoxia Zhang, Dalian University of Technology, ChinaSamantha Chapman, Villanova University, United States
Copyright © 2022 Reents, Möller, Evans, Schoutens, Jensen, Paul, Bouma, Temmerman, Lustig, Kudella and Nolte. This is an open-access article distributed under the terms of the Creative Commons Attribution License (CC BY). The use, distribution or reproduction in other forums is permitted, provided the original author(s) and the copyright owner(s) are credited and that the original publication in this journal is cited, in accordance with accepted academic practice. No use, distribution or reproduction is permitted which does not comply with these terms.
*Correspondence: Svenja Reents, c3ZlbmphLnJlZW50c0Bhd2kuZGU=