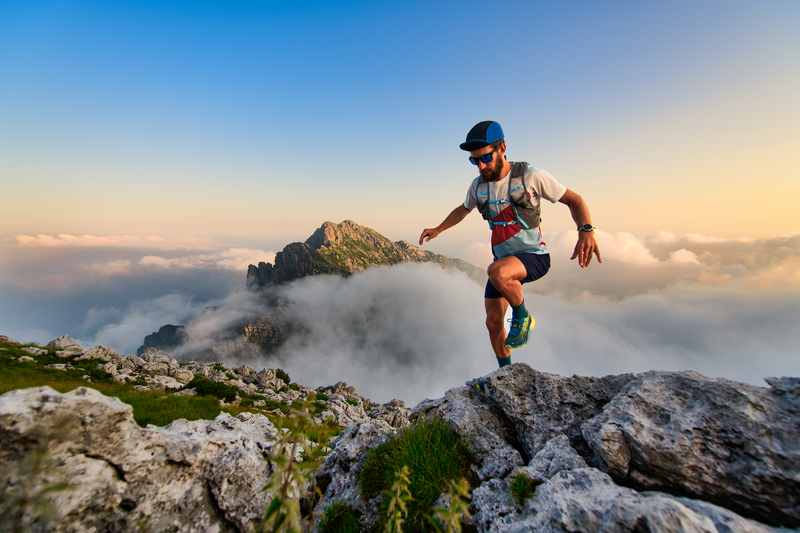
95% of researchers rate our articles as excellent or good
Learn more about the work of our research integrity team to safeguard the quality of each article we publish.
Find out more
ORIGINAL RESEARCH article
Front. Mar. Sci. , 10 October 2022
Sec. Global Change and the Future Ocean
Volume 9 - 2022 | https://doi.org/10.3389/fmars.2022.895354
Global warming and Marine Heat Waves (MHWs) are having large-scale impacts on the seagrasses and their effects on the Mediterranean endemic Posidonia oceanica need to be properly defined. This research aimed to sharpen the knowledge on P. oceanica response to the warming by correlating the shoot morphology and the productivity of the seagrass to temperature conditions and MHW occurrence. Two correlative studies, along a Mediterranean temperature range (sites at the same latitude), were conducted: one explored the associations of summer 2021 Sea Surface Temperature (SST) and MHWs on P. oceanica morphology and the other used a reconstruction technique (lepidochronological analysis) to relate past temperature conditions and MHW occurrence to proxies of seagrass productivity for the corresponding periods. The results showed that the mean summer temperature affected the P. oceanica leaf necrosis and that MHWs occurrence changed the morphology of the plant by lowering the leaf area and increasing leaf necrosis. Interesting results were also found relating the past thermal environment, since rhizome biomass has been negatively affected by the number of MHWs and rhizome length by the temperature range. This research provides fundamental insights about P. oceanica changes linked to warming and MHWs, identifying the potential plant indicators assisting future experimental and modelling studies. To the best of our knowledge, this is the first time a reconstruction technique is used to evaluate the effects of past MHWs on the morphology and productivity of a plant species.
Global warming and climate change are expected to have profound consequences on biodiversity and functioning of ecosystems on earth (Hoegh-Guldberg and Bruno, 2010; Wernberg et al., 2013). The temperature increase has been clearly measured over the past two decades (Jordà et al., 2012; Darmaraki et al., 2019a; Soto-Navarro et al., 2020), but understanding how this physical forcing translates into effects on the living world is far from trivial, especially in the sea. Nevertheless, this information is critical to forecast the range of consequences of global warming and to address management uncertainties and restoration efforts (Pazzaglia et al., 2021).
Extreme climatic events (ECEs, e.g., hurricanes, floods, heat waves), direct consequence of the global warming, are no longer to be considered as natural events (Ruddiman, 2003; Bianchi et al., 2012; Cook et al., 2016) since they are intensifying according to the increase of the greenhouse gas forcing (Coumou and Rahmstorf, 2012). Marine Heat Waves (MHWs), i.e., prolonged discrete anomalously warm water events (Hobday et al., 2016), have increased in intensity, frequency and duration over the past century (Oliver et al., 2018; Darmaraki et al., 2019a) with different implications for marine ecosystems (Frölicher and Laufkötter, 2018; Smale et al., 2019). In fact, the increasing number of MHWs and their intensity were already correlated with increased coral bleaching, decreased kelp biomass and seagrass (Smale et al., 2019). Therefore, these warming events are associated to the reduction in the abundance of critical foundation species and the subsequent shift in community structure towards a depauperate state (Wernberg et al., 2013). Furthermore, damages in ecosystems due to warming events can also bring political and socio-economic issues when affecting, for example, aquaculture or important fishery species (Frölicher and Laufkötter, 2018).
Global warming and MHWs can have large-scale impacts on the seagrass communities (Orth et al., 2006; Strydom et al., 2020) and on the services they provide (Aoki et al., 2021). At a global scale, significant meadow mortalities and subsequent loss of biodiversity related to seagrass die-offs have been correlated to MHWs occurrence (Marbà and Duarte, 2010; Fraser et al., 2014; Lefcheck et al., 2017; Nowicki et al., 2019; Shields et al., 2019; Smale et al., 2019; Strydom et al., 2020). The rates of seagrass decline, due to a wide variety of human activities (Duffy, 2006; Orth et al., 2006; Dunic et al., 2021; Turschwell et al., 2021), have accelerated from a median of 0.9% per year before the 1940’s to 7% per year since 1990 (Waycott et al., 2009) and, even though encouraging results have been found for most of the fast-growing species (de Los Santos et al., 2019), the alarming rate of deterioration might trigger significant ecological consequences. Warming in the Mediterranean Sea is two to three-fold faster than in the global ocean (Diffenbaugh et al., 2007) and a fast increase in the mean sea surface temperature (SST) and in the frequency and duration of MHWs is projected for the near future (Giorgi and Lionello, 2008). This is because the Mediterranean (1) lies between two climatic regimes, the arid North Africa and the temperate, rainy central European, which renders climate vulnerable even to small changes in circulation (Giorgi and Lionello, 2008), and (2) is a semi-enclosed basin (Diffenbaugh et al., 2007) with limited hydrological exchange with the Atlantic ocean, which results in about 100 years hydrological residence time and a capacity to store heat (Bethoux and Gentili, 1999). The SST has increased in the last decades at an average ( ± SD) rate of 0.03 ± 0.008°C yr–1 in the western basin and 0.05 ± 0.009°C yr–1 in the eastern basin (Nykjaer, 2009; Bianchi et al., 2012) and the change projected by the end of this century ranges between 0.81 and 3.71°C in the upper layer (0 – 150 m) (Soto-Navarro et al., 2020).
The Mediterranean warming could produce an irreversible regression of P. oceanica (L.) Delile (Jordà at al., 2012; Chefaoui et al., 2018). This iconic seagrass is an endemic species to the Mediterranean Sea, where forms extended meadows widely distributed and occupying ca. 26% of the whole Basin coastal areas, notwithstanding slow growth and recovery rates (Telesca et al., 2015). As other seagrass species, P. oceanica is experiencing a widespread decline due to several anthropogenic local stressors, such as coastal development, dredging, pollution, fish farming, mooring and invasive species (Holon et al., 2015; Telesca et al., 2015; Burgos et al., 2017). In addition to those stressors, climate warming and ECEs seem to play a major role in the decline of this seagrass (see Nguyen et al., 2021 for a review).
An important shoot mortality of P. oceanica was estimated in meadows during 2002-2006 where two intense MHWs occurred in Spain (Marbà and Duarte, 2010). Depending on the temperature intensity and heat duration, MHWs can also have sublethal morphological effects on both P. oceanica cuttings (Stipcich et al., 2022) and seedlings (Guerrero-Meseguer et al., 2017), by inducing leaf loss, reducing leaf growth, and triggering leaf necrosis. Seagrasses may be highly susceptible to sudden warming events (Chefaoui et al., 2018) and a functional extinction of P. oceanica by the middle of this century has been hypothesized (Jordà et al., 2012). The abrupt decline experienced by P. oceanica populations, especially after recent summer MHWs (Garrabou et al., 2009; Marbà and Duarte, 2010), has raised serious questions about its sensitivity, vulnerability, and persistence for the coming decades, as its fundamental niche could shrink for the general warming and/or heat events (Pergent et al., 2015). The performance of the P. oceanica, in terms of shoot morphology and productivity, related to the heat can be trait-mediated (Peirano et al., 2011; Pansini et al., 2021). However, contrasting results about the resilience of P. oceanica depending on the regional differences in ocean climate were found (Marín-Guirao et al., 2016; Marín-Guirao et al., 2019; Bennett et al., 2021; Bennett et al., 2022) and understanding whether the thermal performance of the plant reflects the thermal geography is still controversial. Therefore, defining the importance of local adaptation and phenotypic plasticity among P. oceanica populations remains an urgent issue to predict when and where climate change impacts will occur. In this context, the identification of early indicators of both nearly lethal thermal conditions and adjustments of the habitat structure is also necessary to improve predictions about the future ecology of the seagrass.
Photoperiod controls many developmental responses of plants by affecting the detection of the light signal in the leaves, the entrainment of circadian rhythms, and the production of a mobile signal which is transmitted throughout the plant (Jackson, 2009). Therefore, using sites located all at the same latitude but in a wide temperature gradient could function as a natural laboratory to investigate the potential impacts of climate warming precluding undesired effects of the photoperiod (De Frenne et al., 2013).
To sharpen the forecasting capability on P. oceanica response to the warming and MHW occurrence two correlative studies (summer 2021 study and reconstruction study) were performed using five sites along a Mediterranean-wide water temperature range at the same latitude. Particularly, the summer 2021 study has tested the hypothesis that summer water temperature and MHWs negatively affect P. oceanica shoot morphology and has estimated their effects on shoot descriptors (number of leaves, leaf width, leaf area and leaf necrosis). Furthermore, the reconstruction study has tested the hypothesis that rhizome productivity (rhizome length, rhizome width, rhizome biomass and # of sheaths per year) is negatively related to water temperature (mean and range) and MHWs occurrence (frequency and duration) during the reconstructed period with the aim of understanding what will be the response of the plant in the future Mediterranean climate scenario based on the past performance to these stressors.
Five sites were selected nearly at the same latitude across the wide longitudinal range (between 10-35°E, centred around 35°N) in the Mediterranean Sea, (Figure 1): Konnos Bay (C1) and Akrotiri Cape (C2) in Cyprus (South-eastern coast and South of Cyprus, respectively), Pigadia (KA) and Gournes (CR) in Greece (South-eastern coast of Karpathos, and Northern Crete, respectively), and Cala Pulcino (LA) in Italy (South of Lampedusa Island). All the selected sites were far from direct anthropogenic stressors due to urbanization and ports. In each site, three areas with P. oceanica meadows on sandy bottom at about 10 m of depth, distant 50 – 100 m apart, were randomly selected. At each area, meadows were always dense (400 – 700 shoots/m2 according to Giraud, 1977).
Figure 1 Location of the study sites: LA, Cala Pulcino; CR, Gournes; KA, Pigadia; C1, Konnos Bay; C2, Akrotiri cape.
Due to the Mediterranean Sea circulation, which is affected in turn by wind stress, heat flux, surface salinity, topography, and lateral boundary runoff (Zavatarielli and Mellor, 1995), the five sites represent distinct sea water thermal environments (Table 1). To characterize the sites and to identify temperature gradients, daily SST of the last 30 years (1990-2020) was acquired through the website https://coastwatch.pfeg.noaa.gov/erddap/index.html. The annual and summer seasonal means, max and min temperature were calculated as well as the sea water climatology using the rerddap (Scott, 2021) package in (Core team, R.C.T.R, 2013). Furthermore, all the MHWs that occurred in each site during summer 2021 and the reconstructed period were identified using the heatwaveR (Schlegel and Smit, 2018) package in R. MHWs were calculated based on the definition of Hobday et al. (2016), i.e. prolonged discrete anomalously warm water events, where an event becomes ‘anomalously warm’ if the SST is above the 90th percentile of the climatology (based on minimum 30 years of SST data), ‘discrete’ is referred to the well-defined start and end times, and ‘prolonged’ when the SST over the threshold is recorded for minimum 5 days. If between two events there is a gap (SST below the threshold) of two or less days, the two events are considered as one whole event. A MHW is commonly defined by its intensity (°C over the threshold), duration (in days) and frequency (# of events over time). In this study only MHW duration and frequency were considered.
Table 1 Temperature and MHW conditions at the five sites (Konnos Bay=C1, Akrotiri Cape=C2, Pigadia=KA, Gournes=CR, Cala Pulcino=LA).
At each site, for each area, P. oceanica shoot density was estimated in June 2021 using 40×40 cm quadrats randomly placed within the meadows (n=12 per site, Figure S1). During summer 2021, three or four sampling times (T1=end of June, T2=end of July, T3=end of August and T4=end of September) about 30 days apart (depending on the site) were considered. In each site, ten P. oceanica orthotropic shoots per area were manually collected on each time (n=30 per site) by scuba-divers. Once collected, P. oceanica shoots were transported to the laboratory and stored frozen. In the laboratory, for each shoot leaves and rhizomes were separated: leaves morphometric data were used for the summer 2021 study, while rhizomes of shoots collected in T1 were used for the reconstruction study (Figure 2).
Figure 2 Summary of the usage of P. oceanica shoots collected. In the centre, information about the analyses done using the leaves and rhizome. On the right, the response variables obtained from the two analyses.
For the summer 2021 study, morphological variables of each shoot (number of leaves, leaf width (cm), total leaf area (cm2) and the portion (%) of leaf necrosis) were estimated for every sampling time (T1, T2, T3 and T4) by measuring the leaves previously separated (Figure S2).
Then, each sampling time was attributed to a summer period (T1 and T2 to early summer=ES; T3 and T4 to late summer=LS) according to the seasonal morphological changes of P. oceanica (Alcoverro et al., 1995). Then, at each site, for each sampling time, we used mean temperature, temperature range, and number of MHWs and MHW duration occurring within the period since the previous sampling time (for the first sampling time the period since June 1st to T1 was considered) and, to predict seagrass morphological variables (Table 1).
In the reconstruction study, a lepidochronological analysis was used to reconstruct the rhizome productivity of the 30 shoots collected in each site in T1. This analysis is based on the cyclic annual variation of the sheath thickness (Pergent-Martini et al., 1994); the sheath is the leaf portion that remains attached to the rhizome after each leaf falls. For each shoot, sheaths were detached and the thickness of each of them was measured with a caliper. The number of sheaths produced between two pairs of minimum thickness corresponds to the number of leaves produced during that lepidochronological (Lep) year. Each Lep year is the period delimited by two consecutive sheaths-thickness minima in the rhizome (Pergent-Martini and Pergent, 1996). Based on the notion the minimum thickness appears in spring and the maximum thickness in autumn (Pergent-Martini et al., 1994), April 1st was assumed to be the first day of every Lep year. Thanks to this analysis it was possible to estimate for each shoot several proxies of the rhizome production per Lep year (i.e. number of leaves by counting the sheaths), rhizome width and length (by using a caliper) and rhizome biomass (by using an electronic analytic scale, after drying rhizomes for 48 h at 60°C).
Moreover, for each site, the water climatology and the daily SST from the oldest reconstructed Lep year to the beginning of 2020 (but excluding from computations the last one year and half) were used to estimate the explanatory variables, annual mean and temperature range, and the number (frequency) and duration of MHWs for each Lep year (Tables 2, 3), which were averaged for each Lep year and linked to the lepidochronological variables of the same periods.
Before statistical analyses, data were explored following Zuur et al. (2009). Outliers were inspected with Cleveland dot-plots and normality with histograms and Q–Q plots. Collinearity between continuous explanatory variables was inspected with pair-plots, and variance inflation factors (VIFs) were calculated. In the summer 2021 dataset, mean temperature, range of temperature and MHW duration were correlated, and therefore only mean temperature and number of MHWs (as a factor with three levels: 0, 1 and 2) were considered. Further, meadow shoot density was also considered as an explanatory variable in the related analyses, since it may influence P. oceanica morphological traits (Pansini et al., 2021). In the reconstruction study dataset, no correlation was found between explanatory variables, thus they were all included in the analysis.
Since data exploration indicated non-linear relationships between each response variable and the explanatory variables, generalized additive models (GAMs) were used. For the summer 2021 study, four distinct GAMs were run to correlate separately the morphological response variables (leaf area, leaf width, number of leaves and necrotic leaf portion) with the continuous explanatory variables (mean temperature and shoot density as smoothed non-linear terms) and the factors (site, period and number of MHWs as categorical variables):
Yi = α + f1(mean temperature) + f2(shoot density) + site + period + number of MHWs + ϵi
where Y is the response variable, fi are the smooth functions estimated by the models and ϵi ∼ N(0, σ2).
For the reconstruction study, four distinct GAMs were run to correlate separately the productivity response variables (number of sheaths, rhizome length, width and biomass) with the continuous explanatory variables (mean temperature, temperature range, MHW duration and number of MHWs as smoothed non-linear terms) and the factor (site as categorical variable):
Yi = α + f1(mean temperature) + f2(temperature range) + f3(MHW duration) + f4(number of MHW) + site + ϵi
where Y is the response variable, fi are the smooth functions estimated by the models and ϵi ∼ N(0, σ2).
The function gam in the mgcv package in R was used to perform the analysis. To avoid model overfitting, the degrees of freedom of the smooth were limited using the k parameter and the REML (restricted maximum likelihood) method was used to increase the robustness, as suggested to cope with small sample sizes (Zuur, 2012).
Following a forward selection approach (Zuur et al., 2009), the choice of the best fitting explanatory variables used in the final model was undertaken based on the lowest AIC (Akaike information criterion) (Figures S3-10). Model validations were run calculating and plotting the Pearson residuals against the (i) fitted values, (ii) each explanatory variable in the model, and (iii) each explanatory variable not in the model (Figures S3-10) (Wood, 2006). A significant p-value was set at <0.01.
The water climatology varied widely thought sites (Table 1 and Figure 3) and the annual temperature range changed between 8.75°C and 11.46°C in KA and LA, respectively. In terms of mean annual SST there was a maximum of 1.76°C of difference from 19.81°C in CR to 21.57°C in C1, while mean summer SST showed a maximum of 2.74°C of difference from 23.96°C up to 26.70°C in KA and C1, respectively. The latter was similar to the difference (2.73°C) in maximum SST which ranged between 24.81°C in KA and 27.54°C in C1.
Figure 3 Climatology monthly variation (SST from 1990-2020) in the five study sites (C1, Konnos Bay; C2, Akrotiri cape; KA, Pigadia; CR, Gournes; LA, Cala Pulcino).
During the summer 2021 study, MHWs occurred in both periods (ES and LS) in two sites (CR and KA), while for all the other sites MHWs occurred only during the LS (Figure 4). The longest MHW occurred in LA, while the more intense was found in C2, both in the LS. The study of the past MHWs at the sites (reconstruction study) revealed that the maximum wave intensity of 1.6°C over the threshold (90th percentile of the climatology, Hobday et al., 2016) was reached at CR, while the maximum duration (more than 100 days) was recorded in KA between November 2020 and March 2021, an exceptionally long wave. MHWs in LA were overall the less intense and shorter, lasting on average 12.65 days (Figure 4).
Figure 4 (A) Summer 2021 study. Marine Heat Waves that occurred in the five sites during Early (ES, triangle) and Late (LS, circle) summer 2021 periods. (B) Reconstruction study. Intensity and duration of the Marine Heat Waves that occurred during the reconstructed period (2011-2021) in the five study sites. C1, Konnos Bay; C2, Akrotiri cape; KA, Pigadia; CR, Gournes; LA, Cala Pulcino.
The influence of the mean summer temperature and MHWs on the morphological variables was detectable, although an effect of meadow density, site and period was also found. Leaf area was negatively affected by MHWs, being reduced by their increasing frequency, while leaf width was positively associated with the number of MHWs only if higher than one (Figures 5A, B, Table 4 and Tables S1, S2). The leaf necrosis increased with higher mean summer temperature and number of MHWs (Figure 5C, Table 4 and Table S3). During summer (from the ES to the LS), leaf area decreased (Figure 5A). Leaf area and the leaf necrosis were negatively related to shoot density, if higher than 700/m2 and 600/m2, respectively (Figures 5A, C, Table 4 and Tables S1, S3). All the morphological variables were associated with the site, although the deviance explained by the models for leaf width and number of leaves was low (Figures 5A-D, Table 4 and Tables S1-S4).
Figure 5 Summer 2021 study. Summary of GAM results on morphological variables (A)= leaf area; (B)=leaf width; (C)=leaf necrosis; (D)=# of leaves) showing the significant explanatory continuous variables of the models: mean temperature and shoot density. The y-axis represents the additive predictor by GAM, which is a smoothed function for mean temperature and shoot density. The solid line is the smoother and the dotted lines are 95% point-wise confidence bands. The boxplots show the GAM results for the significant parametric terms: i) # of MHWs (0, 1 and 2); ii) site (C1, C2, CR, KA, and LA); iii) period (ES and LS). The thick black lines represent the medians, the boxes encompass the 25% and 75% quartiles, the whiskers extend to the most extreme data points within 1.5× the interquartile range outside the box, and the circles show data points beyond the whiskers. Leaf area is expressed in cm2; leaf width in cm and leaf necrosis in %.
The lepidochronological analysis allowed to reconstruct up to 16 years in C1, nine in C2, 11 in KA, 14 in CR and eight in LA. The thermal descriptors influenced all the P. oceanica lepidochronological variables, with the only exception of the number of sheaths. Particularly, we found: i) a positive relationship between rhizome length and the mean temperature, and a slightly negative relationship with the temperature range (Figure 6A; Table 5 and Table S5; ii) a negative association between rhizome biomass and the number of MHWs (Figure 6C; Table 5 and Table S7), and iii) a negative association between rhizome width and MHW duration (up to 60 days) (Figure 6B; Table 5 and Table S6). All the response variables were associated with site (Figures 6A-D; Table 5 and Tables S5-S8).
Figure 6 Reconstruction study. Summary of GAM results on lepidochronological variables (A)=rhizome length; (B)=rhizome width; (C)=rhizome biomass; (D)=# of sheaths) showing the significant explanatory continuous variables of the models: mean temperature, range temperature, # of MHWs and MHW duration. The y-axis represents the additive predictor by GAM, which is a smoothed function for all the variables. The solid line is the smoother and the dotted lines are 95% point-wise confidence bands. The boxplots show the GAM results for the significant parametric term site (C1, C2, CR, KA, and LA). The thick black lines represent the medians, the boxes encompass the 25% and 75% quartiles, the whiskers extend to the most extreme data points within 1.5× the interquartile range outside the box, and the circles show data points beyond the whiskers. Rhizome length and width are expressed in cm; rhizome biomass in g.
The results obtained from the summer 2021 study using the temperature gradient sites at the same latitude indicated that the water thermal environment can deeply affect the P. oceanica shoot morphology. During summer 2021, leaf necrosis was related to the mean temperature while all the morphological traits of P. oceanica were related to the number of MHWs (except the number of leaves). Detection of these changes was overall possible despite the high variation among sites in these plant traits. The strong relationship between traits and temperature changes may point to local adaptation to temperature and suggests that response to future climate warming might also vary at the same latitude depending on the thermal environment (Tryjanowski et al., 2006). The total leaf area decreased when the number of MHWs increased, suggesting that temperature intensity would be crucial in discerning facilitative from inhibition effects. The summer period (ES vs LS) was also associated to the total leaf area which decreased in the late season, possibly due to the natural rhythm of the species (Pergent and Pergent-Martini, 1991). Nevertheless, the summer period did not affect the leaf necrosis which instead was associated to the temperature: for this variable, the analysis allowed us to disentangle the effect of the heat from that one due to the natural cycle of the plant, suggesting that leaf necrosis may be an important morphological warning signal to the mean summer temperature and number of MHWs. This indication converges with evidence gained from other seagrass species (Pagès et al., 2010; Llagostera et al., 2016), whereby leaf necrosis is considered as an indicator of irreversible heat-induced damage of the leaf tissue (Beca-Carretero et al., 2020). However, further research is necessary to question to what extent the necrosis should be seen as a sign of leaf senescence or a manifestation of the activation of a process that could improve the survival of the plant, as a higher percentage of necrosis was found after the MHWs in P. oceanica shoots from warmer sites compared to those from colder sites (Stipcich et al., 2022). Overall, shoot density of P. oceanica meadows at the study sites was found to influence morphological traits. In fact, shoot density not only can provide important information about the health status of the meadow and the human influences on this habitat (Pergent et al., 1995; Montefalcone, 2009), but it can also indicate changes in morphological traits (Pansini et al., 2021). In the present research, in fact, the leaf area and leaf necrosis decreased over a shoot density threshold. It is well known that the plant uses changes in shoot density as an adaptation to minimize the self-shading, due to increasing depth or sedimentation (e.g. Olesen et al., 2002: Ralph et al., 2007; Kletou et al., 2020). According to the results, some morphological traits (leaf area and necrosis) change at high shoot densities to cope with a similar issue, in order to maximize the efficiency of the photosynthesis.
Furthermore, the reconstruction study has provided evidence that rhizome productivity has been highly affected by MHWs through the past years. The yearly production of rhizome biomass, in fact, decreased with MHW frequency. This result provides relevant information to understanding P. oceanica performance in the near future, since MHWs are predicted to increase in duration, intensity, and frequency (Darmaraki et al., 2019b). Many studies have already pointed out the importance that MHWs can have on seagrasses in terms of die-offs and habitat losses (Wernberg et al., 2013; Smale et al., 2019), rates of photosynthesis and respiration (Marín-Guirao et al., 2016), and sediment carbon stocks (Aoki et al., 2021), but referring these processes mostly to summer heat waves. In our research, rhizome production of P. oceanica was associated to the MHWs identified during the annual past cycles, providing supporting evidence that the deleterious effect of this climate change-related stressor is detectable even in reconstruction analyses. Particularly, we identified patterns for both the components of rhizome biomass and the width: a threshold of MHWs duration (about 30 days) was detected over which rhizome width decreased, while changes in rhizome biomass were found associated to the number of MHWs up to six per year. Furthermore, rhizome length was also positively associated to the mean annual temperature, as it did not change within the 21-22.5°C interval, suggesting the stimulating effect of the average temperature. Consistent changes in rhizome productivity were already demonstrated in P. oceanica (Peirano et al., 2011; Pansini et al., 2021) and other seagrasses (Masini et al., 2001; Collier et al., 2017) in relation to mean seasonal or annual temperature, but the identification of past MHWs and temperature range effects represents a novelty. The rhizome length, in fact, decreased when the temperature range increased assuming that, for a wide temperature interval (over 13°C), the plant may not be able to develop the same survival strategy. Plant productivity was also tightly associated to the site effect in all the variables considered showing as well a local adaptability. Indeed, the number of sheaths per year was not related to the climate change related stressors (MHWs and SST increase), but likely reflected other local environmental conditions (Pergent-Martini et al., 1994; Guidetti, 2001; Balestri et al., 2004).
In conclusion, P. oceanica shoot morphology is likely to be deeply affected by the global warming. The expected morphological changes of the leaves, based on the evidence collected (higher leaf width and higher leaf necrosis), might represent the plasticity response to the future temperature conditions. However, in the long run, the morphological changes may have consequences on the functions of this habitat compromising the maintenance of goods and services that seagrass meadows provide. For example, because leaf morphological changes are commonly tied to biochemical properties (Marín-Guirao et al., 2018; Ruocco et al., 2018; Stipcich et al., 2022), they may also be linked to changes in trophic interactions between the producer and consumers: in fact, if metabolites are remodeled due to physical conditions changes in epiphyte community (Piazzi et al., 2018), nutritional values and palatability of the seagrass are also expected (Carmen et al., 2012). Future meadow services could be also affected by the rhizome morphology (higher rhizome length but lower width and biomass) linked to a higher temperature environment with higher MHWs frequency. Loss of rhizome biomass and length could negatively affect the rhizome carbon storage (Libes and Boudouresque, 1987; Peirano et al., 2011) and the resistance of the meadow to future stress as storms, a disturbance whose frequency and intensity is predicted to increase with climate change (Easterling et al., 2000; Beniston et al., 2007).
The results have also highlighted that P. oceanica resilience to future warming conditions is likely threatened by the increase in frequency and duration of MHWs. However, with this study it was not possible to investigate the interactive effect between MHW intensity and duration, thus further investigations are necessary to disentangle their potential synergistic effects. According to forecasted conditions, which are depending on the future greenhouse gas emission scenarios, Mediterranean summer MHWs by 2100 will be up to three months longer, about four times more intense and 42 times more severe than present-day events (Darmaraki et al., 2019b). The increasing frequency of MHWs over the coming decades is correlated to decreased ecological status of foundation species (Smale et al., 2019; Strydom et al., 2020). From our reconstruction, we could not deduce the importance of the summer waves in relation to those of the other seasons and therefore it cannot be assumed that the greatest concern should be addressed to the summer heat waves: furthermore, specific seasonal adaptations of the seagrass to each season due to morphological and biochemical variations (Alcoverro et al., 1995; Beca-Carrettero et al., 2021; Pazzaglia et al., 2022) could eventually modulate the response of the plant to conditions in different periods. To the best of our knowledge, this is the first time a similar technique is used to evaluate the effects of past MHWs on the shoot morphology and productivity of a seagrass, even though reconstruction techniques associated to the temperature throughout each year have already been used in several terrestrial plants (e.g., dendroclimatology, Fritts, 1971; Hughes et al., 2010). This is possible especially in P. oceanica where the number of annual cycles that can be observed along the rhizomes can be up to 50 (350 sheaths, Pergent, 1987; Pergent et al., 2004), while in other species of Posidonia the decomposition of the sheaths is faster and reconstructions of only five years are feasible (Pergent et al., 2004). Therefore, our study highlights the effectiveness of lepidochronology in P. oceanica to access biological archives, useful to also estimate the thermal environment effects in which the plant developed, and the effects of local stressors (Guidetti, 2001; Balestri et al., 2004). Overall, our results support the call to give more importance to the effects of temperature positive anomalies on several plant variables in order to understand the mechanisms involved in the dramatic shifts in meadow structure during MHW occurrence. This will enable the forecasting of the vulnerability of P. oceanica assisting thus, management actions such as seagrass restoration.
The original contributions presented in the study are included in the article/Supplementary Material. Further inquiries can be directed to the corresponding author.
PS and GC conceived the ideas and designed methodology, PS, EA, NC, PE, CJ, AP, EP, VR collected the data, GLM analysed the data, PS and GC led the writing of the manuscript. All authors contributed to the article and approved the submitted version.
The study has been funded by Italian Ministry of Education and Research PRIN 2017 (MHHWBN) “Marine Habitats restoration in a climate change-impaired Mediterranean Sea” (MAHRES) and by PON – National Operational Programme - Research and Innovation 2014-2020– PhDs and research contracts on innovation-related topics.
We would like to thank the Department of Fisheries and Marine Research (DFMR) and the Department of Environment of Cyprus for giving us on time, all the appropriate permits to study Posidonia oceanica meadows in Cyprus. We also sincerely thank Daniel and Tamara from Cyprus Diving Center, George from Blue Instinct, Dino from Karpathos Diving Center and Simone from Pelagos 2.0 Lampedusa for helping and supporting us in the field. Furthermore, we would like to thank Josh, Valentina and Marie for helping in processing some of the plant material, and Marios Papageorgiou from Enalia Physis Environmental Research Center for the logistics in Cyprus.
The authors declare that the research was conducted in the absence of any commercial or financial relationships that could be construed as a potential conflict of interest.
All claims expressed in this article are solely those of the authors and do not necessarily represent those of their affiliated organizations, or those of the publisher, the editors and the reviewers. Any product that may be evaluated in this article, or claim that may be made by its manufacturer, is not guaranteed or endorsed by the publisher.
The Supplementary Material for this article can be found online at: https://www.frontiersin.org/articles/10.3389/fmars.2022.895354/full#supplementary-material
Alcoverro T., Duarte C. M., Romero J. (1995). Annual growth dynamics of Posidonia oceanica: contribution of large-scale versus local factors to seasonality. Mar. Ecol. Prog. Ser., 203–210. doi: 10.3354/meps120203
Aoki L. R., McGlathery K. J., Wiberg P. L., Oreska M. P., Berger A. C., Berg P., et al. (2021). Seagrass recovery following marine heat wave influences sediment carbon stocks. Front. Mar. Sci. 7, 1170. doi: 10.3389/fmars.2020.576784
Balestri E., Benedetti-Cecchi L., Lardicci C. (2004). Variability in patterns of growth and morphology of Posidonia oceanica exposed to urban and industrial wastes: contrasts with two reference locations. J. Exp. Mar. Biol. Ecol. 308 (1), 1–21. doi: 10.1016/j.jembe.2004.01.015
Beca-Carretero P., Azcárate-García T., Julia-Miralles M., Stanschewski C. S., Guihéneuf F., Stengel D. B. (2021). Seasonal acclimation modulates the impacts of simulated warming and light reduction on temperate seagrass productivity and biochemical composition. Front. Mar. Sci. 1261. doi: 10.3389/fmars.2021.731152
Beca-Carretero P., Guihéneuf F., Krause-Jensen D., Stengel D. B. (2020). Seagrass fatty acid profiles as a sensitive indicator of climate settings across seasons and latitudes. Mar. Environ. Res. 161, 105075. doi: 10.1016/j.marenvres.2020.105075
Beniston M., Stephenson D. B., Christensen O. B., Ferro C. A., Frei C., Goyette S., et al. (2007). Future extreme events in European climate: an exploration of regional climate model projections. Climatic. Change 81 (1), 71–95. doi: 10.1007/s10584-006-9226-z
Bennett S., Alcoverro T., Kletou D., Antoniou C., Boada J., Buñuel X., et al (2022). Resilience of seagrass populations to thermal stress does not reflect regional differences in ocean climate. New Phytol 233 (4), 1657–66. doi: 10.1111/nph.17885
Bennett S., Marba N., Jorda G., Vaquer-Sunyer R., Forteza M., Roca G. (2021). Thermal performance of seaweeds and seagrasses across a regional climate gradient. Front. Mar. Sci. 61. doi: 10.3389/fmars.2022.733315
Bethoux J. P., Gentili B. (1999). Functioning of the Mediterranean Sea: past and present changes related to freshwater input and climate changes. J. Mar. Syst. 20 (1-4), 33–47. doi: 10.1016/S0924-7963(98)00069-4
Bianchi C. N., Morri C., Chiantore M., Montefalcone M., Parravicini V., Rovere A. (2012). Mediterranean Sea Biodiversity between the legacy from the past and a future of change. Life Mediterr. Sea.: look. at. habitat. changes. 1, 55.
Burgos E., Montefalcone M., Ferrari M., Paoli C., Vassallo P., Morri C., et al. (2017). Ecosystem functions and economic wealth: Trajectories of change in seagrass meadows. J. cleaner. production. 168, 1108–1119. doi: 10.1016/j.jclepro.2017.09.046
Carmen B., Brun F. G., Onoda Y., Cambridge M. L., Bouma T. J., Vergara J. J., et al. (2012). Leaf-fracture properties correlated with nutritional traits in nine Australian seagrass species: implications for susceptibility to herbivory. Mar. Ecol. Prog. Ser. 458, 89–102. doi: 10.3354/meps09757
Chefaoui R. M., Duarte C. M., Serrão E. A. (2018). Dramatic loss of seagrass habitat under projected climate change in the Mediterranean Sea. Global Change Biol. 24 (10), 4919–4928. doi: 10.1111/gcb.14401
Collier C. J., Ow Y. X., Langlois L., Uthicke S., Johansson C. L., O'Brien K. R., et al. (2017). Optimum temperatures for net primary productivity of three tropical seagrass species. Front. Plant Sci. 8, 1446. doi: 10.3389/fpls.2017.01446
Cook J., Oreskes N., Doran P. T., Anderegg W. R., Verheggen B., Maibach E. W., et al (2016). Consensus on consensus: a synthesis of consensus estimates on human-caused global warming. Environ. Res. Lett. 11 (4), 048002. doi: 10.1088/1748-9326/11/4/048002
Core Team, R. C. T. R (2013). R: A language and environment for statistical computing (Vienna: R Foundation for statistical computing).
Coumou D., Rahmstorf S. (2012). A decade of weather extremes. Nat. Climate Change 2, 491–496. doi: 10.1038/nclimate1452
Darmaraki S., Somot S., Sevault F., Nabat P. (2019a). Past variability of Mediterranean Sea marine heatwaves. Geophys. Res. Lett. 46, 9813–9823. doi: 10.1029/2019GL082933
Darmaraki S., Somot S., Sevault F., Nabat P., Narvaez W. D. C., Cavicchia L., et al. (2019b). Future evolution of marine heatwaves in the Mediterranean Sea. Climate Dynamics. 53 (3), 1371–1392. doi: 10.1007/s00382-019-04661-z
De Frenne P., Graae B. J., Rodríguez-Sánchez F., Kolb A., Chabrerie O., Decocq G., et al. (2013). Latitudinal gradients as natural laboratories to infer species' responses to temperature. J. Ecol. 101 (3), 784–795. doi: 10.1111/1365-2745.12074
de Los Santos C. B., Krause-Jensen D., Alcoverro T., Marbà N., Duarte C. M., Van Katwijk M. M., et al. (2019). Recent trend reversal for declining European seagrass meadows. Nat. Commun. 10 (1), 1–8. doi: 10.1038/s41467-019-11340-4
Diffenbaugh N. S., Pal J. S., Giorgi F., Gao X. (2007). Heat stress intensification in the Mediterranean climate change hotspot. Geophys. Res. Lett. 34 (11), L11706. doi: 10.1029/2007GL030000
Duffy J. E. (2006). Biodiversity and the functioning of seagrass ecosystems. Mar. Ecol. Prog. Ser. 311, 233–250. doi: 10.3354/meps311233
Dunic J. C., Brown C. J., Connolly R. M., Turschwell M. P., Côté I. M. (2021). Long-term declines and recovery of meadow area across the world’s seagrass bioregions. Global Change Biol. 27 (17), 4096–4109. doi: 10.1111/gcb.15684
Easterling D. R., Evans J. L., Groisman P. Y., Karl T. R., Kunkel K. E., Ambenje P. (2000). Observed variability and trends in extreme climate events: a brief review. Bull. Am. Meteorol. Soc. 81 (3), 417–426. doi: 10.1175/1520-0477(2000)081<0417:OVATIE>2.3.CO;2
Fraser M. W., Kendrick G. A., Statton J., Hovey R. K., Zavala-Perez A., Walker D. I. (2014). Extreme climate events lower resilience of foundation seagrass at edge of biogeographical range. J. Ecol. 102 (6), 1528–1536. doi: 10.1111/1365-2745.12300
Fritts H. C. (1971). Dendroclimatology and dendroecology. Quaternary. Res. 1 (4), 419–449. doi: 10.1016/0033-5894(71)90057-3
Frölicher T. L., Laufkötter C. (2018). Emerging risks from marine heat waves. Nat. Commun. 9 (1), 1–4. doi: 10.1038/s41467-018-03163-6
Garrabou J., Coma R., Bensoussan N., Bally M., Chevaldonné P., Cigliano M., et al. (2009). Mass mortality in northwestern Mediterranean rocky benthic communities: effects of the 2003 heat wave. Global Change Biol. 15 (5), 1090–1103. doi: 10.1111/j.1365-2486.2008.01823.x
Giorgi F., Lionello P. (2008). Climate change projections for the Mediterranean region. Global planet. Change 63 (2-3), 90–104. doi: 10.1016/j.gloplacha.2007.09.005
Giraud G. (1977). “Contribution à la description et a la phenologie quantitative des herbiers de posidonia oceanica (L.) delile,” in Doctorat de spécialité en oceanologie (Marseille: Université Aix-Marseille II, Fac. Des Sciences de Lumy), 1–50.
Guerrero-Meseguer L., Marín A., Sanz-Lázaro C. (2017). Future heat waves due to climate change threaten the survival of Posidonia oceanica seedlings. Environ. pollut. 230, 40–45. doi: 10.1016/j.envpol.2017.06.039
Guidetti P. (2001). Detecting environmental impacts on the Mediterranean seagrass Posidonia oceanica (L.) delile: the use of reconstructive methods in combination with ‘beyond BACI’designs. J. Exp. Mar. Biol. Ecol. 260 (1), 27–39. doi: 10.1016/S0022-0981(01)00245-3
Hobday A. J., Alexander L. V., Perkins S. E., Smale D. A., Straub S. C., Oliver E. C., et al. (2016). A hierarchical approach to defining marine heatwaves. Prog. Oceanogr. 141, 227–238. doi: 10.1016/j.pocean.2015.12.014
Hoegh-Guldberg O., Bruno J. F. (2010). The impact of climate change on the world’s marine ecosystems. Science 328 (5985), 1523–1528. doi: 10.1126/science.118993
Holon F., Boissery P., Guilbert A., Freschet E., Deter J. (2015). The impact of 85 years of coastal development on shallow seagrass beds (Posidonia oceanica L.(Delile)) in south Eastern France: A slow but steady loss without recovery. Estuar. Coast. Shelf. Sci. 165, 204–212. doi: 10.1016/j.ecss.2015.05.017
Hughes M. K., Swetnam T. W., Diaz H. F. (Eds.) (2010). Dendroclimatology: progress and prospects (Vol. 11) (Berlin: Springer Science & Business Media).
Jackson S. D. (2009). Plant responses to photoperiod. New Phytol. 181 (3), 517–531. doi: 10.1111/j.1469-8137.2008.02681.x
Jordà G., Marbà N., Duarte C. M. (2012). Mediterranean Seagrass vulnerable to regional climate warming. Nat. Climate Change 2 (11), 821–824. doi: 10.1038/nclimate1533
Kletou D., Kleitou P., Savva I., Attrill M. J., Charalambous S., Loucaides A., et al. (2020). Seagrass of vasiliko bay, Eastern Mediterranean: Lost cause or priority conservation habitat? J. Mar. Sci. Eng. 8 (9), 717. doi: 10.3390/jmse8090717
Lefcheck J. S., Wilcox D. J., Murphy R. R., Marion S. R., Orth R. J. (2017). Multiple stressors threaten the imperiled coastal foundation species eelgrass (Zostera marina) in Chesapeake bay, USA. Global Change Biol. 23 (9), 3474–3483. doi: 10.1111/gcb.13623
Libes M., Boudouresque C. F. (1987). Uptake and long-distance transport of carbon in the marine phanerogam posidonia oceanica. Mar. Ecol. Prog. Ser., 177–186. doi: 10.3354/meps038177
Llagostera I., Cervantes D., Sanmartí N., Romero J., Pérez M. (2016). Effects of copper exposure on photosynthesis and growth of the seagrass cymodocea nodosa: an experimental assessment. Bull. Environ. contamination. Toxicol. 97 (3), 374–379. doi: 10.1007/s00128-016-1863-y
Marbà N., Duarte C. M. (2010). Mediterranean Warming triggers seagrass (Posidonia oceanica) shoot mortality. Global Change Biol. 16 (8), 2366–2375. doi: 10.1111/j.1365-2486.2009.02130.x
Marín-Guirao L., Bernardeau-Esteller J., García-Muñoz R., Ramos A., Ontoria Y., Romero J., et al. (2018). Carbon economy of Mediterranean seagrasses in response to thermal stress. Mar. pollut. Bull. 135, 617–629. doi: 10.1016/j.marpolbul.2018.07.050
Marín-Guirao L., Entrambasaguas L., Ruiz J. M., Procaccini G. (2019). Heat-stress induced flowering can be a potential adaptive response to ocean warming for the iconic seagrass posidonia oceanica. Mol. Ecol. 28 (10), 2486–2501. doi: 10.1111/mec.15089
Marín-Guirao L., Ruiz J. M., Dattolo E., Garcia-Munoz R., Procaccini G. (2016). Physiological and molecular evidence of differential short-term heat tolerance in Mediterranean seagrasses. Sci. Rep. 6 (1), 1–13. doi: 10.1038/srep28615
Masini R. J., Anderson P. K., McComb A. J. (2001). A halodule-dominated community in a subtropical embayment: physical environment, productivity, biomass, and impact of dugong grazing. Aquat. Bot. 71 (3), 179–197. doi: 10.1016/S0304-3770(01)00181-4
Montefalcone M. (2009). Ecosystem health assessment using the Mediterranean seagrass Posidonia oceanica: a review. Ecol. Indic. 9 (4), 595–604. doi: 10.1016/j.ecolind.2008.09.013
Nguyen H. M., Ralph P. J., Marín-Guirao L., Pernice M., Procaccini G. (2021). Seagrasses in an era of ocean warming: a review. Biol. Rev 96 (5), 2009–30. doi: 10.1111/brv.12736
Nowicki R., Heithaus M., Thomson J., Burkholder D., Gastrich K., Wirsing A. (2019). Indirect legacy effects of an extreme climatic event on a marine megafaunal community. Ecol. Monogr. 89 (3), e01365. doi: 10.1002/ecm.1365
Nykjaer L. (2009). Mediterranean Sea Surface warming 1985–2006. Climate Res. 39 (1), 11–17. doi: 10.3354/cr00794
Olesen B., Enríquez S., Duarte C. M., Sand-Jensen K. (2002). Depth-acclimation of photosynthesis, morphology and demography of posidonia oceanica and cymodocea nodosa in the Spanish Mediterranean Sea. Mar. Ecol. Prog. Ser. 236, 89–97. doi: 10.3354/meps236089
Oliver E. C., Donat M. G., Burrows M. T., Moore P. J., Smale D. A., Alexander L. V., et al. (2018). Longer and more frequent marine heatwaves over the past century. Nat. Commun. 9 (1), 1–12. doi: 10.1038/s41467-018-03732-9
Orth R. J., Carruthers T. J., Dennison W. C., Duarte C. M., Fourqurean J. W., Heck K. L., et al. (2006). A global crisis for seagrass ecosystems. Bioscience 56 (12), 987–996. doi: 10.1641/0006-3568(2006)56[987:AGCFSE]2.0.CO;2
Pagès J. F., Pérez M., Romero J. (2010). Sensitivity of the seagrass cymodocea nodosa to hypersaline conditions: a microcosm approach. J. Exp. Mar. Biol. Ecol. 386 (1-2), 34–38. doi: 10.1016/j.jembe.2010.02.017
Pansini A., La Manna G., Pinna F., Stipcich P., Ceccherelli G. (2021). Trait gradients inform predictions of seagrass meadows changes to future warming. Sci. Rep. 11 (1), 1–12. doi: 10.1038/s41598-021-97611-x
Pazzaglia J., Badalamenti F., Bernardeau-Esteller J., Ruiz J. M., Giacalone V. M., Procaccini G., et al. (2022). Thermo-priming increases heat-stress tolerance in seedlings of the Mediterranean seagrass p. oceanica. Mar. pollut. Bull. 174, 113164. doi: 10.1016/j.marpolbul.2021.113164
Pazzaglia J., Nguyen H. M., Santillán-Sarmiento A., Ruocco M., Dattolo E., Marín-Guirao L., et al. (2021). The genetic component of seagrass restoration: What we know and the way forwards. Water 13 (6), 829. doi: 10.3390/w13060829
Peirano A., Cocito S., Banfi V., Cupido R., Damasso V., Farina G., et al. (2011). Phenology of the Mediterranean seagrass Posidonia oceanica (L.) delile: Medium and long-term cycles and climate inferences. Aquat. Bot. 94 (2), 77–92. doi: 10.1016/j.aquabot.2010.11.007
Pergent G. (1987). Recherches lepidochronologiques chez posidonia oceanica (Potamogetonaceae). fluctuations des parame'tres anatomiques et morphologiques des ecailles des rhizomes. These. Doct. Oceanol.
Pergent-Martini C., Pergent G. (1996). Lepidochronological analysis in the Mediterranean seagrass posidonia oceanica: state of the art and future developments. Oceanogr. Literature. Rev. 2 (43), 193.
Pergent-Martini C., Rico-Raimondino V., Pergent G. (1994). Lepidochronological analysis in the mediterranean seagrass posidonia-oceanica state-of-the-art and future-developments. Oceanologica acta 17 (6), 673–81.
Pergent G., Pergent-Martini C. (1991). Leaf renewal cycle and primary production of Posidonia oceanica in the bay of lacco ameno (Ischia, Italy) using lepidochronological analysis. Aquat. Bot. 42 (1), 49–66. doi: 10.1016/0304-3770(91)90105-E
Pergent G., Pergent-Martini C., Bein A., Dedeken M., Oberti P., Orsini A., et al. (2015). Dynamic of posidonia oceanica seagrass meadows in the northwestern Mediterranean: Could climate change be to blame? Comptes. rendus. biologies. 338 (7), 484–493. doi: 10.1016/j.crvi.2015.04.011
Pergent G., Pergent-Martini C., Boudouresque C. F. (1995). Utilisation de l'herbier à posidonia oceanica comme indicateur biologique de la qualité du milieu littoral en méditerranée: état des connaissances. Mésogée. (Marseille). 54, 3–27.
Pergent G., Pergent-Martini C., Fernandez C., Pasqualini V., Walker D. (2004). Morpho-chronological variations and primary production in posidonia sea grass from Western Australia. J. Mar. Biol. Assoc. United. Kingdom. 84 (5), 895–899. doi: 10.1017/S0025315404010161h
Piazzi L., Bonaviri C., Castelli A., Ceccherelli G., Costa G., Curini-Galletti M., et al. (2018). Biodiversity in canopy-forming algae: structure and spatial variability of the Mediterranean cystoseira assemblages. Estuar. Coast. Shelf. Sci. 207, 132–141. doi: 10.1016/j.ecss.2018.04.001
Ralph P. J., Durako M. J., Enriquez S., Collier C. J., Doblin M. A. (2007). Impact of light limitation on seagrasses. J. Exp. Mar. Biol. Ecol. 350 (1-2), 176–193. doi: 10.1016/j.jembe.2007.06.017
Ruddiman W. F. (2003). The anthropogenic greenhouse era began thousands of years ago. Climatic. Change 61 (3), 261–293. doi: 10.1023/B:CLIM.0000004577.17928.fa
Ruocco M., Marín-Guirao L., Ravaglioli C., Bulleri F., Procaccini G. (2018). Molecular level responses to chronic versus pulse nutrient loading in the seagrass Posidonia oceanica undergoing herbivore pressure. Oecologia 188 (1), 23–39. doi: 10.1007/s00442-018-4172-9
Schlegel R. W., Smit A. J. (2018). heatwaveR: A central algorithm for the detection of heatwaves and cold-spells. J. Open Source Software 3 (27), 821. doi: 10.21105/joss.00821
Scott C. (2021). rerddap: General Purpose Client for 'ERDDAP' Servers. R package version 0.7.6. Available at: https://CRAN.R-project.org/package=rerddap.
Shields E. C., Parrish D., Moore K. (2019). Short-term temperature stress results in seagrass community shift in a temperate estuary. Estuar. Coasts. 42 (3), 755–764. doi: 10.1007/s12237-019-00517-1
Smale D. A., Wernberg T., Oliver E. C., Thomsen M., Harvey B. P., Straub S. C., et al. (2019). Marine heatwaves threaten global biodiversity and the provision of ecosystem services. Nat. Climate Change 9 (4), 306–312. doi: 10.1038/s41558-019-0412-1
Soto-Navarro J., Jordá G., Amores A., Cabos W., Somot S., Sevault F., et al. (2020). Evolution of Mediterranean Sea water properties under climate change scenarios in the med-CORDEX ensemble. Climate Dynamics. 54 (3), 2135–2165. doi: 10.1007/s00382-019-05105-4
Stipcich P., Marín-Guirao L., Pansini A., Pinna F., Procaccini G., Pusceddu A., et al. (2022). Effects of current and future summer marine heat waves on posidonia oceanica: Plant origin matters? Front. Climate 4, 844831. doi: 10.3389/fclim.2022.844831
Strydom S., Murray K., Wilson S., Huntley B., Rule M., Heithaus M., et al. (2020). Too hot to handle: unprecedented seagrass death driven by marine heatwave in a world heritage area. Global Change Biol. 26 (6), 3525–3538. doi: 10.1111/gcb.15065
Telesca L., Belluscio A., Criscoli A., Ardizzone G., Apostolaki E. T., Fraschetti S., et al. (2015). Seagrass meadows (Posidonia oceanica) distribution and trajectories of change. Sci. Rep. 5 (1), 1–14. doi: 10.1038/srep12505
Tryjanowski P., Panek M., Sparks T. (2006). Phenological response of plants to temperature varies at the same latitude: case study of dog violet and horse chestnut in England and Poland. Climate Res. 32 (1), 89–93. doi: 10.3354/cr032089
Turschwell M. P., Connolly R. M., Dunic J. C., Sievers M., Buelow C. A., Pearson R. M., et al. (2021). Anthropogenic pressures and life history predict trajectories of seagrass meadow extent at a global scale. Proc. Natl. Acad. Sci. 118 (45), e2110802118. doi: 10.1073/pnas.2110802118
Waycott M., Duarte C. M., Carruthers T. J., Orth R. J., Dennison W. C., Olyarnik S., et al. (2009). Accelerating loss of seagrasses across the globe threatens coastal ecosystems. Proc. Natl. Acad. Sci. 106 (30), 12377–12381. doi: 10.1073/pnas.0905620106
Wernberg T., Smale D. A., Tuya F., Thomsen M. S., Langlois T. J., De Bettignies T., et al. (2013). An extreme climatic event alters marine ecosystem structure in a global biodiversity hotspot. Nat. Climate Change 3 (1), 78–82. doi: 10.1038/nclimate1627
Wood S. N. (2006). Generalized additive models: an introduction with r. chapman and hall/CRC. doi: 10.1201/9781420010404
Zavatarielli M., Mellor G. L. (1995). A numerical study of the Mediterranean Sea circulation. J. Phys. Oceanogr. 25 (6), 1384–1414. doi: 10.1175/1520-0485(1995)025<1384:ANSOTM>2.0.CO;2
Zuur A. F. (2012). A beginner's guide to generalized additive models with r (Newburgh, NY, USA: Highland Statistics Limited), 1–206.
Keywords: climate change, lepidochronological analysis, marine heat waves, reconstruction, shoot morphology, warming
Citation: Stipcich P, Apostolaki ET, Chartosia N, Efthymiadis PT, Jimenez CE, La Manna G, Pansini A, Principato E, Resaikos V and Ceccherelli G (2022) Assessment of Posidonia oceanica traits along a temperature gradient in the Mediterranean Sea shows impacts of marine warming and heat waves. Front. Mar. Sci. 9:895354. doi: 10.3389/fmars.2022.895354
Received: 13 March 2022; Accepted: 26 September 2022;
Published: 10 October 2022.
Edited by:
João Canning-Clode, Center for Marine and Environmental Sciences (MARE), PortugalReviewed by:
Gilles Lepoint, Fonds National de la Recherche Scientifique (FNRS), BelgiumCopyright © 2022 Stipcich, Apostolaki, Chartosia, Efthymiadis, Jimenez, La Manna, Pansini, Principato, Resaikos and Ceccherelli. This is an open-access article distributed under the terms of the Creative Commons Attribution License (CC BY). The use, distribution or reproduction in other forums is permitted, provided the original author(s) and the copyright owner(s) are credited and that the original publication in this journal is cited, in accordance with accepted academic practice. No use, distribution or reproduction is permitted which does not comply with these terms.
*Correspondence: Patrizia Stipcich, cGF0cml6aWFzdGlwY2ljaEBsaWJlcm8uaXQ=
Disclaimer: All claims expressed in this article are solely those of the authors and do not necessarily represent those of their affiliated organizations, or those of the publisher, the editors and the reviewers. Any product that may be evaluated in this article or claim that may be made by its manufacturer is not guaranteed or endorsed by the publisher.
Research integrity at Frontiers
Learn more about the work of our research integrity team to safeguard the quality of each article we publish.