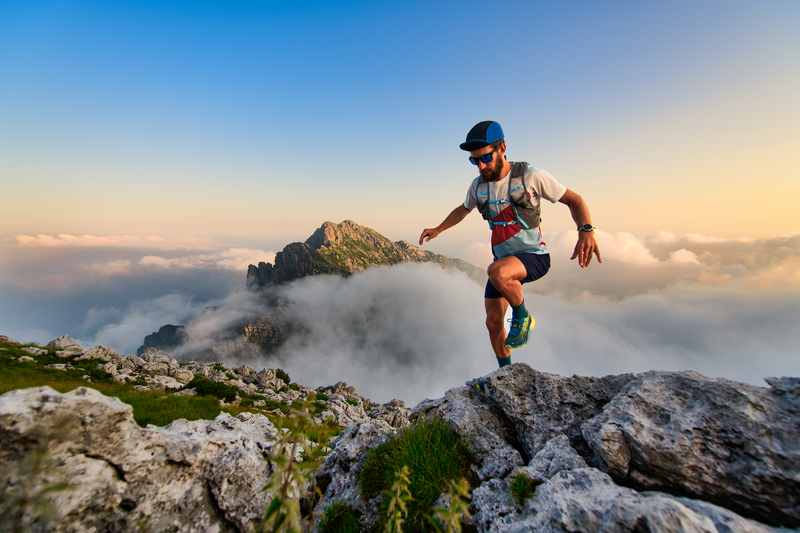
95% of researchers rate our articles as excellent or good
Learn more about the work of our research integrity team to safeguard the quality of each article we publish.
Find out more
ORIGINAL RESEARCH article
Front. Mar. Sci. , 29 July 2022
Sec. Marine Ecosystem Ecology
Volume 9 - 2022 | https://doi.org/10.3389/fmars.2022.892274
This article is part of the Research Topic The Effects of Environmental Change on Anchialine Ecosystems View all 5 articles
The anchialine environment is characterized by a vertical stratification of water masses with different salinities. Cave shrimps of the genus Typhlatya are widespread inhabitants of the aquifer in fresh, brackish, and marine groundwater. Here we describe physiological aspects of three of the most abundant and widespread Typhlatya species that thrive in the fresh and marine groundwater habitats of the anchialine ecosystem of the Yucatan Peninsula. The aerobic scope (AS) of Typhlatya mitchelli, Typhlatya pearsei and Typhlatya dzilamensis was estimated through induced physical activity, whilst monitoring protein carbonylation and lipid peroxidation (as indicators of cellular damage), lactate accumulation (as an indicator of anaerobic metabolism) and the antioxidant system response. The critical thermal limits (CTL) of all three species as an additional measure of physiological plasticity were also determined. Our results showed that metabolic rates, AS and CTL were similar amongst the two species that inhabit fresh groundwater habitats, and differed markedly from T. dzilamensis, a species typically found in marine groundwater. The antioxidant system response in all three Typhlatya species accompanied the levels of aerobic metabolism following physical activity. However, the large amount of GSH observed in T. dzilamensis may be indicative of an adaptive trait to a more heterogeneous environment. The differences observed among Typhlatya species reflect different physiological adaptations that correspond to the environmental heterogeneity of their natural habitats. Our results suggest that the marine groundwater species, T. dzilamensis, could be better prepared to respond to a naturally more heterogeneous environment, in contrast to Typhlatya mitchelli and Typhlatya pearsei which rarely face environmental clines in the fresh groundwater habitat. Our findings contribute to a better understanding of the consequences of environmental change on ecologically important species that are restricted to live in the aquifer.
Species restricted to living in flooded caves (stygofauna) possess adaptations, known as troglomorphisms, that distinguish them from aquatic species living in surface water bodies (Christiansen, 1962; Pipan and Culver, 2012). Morphological differences, such as the reduction or loss of eyes and pigments, and extension of appendages are some of the most obvious. However, physiological traits of stygobionts in response to the distinct conditions of anchialine caves are determinant for the persistence of their populations. In the adaptation process, individuals in a population respond with a set of behavioral, physiological, biochemical, and metabolic mechanisms to environmental change. This responsiveness to different stimuli can be considered phenotypic plasticity, which can be fixed in a population through natural selection over evolutionary time scales, ultimately resulting in a population with greater fitness to the novel environment (Pigliucci et al., 2006; Norin and Metcalfe, 2019). The study of physiological plasticity and its relation to the adaptive capacity of species to their environment has allowed to establish tolerance limits to different environmental factors, such as temperature, oxygen or salinity (Guderley and Pörtner, 2010; Pörtner, 2010; Sokolova et al., 2012).
The response to environmental change, whether of biotic or abiotic origin, necessarily involves changes in energy production and allocation (Sokolova et al., 2012). Metabolism is the process that produces energy (ATP) at the cellular level, and the metabolic rate (the speed at which it is produced) measures the amount of energy required (and supplied) by the organism. The energy required for the maintenance of essential functions, such as respiration, blood circulation, and excretion, is known as the basal metabolic rate (BMR). Due to methodological difficulties in measuring BMR, the standard metabolic rate (SMR) has been used as the practical measure of BMR (Chabot et al., 2016a).
Once the energy requirements for the maintenance of homeostasis have been met, organisms can generate a surplus of energy for growth, reproduction, food digestion, physical activity, and response to environmental variations (Pörtner and Farrell, 2008; Sokolova et al., 2012). One way to assess that capacity is by measuring the maximum metabolic rate (MMR), which has been recognized as the physiological limit at which an individual can produce ATP via the aerobic pathway. The difference between the MMR and SMR was first defined as the aerobic scope of metabolic activity (Fry, 1947) and is now called the aerobic scope (AS). The AS is the energy that an individual can produce beyond the metabolic cost of basal processes and is intended to perform ecological functions (Sokolova et al., 2012; Norin and Metcalfe, 2019). Since basal maintenance always prioritizes energy resource allocation over any other process, when energy production is limited or the cost of basal maintenance increases, AS is reduced (Sokolova et al., 2012). Therefore, higher AS has been linked to greater fitness, both in long-term processes, such as adaptive potential (Farrell et al., 2008; Schulte 2015; Norin and Metcalfe, 2019), growth and reproduction (Meza-Buendía et al., 2021), as well as in the short-term processes, such as predator evasion, foraging and response to immediate environmental disturbances (Pörtner and Knust, 2007; Pörtner and Farrell, 2008; Clark et al., 2013). Likewise, changes in AS may distinguish an optimal situation from one of moderate or even critical stress (Pörtner, 2002; Pörtner, 2010; Sokolova et al., 2012; Pörtner et al., 2017).
One way to quantify stress is by assessing the energetic-metabolic costs that environmental conditions produce in an organism (Pörtner, 2010; Lushchak, 2011; Sokolova et al., 2012; Paschke et al., 2018). It is possible to establish how environmental changes modulate the physiological mechanisms that enable the response of individuals to the environment (Sokolova et al., 2012). This is how the study of metabolism, and its response to environmental change allows the evaluation of the capacity to obtain energy under a variety of stress conditions (Sokolova et al., 2012; Paschke et al., 2018). Moreover, physiological response to environmental heterogeneity contributes to the definition of some dimensions of the fundamental ecological niche (Hutchinson, 1957; Elton and Charles, 2001; Soberón and Peterson, 2005; Ángeles-González et al., 2020; Ángeles-González et al., 2021).
In order to increase aerobic metabolism, an increase of oxygen influx into the mitochondria is needed. During this process, the monovalent reduction of oxygen generates a series of free radicals (reactive oxygen species, ROS), which can be harmful to organisms as they oxidize other molecules to reach ionic equilibrium (Martínez-Álvarez et al., 2005). Oxidative stress occurs when the production of ROS is greater than the capacity of the antioxidant system to eliminate them (Martínez-Álvarez et al., 2005; Díaz-Acosta and Membrillo-Hernández, 2006). Organisms rely on a series of oxidation-reduction reactions (the antioxidant system, AOS) that continuously maintain cells in a highly dynamic equilibrium to prevent cellular damage from ROS. Antioxidants yield electrons to ROS, transforming compounds into less reactive or detoxified molecules (Regoli et al., 2011; Gumulec et al., 2013). An increase in the concentration of antioxidants such as superoxide dismutase (SOD), Catalase (CAT), Glutathione (GSH) and Glutathione S-transferase (GST) indicate an increase in ROS production, whereas lipid peroxidation (LPO) and protein carbonylation (PO) are biomarkers of oxidative stress showing cellular damage.
Typhlatya mitchelli, Typhlatya pearsei and Typhlatya dzilamensis occupy distinct habitats in the cenotes (local term for dolines/sinkholes) and caves of the Yucatan Peninsula (Chávez-Solís et al., 2020). These anchialine habitats are characterized by vertically stratified water bodies, where temperature exhibits slight variations (~1°C) across this gradient, but salinity may change abruptly with depth, frequently accompanied by changes in dissolved oxygen, oxidation-reduction (redox) potential and pH (Pohlman, 2011; Brankovits et al., 2017; Benítez et al., 2019). Such systems, therefore, present unique abiotic characteristics that generally keep fresh and marine groundwater species spatially separated. Here, T. mitchelli, and T. pearsei inhabit strictly freshwater environments, while T. dzilamensis inhabit environments with higher salinity levels (Espinasa et al., 2019; Chávez-Solís et al., 2020).
The thermal window, the metabolic capacities and the antioxidant mechanism have been used to understand how the environment can influence the adaptive capacity of aquatic organisms (Vinagre et al., 2016; Vinagre et al., 2021). These findings have been key to understanding species’ adaptation from an eco-physiological point of view (Niklitschek and Secor, 2009; Paschke et al., 2013; Sumaila et al., 2020; Eddy et al., 2021) and help to predict changes in future warming scenarios (Cheung et al., 2008; Cheung et al., 2010; Maharaj et al., 2018). If there is a strong association between the physiology of an organism and its habitat (Huey, 1991), it is reasonable to hypothesize that differences in the distribution of these Typhlatya species correspond to certain physiological traits, which were selected by the constraints of past environmental conditions and fixed through evolution. Thus, the comparison of metabolic, behavioral, and biochemical indicators among these closely related species presents a sublime opportunity to evaluate their physiological capacities and discuss them from an adaptive perspective.
This work aimed to characterize the physiology of three Typhlatya species through its thermal window, respiratory metabolism and biochemical indicators reflecting their aerobic capacity under the conditions of their natural habitats in the anchialine ecosystems of the Yucatan Peninsula. For this purpose, in situ experiments to measure thermal window and oxygen consumption rates under routine conditions and in response to swimming activity were carried out. In addition, samples were taken to examine the response of the antioxidant system (enzymes and metabolites indicators of oxidative damage) and anaerobic metabolism derived from the swimming activity at different times throughout the experiments.
Individuals of T. mitchelli, T. pearsei and T. dzilamensis were collected manually using SCUBA cave diving techniques in the Tza Itza (20.730311° N, 89.46608° W), Nohmozón (20.623266° N, 89.384207° W) and Ponderosa system (entering through cenote Xtabay 20.499183° N, 87.260842° W). Tza Itza and Nohmozón are characterized by being totally freshwater (0.64 ± 0.02 ups), while the Ponderosa system is an anchialine, vertically stratified system with freshwater body (3 ups) floating above a marine intrusion (36 ups). The experiments performed in the present study considered that T. mitchelli and T. pearsei are native to the fresh groundwater, while T. dzilamensis is native to the marine groundwater portion of the anchialine ecosystems. T. mitchelli (0.030 ± 0.013 g) were collected from the cavern at Tza Itza in depths ranging from 8 to 14 m; T. pearsei (0.038 ± 0.013 g) were collected at night from the cenote pool at Nohmozon in depths ranging from 8 to 14 m; and T. dzilamensis (0.020 ± 0.011) were collected from the cave at Ponderosa in depths between 13 and 14.5 m. Whilst temperature is relatively constant throughout the water column in Nohmozon and Tza Itza (26.2 and 27.3°C, respectively), the deeper, marine layer in the Ponderosa system is 26°C, just over 1°C warmer than the surface (for further details on these and other physical parameters see Chávez-Solís et al., 2020 and Ballou et al., 2022). In order to keep individuals of all species in conditions similar to their natural habitats, ambient water from the three collection sites was additionally transported to the surface of the cenotes in sealed bags.
Samples were collected in compliance with the permits granted by the Dirección General de Vida Silvestre of the SEMARNAT: SGPA/DGVS/02068/17,/004471/18 and/05996/19. A total of 46, 41, and 45 individuals of T. mitchelli, T. pearsei and T. dzilamensis were captured, respectively, and used in the in situ experiments described herein.
The AS of T. mitchelli, T. pearsei and T. dzilamensis was calculated as the difference between the routine metabolic rate (RMR) and the MMR. The RMR was obtained from individuals maintained in a resting state and without external disturbance, while the MMR was measured in the same individuals after being submitted to physical activity for 5 minutes (Figure 1). All the individuals used to measure metabolic rates were captured directly in 17 ml clear-glass bottles (respirometer chambers) by the cave divers, and placed into a submerged rack where oxygen concentration was measured. The time elapsed from collection to the beginning of oxygen measurements was approximately 15-30 minutes for T. mitchelli and T. pearsei and 20-45 minutes for T. dzilamensis. The temperatures at which RMR and MMR were measured were 27, 26 and 25°C for T. mitchelli, T. pearsei, and T. dzilamensis, respectively. In order to maintain water temperature in the chambers constant, the rack was placed at a depth of 50 cm from the surface. The respiratory rate of each individual was measured in each respirometer chamber by means of an oxygen sensor connected through an external optic fiber to an amplifier (Witrox 4; Loligo systems, Denmark) that sent the signal to a computer. This allowed continuous monitoring at a rate of one record of oxygen concentration per second (see video in Supplementary Material).
Figure 1 Flow chart showing the methods used for the metabolic, biochemical and thermal characterization of T. mitchelli, T. pearsei and T. dzilamensis. The number outside each box indicates the number of individuals (n) used for each experimental test or control. Baseline (BL) individuals are those that did not undergo an experimental treatment. The evaluation of biochemical indicators (BI) includes indicators of the antioxidant system: catalase (CAT), total glutathione (GSH), glutathione S-transferase (GST), and superoxide dismutase (SOD); indicators of oxidative damage: lipid peroxidation (LPO) and protein carbonylation (PO) and lactate (LAC) accumulation by anaerobic metabolism. CT max and CT min are critical thermal maxima and minima, respectively.
The RMR of eight individuals of each species was measured for a period of 150 minutes, during which shrimp remained undisturbed (Figure 1). Following this time, the water in the chambers was totally renewed, and chambers were placed in a system that rotated along its longitudinal axis at 25 rpm for 5 min, thereby inducing animals to physical activity by swimming. Swimming by shrimps was confirmed by direct observation. Immediately after exercise, respirometer chambers were returned to the underwater rack and connected to the optic fiber. MMR was obtained after physical activity from the first 5 minutes of oxygen consumption, nevertheless, these individuals were kept in the same chamber for 120 min, to observe their respiratory behavior (data not shown, Figure 1). Those animals were sampled after 120 min and included in biochemical analysis as T3 group. Oxygen consumption was also measured in control chambers containing only ambient water.
The initial oxygen concentration in the respirometer chambers represented natural conditions, hence varied between species (see Chávez-Solís et al., 2020). Trials with T. mitchelli and T. dzilamensis began with dissolved oxygen concentrations at 3 mg/L, and with T. pearsei at 5 mg/L. In all the cases shrimp remained in the respirometer chambers enough time to register a reduction of dissolved oxygen from 0.7-1 mg/L. To assure that oxygen concentration in the respirometer chamber did not limit the metabolic rate of shrimp, only the slopes of the linear segment of the regressions were considered (i.e. oxygen concentration measurements were taken before oxygen consumption would become dependent of oxygen levels, irrespective of the relative or absolute magnitudes of dissolved oxygen). The duration of measurements was established on the basis of preliminary observations in which a drastic drop in oxygen consumption by T. dzilamensis (n = 5) was observed after 150 minutes of captivity. For the purpose of comparison, the same threshold was used for T. mitchelli and T. pearsei.
The Witrox sensors and computer were installed near the entrance of the cenote and connected to a GoalZero Yeti 1250 portable battery or a Honda gasoline electric generator (Inverter EU 2000i), located outside the cenote gallery at each study site. Once measurements were completed, all individuals were placed in Eppendorf tubes and frozen in liquid nitrogen for further biochemical analysis in the laboratory (Figure 1).
The RMR was calculated as the decreasing slope of the oxygen concentration over at least 500 seconds. Special care was taken to select intervals where reduction was constant, excluding variations attributed to the manipulation of the chamber and sensor adjustments during the first moments of measuring. The MMR was obtained from the slope of the decrease in oxygen concentration for 3 minutes of stable reading within the first 5 minutes immediately after the induced physical activity. Similarly, initial variations caused by sensor adjustment or manipulation of the respirometer chamber were excluded. The metabolic rate of each individual was calculated using the following equation:
Where VO2 is the oxygen consumption of each individual (expressed in mgO2 hr-1 g-1); δPO2 is the slope of oxygen concentration change over time (expressed in mgO2 seg-1); Vr is the volume in the chamber, calculated as the total volume of the chamber (L) minus the weight of each individual (g) (assuming its density was equal to that of water); C is the slope of the reduction in oxygen concentration within the control chamber (mgO2 seg-1); T is the time (hour); and W is the weight of the organism (g).
In order to assess differences in metabolic rates (RMR, MMR) and the magnitude of the AS between species, a MANOVA with permutations was used (Anderson, 2017). Data were log-transformed (log(x+1)) and normalized prior to obtaining a resemblance matrix based on the Euclidean distance between all pairs of samples. A fixed factor (species with three levels) was used as the underlying model, and 9999 unrestricted permutations were used to obtain the empirical distribution of pseudo-F values under the null hypothesis.
The response of the antioxidant system, oxidative damage, and lactate accumulation in the tissues of individuals of the three species of Typhlatya was characterized at different moments throughout a period of recovery after physical activity. Nine individuals of each species were placed in 50 ml Falcon tubes and subjected to the physical activity described previously (Figure 1). Three individuals of each species at each of three post-exercise moments were sampled: 0 (immediately), 30, and 60 minutes after physical activity. These samples, together with 8 additional individuals that were maintained 120 minutes after exercise, constituted the four groups referred to as T0, T1, T2, and T3, respectively. An additional group of 15 individuals of T. mitchelli, 12 of T. pearsei and 16 of T. dzilamensis was placed in liquid nitrogen immediately after collection to establish a baseline (BL) as a reference for all the indicators quantified. Samples of all individuals were frozen in liquid nitrogen and further stored at -80°C until analysis.
The following components of the antioxidant system (AOS) were quantified in each sampled individual: catalase (CAT), glutathione S-transferase (GST), total glutathione (GSH), and superoxide dismutase (SOD). Oxidative damage by reactive oxygen species (ROS) was estimated by quantifying protein carbonylation (PO) and lipid peroxidation (LPO). Finally, lactate (LAC) as a residue of anaerobic metabolism was quantified. For the purposes of this study, the set of indicators of the antioxidant system, oxidative damage, and anaerobic metabolism will be referred to as biochemical indicators (BI).
Each sample was homogenized in a Potter-Elvehjem homogenizer with a PTFE pistil immersed in ice. According to procedures described in Rodríguez-Fuentes et al. (2008), and depending on the weight of each individual, Tris buffer pH 7.4 with a concentration of 0.05M (pre-set crystals pH7.4 of Tris [hydroxymethyl] aminomethane and Tris HCL) was added at a proportion of 1 ml of buffer per 50 mg of wet tissue. PO, LPO and GSH are reported per mg wet tissue. The rest of the homogenate was centrifuged at 10,000 rpm for 5 minutes at 4°C and the supernatant was used for the analysis of the antioxidant enzymes. SOD, CAT and GST activities were calculated per mg of protein.
A Sigma CA0260 kit was used to determine GSH levels. GST activity was obtained following the methods of Ellman et al. (1961) and Habig et al. (1974) using a Sigma CS04 kit with CDNB as the substrate for spectrophotometric measurement at 412 nm every 15 seconds for 5 minutes. CAT activity was determined, using the molybdate method (Góth, 1991) as modified by Hadwan and Abed (2016), by measuring the reduction rate of hydrogen peroxide at 405nm upon reaction with ammonium molybdate. SOD was determined using a Sigma 19160 kit. Total protein was determined using the Bradford method (1976) adapted to microplate with the protocol developed by personnel from the Ecotoxicology laboratory of the Faculty of Chemistry, UNAM, in Sisal, Yucatan, Mexico. PO quantification was based on the methods of Mesquita (2014), while for LPO quantification, the FOX method was followed using the peroxidetect kit (PD1) from Sigma. Finally, LAC was quantified using TRINITY and Pointe Scientific reagents following the procedures indicated by the manufacturer.
All determinations were performed with duplicate subsamples. This allowed negative absorbances to be treated according to the following criteria: 1) when both replicates resulted in negative absorbances, the value was considered as zero, i.e., not detected; 2) when one of the two replicates was negative, only the positive value was considered.
To analyze changes in the AOS and oxidative damage of the three species during the recovery period, a principal coordinate analysis (PCoA) was applied to a resemblance matrix of Euclidean distances between samples. Data were square-root transformed and normalized prior to analysis. A permutational MANOVA with 9999 random permutations of the residuals under the reduced model (Anderson, 2001) was applied on the matrix. A bifactorial model was used with species (3 levels: T. mitchelli, T. pearsei and T. dzilamensis) and time (5 levels: T0, T1, T2, T3 and BL) as fixed factors. A significant interaction would imply that changes in AOS and oxidative damage over time, and relative to the baseline (BL) were different depending on the species of Typhlatya. Likewise, paired comparisons between species centroids for each time would establish whether species returned to a BL condition at different times.
To compare the amount of LAC in the three species before and at different moments after physical activity, a two-factor ANOVA with the same underlying model was applied to the data. Non-significant terms were eliminated sequentially as a selection procedure to obtain the optimum model. Post hoc comparisons between group means were performed using Tukey’s Honest Significant Difference (Tukey´s HSD) (Hothorn et al., 2008).
The procedure for determining critical thermal limits requires exposing individuals to a sufficiently rapid increase in temperature so the physiological mechanisms of thermal adjustments cannot be expressed (Lutterschmidt and Hutchison, 1997b). Following a discussion on critical temperatures in the past decade (Rezende et al., 2011; Terblanche et al., 2011; Mermillod-Blondin et al., 2013), there is consensus that increasing the temperature at the rate of 1°C per minute is appropriate to meet the stipulations of the method (Hutchison, 1961). The critical thermal limit is “the arithmetic mean of the collective thermal points at which locomotor activity becomes disorganized and the animals lose their ability to escape from conditions that will promptly lead to their death, when heated at a constant rate that allows deep body temperatures to follow environmental test temperatures without a significant time lag” (Hutchison, 1961). The definition implies that no damage at the neuronal, physiological or biochemical levels should remain after a brief period at this temperature. The thermal window is the temperature range between the upper and lower critical temperatures (Thyrring et al., 2019), and it indicates the physiological temperature limits that an organism can tolerate and survive, hence delimiting its thermal niche.
The CTmax and CTmin were estimated following the method described by Lutterschmidt and Hutchison (1997a). To obtain the CTmax, specimens of T. mitchelli (n=8), T. pearsei (n=6) and T. dzilamensis (n=5) were placed individually in 50 ml Falcon tubes filled with water from the cenote where each species came from. The tubes were placed in a 20 L glass container filled with water, a CheckTemp®1 digital thermometer (Hanna Instruments), a 1000 W heater, an aerator and a water pump connected to a Honda EU2000i gasoline generator. The volume of water that was needed for the heater to raise the temperature inside the Falcon tubes at a rate of 1°C min-1 was previously calculated and tested again in situ before introducing the individuals. As temperature increased, the behavior of individuals was continuously recorded until the critical temperature was reached. This was defined as the temperature at which an animal showed the onset of spasms or tail flip, followed by loss of movement or constant loss of equilibrium (Lutterschmidt and Hutchison, 1997a; Díaz et al., 2013). Once CTmax was reached, each individual was immediately placed in an Eppendorf, and frozen in liquid nitrogen for the subsequent biochemical characterization.
To obtain the CTmin, specimens of T. mitchelli (n=6), T. pearsei (n=6) and T. dzilamensis (n=5) were also placed individually in 25 ml Falcon tubes within a 12 L glass container similar to the one used previously. The glass container was now placed inside an aluminum box containing a mixture of crushed ice, salt, and alcohol (96°). The water volume within the glass container was also previously calibrated so that temperature would decrease at a rate of 1°C min-1 inside the Falcon tubes. The behavior of each individual was again recorded until CTmin was identified as a loss of equilibrium without imminent recovery, spasms or the total absence of movement.
Comparisons of the critical thermal maximum and minimum between species were evaluated using two separate one-way ANOVAs followed by post hoc tests using Tukey’s HSD. Biochemical indicators to characterize the response of the AOS and assess cell damage as a response to the critical temperature procedure were evaluated using a PCoA. Data were square-root transformed and normalized prior to analysis. In this case, the bifactorial model had two fixed factors: species with 3 levels (T. mitchelli, T. pearsei and T. dzilamensis) and treatment with (3 levels that included CTmax, CTmin and baseline individuals that received no thermal treatment and were placed in liquid nitrogen immediately after collection (BL). Results were statistically evaluated with a permutational MANOVA with 9999 random permutations of the residuals under the reduced model (Anderson, 2001). A significant interaction would imply that cell damage and AOS response to instantaneous exposure to critical thermal limits vary depending on the species. The amount of LAC resulting from the critical thermal limits elicitation was evaluated with an ANOVA, followed by post hoc comparisons using Tukey’s HSM.
All statistical analyses and graphic representations of results were performed using the ggplot2 library (Wickham, 2016) of the R platform (R Core Team, 2019) and the PRIMER 7 program (Clarke and Gorley, 2015) with PERMANOVA (Anderson et al., 2008).
While the RMR of the three species was markedly similar (Figure 2A), the MMR of T. dzilamensis, was 9.9 and 6.2 times greater than in T. mitchelli and T. pearsei, respectively. This resulted in an AS of T. dzilamensis 12.4 and 7.5 times greater than T. mitchelli and T. pearsei, respectively (Figure 2A). It should be noted that the mean high values in T. dzilamensis were associated with greater variability, indicating considerable heterogeneity in activity metabolism in this species.
Figure 2 (A) Routine metabolic rate (RMR), maximum metabolic rate (MMR) and aerobic scope (AS) of T. mitchelli, T. pearsei and T. dzilamensis. Numbers above the columns are the mean oxygen consumption values for each species and treatment, also showing a standard deviation. (B) Principal coordinate analysis (PCoA) of oxygen consumption of individuals of the three species in RMR, MMR, and AS.
The PCoA applied to the physiological indicators of aerobic metabolism (Figure 2B) showed a clear separation of T. dzilamensis with high values of MMR and AS, but not RMR. These differences were confirmed by the MANOVA, which showed significant differences in these indicators between at least two species (pseudo-F = 3.99; p < 0.01; 9951 unique permutations). Further post hoc showed significant differences between T. dzilamensis and both T. pearsei (pseudo-t = 2.24; p < 0.01; 5052 unique permutations) and T. mitchelli (pseudo-t = 1.188; p < 0.05; 330 unique permutations), although the latter were marginal. By contrast, these indicators in T. pearsei and T. mitchelli were statistically similar (pseudo-t = 1.051; p > 0.05; 495 unique permutations).
PCoA also showed a markedly large dispersion among individuals of T. dzilamensis compared to the other two species (Figure 2B). In the context of a confirmed heterogeneity of variance (pseudo-F = 15.43; p < 0.001), it is not clear whether statistical differences occurred among the centroids representing these species or they are a product of large and unequal multivariate dispersion. Nevertheless, several individuals of T. dzilamensis showed higher indicators of aerobic metabolism, particularly MMR and AS than any of the other two species (Figure 2B).
The PCoA applied to indicators of the AOS, and oxidative damage in Typhlatya species at rest and post-activity explained 50% of the total variation in the first two principal coordinates (Figure 3). The eigenvectors that contributed most to sample separation in the first coordinate were SOD (0.8637), GST (0.8236) and, to a lesser extent, PO (0.4365). Data points corresponding to BL in T. dzilamensis were clearly separated from the rest on the horizontal axis (Figure 3). For the second principal coordinate, LPO (0.7807), CAT (-0.6409), and GSH (0.3935) were the eigenvectors that contributed most to the separation of samples. However, samples with high values of these indicators did not correspond to any species or experimental treatment in particular.
Figure 3 Principal coordinate analysis (PCoA) of antioxidant system indicators (CAT, GST, GSH and SOD) and cell damage (PO and LPO) from untreated (BL) T. mitchelli, T. pearsei and T. dzilamensis and individuals sampled 0, 30, 60 and 120 minutes (T0 to T3) after induced activity.
The MANOVA showed a significant interaction between species and time (pseudo-F = 1.46; p < 0.05; 9887 unique permutations; Table 1A), indicating that the response of the AOS and oxidative damage to physical exercise over time varied between Typhlatya species. Post hoc paired comparisons (Table 1B) indicated that physical activity significantly impacted AOS in T. dzilamensis through differences between BL and samples taken at all times after exercise. Only marginal differences were found between BL and T0 in T. mitchelli, possibly due to an individual with anomalous cell damage (PO and LPO). No significant differences in AOS indicators and oxidative damage derived from exercise were found in T. pearsei. Overall, these results suggest that exercise-derived metabolic activation in T. mitchelli and T. pearsei was not associated with oxidative damage or alterations in AOS indicators.
Table 1 Results of the permutational MANOVA on the biochemical indicators of individuals of T. mitchelli, T. pearsei and T. dzilamensis subjected to five minutes of exercise and sampled at four time intervals: 0 (immediately), 30, 60 and 120 minutes after physical activity (T0 – T3 respectively), which were compared to baseline individuals (BL).
When comparing AOS indicators between species at each sampling time, differences were only found between the BL of T. dzilamensis and that of the other two species (pseudo-t between 1.63 and 2.76; p < 0.05; 9953 and 9801 unique permutations). These results indicate that AOS enzymes under routine metabolism conditions vary among species and suggest that T. dzilamensis could be constitutively primed to respond to environmental changes.
The ANOVA on LAC quantity only showed significant differences between species (Table 2A), implying that there was no accumulation of lactate derived from exercise at any time. Post hoc comparisons confirmed that T. dzilamensis had a higher mean lactate concentration than T. mitchelli and T. pearsei (Table 2B), while the two latter had similar concentrations. In general, these results suggest that physical activity did not involve lactate accumulation in any of the Typhlatya species and that T. dzilamensis has more LAC than any of the two other species studied.
Table 2 Lactate content (LAC) in BL and post-exercise individuals of T. mitchelli, T. pearsei and T. dzilamensis.
One-way ANOVAs, used to compare the critical thermal limits of the three species, showed significant differences in CTmax between species (F = 10.32; p < 0.01). Multiple comparisons showed that the CTmax of T. dzilamensis (36.5 ± 0.8°C) was significantly higher than T. mitchelli (34.9 ± 0.9°C; mean difference = 1.55, adjusted p <0.01) and T. pearsei (34.7± 0.3°C; mean difference = 1.77, adjusted p <0.01), whilst no difference was found between the latter (mean difference = 0.22, adjusted p = 0.88) (Figure 4). In contrast, the CTmin (F = 1.13; p = 0.35) of T. mitchelli (13.8 ± 0.7°C), T. pearsei (13.9 ± 0.5°C) and T. dzilamensis (14.3 ± 0.7°C) was statistically similar (Figure 4).
Figure 4 Critical thermal maximum and minimum of T. mitchelli, T. pearsei and T. dzilamensis. The black line in each box shows the median of each group, the white dot the mean, the whiskers extend to an extreme data not greater than two standard deviations and the black dots represent outliers. Different letters represent means that resulted significantly different.
The thermal window for the three species was 20.8°C for T. mitchelli, 21.1°C for T. pearsei and 22.2°C for T. dzilamensis. The net difference in the thermal window of T. dzilamensis compared to that of T. pearsei and T. mitchelli was 1.4 and 1.1°C, respectively.
PCoA applied to indicators of AOS and oxidative damage in individuals subject to experimental procedures to determine CTmax and CTmin explained 51% of the total variation in the first two principal coordinates (Figure 5). The indicators that contributed most to the variation in the first principal coordinate (horizontal) were SOD (0.8763) and GST (0.7911), followed by PO (0.4698) and GSH (-0.4246). The indicators with the highest contribution to the second coordinate (vertical) were CAT (0.7539) and LPO (-0.6233). The ordination showed that samples corresponding to T. dzilamensis were all associated with high GSH and low SOD, GST, and PO values, with no marked distinctions between BL individuals and those used to determine critical thermal limits (Figure 5). Samples corresponding to T. pearsei and T. mitchelli were located to the left of the ordination map, associated with relatively high SOD, GST, and PO values. Whereas T. mitchelli samples presented a higher concentration of CAT, T. pearsei samples had relatively higher values of LPO. Here again, no significant differences were observed between BL individuals and those used to determine critical thermal limits (Figure 5).
Figure 5 Principal coordinate analysis (PCoA) of antioxidant system indicators [Catalase (CAT), Glutathione S-transferase (GST), total Glutathione (GSH) and Superoxide dismutase (SOD)] and cellular damage [protein carbonylation (PO) and lipid peroxidation (LPO)] of T. mitchelli, T. pearsei and T. dzilamensis individuals sampled at the end of the critical thermal limits procedures (CTmax and CTmin), and compared to baseline (BL) individuals.
The MANOVA showed a significant interaction between treatment and species (Table 3A). However, further paired comparisons showed that BL, CTmax and CTmin samples within each species were generally similar, with only two cases having marginal differences (Table 3B). These results indicate that the procedures to determine critical thermal limits, did not have a significant effect on oxidative damage at the cellular level or on the activity of AOS enzymes compared to a baseline condition. The MANOVA also showed significant differences between species, suggesting that the overall AOS enzyme activity differed between T. dzilamensis, T. pearsei and T. mitchelli irrespective of having been subject to critical thermal determinations.
Table 3 Results of the permutational MANOVA on the biochemical indicators of individuals of T. mitchelli, T. pearsei and T. dzilamensis subjected to procedures to obtain the critical thermal maximum and minimum (CTmax and CTmin) which were compared to baseline individuals (BL).
In close correspondence, LAC content also only differed between species (Table 4A), indicating that the procedures to determine critical thermal limits did not involve anaerobic metabolism in these species. Post hoc comparisons showed a higher concentration of lactate in T. dzilamensis compared to T. mitchelli and T. pearsei, while the latter did not differ significantly from each other (Table 4B).
Table 4 Lactate accumulation before and after attainment of critical thermal limits in individuals of T. mitchelli, T. pearsei and T. dzilamensis.
The present study for the first time compares metabolic, thermal and biochemical indicators of three closely related species of Typhlatya, and shows striking differences related to the characteristics of the subterranean systems of the Yucatan Peninsula in which they occur. Results indicate that T. dzilamensis, the only species found in marine groundwater, has a higher AS and thermal tolerance than T. mitchelli and T. pearsei, which occur only in fresh groundwater habitats. This difference is noteworthy because all three species showed a similar RMR, indicating that the energetic cost of routine activities is independent of the environments they inhabit. It also shows that the difference in AS between species is mainly due to variations in the MMR attained after being induced to the same type, intensity, and duration of physical exercise. Considering the strong effect of environmental conditions on the metabolism of aquatic animals (Pörtner, 2002; Levin, 2003; Pörtner, 2010; Lushchak, 2012; Fang et al., 2019), results herein are discussed in the light of the physiological traits characterizing each species and how these relate to their distribution patterns in the anchialine systems of the Yucatan Peninsula.
According to several authors (Sokolova et al., 2012; Farrell, 2016; Pörtner et al., 2017; Rodríguez-Fuentes et al., 2017) the AS is an indicator of the metabolic energy available once the basal physiological requirements have been satisfied. Therefore, differences in the ability to adjust metabolism in the face of the same stressor – in this case induced physical activity – can be interpreted as differences in physiological plasticity amongst species. The greater AS as a result of MMR in T. dzilamensis suggests that the metabolic increase involves physical capacities to deliver oxygen to the cells, as well as the onset of biochemical mechanisms for energy production and pathways to control ROS, amongst others. Physiological plasticity has been linked to an increased resilience (Tepolt and Somero, 2014; Magozzi and Calosi, 2015; Seebacher et al., 2015; Vinagre et al., 2016; Norin and Metcalfe, 2019) and greater adaptive capacity (Gorban et al., 2016; Norin and Metcalfe, 2019). Furthermore, it has been proposed that a limited antioxidant capacity implies a lower acclimation potential, leading to reduced fitness and, ultimately, an inability to respond to environmental stress (Madeira et al., 2013; Pallarés et al., 2020). In our study, T. dzilamensis was the species with the greatest metabolic response to physical activity, coupled with a primed and responsive AOS and a greater CTmax. Whilst research directed to compare physiological tolerance to salinity is needed, it is possible that the greater physiological plasticity and resilience found in T. dzilamensis relate to its occurrence in saline underground environments beyond the limits of those where T. mitchelli and T. pearsei can endure.
Considering that osmoregulation in euryhaline crustaceans requires, among other things, an increase of metabolic rate (Ramaglia et al., 2018), it is likely that those individuals with a broad AS could meet the energy expenditure that a change in environmental salinity demands. T. dzilamensis is the only species recorded in both fresh and marine groundwater bodies of the Yucatan Peninsula (Benítez et al., 2019), and shrimp have been seen crossing the halocline in a time span of a few minutes (Chavez.Solís pers. obs.). Vertical migrations in search of food or escaping from predators could drive T. dzilamensis to an abrupt and extreme environmental change that requires an instantaneous increase in energy production. Whilst such an euryhaline nature still remains to be investigated, results herein suggest that an increased AS could be one of the key differences allowing this species to survive on either side of the halocline.
According to our results, such physiological adjustments are realistic and have been previously described in Halocaridina rubra, an Atyid from Hawaiian anchialine systems, where salinity fluctuates abruptly and frequently (~20‰ in 24-hour periods). This species presents structural modifications, an increase in mitochondria-rich cells (involved in osmoregulation) in the gills (Havird et al., 2014a), and maintains active the mechanisms and expression of genes involved in osmoregulation (Henry, 2001; Jayasundara et al., 2007; Rahi et al., 2018; Havird et al., 2019). Although the presence of these adaptations has not been studied in any Typhlatya species, results herein may help to explain the presence of T. dzilamensis on either side of the halocline.
Food availability and primary production in anchialine systems are heterogeneous. Cenotes receive a greater input of allochthonous organic matter, and in some instances, there can be photosynthesis (Pohlman et al., 1997; Chávez-Solís et al., 2020). In contrast, caves are considered oligotrophic due to a severe and continuous lack of food availability (Brankovits et al., 2017; Hershey and Barton, 2018). In environments where food is scarce, the survival of a population may be determined by extreme physiological modifications. Such is the case of cavefish Astyanax mexicanus that have developed insulin resistance, allowing them to survive for long periods without feeding (Riddle et al., 2018). Auer et al. (2018) observed that juvenile wild salmons with greater MMR had higher survival rates in environments with lower food availability, suggesting that higher MMR confers greater competitive ability in an environment where food is the limiting resource. Along with the differences in the dietary carbon apportionment amongst the studied Typhlatya species, (Chávez-Solís et al., 2020), it seems that the higher MMR observed in T. dzilamensis could constitute an advantage in the oligotrophic cave environment below the halocline. Under the strong selective pressure exerted by food scarcity, a higher AS could represent an expansion in the availability of potential energy for foraging or migrating in search of food.
One noteworthy aspect of the exercise-derived metabolic responses measured herein is the high intraspecific heterogeneity observed in T. dzilamensis. In addition to the inherent variability of measuring physiological indicators in the laboratory, it is necessary to keep in mind that the experiments were conducted in situ, with wild organisms that had not been subject to previous conditions standardizing their experience. Although we do not have enough information to explain such variability, sources of variation in metabolic descriptors of individuals of the same species are usually related to size, reproductive state, feeding, sex, and molting stage in the case of crustaceans (Alter et al., 2015; Chabot et al., 2016b). While individual differences were methodologically unfeasible to control in the field experiments, it can be assumed that these sources of variation were common to the three species studied. Nevertheless, it is interesting to note that individuals of T. mitchelli and T. pearsei were more homogeneous in their responses compared to T. dzilamensis, something that could be explained if physiological responses to stress that are smaller in their mean magnitude also tend to be more homogeneous than those with larger mean magnitudes.
Aerobic metabolism in the mitochondria generates about 90% of ROS even during basal metabolism (Sies, 1993; Balaban et al., 2005), and ROS increase with increasing metabolic rate (Storey, 1996; Rosa et al., 2008; Rodríguez-Fuentes et al., 2017). An increased production of reactive molecules can damage proteins such as DNA and lipid membranes and, if the propagation of these ROS is not prevented, the organism may undergo severe cell stress, lose functionality, and ultimately die. The AOS response in all three Typhlatya species was in close correspondence with the levels of aerobic metabolism following physical activity. As T. dzilamensis increased oxygen consumption, the antioxidant system was activated accordingly to the increase in ROS production, thus preventing cellular damage. In contrast, T. mitchelli and T. pearsei did not significantly increase oxygen consumption and consequently did not show an increase in AOS indicators or cell damage. As expected, T. mitchelli and T. pearsei display an efficient regulation of ROS even under induced metabolic activity, demonstrating that the energetic and biochemical mechanisms triggered by maximum activity are highly efficient in the caves where they inhabit.
A striking difference from the antioxidant analysis was the high quantity of GSH in BL individuals of T. dzilamensis compared to T. mitchelli and T. pearsei. Storey (1996) was the first to propose that animals that frequently face oxidative stress maintain the AOS constitutively activated. This strategy has been termed “defensive priming” and was first postulated in species with high thermal tolerance (Dong et al., 2008), and a similar mechanism has been suggested in sulfur-tolerant populations (Joyner-Matos and Julian, 2012). An alternative strategy to defensive priming is a quick activation of the AOS when ROS increase and the activation of oxidative stress repair mechanisms is needed (Joyner-Matos and Julian, 2012). The high concentration of GSH observed in T. dzilamensis could be indicative of a defensive priming strategy that would bring advantages to individuals that inhabit a more heterogeneous environment where frequent interactions with the halocline and hypoxia conditions are likely to occur. In contrast, T. mitchelli and T. pearsei may have a more conventional stress response strategy, as they exclusively inhabit freshwater environments, where there is little or no interactions with haloclines (Chávez-Solís et al., 2020).
Compared to surface inland water bodies, temperature in anchialine systems is remarkably constant both on daily and annual time scales (Chávez-Solís, 2015; Mejía-Ortíz et al., 2020). Whilst this led to expect similar thermal tolerance in the three Typhlatya species, T. dzilamensis presented a higher CTmax than T. mitchelli and T. pearsei. A greater physiological plasticity has been linked to higher thermal tolerance and the potential to invade and colonize diverse habitats (Sokolova and Pörtner, 2003; Lande, 2009; Mascaró et al., 2016). Tepolt and Somero (2014) suggested that due to its thermal tolerance (measured as critical thermal maxima and cardiac function), Carcinus maenas could colonize large subpolar areas of the European continent, as well as temperate and subtropical areas of the American continent. Vinagre et al. (2016) and Cumillaf et al. (2016) found a direct relationship between the size of the thermal window and tolerance to warming in 20 species of crustaceans and fish, showing that species from temperate environments and wider thermal windows have greater tolerance to warming than the tropical species. A wider thermal window in T. dzilamensis further emphasizes the relatively greater tolerance of this species to environmental heterogeneity.
The critical temperature is a metric that integrates cellular, biochemical and tissue scales which allows a direct comparison of temperature sensitivity among populations, species and communities (Bates and Morley, 2020). The similarity of the biochemical indicators from BL individuals of all species and those who underwent the critical thermal limits procedures is evidence that the method complies with the theoretical expectations when applied to Typhlatya, and that there is a difference in thermal tolerance among these species.
None of the species studied showed accumulation of LAC derived from either exercise or short-term exposure to critical temperatures, which indicates that these conditions or procedures did not require resorting to anaerobiosis to satisfy the demand for metabolic energy. It is noteworthy, however, the comparatively higher baseline concentration of lactate in T. dzilamensis. Records of Typhlatya swimming in sulfur clouds (Pakes et al., 2014), hypoxic zones (Brankovits et al., 2017; Chávez-Solís et al., 2020), as well as lower levels of dissolved oxygen below the halocline in Ponderosa (Chávez-Solís et al., 2020), suggest that T. dzilamensis may have to resort to anaerobic metabolism frequently. It is possible that these behaviors together with habitat conditions common to T. dzilamensis result in chronic lactate accumulation. An effect such as this has been reported in Niphargus virei, a hypoxia-resistant amphipod (Hervant et al., 1999), where lactate accumulated during periods of hypoxia can be de novo synthesized to glycogen once re-oxygenation is possible. Similarly, experiments with H. rubra (Atyidae) have revealed their capacity to survive up to 7 days under anoxia conditions without any oxygen consumption (Havird et al., 2014b). Although lactate concentration was not measured in their study, the authors concluded that this species must rely on anaerobic metabolism to meet metabolic energy demands. These and other questions on lactate fluctuations and the role of anaerobiosis in supplying energy makes it necessary to further investigate hypotheses on physiological capacities in species that inhabit environments where hypoxic or anoxic conditions are common.
According to Pigliucci et al. (2006), phenotypic plasticity can be defined as the attribute of an individual’s genotype to produce different phenotypes in response to environmental stimuli. Physiological plasticity, therefore, is a component of the response repertoire of the expressed phenotypes and is related to the organic functioning of systems at various levels of organization. It has been also postulated that physiological plasticity allows the emergence of a new environmentally induced phenotype, the expression of which can be fixed through natural selection (Pigliucci et al., 2006). Recent ancestral reconstructions support the hypothesis of a low salinity common ancestor for the Yucatan Typhlatya (Ballou et al., 2022), which in turn suggests that the salinity tolerance trait observed in T. dzilamensis is an independent secondary transition. In their study, Ballou et al. (2022) recorded at least two unknown Typhlatya species in the Yucatan Peninsula that were genetically identified, but have not yet been morphologically described. Whilst cryptic speciation amongst Typhlatya is possible, COI barcoding allowed to confirm that all of the specimens in the present study were correctly identified. Results herein, thus, provide evidence in support of a physiological plasticity observed in T. dzilamensis compared to its sister species that closely relates to the heterogeneous environment they inhabit.
The species of the genus Typhlatya in the present study possess contrasting physiological traits that correspond to the environmental characteristics of the microhabitat in which each species is distributed. T. dzilamensis, commonly found in the subterranean saltwater environment, displayed the greatest physiological plasticity compared to T. mitchelli and T. pearsei, hence has a greater resilience to environmental fluctuations. The activity of GSH and lactate concentration in T. dzilamensis further suggest that this species has a permanent “defensive priming” strategy that keeps the antioxidant system constitutively prepared for the eventuality of physiological stress, a recurrent condition in the relatively heterogeneous environment they inhabit.
In contrast to T. dzilamensis, the freshwater species T. mitchelli and T. pearsei, were very similar in exhibiting low aerobic scope, low lactate and an overall low reactivity to imposed physical activity. This suggests that T. mitchelli and T. pearsei have less physiological capacity for an immediate response to intensive exercise, which indicates a reduced plasticity, and thus expected to be more vulnerable to abrupt environmental fluctuations.
Current anthropogenic pressures on the aquifer may pose an increased risk to these species, all the more knowing that freshwater species may be particularly susceptible to environmental shifts. Future research should focus on evaluating physiological limits and resilience of species to changes in salinity, dissolved oxygen, and the presence of contaminants in the aquifer for the purpose of prevention and restoration of subterranean aquatic ecosystems.
The raw data supporting the conclusions of this article will be made available by the authors, without undue reservation.
EC-S, KP, CR, and MM contributed to the conception and design of the study. EC and MM performed the statistical analysis and prepared de figures. EC-S, FD, MM, KP, DR, CR, and NT performed field experiments, analysed metabolic response and thermal tolerance. EC and GR-F obtained and analysed the biochemical indicators. EC wrote the first draft of the manuscript. All authors contributed to manuscript revision, read and approved the submitted version.
EC-S was awarded a CONACYT scholarship for PhD studies (CVU/Becario: 545211/294499). Field work was partially funded by project IN228319 (PAPIIT-DGAPA-UNAM).
EC-S acknowledges the CONACYT grant for PhD studies (545211/294499), as this manuscript is part of the requirements for the doctoral program of the Posgrado en Ciencias Biológicas UNAM. Field work was supported by the Cenoteando organization. Authors are grateful to Quetzalli Hernández, Dorottya Angyal, Michel Vázquez, and Arturo Mora for their cavediving support, and Parque Ecológico Chikin Ha for their support in our field experiments. Authors are grateful to Claudia Caamal, Ariadna Sanchez Arteaga and Karen Ortega for their support in obtaining biochemical indicators in the laboratory.
The authors declare that the research was conducted in the absence of any commercial or financial relationships that could be construed as a potential conflict of interest.
All claims expressed in this article are solely those of the authors and do not necessarily represent those of their affiliated organizations, or those of the publisher, the editors and the reviewers. Any product that may be evaluated in this article, or claim that may be made by its manufacturer, is not guaranteed or endorsed by the publisher.
The Supplementary Material for this article can be found online at: https://www.frontiersin.org/articles/10.3389/fmars.2022.892274/full#supplementary-material
Alter K., Paschke K., Gebauer P., Cumillaf J.-P., Pörtner H. O. (2015). Differential physiological responses to oxygen availability in early life stages of decapods developing in distinct environments. Mar. Biol. 162, 1111–1124. doi: 10.1007/s00227-015-2654-4
Anderson M. J. (2001). Permutation tests for univariate or multivariate analysis of variance and regression. Can. J. Fisheries Aquat. Sci. 58, 626–639. doi: 10.1139/f01-004
Anderson M. J. (2017). “Permutational multivariate analysis of variance (PERMANOVA),” in Wiley StatsRef: Statistics reference online. (Massey University, Auckland, New Zealand: John Wiley & Sons, Ltd).
Anderson M. J., Gorley R. N., Clarke K. R. (2008). PERMANOVA+ for PRIMER: Guide to software and statistical methods. Plymouth, Uk: PRIMER-E
Ángeles-González L. E., Martínez-Meyer E., Rosas C., Guarneros-Narváez P. V., López-Rocha J. A., Escamilla-Aké Á., et al. (2021). Long-term environmental data explain better the abundance of the red octopus (Octopus maya) when testing the niche centroid hypothesis. J. Exp. Mar. Biol. Ecol. 544, 151609. doi: 10.1016/J.JEMBE.2021.151609
Ángeles-González L. E., Martínez-Meyer E., Yañez-Arenas C., Velázquez-Abunader I., Garcia-Rueda A., Díaz F., et al. (2020). Using realized thermal niche to validate thermal preferences from laboratory studies. How do they stand? Ecol. Indic. 118, 106741. doi: 10.1016/J.ECOLIND.2020.106741
Auer S. K., Anderson G. J., McKelvey S., Bassar R. D., McLennan D., Armstrong J. D., et al. (2018). Nutrients from salmon parents alter selection pressures on their offspring. Ecol. Lett. 21, 287–295. doi: 10.1111/ele.12894
Balaban R. S., Nemoto S., Finkel T. (2005). Mitochondria, oxidants, and aging. Cell 120, 483–495. doi: 10.1016/j.cell.2005.02.001
Ballou L., Brankovits D., Chávez-Solís E. M., Chávez Díaz J. M., Gonzlez B. C., Rohret S., et al. (2022). An integrative re-evaluation of typhlatya shrimp within the karst aquifer of the yucatán peninsula, Mexico. Sci. Rep. 12, 1–17. doi: 10.1038/s41598-022-08779-9
Bates A. E., Morley S. A. (2020). Interpreting empirical estimates of experimentally derived physiological and biological thermal limits in ectotherms. Can. J. Zoolog 98, 237–244. doi: 10.1139/cjz-2018-0276
Benítez S., Illife T. M., Quiroz-Martínez B., Alvarez F. (2019). How is the anchialine fauna distributed within a cave? a study of the ox Bel ha system, Yucatan peninsula, Mexico. Subterranean Biol. 31, 15–28. doi: 10.3897/subtbiol.31.34347
Brankovits D., Pohlman J. W., Niemann H., Leigh M. B., Leewis M. C., Becker K. W., et al. (2017). Methane-and dissolved organic carbon-fueled microbial loop supports a tropical subterranean estuary ecosystem. Nat. Commun. 8, 1–3. doi: 10.1038/s41467-017-01776-x
Chabot D., McKenzie D. J., Craig J. F. (2016a). Metabolic rate in fishes: Definitions, methods and significance for conservation physiology. J. Fish Biol. 88, 1–9. doi: 10.1111/jfb.12873
Chabot D., Steffensen J. F., Farrell A. P. (2016b). The determination of standard metabolic rate in fishes. J. Fish Biol. 88, 81–121. doi: 10.1111/jfb.12845
Chávez-Solís E. M. (2015). Aspectos ecológicos y etológicos de decápodos estigobios (Creaseria morleyi y typhlatya spp.) en cenotes de yucatán: utilización espacio- temporal, cambios anuales y relaciones interespecíficas. (Tesis de Maestría). Universidad Nacional Autónoma de México, Mexico City, Mexico. Available at: https://repositorio.unam.mx/contenidos/80607
Chávez-Solís E. M., Solís C., Simões N., Mascaró M. (2020). Distribution patterns, carbon sources and niche partitioning in cave shrimps (Atyidae: Typhlatya). Sci. Rep. 10, 1–16. doi: 10.1038/s41598-020-69562-2
Cheung W. W. L., Close C., Lam V., Watson R., Pauly D. (2008). Application of macroecological theory to predict effects of climate change on global fisheries potential. Mar. Ecol. Prog. Ser. 365, 187–197. doi: 10.3354/MEPS07414
Cheung W. W., Lam V. W., Sarmiento J. L., Kearney K., Watson R., Zeller D., et al. (2010). Large-Scale redistribution of maximum fisheries catch potential in the global ocean under climate change. Global Change Biol. 16, 24–35. doi: 10.1111/j.1365-2486.2009.01995.x
Christiansen K. (1962). Proposition pour la classification des animaux cavernicoles. Spelunka 2, 75–78.
Clarke K., Gorley R. (2015). PRIMER version 7: User manual/tutorial. Plymouth, UK (Plymouth, United Kingdom: PRIMER-E Ltd).
Clark T. D., Sandblom E., Jutfelt F. (2013). Aerobic scope measurements of fishes in an era of climate change: respirometry, relevance and recommendations. J. Exp. Biol. 216, 2771–2782. doi: 10.1242/jeb.084251
Cumillaf J. P., Blanc J., Paschke K., Gebauer P., Diaz F., Re D., et al. (2016). Thermal biology of the sub-polar-temperate estuarine crab hemigrapsus crenulatus (Crustacea: Decapoda: Varunidae). Biol. Open 5, 220–228. doi: 10.1242/bio.013516
Díaz-Acosta A. E., Membrillo-Hernández J. (2006). Consecuencias fisiológicas de la oxidación de proteínas por carbonilación en diversos sistemas biológicos. TIP. Rev. especializada en Cienc. químico-biológicas 9, 34–44.
Díaz F., Re A. D., Sánchez A., Cruz H., González R. A., Sánchez L. N., et al. (2013). The effects of exposure to critical thermal maxima on the physiological, metabolic, and immunological responses in adult white shrimp litopenaeus vannamei (Boone). Mar. Freshw. Behav. Physiol. 45, 365–374. doi: 10.1080/10236244.2013.771911
Dong Y., Miller L. P., Sanders J. G., Somero G. N. (2008). Heat-shock protein 70 (Hsp70) expression in four limpets of the genus lottia: Interspecific variation in constitutive and inducible synthesis correlates with in situ exposure to heat stress. Biol. Bull 215 (2), 173–81. doi: 10.2307/25470698
Eddy T. D., Bernhardt J. R., Blanchard J. L., Cheung W. W. L., Colléter M., du Pontavice H., et al. (2021). Energy flow through marine ecosystems: Confronting transfer efficiency. Trends Ecol. Evol. 36, 76–86. doi: 10.1016/J.TREE.2020.09.006
Ellman G., Courtney K., Andres J., Featherstone R. (1961). A new and rapid colorimetric determination of acetylcholinesterase activity. Biochem. Pharmacol. 7, 88–95. doi: 10.1016/0006-2952(61)90145-9
Espinasa L., Solís E. M. C., Mascaró M., Rosas C., Simoes N., Violette G. (2019). A new locality and phylogeny of the stygobitic typhlatya shrimps for the Yucatan peninsula. Speleobiol. Notes 10, 19–27. doi: 10.5563/spbn.v10i0.91
Fang Y., Chan V. K. S., Hines C. W., Stiller K. T., Richards J. G., Brauner C. J. (2019). The effects of salinity and photoperiod on aerobic scope, hypoxia tolerance and swimming performance of coho salmon (Oncorhynchus kisutch) reared in recirculating aquaculture systems. Comp. Biochem. Physiol. -Part A: Mol. Integr. Physiol. 231, 82–90. doi: 10.1016/j.cbpa.2019.01.026
Farrell A. P. (2016). Pragmatic perspective on aerobic scope: Peaking, plummeting, pejus and apportioning. J. Fish Biol. 88, 322–343. doi: 10.1111/jfb.12789
Farrell A. P., Hinch S. G., Cooke S. J., Patterson D. A., Crossin G. T., Lapointe M., et al. (2008). Pacific salmon in hot water: Applying aerobic scope models and biotelemetry to predict the success of spawning migrations. Physiol. Biochem. Zool. 81, 697–708. doi: 10.1086/592057
Fry F. E. J. (1947). Effects of the environment on animal activity. Publications Ontario Fisheries Res. Lab. 68, 1–52.
Gorban A. N., Tyukina T. A., Smirnova E. V., Pokidysheva L. I. (2016). Evolution of adaptation mechanisms: Adaptation energy, stress, and oscillating death. J. Theor. Biol. 405, 127–139. doi: 10.1016/j.jtbi.2015.12.017
Góth L. (1991). A simple method for determination of serum catalase activity and revision of reference range. Clinica Chimica Acta 196, 143–151. doi: 10.1016/0009-8981(91)90067-M
Guderley H., Pörtner H. O. (2010). Metabolic power budgeting and adaptive strategies in zoology: Examples from scallops and fish. Can. J. Zoolog 88, 753–763. doi: 10.1139/Z10-039
Gumulec J., Raudenska M., Hlavna M., Stracina T., Sztalmachova M., Tanhauserova V., et al. (2013). Determination of oxidative stress and activities of antioxidant enzymes in guinea pigs treated with haloperidol. Exp. Ther. Med. 5, 479–484. doi: 10.3892/etm.2012.822
Habig W. H., Pabst M. J., Jakoby W. B. (1974). Glutathione s-transferases. the first enzymatic step in mercapturic acid formation. J. Biol. Chem. 249, 7130–7139. doi: 10.1016/S0021-9258(19)42083-8
Hadwan M. H., Abed H. N. (2016). Data supporting the spectrophotometric method for the estimation of catalase activity. Data Brief 6, 194–199. doi: 10.1016/j.dib.2015.12.012
Havird J. C., Meyer E., Fujita Y., Vaught R. C., Henry R. P., Santos S. R. (2019). Disparate responses to salinity across species and organizational levels in anchialine shrimps. J. Exp. Biol. 222. doi: 10.1242/jeb.211920
Havird J. C., Santos S. R., Henry R. P. (2014a). Osmoregulation in the Hawaiian anchialine shrimp halocaridina rubra (Crustacea: Atyidae): expression of ion transporters, mitochondria-rich cell proliferation and hemolymph osmolality during salinity transfers. J. Exp. Biol. 217, 2309–2320. doi: 10.1242/jeb.103051
Havird J. C., Vaught R. C., Weeks J. R., Fujita Y., Hidaka M., Santos S. R., et al. (2014b). Taking their breath away: Metabolic responses to low-oxygen levels in anchialine shrimps (Crustacea: Atyidae and alpheidae). Comp. Biochem. Physiol. -Part A: Mol. Integr. Physiol. 178, 109–120. doi: 10.1016/j.cbpa.2014.08.015
Henry R. P. (2001). Environmentally mediated carbonic anhydrase induction in the gills of euryhaline crustaceans. J. Exp. Biol. 204, 991–1002. doi: 10.1242/jeb.204.5.991
Hershey O. S., Barton H. A. (2018). The microbial diversity of caves. In: Moldovan O., Kováč Ľ., Halse S. (eds). 235. Cham: Springer. doi: 10.1007/978-3-319-98852-8_5
Hervant F., Garin D., Mathieu J., Freminet A. (1999). Lactate metabolism and glucose turnover in the subterranean crustacean niphargus virei during post-hypoxic recovery. J. Exp. Biol. 202, 579–592. doi: 10.1242/jeb.202.5.579
Hothorn T., Bretz F., Westfall P. (2008). Simultaneous inference in general parametric models. Biometrical J. 50, 346–363. doi: 10.1002/bimj.200810425
Huey R. B. (1991). Physiological consequences of habitat selection. Am. Nat. 137, S91–S115. doi: 10.1086/285141
Hutchison V. H. (1961). Critical thermal maxima in salamanders. Physiol. Zoolog 34, 92–125. doi: 10.1086/physzool.34.2.30152688
Jayasundara N., Towle D. W., Weihrauch D., Spanings-Pierrot C. (2007). Gill-specific transcriptional regulation of Na+/K+-ATPase -subunit in the euryhaline shore crab pachygrapsus marmoratus: sequence variants and promoter structure. J. Exp. Biol. 210, 2070–2081. doi: 10.1242/jeb.004309
Joyner-Matos J., Julian D. (2012). “Oxidative stress in sulfidic habitats,” in Oxidative stress in aquatic ecosystems. Eds. Abele D., Vázquez-Medina J. P., Zenteno-Savín T. (Chichester: Blackwell Publishing Ltd), 677–690.
Lande R. (2009). Adaptation to an extraordinary environment by evolution of phenotypic plasticity and genetic assimilation. J. Evolutionary Biol. 22, 1435–1446. doi: 10.1111/j.1420-9101.2009.01754.x
Levin L. A. (2003). Oxygen minimum zone benthos: Adaptation and community response to hypoxia. Oceanog. And Mar. Biologyl. Mar. Biol.: an Annu. Rev. 41, 1–45. CRC Press, Taylor & Francis Group LLC
Lushchak V. I. (2011). Environmentally induced oxidative stress in aquatic animals. Aquat. Toxicol. 101, 13–30. doi: 10.1016/j.aquatox.2010.10.006
Lushchak V. I. (2012). “Environmentally induced oxidative stress in fish,” in Oxidative stress in aquatic ecosystems. Eds. Abele D., Vázquez-Medina J. P., Zenteno-Savín T. (Chichester: Blackwell Publishing Ltd), 295–307.
Lutterschmidt W. I., Hutchison V. H. (1997a). The critical thermal maximum: Data to support the onset of spasms as the definitive end point. Can. J. Zoolog 75, 1553–1560. doi: 10.1139/z97-782
Lutterschmidt W. I., Hutchison V. H. (1997b). The critical thermal maximum: History and critique. Can. J. Zoolog 75, 1561–1574. doi: 10.1139/z97-783
Madeira D., Narciso L., Cabral H. N., Vinagre C., Diniz M. S. (2013). Influence of temperature in thermal and oxidative stress responses in estuarine fish. Comp. Biochem. Physiol. - A Mol. Integr. Physiol. 166, 237–243. doi: 10.1016/j.cbpa.2013.06.008
Magozzi S., Calosi P. (2015). Integrating metabolic performance, thermal tolerance, and plasticity enables for more accurate predictions on species vulnerability to acute and chronic effects of global warming. Global Change Biol. 21, 181–194. doi: 10.1111/gcb.12695
Maharaj R. R., Lam V. W. Y., Pauly D., Cheung W. W. L. (2018). Regional variability in the sensitivity of Caribbean reef fish assemblages to ocean warming. Mar. Ecol. Prog. Ser. 590, 201–209. doi: 10.3354/MEPS12462
Martínez-Álvarez R. M., Morales A. E., Sanz A. (2005). Antioxidant defenses in fish: Biotic and abiotic factors. Rev. Fish Biol. Fisheries 15, 75–88. doi: 10.1007/s11160-005-7846-4
Mascaró M., Amaral-Ruiz M., Huipe-Zamora I., Martínez-Moreno G., Simoes N., Rosas C. (2016). Thermal tolerance and phenotypic plasticity in juvenile hippocampus erectus perry 1810: effect of acute and chronic exposure to contrasting temperatures. J. Exp. Mar. Biol. Ecol. 483, 112–119. doi: 10.1016/j.jembe.2016.07.005
Mejía-Ortíz L., Christman M. C., Pipan T., Culver D. C. (2020). What’s the temperature in tropical caves? PloS One 15, 1–21. doi: 10.1371/journal.pone.0237051
Mermillod-Blondin F., Lefour C., Lalouette L., Renault D., Malard F., Simon L., et al. (2013). Thermal tolerance breadths among groundwater crustaceans living in a thermally constant environment. J. Exp. Biol. 216, 1683–1694. doi: 10.1242/jeb.081232
Meza-Buendía A. K., Trejo-Escamilla I., Piu M., Caamal-Monsreal C., Rodríguez-Fuentes G., Diaz F., et al. (2021). Why high temperatures limit reproduction in cephalopods? Case Octopus maya. Aquacult. Res. 52, 5111–5123. doi: 10.1111/ARE.15387
Niklitschek E. J., Secor D. H. (2009). Dissolved oxygen, temperature and salinity effects on the ecophysiology and survival of juvenile Atlantic sturgeon in estuarine waters: II. model development and testing. J. Exp. Mar. Biol. Ecol. 381, S161–S172. doi: 10.1016/J.JEMBE.2009.07.019
Norin T., Metcalfe N. B. (2019). Ecological and evolutionary consequences of metabolic rate plasticity in response to environmental change. Philos. Trans. R. Soc. B: Biol. Sci. 374, 1–9. doi: 10.1098/rstb.2018.0180
Pakes J., Weis A. K., Mejía-Ortiz L. (2014). Arthropods host intracellular chemosynthetic symbionts, too: cave study reveals an unusual form of symbiosis. J. Crustacean Biol. 34, 334–341. doi: 10.1163/1937240X-00002238
Pallarés S., Sanchez-Hernandez J. C., Colado R., Balart-García P., Comas J., Sánchez-Fernández D. (2020). Beyond survival experiments: using biomarkers of oxidative stress and neurotoxicity to assess vulnerability of subterranean fauna to climate change. Conserv. Physiol. 8, 1–12. doi: 10.1093/conphys/coaa067
Paschke K., Agüero J., Gebauer P., Díaz F., Mascaró M., López-Ripoll E., et al. (2018). Comparison of aerobic scope for metabolic activity in aquatic ectotherms with temperature related metabolic stimulation: A novel approach for aerobic power budget. Front. Physiol. 9. doi: 10.3389/fphys.2018.01438
Paschke K., Cumillaf J. P., Chimal M. E., Díaz F., Gebauer P., Rosas C. (2013). Relationship between age and thermoregulatory behaviour of lithodes santolla (Molina 1782) (Decapoda, lithodidae) juveniles. J. Exp. Mar. Biol. Ecol. Complete 448 (11), 141–145. doi: 10.1016/J.JEMBE.2013.06.018
Pigliucci M., Murren C. J., Schlichting C. D. (2006). Phenotypic plasticity and evolution by genetic assimilation. J. Exp. Biol. 209, 2362–2367. doi: 10.1242/jeb.02070
Pipan T., Culver D. C. (2012). Convergence and divergence in the subterranean realm: A reassessment. Biol. J. Linn. Soc. 107, 1–14. doi: 10.1111/j.1095-8312.2012.01964.x
Pohlman J. W. (2011). The biogeochemistry of anchialine caves: progress and possibilities. Hydrobiologia 677, 33–51. doi: 10.1007/s10750-011-0624-5
Pohlman J. W., Iliffe T., Cifuentes L. A. (1997). A stable isotope study of organic cycling and the ecology of an anchialine cave ecosystem. Mar. Ecol. Prog. Ser. 155, 17–27. doi: 10.3354/meps155017
Pörtner H. O. (2002). Climate variation and physiology basis to temperature dependent biogeography: sistemic to molecular hierarchy of thermal tolerance in animals. Comp. Biochem. Physiol. 132A, 739–761. doi: 10.1016/S1095-6433(02)00045-4
Pörtner H. O. (2010). Oxygen- and capacity-limitation of thermal tolerance: a matrix for integrating climate-related stressor effects in marine ecosystems. J. Exp. Biol. 213, 881–893. doi: 10.1242/jeb.037523
Pörtner H. O., Bock C., Mark F. C. (2017). Oxygen- and capacity-limited thermal tolerance: bridging ecology and physiology. J. Exp. Biol. 220, 2685–2696. doi: 10.1242/JEB.134585
Pörtner H. O., Farrell A. P. (2008). Physiology and climate change. Science 322, 690–692. doi: 10.1126/science.1163156
Pörtner H. O., Knust R. (2007). Climate change affects marine fishes through the oxygen limitation of thermal tolerance. Science 315, 95–97. doi: 10.1126/science.1135471
Rahi M. L., Moshtaghi A., Mather P. B., Hurwood D. A. (2018). Osmoregulation in decapod crustaceans: physiological and genomic perspectives. Hydrobiologia 825, 177–188. doi: 10.1007/s10750-018-3690-0
Ramaglia A. C., de Castro L. M., Augusto A. (2018). Effects of ocean acidification and salinity variations on the physiology of osmoregulating and osmoconforming crustaceans. J. Comp. Physiol. B: Biochem. Systemic Environ. Physiol. 188, 729–738. doi: 10.1007/s00360-018-1167-0
R Core Team (2019). R: A language and environment for statistical computing. Vienna, Austria, www.R-project.org
Regoli F., Giuliani M. E., Benedetti M., Arukwe A. (2011). Molecular and biochemical biomarkers in environmental monitoring: A comparison of biotransformation and antioxidant defense systems in multiple tissues. Aquat. Toxicol. 105, 56–66. doi: 10.1016/j.aquatox.2011.06.014
Rezende E. L., Tejedo M., Santos M. (2011). Estimating the adaptive potential of critical thermal limits: Methodological problems and evolutionary implications. Funct. Ecol. 25, 111–121. doi: 10.1111/j.1365-2435.2010.01778.x
Riddle M. R., Aspiras A. C., Gaudenz K., Peuß R., Sung J. Y., Martineau B., et al. (2018). Insulin resistance in cavefish as an adaptation to a nutrient-limited environment. Nature 555, 647–651. doi: 10.1038/nature26136
Rodríguez-Fuentes G., Armstrong J., Schlenk D. (2008). Characterization of muscle cholinesterases from two demersal flatfish collected near a municipal wastewater outfall in southern california. ecotoxicol. Environ. Saf. 69, 466–471. doi: 10.1016/j.ecoenv.2007.06.008
Rodríguez-Fuentes G., Murúa-Castillo M., Díaz F., Rosas C., Caamal-Monsreal C., Sánchez A., et al. (2017). Ecophysiological biomarkers defining the thermal biology of the Caribbean lobster panulirus argus. Ecol. Indic. 78, 192–204. doi: 10.1016/j.ecolind.2017.03.011
Rosa C. E., Figueiredo M. A., Lanes C. F. C., Almeida D. V., Monserrat J. M., Marins L. F. (2008). Metabolic rate and reactive oxygen species production in different genotypes of GH-transgenic zebrafish. Comp. Biochem. Physiol. - B Biochem. Mol. Biol. 149, 209–214. doi: 10.1016/j.cbpb.2007.09.010
Schulte P. M. (2015). The effects of temperature on aerobic metabolism: towards a mechanistic understanding of the responses of ectotherms to a changing environment. J. Exp. Biol. 218, 1856–1866. doi: 10.1242/jeb.118851
Seebacher F., White C. R., Franklin C. E. (2015). Physiological plasticity increases resilience of ectothermic animals to climate change. Nat. Climate Change 5, 61–66. doi: 10.1038/nclimate2457
Sies H. (1993). Strategies of antioxidant defense. Eur. J. Biochem. 215, 213–219. doi: 10.1111/j.1432-1033.1993.tb18025.x
Soberón J., Peterson A. T. (2005). Interpretation of models of fundamental ecological niches and species’ distributional areas. Biodiversity Inf. 2, 1–10. doi: 10.1002/pssc.200778935
Sokolova I. M., Frederich M., Bagwe R., Lannig G., Sukhotin A. A. (2012). Energy homeostasis as an integrative tool for assessing limits of environmental stress tolerance in aquatic invertebrates. Mar. Environ. Res. 79, 1–15. doi: 10.1016/j.marenvres.2012.04.003
Sokolova I. M., Pörtner H. O. (2003). Metabolic plasticity and critical temperatures for aerobic scope in a eurythermal marine invertebrate (Littorina saxatilis, Gastropoda: Littorinidae) from different latitudes. J. Exp. Biol. 206, 195–207. doi: 10.1242/jeb.00054
Storey K. B. (1996). Oxidative stress: Animal adaptations in nature. Braz. J. Med. Biol. Res. 29, 1715–1733. Available at: https://www.semanticscholar.org/paper/Oxidative-stress%3A-animal-adaptations-in-nature.-Storey/fb74fcfbef4720a350c13bf89172a3025bb49f54Levin 20Publications/.pdf.
Sumaila U. R., Palacios-Abrantes J., Cheung W. W. L. (2020). Climate change, shifting threat points, and the management of transboundary fish stocks. Ecol. Soc. 25, 1–9. doi: 10.5751/ES-11660-250440
Tepolt C. K., Somero G. N. (2014). Master of all trades: Thermal acclimation and adaptation of cardiac function in a broadly distributed marine invasive species, the European green crab, carcinus maenas. J. Exp. Biol. 217, 1129–1138. doi: 10.1242/jeb.093849
Terblanche J. S., Hoffmann A. A., Mitchell K. A., Rako L., le Roux P. C., Chown S. L. (2011). Ecologically relevant measures of tolerance to potentially lethal temperatures. J. Exp. Biol. 214, 3713–3725. doi: 10.1242/jeb.061283
Thyrring J., Tremblay R., Sejr M. K. (2019). Local cold adaption increases the thermal window of temperate mussels in the Arctic. Conserv. Physiol. 7, 1–10. doi: 10.1093/conphys/coz098
Vinagre C., Leal I., Mendonça V., Madeira D., Narciso L., Diniz M. S., et al. (2016). Vulnerability to climate warming and acclimation capacity of tropical and temperate coastal organisms. Ecol. Indic. 62, 317–327. doi: 10.1016/J.ECOLIND.2015.11.010
Vinagre C., Madeira D., Mendonça V., Madeira C., Diniz M. S. (2021). Warming in shallow waters: Seasonal response of stress biomarkers in a tide pool fish. Estuarine Coast. Shelf Sci. 251, 107187. doi: 10.1016/J.ECSS.2021.107187
Keywords: anchialine, groundwater, stygobionts, metabolic rates, thermal tolerance, antioxidants
Citation: Chávez-Solís EM, Díaz F, Paschke K, Re D, Rosas C, Rodríguez-Fuentes G, Tremblay N and Mascaró M (2022) Physiological characterization of Typhlatya cave shrimps: linking habitat with aerobic metabolism. Front. Mar. Sci. 9:892274. doi: 10.3389/fmars.2022.892274
Received: 08 March 2022; Accepted: 04 July 2022;
Published: 29 July 2022.
Edited by:
Gisela Lannig, Alfred Wegener Institute Helmholtz Centre for Polar and Marine Research (AWI), GermanyReviewed by:
Carolina Madeira, NOVA University Lisbon, PortugalCopyright © 2022 Chávez-Solís, Díaz, Paschke, Re, Rosas, Rodríguez-Fuentes, Tremblay and Mascaró. This is an open-access article distributed under the terms of the Creative Commons Attribution License (CC BY). The use, distribution or reproduction in other forums is permitted, provided the original author(s) and the copyright owner(s) are credited and that the original publication in this journal is cited, in accordance with accepted academic practice. No use, distribution or reproduction is permitted which does not comply with these terms.
*Correspondence: Maite Mascaró, bW1tQGNpZW5jaWFzLnVuYW0ubXg=
Disclaimer: All claims expressed in this article are solely those of the authors and do not necessarily represent those of their affiliated organizations, or those of the publisher, the editors and the reviewers. Any product that may be evaluated in this article or claim that may be made by its manufacturer is not guaranteed or endorsed by the publisher.
Research integrity at Frontiers
Learn more about the work of our research integrity team to safeguard the quality of each article we publish.