- 1Biosecurity Group, Cawthron Institute, Nelson, New Zealand
- 2Institute of Marine Science, University of Auckland, Auckland, New Zealand
- 3New-Zealand Forest Research Institute Limited, Scion, Rotorua, New Zealand
- 4School of Biological Sciences, University of Auckland, Auckland, New Zealand
Impacts of Marine Plastic Debris (MPD) on marine ecosystems are among the most critical environmental concerns of the past three decades. Virgin plastic is often cheaper to manufacture than recycled plastics, increasing rates of plastic released into the environment and thereby impacting ecosystem health and functioning. Along with other environmental effects, MPD can serve as a vector for marine hitchhikers, facilitating unwanted organisms’ transport and subsequent spread. Consequently, there is a growing demand for more eco-friendly replacements of conventional plastic polymers, ideally with fit-for-purpose properties and a well-understood life cycle. We enriched polybutylene succinate (PBS) with three different concentrations of oyster shell to investigate the dynamics of biofouling formation over 18 weeks at the Nelson Marina, Aotearoa/New Zealand. Our study focused on oyster shell concentration as a determinant of fouling assemblages over time. While generally considered as a waste in the aquaculture sector, we used oyster shells as a variable of interest to investigate their potential for both, environmental and economic benefits. Using bacterial 16S and eukaryotic 18S rRNA gene metabarcoding, our results revealed that following immersion in seawater, time played a more critical role than substrate type in driving biofouling community structures over the study period. In total, 33 putative non-indigenous species (NIS) and 41 bacterial families with putative plastic-degrading capability were detected on the different substrates. Our analysis of NIS recruitment revealed a lower contribution of NIS on shell-enriched substrates than unadulterated polymers samples. In contrast, the different concentrations of oyster shells did not affect the specific recruitment of bacterial degraders. Taken together, our results suggest that bio-based polymers and composites with increased potential for biodegradability, recyclability, and aptitude for the selective recruitment of marine invertebrates might offer a sustainable alternative to conventional polymers, assisting to mitigate the numerous impacts associated with MPD.
Introduction
Plastic pollution has become a worldwide environmental threat, impacting nearly all aquatic and terrestrial ecosystems (Galgani et al., 2019). Since mass production of plastics began in the 1950s, their use has increased exponentially across consumer groups and industry sectors (Galgani et al., 2017). As plastic production continues to increase, particularly for packaging and single-use applications (i.e. 30-40% of annual production; PlasticsEurope (2018)), so does their release into natural systems (Rochman et al., 2013; Geyer et al., 2017). Jambeck et al. (2015) estimated that 275 million metric tons of plastic waste were generated in 2010 from 192 coastal countries, with 4.8 to 12.7 million metric tons entering the ocean. Isobe et al. (2021) analyzed both published and unpublished microplastics abundance data between 2000 and 2019, revealing a total of 24.4 trillion plastic particles (8.2 x 104 ~ 57.8 x 104 tons) in the world’s upper oceans. Past investigations have reported impacts on marine birds (Wilcox et al., 2015; Thiel et al., 2018), mammals (Campani et al., 2013; Panti et al., 2019), fishes (Romeo et al., 2015; Miranda and de Carvalho-Souza, 2016), bivalves (Rochman et al., 2015; Li et al., 2020) and planktonic organisms (Lin, 2016), with the potential transfer of microplastics through the planktonic food web (Setälä et al., 2014). Moreover, new studies are highlighting emerging impacts on biodiversity and species biogeography, with marine plastic debris acting as vectors for non-indigenous species (NIS) and pathogen transport (Audrézet et al., 2020; García-Gómez et al., 2021; Meng et al., 2021).
In response, an increasing research effort is directed towards developing bio-based products (i.e., bio-fibers, bio-polymers, and bio-composites) that could eventually replace synthetic materials on the market (Liu et al., 2019; Vinod et al., 2020). Bio-polymers are polymers derived from plants, animals, and microbes. They can be used to manufacture ‘eco-friendly’ bio-plastics for biomedical, industrial (processing and packaging), and household applications (Ganguly et al., 2020; Vinod et al., 2020). Recently, particular interest has been given to the revalorization of food waste to produce polymers and additives applicable in bio-plastics production (Balart et al., 2021). For example, agro-industrial waste (e.g., the skin of citrus, banana, potato, coffee waste, sugar molasses) and aquaculture waste (e.g., fish skin, carcasses, heads, bones, shrimp waste, shell waste) contain compounds that can be used as renewable resources for bio-polymer production (Sharmila et al., 2020; Lionetto and Esposito Corcione, 2021). In aquaculture and particularly in the shellfish industry, conventional plastics are used at every step, whether it is for tanks, ropes, trays, pipping, buoys, or equipment for packaging (Moore, 2014). On the other hand, the shellfish industry generates considerable amounts of shell waste that could be reused and/or recycled for environmental and economic benefits. The shell is a composite biomaterial, in which the mineral phase (i.e., calcium carbonate) accounts for 95-99% by weight, with the remaining 1-5% representing an organic matrix (Marin and Luquet, 2004). Hence, calcium carbonate from shell waste could be exploited and valorized for a wide range of subsequent applications (Barros et al., 2009). Bio-polymers enriched with aquaculture waste (e.g., shrimp and shell waste) are being tested as potential substitutes to conventional plastics in the marine aquaculture industry (Kandra et al., 2012). However, despite increasing research efforts in developing more eco-friendly bio-polymers, little is known about their fate in the marine environment and their interactions with biological communities (Dussud et al., 2018a; Dussud et al., 2018b).
Considering the ever-increasing production, release, and impacts of MPD on marine ecosystems (Haram et al., 2021), we wanted to evaluate the differential recruitment and succession of marine micro- and macro-organisms onto custom-made biodegradable polymers enriched with oyster shells recycled from the aquaculture sector. Shells are used in oyster restoration programs, particularly as a substrate on which seed oysters are stocked, to build or rehabilitate oyster reefs (Peterson et al., 2003; Nelson et al., 2004). Hence, we sought to incorporate different concentrations of oyster shells into a bio-based polymer to study potential effects on marine biofoulers’ recruitment and investigate how bio-polymers enriched with oyster shell filler perform in marine conditions. We selected polybutylene succinate (PBS) as a base polymer to manufacture shell-enriched bio-polymers, since it is biodegradable and easy to process or handle while mixing with other materials, and able to degrade at high rates over short periods of time (Rafiqah et al., 2021). Thus, the general aim of this study was to investigate if these newly designed bio-polymers could eventually mitigate the biosecurity risks associated with MPD and provide a viable alternative to conventional polymers, particularly in the aquaculture sector.
In this controlled field exposure experiment, we used well-established high-throughput DNA sequencing and metabarcoding protocols to characterize, for the first time, microbial and eukaryotic assemblages associated with oyster-shell amended bio-polymers, including non-indigenous marine species (Wood et al., 2013; Zaiko et al., 2016; Pochon et al., 2017; von Ammon et al., 2018) and potential plastic degraders (Gambarini et al., 2021). We hypothesized that i) bacterial and eukaryotic communities will exhibit differential settlement preferences and temporal succession patterns on bio-polymers augmented with three different concentrations of oyster shell, and ii) putative marine non-indigenous species and bacterial taxa with plastic biodegradation potency will demonstrate specific affinities to particular bio-polymer types.
Methods
Description of Bio-Polymers and Extrusion
Four different types of PBS-based polymer sheets (15 cm × 15 cm) were manufactured by SCION™ (Rotorua, New-Zealand) and used as ‘polymer type’ treatments in the experiment (Figure 1A): (i) OYS20 – PBS containing 20% of oyster shell content; (ii) OYS40 – PBS containing 40% of oyster shell; (iii) OYS60 - PBS containing 60% of oyster shell; (iv) Control-PBS only, with no additives added. Polybutylene succinate (PBS, PTT MCC biochemical, grade FD92PM) was purchased from Chemiplas NZ (Auckland, New Zealand). Pellets were dried at 60°C overnight before use. Oyster shells were ground and sieved. Only the fraction between 125 and 250 µm was kept. The oyster shell powder was dried at 50°C overnight before use.
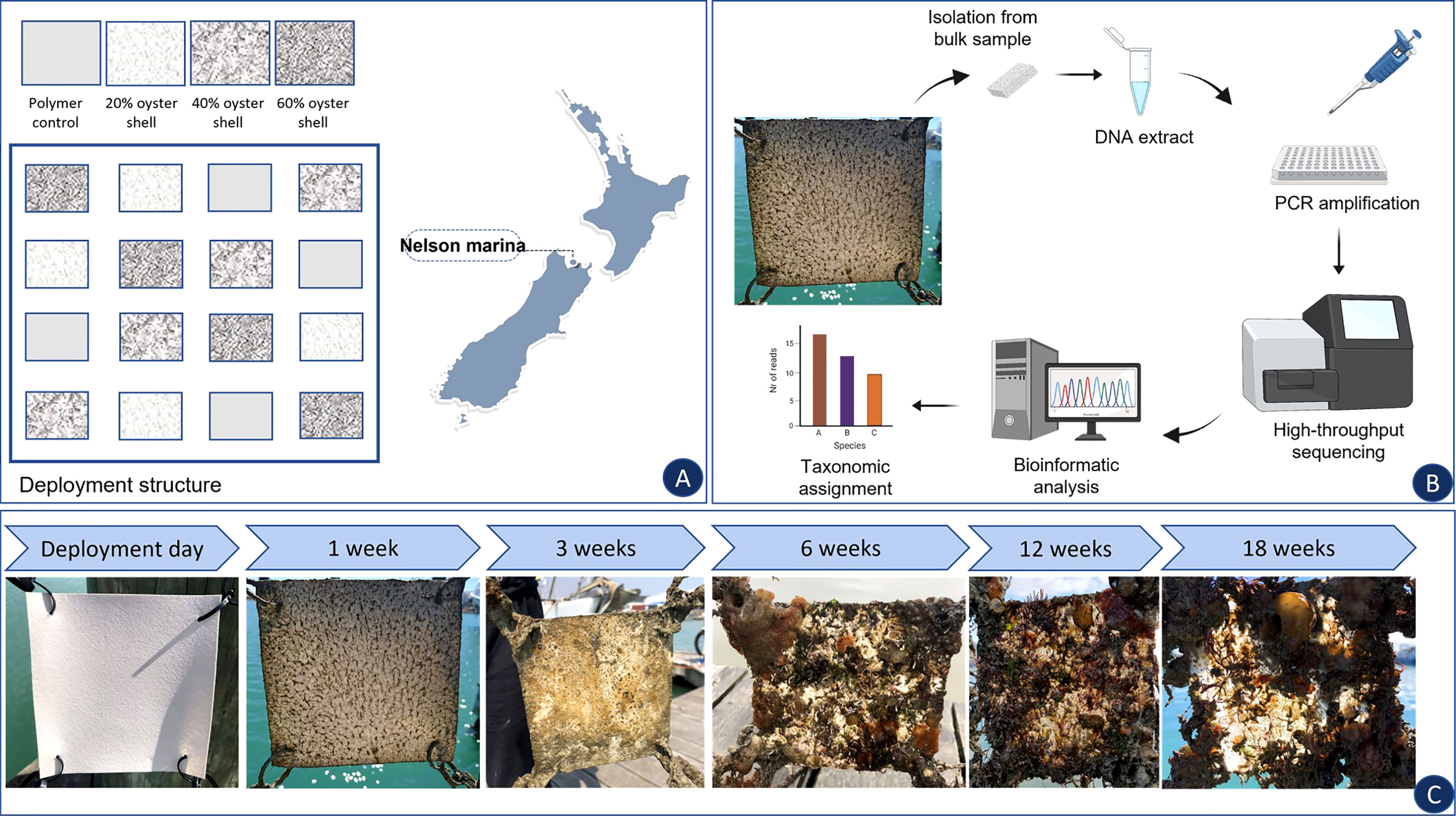
Figure 1 Experimental design and study location. (A) In total, three PVC frames with 12 bio-polymers sheets, comprising four polybutylene succinate (PBS) control sheets, four PBS sheets enriched with 20% of oyster shell filler, four PBS sheets enriched with 40% of oyster shell filler, and four PBS sheets enriched with 60% of oyster shell filler were deployed alongside floating pontoon at the study location; (B) Sample processing as described in section 2.3; (C) Sample collection with established sampling time points.
The extrusion process consisted of:
a. Compounding: Oyster shells (60 wt.%) and PBS compound was made using 900 g of oyster shell and 600 g of PBS hand mixed and placed into a volumetric feeder. The extrusion (i.e., a part or length of material formed by pushing material through a die with a particular cross-section) was carried out on a co-rotating twin-screw extruder (OMC, Saronno, Italy) with 19 mm diameter and L/D (length to diameter ratio) of 30:1 using a single strand die (4 mm diameter). The screw speed was 160 rpm and the temperature 150°C in all zones.
b. Calendering: Films were extruded and calendered (i.e., process used to smooth and thin the material) using the same extruder equipped with a fishtail film die and calendering rolls. The screw speed was set to 160 rpm. The extruder feed rate was between 7 - 9 rpm, torque 40-50%, and pressure 4-6 bar. The calendar speed was set to 20 rpm, and the rolls chilled to 20°C. Initially, the barrel and head temperatures were set to 130°C. Oyster shell formulations were blended at the appropriate loadings using the 60% oyster shell masterbatch (60OS-40PBS MB) and pure PBS (both dried overnight before use) to obtain OYS20, OYS40, and OYS60 films for the experiment.
Study Design
For deployment, three PVC structures (120 cm × 120 cm) were fabricated, each comprising polymer sheets attached to PVC pipes (Figure 1A). The sheets were arranged on the structure following a Latin square design, which allowed for randomization of the bio-polymer and control sheets across the structure (Gao, 2005). Experimental frames were deployed on a floating pontoon at 0.5 m depth (polymers remained submerged at the same depth throughout the experiment) in the Nelson Marina (Nelson, New Zealand) (S -41.26°; W 173.27°), on the 29th of January 2019, and the bio-polymer sheets were sampled 1, 3, 6, 12 and 18 weeks after deployment (Figure 1C) for environmental DNA (eDNA) analysis. Temperature data loggers (HOBO® Pendant temperature loggers, Onset, USA) were attached to each of the three deployment structures, and the temperature was recorded every 15 min for the duration of the experiment. The temperature at the study site ranged between 23.3°C in January 2019 and 13.7°C in May 2019 (Figure S1).
Sample Collection, DNA Extraction, and High-Throughput Sequencing
At each sampling time, three sheets of each bio-polymer (including the control) were collected (i.e., n = 12 sheets per sampling point). Biofouling isolation was undertaken in a non-destructive manner in the field, wearing sterile gloves for each sample. At each sampling time, a new polymer sheet was collected. On weeks one and three, early biofilm samples were collected using sterilized sponges (Whirl-Pak™, Speci-sponges™, Nasco, WI, USA) and thoroughly rubbing ¼ of the surface of the bio-polymer sheets on the front and back side separately. The sponge and collected material from each side were isolated into individually sealed sterile plastic bags provided by the same manufacturer. In the subsequent weeks (when mature biofouling has been established), sterile stainless-steel surgical blades (Swann-Morton, Sheffield, United Kingdom) were used to carefully detach biofouling from ¼ of the surface from the front and back side of the bio-polymer sheets. Each sample was then isolated into a sterile 50 mL centrifuge tube. Collected samples were transported on ice to the Cawthron Institute laboratory located 5 min away and processed immediately for DNA extraction Figure 1B.
In the laboratory, biofilm samples collected with sponges and blades were processed as described in Zaiko et al. (2016) and von Ammon et al. (2019), respectively. Briefly, RNA/DNA free water (40 ml; Life Technologies, Carlsbad, CA, USA) was added to each plastic bag containing a sponge sample, and the bag was crushed using a Colworth 400 laboratory stomacher press (Seward, Worthing, UK) for 2 minutes at maximum speed. Excess liquid was then squeezed from the sponges, and the resulting suspensions were pelleted by centrifugation (4,000 g, 15 min). Then, the supernatant was discarded, and pellets were used for DNA extraction. The blade harvested samples were homogenized by bead beating for 2 min (1,600 strokes/min; 1600 MiniG Spex SamplePrep), using stainless steel beads (SPEX™ SamplePrep Stainless-Steel Grinding Balls 3/8”, 9.5 mm diameter, Fisher Scientific, New Hampshire, United-States), centrifuged (15,000 g, 5 min) and the supernatant discarded. A single subsample of 0.2 g was taken from the homogenized material for subsequent DNA extraction.
DNA from all biofouling samples (n = 120) was extracted using the PowerSoil® DNA Isolation Kit (QIAGEN, MOBIO, Carlsbad, USA), following the manufacturer’s protocol. A DNA extraction blank was included for every 40 samples (three blanks in total). The quantity and quality of the extracted DNA were assessed using a NanoPhotometer (Implen, Munich, Germany).
The V3-V4 regions of the bacterial 16S ribosomal RNA (16S rRNA) gene and the V4 region of the eukaryote nuclear 18S ribosomal RNA (18S rRNA) gene were amplified by Polymerase Chain Reaction (PCR), using respectively the bacteria-specific primers 341F: 5’-CCT ACG GGN GGC WGC AG-3’ and 805R: 5’-GAC TAC HVG GGT ATC TAA TCC-3’ (Herlemann et al., 2011; Klindworth et al., 2013) and eukaryotic-specific primers Uni18SF: 5’-AGG GCA AKY CTG GTG CCA GC-3’ and Uni 18SR: 5’ -GRC GGT ATC TRA TCG YCT T-3’ (Zhan et al., 2013). Both primer sets contained an Illumina overhang adapter for NEXTERA indexing, as in Pochon et al. (2019). Polymerase Chain Reaction analyses were done in an Eppendorf Mastercycler (Eppendorf, Hamburg, Germany) in a total volume of 50 µL using MyFi™ PCR Master Mix (sample concentration ranging from 18.9 to 45.82 ng/µL) (Bioline Meridian Bioscience, Memphis, Tennessee, USA), 2 µL of each primer (10 mM stock), and 1 µL of template DNA. The PCR cycles for the 16S rRNA gene amplification were as follows: 95°C for 3 min followed by 33 cycles of 94°C (20 s), 52°C (30 s) and 72°C (30 s), with a final extension at 72°C for 5 min. Amplifications for the 18S rRNA gene were 95°C for 3 min followed by 37 cycles of 94°C (20 s), 54°C (20 s), and 72°C (30 s) with a final extension at 72°C for 7 min. Negative (no-template) PCR controls were included in each PCR run. Amplicon PCR products were purified using AMPure XP PCR Purification beads (Agencourt, Beverly, MA, USA), quantified using a Qubit Fluorometer (Life Technologies, Carlsbad, CA, USA), and diluted to 3 ng/µL. Water control was added to test for potential contamination during the sequencing workflow. Normalized PCR products and controls (n = 133; 120 samples, four extraction blanks, seven PCR blanks, and two water blanks) were sent for library preparation and sequencing on an Illumina MiSeq™ platform at Auckland Genomics, University of Auckland, New Zealand, following the Illumina 16S rRNA metagenomics library preparation manual (D’Amore et al., 2016). Sequencing adapters and sample-specific indices were added to each amplicon via a second round of PCR using a Nextera Index kit. After that, five μl of each indexed sample was pooled, and a single clean-up of pooled PCR products was undertaken. A bioanalyzer was used to check the quality of the library, which was then diluted to 4 nM and denatured. The library was diluted to a final loading concentration of 7 ρM with a 15% spike of PhiX. Paired-end sequences (2 x 250 bp) were generated on an Illumina MiSeq instrument. Raw sequences were deposited in the NCBI short read archive under accession: PRJNA811748.
Bioinformatics and Statistical Data Analyses
Raw sequence reads (with Illumina adapter sequences removed by sequencing facility) were trimmed using cutadapt v2.10 to remove primer DNA sequences (Martin, 2011), with no primer mismatch allowed. Then quality filtering, denoising, merging pair-end sequences, and calling amplicon sequence variants [ASVs] were performed using the DADA2 version 1.20.0 package, implemented in R version 4.0.5 (Callahan et al., 2016). Following exploration of the DNA sequence quality plots, the following parameters were applied for quality filtering and denoising for 16S rRNA and 18S rRNA datasets, respectively: “truncLen=c(220,220), maxEE=c(2,6), truncQ=2, ndmaxN=0” and “truncLen=c(150,150), maxEE=c(2,6), truncQ=2, ndmaxN=0”. Singleton ASV data were discarded, and the remaining paired-end reads were merged with a minimum overlap of 25 bp and one mismatch allowed in the overlap region. Chimera removal was performed using the default (consensus) method and the de-noised ASVs were taxonomically classified against the SILVA 132 database for 16S rRNA (Quast et al., 2012) and PR2 database for 18S rRNA (Guillou et al., 2012) using DADA2 “assignTaxonomy” command, based on the RDP classifier (Wang et al., 2007).
The 16S rRNA dataset was filtered to exclude any ASVs classified as Eukaryota in the rank Kingdom, Chloroplast in the rank Class, and Mitochondria in the rank Family using the “subset_taxa” command implemented in R package phyloseq (McMurdie and Holmes, 2013). The maximum number of ASVs found across negative controls was subtracted from the corresponding ASVs to offset potential contamination noise (Bell et al., 2019; Clark et al., 2020). Then, rarefaction curves were plotted for both 16S rRNA and 18S rRNA datasets using the ‘ggrare’ function in R (package ranacapa; Kandlikar et al. (2018)). The 16S rRNA rarefaction curves indicated that the sequencing depth attained per sample adequately captured biodiversity (i.e., the curves have reached a plateau). In the 18S rRNA dataset, four samples yielded an extremely low post-filtering number of reads (<2,000) and were removed from further analyses (Table S1). For calculating diversity metrics (ASV richness and Shannon index), metabarcoding data were normalized by rarefying at equal depth (10,000 and 2,000 reads for 16S rRNA and 18S rRNA data, respectively). The community structure analyses were performed on the unrarefied datasets transformed into proportional read abundance. Amplicon Sequence Variant (ASV) tables generated by our bioinformatic pipeline were uploaded into the Plymouth Routines in Multivariate Ecological Research (PRIMER 7) v7.0.13 software (Anderson, 2001; Clarke and Gorley, 2015). Permutational Multivariate Analysis of Variance (PERMANOVA) was used to investigate whether bacterial and eukaryotic community structure responds differently across experimental factors ‘Type’ (i.e., different bio-polymer concentrations), ‘Sampling’ (i.e., sampling time), and ‘Side’ (i.e., front or back of the bio-polymer sheets). Bray-Curtis dissimilarity matrices were calculated for bacterial and eukaryotic datasets (at ASV level) (Bukin et al., 2019). Bray-Curtis dissimilarity based on relative abundance of sequence reads was chosen as one of the most commonly used in metabarcoding studies (Bukin et al., 2019) and not affected by the high frequency of zero counts typical for HTS datasets (Pawlowski et al., 2016). Two-way permutational ANOVAs (PERMANOVA) with ‘Sampling time’ as a random factor and ‘Type’ as a fixed factor was performed to explore differences in bacterial and eukaryotic composition across experimental factors. Differences P ≤ 0.05 were deemed statistically significant (Bland and Altman, 1995). In addition, tests of homogeneity of dispersions (PERMDISP), were performed to quantify the variability in community structure between treatments for the bacterial and eukaryotic datasets, using "Sampling" as a group factor. 999 permutations and calculating distances to centroids. Variation in community structures among the five sampling times (1 week, three weeks, six weeks, 12 weeks, and 18 weeks) were ordinated using a Principal Coordinate Analysis (PCoA) for both bacterial and eukaryotic datasets to visualize distribution patterns across ‘Types’ and ‘Sampling.’ One-way analysis of variance (ANOVA) was performed on ASV richness and Shannon-Wiener index, separately on the bacterial and eukaryotic dataset for the group factors ‘Sampling’ and ‘Type’ using the ‘Vegan package in RStudio (Oksanen et al., 2013). Before that, Levene’s and Shapiro-Wilk’s tests were used to confirm that assumptions for normality and heterogeneity are met. Differences at P ≤ 0.05 were deemed statistically significant. Following ANOVA analysis, Tukey’s honestly significant test (Tukey’s HSD) was performed in RStudio to test differences for alpha diversity indices among sampling times with the R package agricolae. Additionally, statistical related differences in the bacterial and eukaryotic communities’ composition were investigated using a pairwise PERMANOVA design. The ten most abundant phyla (bacteria) and class (eukaryote) across datasets related to the ‘Type’ and ‘Sampling’ factors were visualized using bar plots based on standardized, average abundance across replicates.
Non-Indigenous Species (NIS) Analysis
A list of New Zealand marine NIS was assembled based on the inventory by Statistics New Zealand (https://www.stats.govt.nz/indicators/marine-non-indigenous-species). This list was used to compile relevant reference DNA sequences from the nucleotide collection of the National Centre for Biotechnology Information [NCBI]. The 18S rRNA gene sequences were then aligned against this curated dataset using megablast from Blastn application, and the best match results (99% minimum identity threshold) were retained. The resulting subset of the 18S rRNA gene dataset was used for assessing the polymer type effect on the relative abundance of putative NIS using one-way ANOVA. The relative abundance of the ten most contributing NIS was visualized across ‘Type’ and ‘Sampling’ factors using barplots generated with PRIMER 7.
Analysis of Putative Bacterial Plastic Degraders
Taxa reported in the literature to biodegrade plastics were identified using the Annotate Taxa Table tool on the PlasticDB web application (Gambarini et al., 2022). Taxon names were matched to the NCBI taxonomy database (Schoch et al., 2020), and NCBI tax IDs were extracted using the ETE3 toolkit software (Huerta-Cepas et al., 2016). The same software was also used to extract a taxonomic tree topology from NCBI. A python script was developed to conduct taxonomic nomenclature conversions and generate the phylogenetic tree annotation files. For visualization, the tree and its annotation files were uploaded to the iTOL web application (Letunic and Bork, 2021). The relative abundance of the ten most detected bacterial degraders families was visualized across ‘Type’ and ‘Sampling time’ factors using barplots generated with the phyloseq and ggplot2 package in Rstudio.
Results
Over the observation period, the bio-polymers gradually lost structural integrity. Deterioration (i.e., holes, breakages) was observed on all types of bio-polymers (i.e., bio-polymers enriched with increasing concentration of oyster shell) on week-6 after deployment. Metabarcoding analysis of the bacterial 16S rRNA gene V3-V4 regions and eukaryotic 18S rRNA gene V4 region revealed 11,817,258 and 12,344,573 raw sequence reads, with an average number of sequences per sample of 95,527 ± 42,479 for bacteria and 102,872 ± 87,549 for eukaryotes, respectively (mean ± standard error). Following quality filtering (merging, dereplication, and denoising), a total of 6,005,855 bacterial and 5,246,791 eukaryotic sequence reads were obtained, representing 123,778 and 4,225 ASVs, respectively (see Table S1).
Variation in Microbial Community Composition and Diversity Overtime on PBS Containing Different Concentrations of Oyster Shell
Comparison of relative alpha diversity indices for the bacterial dataset indicated significant shifts across sampling times (relative ASV richness and Shannon Index, both p ≤ 0.01, One way ANOVA) (Table S2). The three-week samples yielded the highest relative bacterial diversity estimates (Table S2 and Figure 2), significantly different from other sampling times for ASV richness (Tukey’s HSD test p ≤ 0.01) and from weeks 12 and 18 samples for Shannon diversity (Tukey’s HSD test p = 0.006 and p = 0.0008, respectively). The lowest overall bacterial ASV richness was observed for 18-weeks samples, significantly less than that during sampling weeks 1-6 (Tukey’s HSD p ≤ 0.0001).
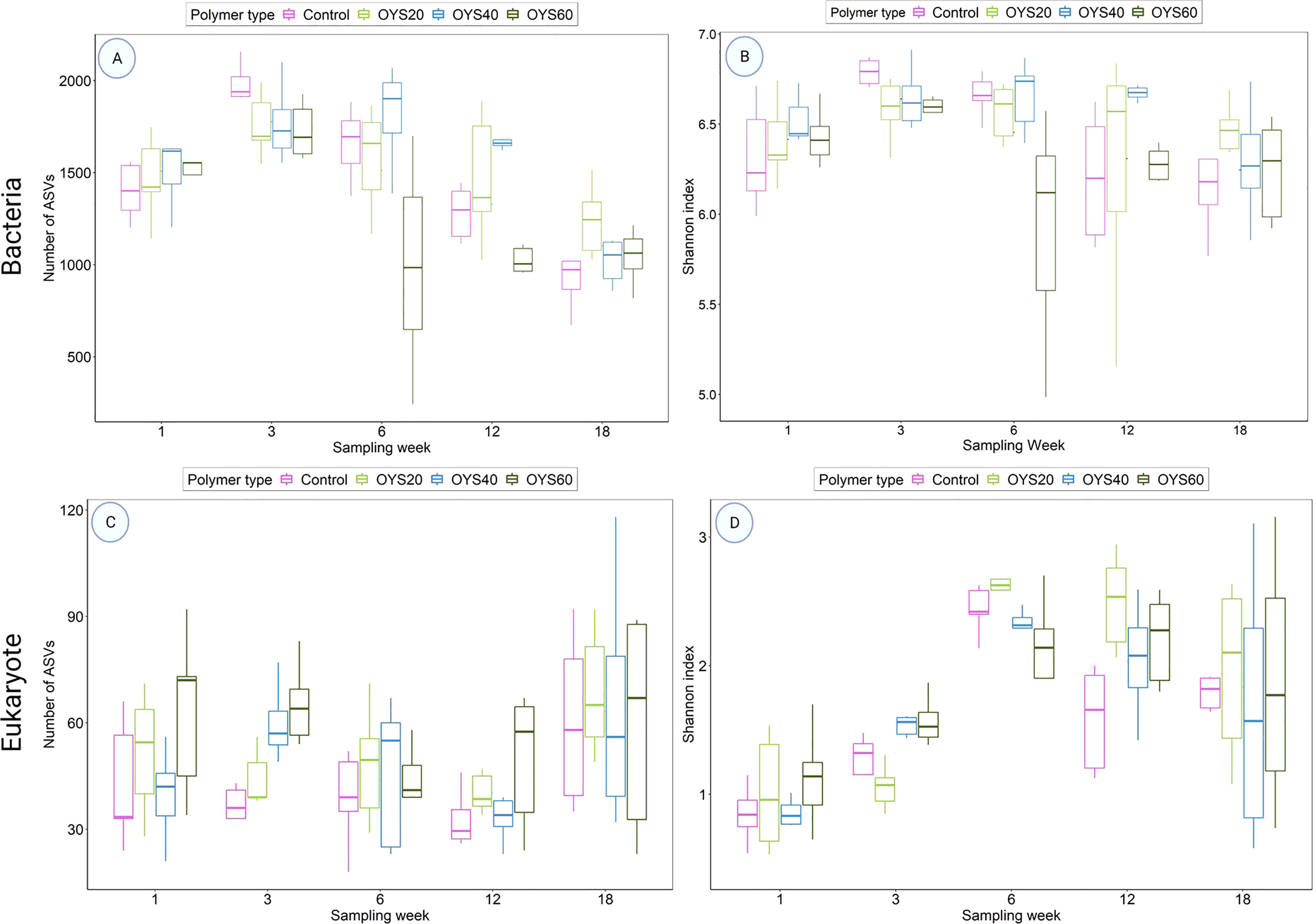
Figure 2 Boxplots of Amplicon Sequence Variants (ASVs) number (A, C) and Shannon index (B, D) comparing bio-polymers and control between sampling times in bacterial (top) and eukaryote (bottom) datasets. The boxes denote interquartile range (IQR), with the median as a black line and whiskers extending the most data extreme points.
Principal coordinate analysis (PCoA) displayed a clear temporal shift in the bacterial community structure (Figure 3A), with increasing dispersion towards the later sampling times (i.e., week-6 and later) and convergence of bacterial communities at the two latest sampling time points (weeks 12 and 18). PERMANOVA analysis confirmed the significant effect of time (i.e., ‘Sampling time’) (p ≤ 0.001). Besides, neither the effect of the ‘Side’ factor (p = 0.458) nor the marginal effect of the ‘Type’ factor (p = 0.0804) on bacterial community structure was detected (Table S3). A test of homogeneity of dispersion (PERMDISP) on the bacterial dataset revealed significant differences in the average distances to centroids among samples grouped by sampling times (p= 0.0016, F = 5,4394, PERMDISP), supporting the observed time-related increasing dispersion in PCoA plots.
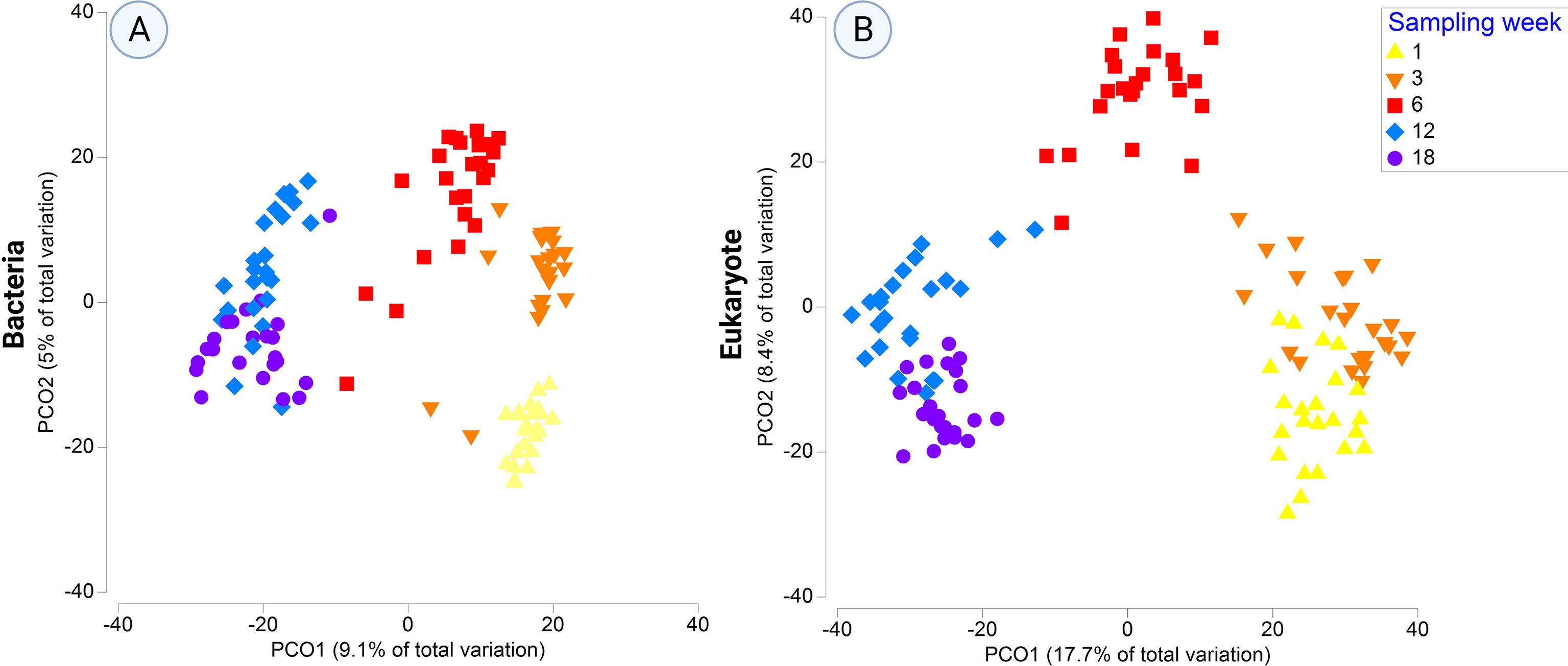
Figure 3 Principal Coordinates Analysis (PCoA) plot of (A) bacterial 16S rRNA gene and (B) eukaryotic 18S rRNA gene data, based on fourth-root transformed data and Bray-Curtis dissimilarity matrix. Colors represent the various sampling weeks.
In the eukaryotic dataset, a comparison of alpha diversity indices revealed significant changes across sampling time points for both ASV richness and Shannon index (One way ANOVA p ≤ 0.001) (Table S2). The highest diversity estimates were observed in the 6-week samples, differing significantly from 18-week samples based on ASV richness (Tukey’s HSD test p ≤ 0.005), and from week-1, week-3, and week-18 samples based on Shannon index (Tukey’s HSD test p ≤ 0.005). Minimum diversity was observed in the week-1 samples, with significant differences in ASV richness compared with week-18 samples (p ≤ 0.05) and significant differences among week-6, week-12, and week-18 samples based on Shannon index values (p ≤ 0.0001).
Similar to the bacterial dataset, PCoA revealed clear shifts in eukaryotic community structure for the ‘Sampling time’ factor (Figure 3B). Eukaryotic communities from the first two sampling weeks are closely related to each other and are distinct from other sampling time points, followed by a noticeable shift in community composition for week-6 samples. Eukaryotic communities at weeks 12 and 18 are most closely related and overlap in the multivariate space. PERMANOVA analysis further confirmed significant differences in samples distribution for the ‘Sampling’ factor (p ≤ 0.001), whereas no statistical differences were found for the ‘Side’ (p = 0.225) or ‘Type’ (p = 0.1191) factors (Table S3). Statistical analysis of community dispersion (PERMDISP) also revealed significant differences in homogeneity of dispersion for the factor ‘Sampling’ (p= 0.001, F = 23,796, PERMDISP).
Differential Succession of Bacterial and Eukaryotic Assemblages on PBS Containing Different Concentrations of Oyster Shell
The overview of taxonomic succession on the substrates revealed higher temporal consistency in the bacterial community data across the tested bio-polymers compared with eukaryotes (Figure 4). Proteobacteria and Bacteroidetes were the most abundant phyla in the bacterial dataset regardless of sampling time or concentration of oyster shells, comprising 66.7% and 20.1% of the overall community, respectively. The high-level taxonomic composition of the bacterial assemblage was similar across polymer types for the first two sampling time points (i.e., weeks 1 and 3), with some changes observed in week-6 samples, with a 3-6% increase in the relative abundance of Epsilonbacteraeota and Firmicutes in the OYS40 and OYS60 treatments. This observation is consistent with the type-related statistical differences reported in the pairwise PERMANOVA analysis, with significant statistical differences in community structure observed between the OYS40 and OYS60 week-6 samples (Table S4 and Figure 4). At the two latest sampling times (i.e., weeks-12 and -18), bacterial community composition was similar regardless of polymer types. A minor change was observed in the control and OYS20 samples at 18 weeks, where the contribution of Actinobacteria was sensitively higher compared to other polymer types (Figure 4).
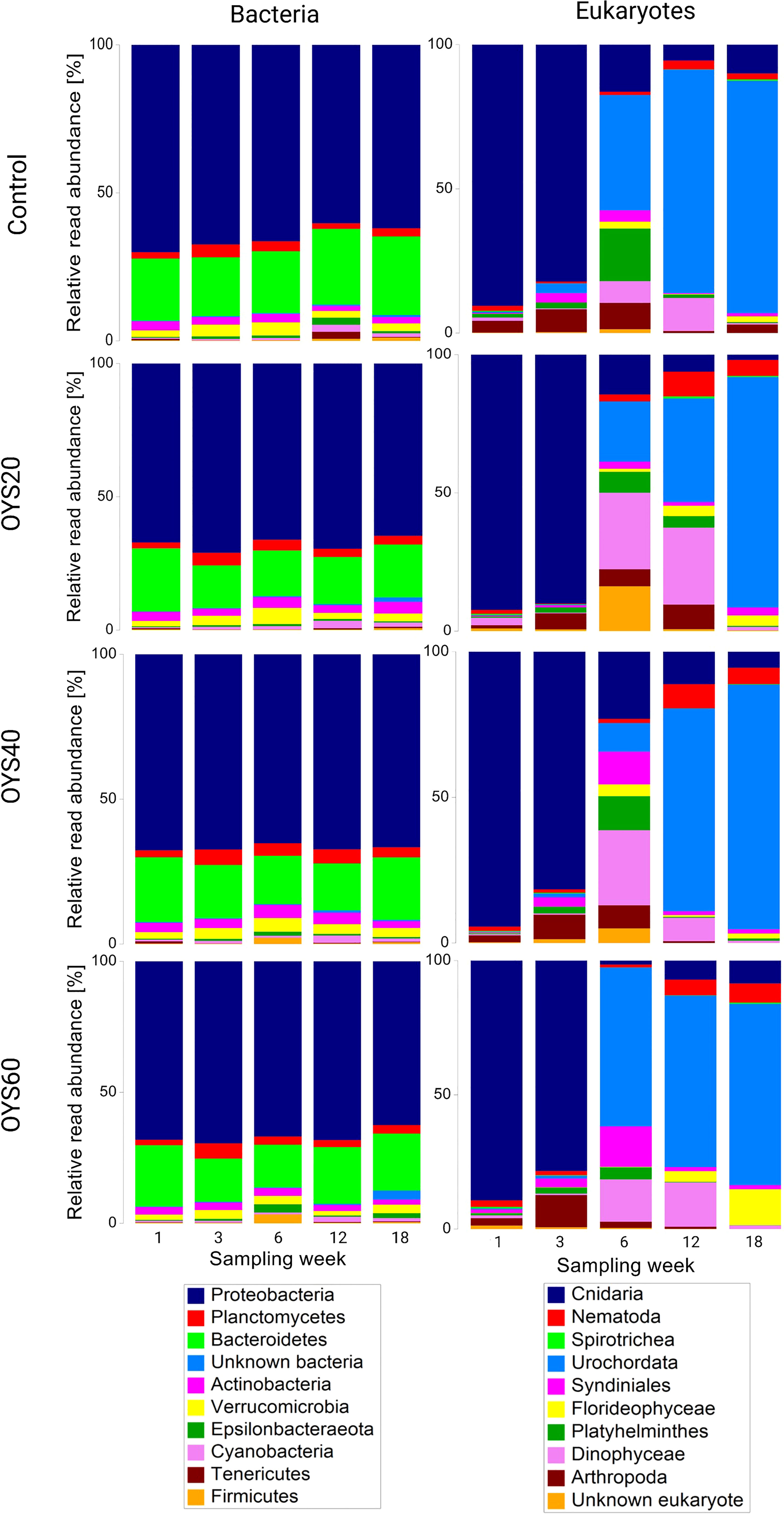
Figure 4 Relative read abundance of the ten most dominant bacterial phyla (left) and eukaryotic classes (right) among the different treatments (Sampling times and Polymer type).
The eukaryote dataset was dominated by Metazoa (84.1% of the overall read abundance). Among the ten most-represented classes (Figure 4), the majority were common biofoulers: Cnidaria (40.5%), Urochordata (35.1%), and algae (e.g., Florideophyceae, Dinophyceae, 12.3%). On week-1 and week-3, Cnidaria dominated eukaryotic assemblages in all treatments, comprising 83%-91.6% of the overall read abundance, and with a noticeable increase in Arthropoda (8.5%) on week-3. The greatest variation in community structure (at the ASV level) across bio-polymer types was detected on week 3, as indicated by the pairwise PERMANOVA analyses (Table S2). On week-6, a clear type-dependent shift in dominant taxa and community structure was observed. Thus, the control samples were dominated by Urochordata (40%), with Cnidaria, Arthropoda, and Plathelminthes comprising a substantial portion of the community (16.4%, 9.1%, and 18.2%, respectively). The OYS20 were dominated by Dinophyceae (27.7%), Urochordata (21.7%), unknown eukaryotes (16.2%), and Cnidaria (14.4%). In the OYS40 treatment, there was a notable decrease in Cnidaria’ relative abundance on week 6 (from 81.6% at week-3 to 23.1% at week-6), as well as an increasing contribution of Dinophyceae, Urochordata, Plathelminthes, and Syndiniales, 25.8%, 9.8%, 11.7%, and 11.2%, respectively. At this time, a substantial proportion of the eukaryotic community in the shell-enriched treatments (OYS40 and OYS60) was comprised of undertermine Syndiniales, a diverse parasitic group of Dinoflagellates (11.2% and 15.1%, respectively). On week–6, tunicates became dominant on OYS60 (59.2%), and later their relative contribution increased across all polymer types, peaking on week-18 in the control and OYS20 treatments (80.6% and 83.4%, respectively). In the latest weeks, the most pronounced difference between bio-polymer treatments was a comparatively higher contribution of red algae (Floridophyceae) in the OYS60 (from 3.9% to 13.4% on week-12 and -18, respectively) and a lower contribution of Nematoda in the control samples (from 3.1% to 2.1% on week-12 and -18 respectively). At the ASV level, no significant difference in eukaryotic community structure was detected between polymers at week-18 (Table S4).
Recruitment of Non-Indigenous Species on Bio-Polymers
From the eukaryote dataset, 344 ASVs were assigned with high confidence (>99% identity) to 33 putative NIS (species or genus level), contributing up to 87.7% of the total sequence abundance at the first sampling time point. Overall, putative NIS dominated eukaryotic assemblages across all sampling time points (Figure 5). Among these, tunicates (e.g., Ascidiella sp. Botryllus planus, Ciona intestinalis, Ciona savignyi, Clavelina meridionalis, Styela plicata) were the most prevalent, followed by red algae (e.g., Heterothamnion sp., Neosiphonia harveyi, Polysiphonia schneideri), green algae (Chaetomorpha crassa, Ulva sp. Cladophora glomerata) sponges (Hymneniacidon sinapium, Leucosolenia sp. Mycale sp.) and polychaete worms (Spirobranchus caraniferus, Pomatoceros lamarckii) (see Table S5). One-way ANOVA revealed a significant effect of the ‘Sampling’ factor (p = 0.0001) on NIS relative read abundances but no effect of the ‘Type’ factor (p = 0.242). However, there was an apparent difference in relative sequence read contribution of individual NIS between bio-polymer treatments. Thus, following week-1 and week-3 timepoints during which eukaryotic communities were heavily dominated by the invasive hydrozoan Bougainvillia sp., the total NIS contribution decreased substantially across all bio-polymer types. This change coincided with the overall community shift mentioned above and was characterized by a higher divergence in NIS contribution across polymer treatments (Figure 5). Thus, on week-6, C. intestinalis and Bougainvillia sp. comprised a substantial part of the control and OYS20 communities (25.8% and 15.1% for C. intestinalis and 8.2% and 10.5% for Bougainvillia sp., respectively). The OYS40 samples were characterized by a high contribution of Bougainvillia sp. (20.3%) and C. intestinalis (7.1%). In the OYS60 treatment, invasives tunicates S. plicata and C. intestinalis contributed up to 52% of the overall diversity. On week-12, the control assemblage again became dominated by NIS, with B. planus, S. plicata, and C. intestinalis comprising 49.3%, 13.7%, and 9.8%, respectively (Figure 5). Interestingly, the relative contribution of invasive tunicates increased over time across all bio-polymer treatments, particularly at the two latest sampling time points (i.e., 12 and 18 weeks), with B. planus being the most abundant, followed by S. plicata and C. intestinalis. The red algae N. harveyi was exclusively detected in week-18 OYS60 samples, contributing 12.1% of the entire diversity (Figure 5).
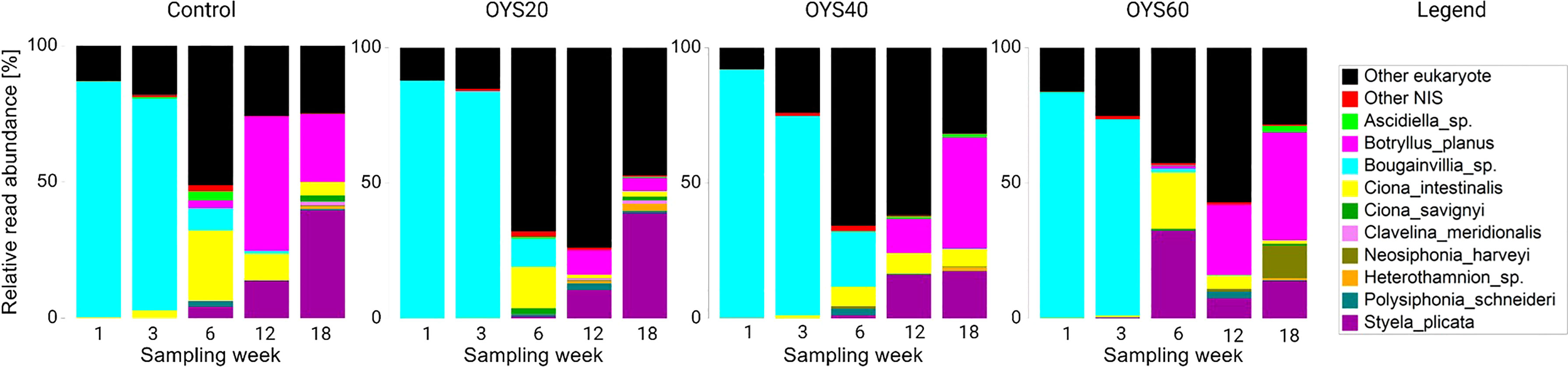
Figure 5 Relative read abundance of non-indigenous species (NIS) and other eukaryotes per sampling week and polymer type. Only the ten most abundant NIS are shown for clarity; NIS with relatively low abundance were pooled in the ‘Other NIS’ category. See Supplementary Table S5 for a detailed list of NIS.
Recruitment of Bacterial Families With Putative Plastic-Degrading Capabilities
In total, 41 bacterial families belonging to the phyla Proteobacteria, Firmicutes, Actinobacteria, and Bacteroidetes were identified with potential plastic-degrading capabilities in the bacterial dataset. Our analysis shows that within this dataset, Proteobacteria was the most frequently detected bacterial phylum of plastic degraders, comprising Gammaproteobacteria (54.1%), Alphaproteobacteria (16.7%), Betaproteobacteria (16.7%), Deltaproteobacteria (8.3%). Output from the PlasticDB tool revealed six families reported to degrade PBS: Streptomycetaceae (Actinobacteria); Thermoactinomycetaceae, Paenibacillaceae, and Bacillaceae (Firmicutes); Comamonadaceae and Pseudomonadaceae (Proteobacteria). In addition, the remaining 35 bacterial families detected in the dataset were reported to degrade other types of plastics (Figure 6 and Figure S2). Among the taxa reported to degrade PBS, we observed that Streptomycetaceae was exclusively associated with shell-enriched bio-polymers. All five other families reported to degrade PBS were detected in all bio-polymer samples, both shell-enriched and controls. Among the families that have been identified by the PlasticDB tool as having species reported to degrade other plastic types: 32 families were detected in all shell-enriched and control samples; Six families were detected only in shell-enriched samples (OYS20, OYS40, and OYS60), and three families were detected in the control samples and some but not all shell-enriched samples (i.e. Sphingobacteriaceae and Halomonadaceae detected in control, OYS20, and OYS40 samples; Caulobacteraceae detected in control, OYS40 and OYS60 samples) (Figure 6). PERMANOVA analysis revealed a significant effect of the factor ‘Sampling’ (p = 0.001) on the relative read abundance of putative bacterial plastic degraders, but no effect of the ‘Type’ factor (p = 0.085).
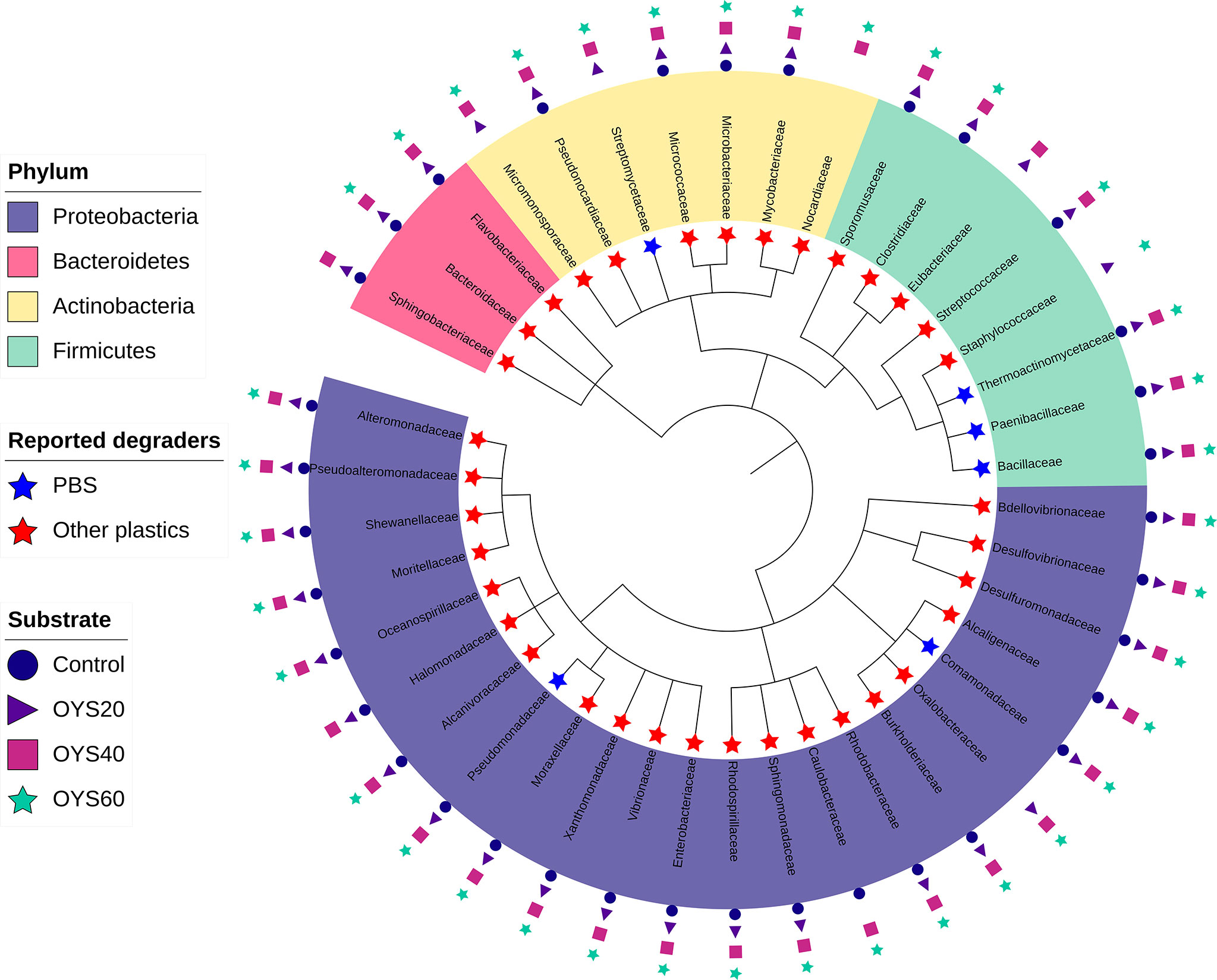
Figure 6 Phylogenetic tree representing all bacterial families detected in this study which previously have been identified as having potential plastic-degrading capabilities. The taxa with reports of plastic degradation were identified using the PlasticDB web server (http://www.plasticdb.org/), and the phylogenetic relationship among the shown taxa was extracted from the NCBI taxonomic database (Schoch et al., 2020). Leaves are colored according to their corresponding phyla. Blue stars represent bacterial families reported to degrade polybutylene succinate (PBS), red stars represent bacterial families reported to degrade other plastics. Icons highlighted on the rings external to the tree represent the substrates associated with bacterial families with plastic-degrading capabilities.
The community change of putative plastic-degrading bacterial families across polymer types revealed a similar pattern for the control, OYS20, and OYS40 samples (Figure 7). On week-1, we observed a substantial contribution of putative degraders, followed by a progressive decline on weeks 3 and 6. Interestingly, the temporal evolution was different in the OYS60 treatment, with a notable increase in the contribution of putative bacterial plastic degraders from week-1 to week-3. However, a general decline was observed on week six across all polymer types, coinciding with the major community shift described above. At the family level, Illumatobacteraceae was the most persistent bacterial family overall, being detected in all polymer types and across all sampling time points. The second most abundant family was Flavobacteriaceae, particularly during the first two sampling time points, with an increased contribution on week-3 and a substantial drop later on (particularly in OYS60 treatment). The family Spongiibacteraceae was exclusively detected during the first two sampling time points across polymer types, contributing up to 30% of the overall diversity in the control, OYS20, and OYS40 treatments on week 1, decreasing afterward. In the OYS60 samples, the relative contribution of Spongiibacteraceae increased from week-1 to week-3. The relative contribution of the Kordiimonadaceae increased towards the later sampling time in the control while being underrepresented in the shell-enriched treatments.
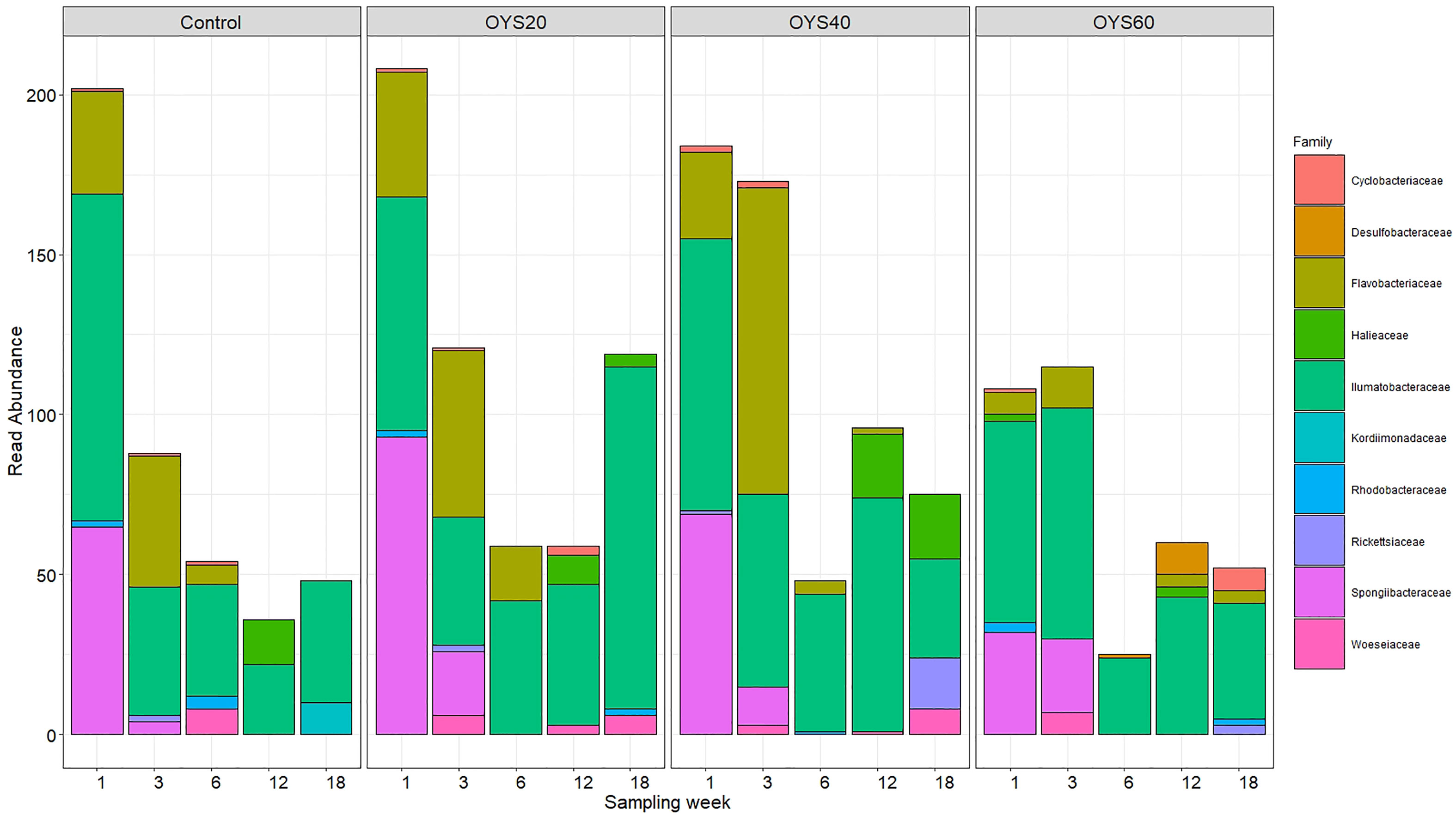
Figure 7 Read abundance of putative bacterial plastic-degrading families per sampling weeks and polymer types.
Discussion
Temporal Succession of Bacterial and Eukaryotic Assemblages on Bio-Polymers
Consistent with several earlier studies (Oberbeckmann et al., 2014; Zaiko et al., 2016; Oberbeckmann and Labrenz, 2020), our results revealed strong temporal patterns associated with both bacterial and eukaryotic community structure on all tested bio-polymers. The period of sample immersion in seawater was more important in shaping biofouling assemblages than the concentration of oyster shell filler, as evidenced by finding similar biological communities between oyster shell-enriched substrates and controls. Most studies that have reported plastic-specific recruitment (Zettler et al., 2013; Oberbeckmann et al., 2018; Hou et al., 2021; Martínez-Campos et al., 2021) have focused on a variety of chemically-distinct polymers (e.g., polystyrene, polyethylene, and polypropylene). Our experiment used the same base polymer (PBS) to manufacture control and treatment substrates. This might explain the lack of high-level taxonomic differentiation between bio-polymer treatments (Lakshmi et al., 2012; Muthukrishnan et al., 2019). However, differences in community structure between substrates were detected at a finer temporal scale, with significant community shifts identified on week-3 for eukaryotes and week-6 for bacteria (Table S4).
Consistent with other studies (Martell et al., 2018; Enrichetti et al., 2021), hydrozoans (cnidarians) acted as primary colonizers, dominating eukaryotic assemblages on all substrate types at the first two sampling time points. In week-3, differences between bio-polymers were mainly driven by the differential abundance of arthropods, ascidians, and parasitic Syndiniales (Figure 4). The community shift in the eukaryotic assemblage could be explained by the gradual increase of biomass on the bio-polymers, which in turn favored the recruitment of macrofoulers (Qian et al., 2007; Dussud et al., 2018a; Dussud et al., 2018b; Abed et al., 2019). In addition, we observed a slight increase in parasitic dinoflagellates (Syndiniales) relative abundance at week-3. Parasites in the order Syndiniales are widespread in marine environments and can infect several types of marine eukaryotes, including crustaceans, dinoflagellates, and cnidarians (Lee et al., 2008; Elifantz et al., 2013; De Tender et al., 2015). Altogether, our results suggest that the significant changes in eukaryotic community composition among substrates at week-3 were dictated by the progressive stabilization of the biofouling assemblage, together with the recruitment of fast-growing macro-invertebrates.
Proteobacteria and Bacteroidetes were the most dominant phyla across sampling time points in the bacterial dataset. Consistently with our findings, previous studies have shown that Proteobacteria act as pioneers in the early stage of biofilm formation in the marine environment, while Bacteroidetes abundance increase over time, acting as a secondary colonizer (Lee et al., 2008; Elifantz et al., 2013; De Tender et al., 2015). While previous reports have highlighted the dominance of Alphaproteobacteria colonizing plastic particles (mainly polyethylene, polypropylene and polystyrene) in the Atlantic Ocean (Debroas et al., 2017), and the Mediterranean Sea (Dussud et al., 2018a; Dussud et al., 2018b), our results revealed that Gammaproteobacteria was the dominant class in the bacterial dataset. Specific polymer-type effect or different study location could potentially explain these differences (Oberbeckmann et al., 2016). Overall, the bio-polymer type-related differences in the bacterial community composition were much more subtle than eukaryotes. The biggest shift observed on week-6 was mainly associated with was associated with increased Epsilonbacteraeota and Firmicutes in shell-enriched treatments. Interestingly, Clostridium and Arcobacter genera, which respectively belong to Firmicutes and Epsilonbacteraeota, have been described in the gut microbiome of the invasive tunicate C. intestinalis, potentially contributing to its invasiveness (Utermann, 2021). Moreover, the Arcobacter genus, which contain species with the potential for plastic degradation and pathogenesis has recently been reported in high abundance on microplastic particles in effluent sewage and estuarine environments (Kelly et al., 2021). Therefore, we assume that the increased contribution of these two bacterial taxa is either linked to high abundance of C. intestinalis detected on week-6, or could be associated with unidentified environmental sources, given the fact that the experiment was conducted in area exposed to environmental pollution.
Other finer-scale differences observed in bacterial communities between bio-polymers could be explained by the likely dominance of bacterial taxa associated with specific eukaryotic biofoulers. Marine invertebrates are holobionts: a functional ecological unit associating the host and its various microbial communities, providing host-beneficial functions (Hoffmann et al., 2009; Thompson et al., 2015). For example, numerous studies identified Proteobacteria and Bacteroidetes as dominant phyla associated with the ascidian microbiome (Erwin et al., 2013; Dror et al., 2019), cnidarians (Brown et al., 2017), and crustaceans (Datta et al., 2018). Hence, the recruitment of particular eukaryotic taxa and change in their relative abundance through time might be directly linked with the change in relative abundance observed in bacteria (Utermann et al., 2020), disguising, to some extent, bio-polymer-related changes in bacterial community composition.
Altogether, our results revealed a highly dynamic assemblage of both bacteria and eukaryotes at the early sampling time points (1-6 weeks). Two months after deployment (i.e., from sampling week-12 onwards), bacterial and eukaryotic communities became more stable, likely indicating the establishment of mature biofouling, significantly different from the early colonization (biofilm) stage. In an earlier study, Pollet et al. (2018) reported an increase in the stability of microbial community composition associated with plastic deployed in a coastal environment after 28 days of immersion. The authors suggested that plastic-associated biofilms might reach a ‘steady state’ over time and hypothesized that the temporal stability of bacterial communities in later succession stages could be explained with the capture of bacterial biofilms developed at the surface of macro-organisms colonizing the substrates (Pollet et al., 2018). Our results support these conclusions and indicate that in the context of the New Zealand coastal environment, the bacterial community associated with tested bio-polymers became more stable between 6 and 12 weeks after immersion.
Recruitment Patterns of Non-Indigenous Species on Bio-Polymers
Putative NIS dominated the eukaryotic assemblages across all sampling time points and bio-polymer types, with tunicates, algae, sponges, and polychaete worms being the most prevalent NIS taxa (see Table S5). These species are usual ‘inhabitants’ of the artificial substrates around port and marinas and were previously reported from the study area (Fletcher et al., 2017; Hawes et al., 2018). Ports and marinas are well-known gates for vessel-mediated biological invasions (Ardura et al., 2015; Borrell et al., 2017; Miralles et al., 2018; Floerl et al., 2021) and hotspots of the local NIS populations (Seebens et al., 2013; Lyons et al., 2020).
Previous studies have shown that the location and timing of introducing a new substrate into the marine environment can considerably affect the recruitment and succession pattern of biofouling assemblages (Jenkins and Martins, 2010; Zaiko et al., 2016). In the present study, bio-polymers were deployed during the summer-fall months (i.e., between January and May), corresponding to the spawning season of many non-indigenous marine invertebrates in New Zealand (O’Driscoll et al., 2003). Hence, the increase of propagules in the water column during this time of the year, coupled with the high abundance of NIS in the study area, might explain the dominance of non-indigenous metazoan detected in the eukaryotic dataset. Moreover, marinas are typical hotspots for the establishment and subsequent spread of non-indigenous species due to the proximity of introduction pathways (recreational watercraft, affinity to shipping ports) and availability of artificial habitats (floating pontoons, ropes, and buoys) suitable for the incursion of biofouling taxa (Afonso et al., 2020). NIS usually exhibit more “opportunistic traits” than native species (e.g., short lifespan, fast growth rates, high fitness); hence, NIS are often more successful than native species in pioneering and establishing on artificial substrates (Ferrario et al., 2017; Quell et al., 2021).
While previous studies reported diatoms (Mincer et al., 2016), Arthropoda (Delacuvellerie et al., 2021), fungi (Kettner et al., 2017), and ascidians (Vieira et al., 2018) as pioneer colonizers of artificial surfaces in the marine environment, our results show that Cnidaria, and particularly the invasive hydroid of the Bougainvillia genus, was the main taxon detected in the eukaryotic dataset during the early stages of recruitment (week-1 and -3). Bougainvillia’s explosive growth could have been favored by the chemical cues from the bio-polymers (Wyatt et al., 2005; Grey et al., 2018) and/or the lack of competition during the early stage of colonization (Rinkevich and Sakai, 2001; Lindquist, 2002). Although members of the family Bougainvilliidae are found worldwide (Vannucci and Rees, 1961), the genus has been described as colonizing artificial surfaces in coastal waters (mostly ports and marinas) from the South Pacific Ocean, particularly in Australia (Wyatt et al., 2005; Grey et al., 2018), and New-Zealand (Schuchert, 1998; Cairns et al., 2009). A recent metabarcoding study of biofouling from submerged surfaces (buoys, ropes, tires, and pontoon structures) in Tahiti revealed the highest relative abundance of Bougainvillia among detected NIS (Pearman et al., 2021). Comparison of the seasonal recruitment of fouling hydroids on settlement panels between cold and warm months in Brazil, Migotto et al. (2001) revealed that Bougainvillia spp. tend to dominate during the warmer months. Similar to our observations, they reported a low persistence of established colonies reflected by a sharp decline during the second month of immersion.
During the observed community shift on week-6, total NIS contribution decreased substantially across all polymer types, particularly in OYS20 and OYS40 treatments, where other eukaryotes became dominant. Interestingly, species richness in OYS20 and OYS40 treatments was slightly higher than other polymer types. Higher NIS contribution in the control and OYS60 samples probably results from the presence of key taxa, potentially depressing species richness once established on the substrate. For example, Blum et al. (2007) compared the species richness between panels from which C. intestinalis was experimentally removed and panels with naturally dense populations in San Francisco Bay, revealing that species richness was negatively correlated with the tunicates abundance. The authors suggested that many species were occurring more often in the absence of C. intestinalis (e.g., Styela spp. and Didemnum spp.). In contrast, other species (e.g., Botrylloides spp.) occurred more often on C. intestinalis-dominated assemblages. Our results support these observations and indicate that a higher contribution of C. intestinalis was found in Control and OYS60 treatments, compared with OYS20 and OYS40.
In general, the relative abundance of invasive tunicates increased over time across all substrates, particularly on weeks 12 and 18. Botryllus planus was the most abundant among tunicates, followed by Styela plicata and C. intestinalis. Interestingly, the contribution of B. planus was somewhat related to the oyster shell concentration in bio-polymers (Figure 5). As previously observed by Dalby Jr and Young (1993), B. planus often occurs on oyster farms, potentially impacting their growth rate. In addition, the clubbed tunicate S. plicata is often reported forming dense clumps on any submerged surfaces, including shells of the commercially important oyster Crassostrea gigas (Rodriguez and Ibarra-Obando, 2008; Marins et al., 2010). In our experiment, this tunicate seemed to recruit earlier on OYS60 bio-polymers, suggesting a specific affinity of this species to the shell-enriched substrate. Similarly, the invasive tunicate C. intestinalis has been described as a significant fouling species in shellfish farms, being able to colonize nearly 100% of the available substrate (Howes et al., 2007; LeBlanc et al., 2007). Today, C. intestinalis represents a major problem for mussel farmers on the Atlantic coast of Nova Scotia (Clancey and Hinton, 2003). Nevertheless, there was no evidence of C. intestinalis preferring the shell-enriched substrates, possibly due to interspecific competition with other colonial ascidians. For example, Paetzold et al. (2012) reported lower recruitment of C. intestinalis on aquaculture gear when other colonial Botryllus spp. tunicates were present. The authors hypothesized that pre-settled substrates might impede the recruitment of C. intestinalis through competition for space or release of negative settlement cues produced by other colonial ascidians. In this study, B. planus dominated the eukaryotic assemblages at 12 and 18 weeks, particularly on shell-enriched bio-polymers (i.e., OYS40 and OYS60), likely repulsing the attachment of C. intestinalis through chemical deterrence of the tunicate larvae (Krug, 2006).
Recruitment Patterns of Bacterial Degraders on Bio-Polymers
Bacterial bio-degraders play a key role in the natural decontamination and bioremediation of environmental contaminants (Devi et al., 2016). Organisms proposed capable of plastic biodegradation are phylogenetically spread around the bacterial tree of life, with over 286 bacteria from five different phyla reported in the literature (Gambarini et al., 2021). Polybutylene succinate is a biodegradable polymer with excellent biodegradability, thermoplastic processability, and balanced mechanical properties (Xu and Guo, 2010). Many studies on PBS have reported significant biodegradation within several months in different environmental matrices such as soil (compost), water, and seawater (Nishioka et al., 1994; Hirotsu et al., 2000; Morohoshi et al., 2018). Moreover, PBS can be naturally degraded in the environment by bacteria (Teeraphatpornchai et al., 2003) and fungi (Kim and Rhee, 2003). The Comamonadaceae family was one of the six families identified with PBS degraders. A search on the PlasticDB webserver revealed only one enzyme described in the literature with depolymerase activity towards PBS. This enzyme was isolated from the bacterium Acidovorax delafieldii, which belongs to the family Comamonadaceae, indicating that bacteria belonging to this family may be associated with biodegradation of PBS in our study.
Overall, our results show that the different concentrations of oyster-shell did not affect the specific recruitment of presumed bacterial degraders, although significant changes were observed between sampling time points (Figure 7). Early time points had a greater abundance of bacterial families with plastic biodegradation reports, while later time points displayed a decrease in abundance of these families. Erni-Cassola et al. (2020) reported this phenomenon for polyethylene strips incubated in situ in coastal seawater. The authors investigated the abundance of bacteria capable of biodegrading hydrocarbons. They found that taxa that might break down hydrocarbons are abundant in very early colonizing populations (two days), following a decrease in their relative abundance at a later time point (nine days). The authors hypothesized that this phenomenon was driven by the depletion of labile, plastic-derived substrates formed by weathering. After this initial depletion, non-hydrolyzable polymers substrates may not serve as an essential carbon source for the microbial biofilm attached to the plastic. Interestingly, a similar pattern was observed on PBS even though, unlike polyethylene, PBS has been described as a biodegradable polymer (Rafiqah et al., 2021). Bacterial degraders detected in the present study are probably outnumbered by later, more generalist bacterial colonizers. Hence, the development of later biofilm stages might hinder our ability to detect putative PBS degraders.
Conclusion
This study showcases for the first time the effect of bio-polymers amended with oyster shell filler on the development and evolution of marine fouling assemblages. Our results revealed a lower contribution of NIS on shell-enriched substrates compared to unadulterated polymers. In contrast, the different concentrations of oyster shells did not affect the specific recruitment of potential bacterial degraders. Although numerous studies have explored the potential application of shell waste in different industry sectors, this research paves the way for future studies investigating the succession of marine micro- and macro- communities onto shell-enriched bio-polymers using DNA metabarcoding. We suggest that bio-polymers enriched with specific oyster shells concentrations might be considered as an alternative to conventional polymers in, e.g., aquaculture or eco-engineering solutions for coastal infrastructure (Floerl et al., 2021), reducing the recruitment and spread of non-indigenous taxa. Despite the fact that bio-polymers have brought a new perspective for developing eco-friendly composites with increased recyclability and degradation (Chowdhury et al., 2017; Zhang et al., 2017), more research is needed to: (i) assess the optimum concentration of oyster shells (or other natural compounds) to improve and control the degradation pattern of the bio-polymers; (ii) investigate if incorporating other types of aquaculture waste could prevent the recruitment of unwanted organisms; and (iii) optimize the design and production of biopolymers to lower the cost of the process towards an economically viable alternative to petroleum-based polymers. Moreover, future research should include additional polymer controls to effectively investigate the benefits of bio-polymers enriched with aquaculture waste in comparison with conventional polymers.
Data Availability Statement
The datasets presented in this study can be found in online repositories. The names of the repository/repositories and accession number(s) can be found below: https://www.ncbi.nlm.nih.gov/, PRJNA850371.
Authors Contributions
FA: Investigation, Data collection, analysis and interpretation of the results, Writing – original draft preparation; AZ: Conceptualization, Data collection and analysis, Review and editing, Supervision, Funding acquisition, Project administration; XP: Conceptualization, Review and editing, Supervision; M-JL: Design and manufacture of the bio-polymers, Review and editing the methods section; OF: Conceptualization, Study design, Writing – review and editing; VG: Data analysis of the bacterial degrader’s section, Review and editing GL: Review and editing; BT: Laboratory analysis. All authors contributed to the article and approved the submitted version.
Funding
This work was supported by the New Zealand Ministry of Business, Innovation and Employment, Endeavour Research Programmes C03X1802 (Impacts of microplastics on New Zealand’s bioheritage systems, environment, and eco-services) and CAWX1904 (A toolbox to underpin and enable tomorrow’s marine biosecurity system).
Conflict of Interest
The authors declare that the research was conducted in the absence of any commercial or financial relationships that could be construed as a potential conflict of interest.
Publisher’s Note
All claims expressed in this article are solely those of the authors and do not necessarily represent those of their affiliated organizations, or those of the publisher, the editors and the reviewers. Any product that may be evaluated in this article, or claim that may be made by its manufacturer, is not guaranteed or endorsed by the publisher.
Acknowledgments
We are particularly grateful to Maxime Barbier (Scion) and Rosalie Collins-Gargan (Scion) for making and testing the composite materials Jekaterina Koldajeva-Fogg for assistance with field deployment and sample collection. Olga Pantos and Grant Northcott for valuable discussions. Louis Tremblay and Susanna Wood for their advice in writing the manuscript. This work was supported by the New Zealand Ministry of Business, Innovation and Employment, Endeavour Research Programmes C03X1802 (Impacts of microplastics on New Zealand’s bioheritage systems, environment, and eco-services) and CAWX1904 (A toolbox to underpin and enable tomorrow’s marine biosecurity system).
Supplementary Material
The Supplementary Material for this article can be found online at: https://www.frontiersin.org/articles/10.3389/fmars.2022.891183/full#supplementary-material
Supplementary Table 1 | Total number of reads per marker (16S and 18S) for each sample before and throughout the DADA2 quality control, filtering, and chimera removal.
Supplementary Table 2 | Alpha diversity measures (ASV number and Shannon index) between subgroups “Sampling week” for bacterial (top) and eukaryotic (bottom) datasets.
Supplementary Table 3 | PERMANOVA partitioning and analysis of bacterial (top) and eukaryotic (bottom) assemblages in relation to the experimental factors ‘Sampling’ (Sa), ‘Type’ (Ty), and ‘Side’ (Si), based on the fourth root transformed. abundance data and Bray-Curtis dissimilarities. Pseudo F statistics were calculated for each term using direct analogs to univariate expectations of mean squares (MS); p-values were obtained using 9999 permutations under a reduced model. Each term is identified as contributing either a fixed or random component to the overall model; “Var” gives the estimated sixes of component of variation, based on multivariate analogs to the classical ANOVA unbiased estimators; “SD” gives the fourth root of these values (in Bray-Curtis unit).
Supplementary Table 4 | Pairwise PERMANOVA based on polymer type (control, OYS20%, OYS40%, OYS60%) when sampled at different times. Significant values are highlighted in bold.
Supplementary Table 5 | Inventory of non-indigenous species (NIS) detected in the present study.
Supplementary Figure 1 | Line graph of time (weeks) vs. Temperature (°C).
Supplementary Figure 2 | Phylogenetic tree representing all bacterial Amplicon Sequence Variants (ASVs) detected in our study and highlighting their family affiliation, which were identified as having potential plastic-degrading capabilities. The taxa with reports of plastic degradation were identified using the PlasticDB webserver (http://www.plasticdb.org/), which contains 562 microbial species. The phylogenetic relationship among the shown taxa was extracted from the NCBI taxonomic database (Schoch et al., 2020). There is a total of 294 bacterial families in our study that were successfully processed by the PlasticDB tool, resulting in 41 families identified as having reports of plastic degradation. Leaves are colored according to their corresponding phyla. Blue stars represent bacterial genus reported to degrade Polybutylene succinate (PBS), red stars represent bacterial genus reported to degrade other types of plastics. Icons highlighted on the rings external to the tree represent the different substrates (Control, OYS20%, OYS40%, OYS60%).
References
Abed R. M., Al Fahdi D., Muthukrishnan T. (2019). Short-Term Succession of Marine Microbial Fouling Communities and the Identification of Primary and Secondary Colonizers. Biofouling 35, 526–540. doi: 10.1080/08927014.2019.1622004
Afonso I., Berecibar E., Castro N., Costa J., Frias P., Henriques F., et al. (2020). Assessment of the Colonization and Dispersal Success of non-Indigenous Species Introduced in Recreational Marinas Along the Estuarine Gradient. Ecol. Indic. 113, 106147. doi: 10.1016/j.ecolind.2020.106147
Anderson M. J. (2001). Permutation Tests for Univariate or Multivariate Analysis of Variance and Regression. Can. J. Fisheries. Aquat. Sci. 58, 626–639. doi: 10.1139/f01-004
Ardura A., Planes S., Garcia-Vazquez E. (2015). Aliens in Paradise. Boat Density and Exotic Coastal Mollusks in Moorea Island (French Polynesia). Marine. Environ. Res. 112, 56–63. doi: 10.1016/j.marenvres.2015.08.007
Audrézet F., Zaiko A., Lear G., Wood S. A., Tremblay L. A., Pochon X. (2020). Biosecurity Implications of Drifting Marine Plastic Debris: Current Knowledge and Future Research. Marine. Pollut. Bull. 162, 111835. doi: 10.1016/j.marpolbul.2020.111835
Balart R., Garcia-Garcia D., Fombuena V., Quiles-Carrillo L., Arrieta M. P. (2021). Biopolymers From Natural Resources. Polymers 13, 2532. doi: 10.3390/polym13152532
Barros M., Bello P., Bao M., Torrado J. (2009). From Waste to Commodity: Transforming Shells Into High Purity Calcium Carbonate. J. Cleaner. Prod. 17, 400–407. doi: 10.1016/j.jclepro.2008.08.013
Bell K. L., Burgess K. S., Botsch J. C., Dobbs E. K., Read T. D., Brosi B. J. (2019). Quantitative and Qualitative Assessment of Pollen DNA Metabarcoding Using Constructed Species Mixtures. Mol. Ecol. 28, 431–455. doi: 10.1111/mec.14840
Bland J. M., Altman D. G. (1995). Multiple Significance Tests: The Bonferroni Method. Br. Med. J. 310, 170–170. doi: 10.1136/bmj.310.6973.170
Blum J. C., Chang A. L., Liljesthröm M., Schenk M. E., Steinberg M. K., Ruiz G. M. (2007). The non-Native Solitary Ascidian Ciona Intestinalis (L.) Depresses Species Richness. J. Exp. Marine. Biol. Ecol. 342, 5–14. doi: 10.1016/j.jembe.2006.10.010
Borrell Y. J., Miralles L., Do Huu H., Mohammed-Geba K., Garcia-Vazquez E. (2017). DNA in a Bottle—Rapid Metabarcoding Survey for Early Alerts of Invasive Species in Ports. PloS One 12, e0183347. doi: 10.1371/journal.pone.0183347
Brown T., Otero C., Grajales A., Rodriguez E., Rodriguez-Lanetty M. (2017). Worldwide Exploration of the Microbiome Harbored by the Cnidarian Model, Exaiptasia Pallida (Agassiz in Verril 1864) Indicates a Lack of Bacterial Association Specificity at a Lower Taxonomic Rank. PeerJ 5, e3235. doi: 10.7717/peerj.3235
Bukin Y. S., Galachyants Y. P., Morozov I. V., Bukin S. V., Zakharenko A. S., Zemskaya T. I. (2019). The Effect of 16S rRNA Region Choice on Bacterial Community Metabarcoding Results. Scientific Data, 6(1), 1–14.
Cairns S. D., Gerhswin L. A., Brook F., Pugh P. R., Dawson E. W., Ocaña V. O., et al. (2009). Phylum Cnidaria; Corals, Medusae, Hydroids, Myxozoa. In: Gordon D. P., ed. New Zealand Inventory of Biodiversity. Christchurch: Canterbury University Press. 1, 59–101.
Callahan B. J., McMurdie P. J., Rosen M. J., Han A. W., Johnson A. J. A., Holmes S. P. (2016). DADA2: High-Resolution Sample Inference From Illumina Amplicon Data. Nat. Methods 13, 581–583. doi: 10.1038/nmeth.3869
Campani T., Baini M., Giannetti M., Cancelli F., Mancusi C., Serena F., et al. (2013). Presence of Plastic Debris in Loggerhead Turtle Stranded Along the Tuscany Coasts of the Pelagos Sanctuary for Mediterranean Marine Mammals (Italy). Marine. Pollut. Bull. 74, 225–230. doi: 10.1016/j.marpolbul.2013.06.053
Chowdhury S. N., Nag S., Tripathi K. (2017). “Recent Advances in Bio-Polymers for Innovative Food Packaging,” in Biopolymers: Structure, Performance, Applications. (Hauppauge, New York State, United States: Nova Science Publishers, Inc)
Clancey L., Hinton R. (2003). Distribution of the Tunicate, Ciona Intestinalis Nova Scotia. (Nova Scotia Department Agriculture Fisheries: Nova Scotia), 6 pp.
Clarke K., Gorley R. (2015). PRIMER V7: User Manual/Tutorial (Plymouth, United Kingdom: Primer-E Ltd).
Clark D., Pilditch C., Pearman J., Ellis J., Zaiko A. (2020). Environmental DNA Metabarcoding Reveals Estuarine Benthic Community Response to Nutrient Enrichment–Evidence From an in-Situ Experiment. Environ. Pollut. 267, 115472. doi: 10.1016/j.envpol.2020.115472
Dalby J. E. Jr, Young C. M. (1993). Variable Effects of Ascidian Competitors on Oysters in a Florida Epifaunal Community. J. Exp. Marine. Biol. Ecol. 167, 47–57. doi: 10.1016/0022-0981(93)90183-O
D’Amore R., Ijaz U. Z., Schirmer M., Kenny J. G., Gregory R., Darby A. C., et al. (2016). A Comprehensive Benchmarking Study of Protocols and Sequencing Platforms for 16S rRNA Community Profiling. BMC Genomics 17, 1–20. doi: 10.1186/s12864-015-2194-9
Datta M. S., Almada A. A., Baumgartner M. F., Mincer T. J., Tarrant A. M., Polz M. F. (2018). Inter-Individual Variability in Copepod Microbiomes Reveals Bacterial Networks Linked to Host Physiology. ISME. J. 12, 2103–2113. doi: 10.1038/s41396-018-0182-1
Debroas D., Mone A., Ter Halle A. (2017). Plastics in the North Atlantic Garbage Patch: A Boat-Microbe for Hitchhikers and Plastic Degraders. Sci. Total. Environ. 599, 1222–1232. doi: 10.1016/j.scitotenv.2017.05.059
Delacuvellerie A., Géron A., Gobert S., Wattiez R. (2021). New Insights Into the Functioning and Structure of the PE and PP Plastispheres From the Mediterranean Sea. Environ. Pollut. 295, 118678. doi: 10.1016/j.envpol.2021.118678
De Tender C. A., Devriese L. I., Haegeman A., Maes S., Ruttink T., Dawyndt P. (2015). Bacterial Community Profiling of Plastic Litter in the Belgian Part of the North Sea. Environ. Sci. Technol. 49, 9629–9638. doi: 10.1021/acs.est.5b01093
Devi R. S., Kannan V. R., Natarajan K., Nivas D., Kannan K., Chandru S., et al. (2016). The Role of Microbes in Plastic Degradation. In Environ Waste Manage ed. Chandra R. pp. 341–370 United States: CRC Press.
Dror H., Novak L., Evans J. S., López-Legentil S., Shenkar N. (2019). Core and Dynamic Microbial Communities of Two Invasive Ascidians: Can Host–Symbiont Dynamics Plasticity Affect Invasion Capacity? Microbial. Ecol. 78, 170–184. doi: 10.1007/s00248-018-1276-z
Dussud C., Hudec C., George M., Fabre P., Higgs P., Bruzaud S., et al. (2018b). Colonization of non-Biodegradable and Biodegradable Plastics by Marine Microorganisms. Front. Microbiol. 9, 1571. doi: 10.3389/fmicb.2018.01571
Dussud C., Meistertzheim A., Conan P., Pujo-Pay M., George M., Fabre P., et al. (2018a). Evidence of Niche Partitioning Among Bacteria Living on Plastics, Organic Particles and Surrounding Seawaters. Environ. Pollut. 236, 807–816. doi: 10.1016/j.envpol.2017.12.027
Ehrenberg C. (1844). Zum Schlufs Legte Hr. Ehrenberg Der Akademie Einige Vorlaufige Resultate Seiner Untersuchungen Der Ihm Von Der Sudpolreise Des Capitain Rofs, So Wie Von Den Herren Schayer Und Darwin Zugekommenen Materialien Uber Das Verhalten Des Kleinsten Lebens in Den Oceanen Und Den Grofsten Bisher Zuganaglichen Tiefen Des Weltmeers Vor. I. Die Sud-Pol-Reise von - Ber 1: 182-207.
Elifantz H., Horn G., Ayon M., Cohen Y., Minz D. (2013). Rhodobacteraceae are the Key Members of the Microbial Community of the Initial Biofilm Formed in Eastern Mediterranean Coastal Seawater. FEMS Microbiol. Ecol. 85, 348–357. doi: 10.1111/1574-6941.12122
Enrichetti F., Bavestrello G., Betti F., Rindi F., Tregrosso A., Bo M. (2021). Fate of Lost Fishing Gears: Experimental Evidence of Biofouling Colonization Patterns From the Northwestern Mediterranean Sea. Environ. Pollut. 268, 115746. doi: 10.1016/j.envpol.2020.115746
Erni-Cassola G., Wright R. J., Gibson M. I., Christie-Oleza J. A. (2020). Early Colonization of Weathered Polyethylene by Distinct Bacteria in Marine Coastal Seawater. Microbial. Ecol. 79, 517–526. doi: 10.1007/s00248-019-01424-5
Erwin P. M., Pineda M. C., Webster N., Turon X., Lopez-Legentil S. (2013). Small Core Communities and High Variability in Bacteria Associated With the Introduced Ascidian Styela Plicata. Symbiosis 59, 35–46. doi: 10.1007/s13199-012-0204-0
Ferrario J., Caronni S., Occhipinti-Ambrogi A., Marchini A. (2017). Role of Commercial Harbours and Recreational Marinas in the Spread of non-Indigenous Fouling Species. Biofouling 33, 651–660. doi: 10.1080/08927014.2017.1351958
Fletcher L. M., Zaiko A., Atalah J., Richter I., Dufour C. M., Pochon X., et al. (2017). Bilge Water as a Vector for the Spread of Marine Pests: A Morphological, Metabarcoding and Experimental Assessment. Biol. Invasions. 19, 2851–2867. doi: 10.1007/s10530-017-1489-y
Floerl O., Atalah J., Bugnot A. B., Chandler M., Dafforn K. A., Floerl L., et al. (2021). A Global Model to Forecast Coastal Hardening and Mitigate Associated Socioecological Risks. Nat. Sustainability. 4, 1060–1067. doi: 10.1038/s41893-021-00780-w
Galgani L., Beiras R., Galgani F., Panti C., Borja A. (2019). Impacts of Marine Litter. Front. Marine. Sci. 6, 208. doi: 10.3389/fmars.2019.00208
Galgani F., Pham C. K., Reisser J. (2017). Plastic Pollution. Front. Marine. Sci. 4, 307. doi: 10.3389/fmars.2017.00307
Gambarini V., Pantos O., Kingsbury J. M., Weaver L., Handley K. M., Lear G. (2021). Phylogenetic Distribution of Plastic-Degrading Microorganisms. Msystems 6, e01112–e01120. doi: 10.1128/mSystems.01112-20
Gambarini V., Pantos O., Kingsbury J. M., Weaver L., Handley K. M., Lear G., et al (2022). Antiphotoaging Effect of Boiled Abalone Residual Peptide ATPGDEG on UVB-induced Keratinocyte HaCaT Cells. Database 2022, baac008. doi: 10.1093/database/baac008
Ganguly A., Sharma K., Majumder K. (2020). Peptides as Biopolymers—Past, Present, and Future. Biopolymer-Based. Formulations.: Biomed. Food Appl., 87–104. doi: 10.1016/B978-0-12-816897-4.00004-7
Gao L. (2005). Latin Squares in Experimental Design (Michigan State University, East Lansing, MI, USA).
García-Gómez J. C., Garrigós M., Garrigós J. (2021). Plastic as a Vector of Dispersion for Marine Species With Invasive Potential. A Review. Front. Ecol. Evol. 9, 208. doi: 10.3389/fevo.2021.629756
Geyer R., Jambeck J. R., Law K. L. (2017). Production, Use, and Fate of All Plastics Ever Made. Sci. Adv. 3, e1700782. doi: 10.1126/sciadv.1700782
Grey E. K., Bernatchez L., Cassey P., Deiner K., Deveney M., Howland K. L., et al. (2018). Effects of Sampling Effort on Biodiversity Patterns Estimated From Environmental DNA Metabarcoding Surveys. Sci. Rep. 8, 1–10. doi: 10.1038/s41598-018-27048-2
Guillou L., Bachar D., Audic S., Bass D., Berney C., Bittner L., et al. (2012). The Protist Ribosomal Reference Database (PR2): A Catalog of Unicellular Eukaryote Small Sub-Unit rRNA Sequences With Curated Taxonomy. Nucleic Acids Res. 41, D597–D604. doi: 10.1093/nar/gks1160
Haram L. E., Carlton J. T., Centurioni L., Crowley M., Hafner J., Maximenko N., et al. (2021). Emergence of a Neopelagic Community Through the Establishment of Coastal Species on the High Seas. Nat. Commun. 12, 1–5. doi: 10.1093/nar/gks1160
Hawes N. A., Tremblay L. A., Pochon X., Dunphy B., Fidler A. E., Smith K. F. (2018). Effects of Temperature and Salinity Stress on DNA Methylation in a Highly Invasive Marine Invertebrate, the Colonial Ascidian Didemnum Vexillum. PeerJ 6, e5003. doi: 10.7717/peerj.5003
Herdman W. A. (1886). Report on the Tunicata Collected During the Voyage of HMS Challenger During the Years 1873-76. Ascidiae simplices. Edinburg, Report of the Scientific Results of the Voyage of H.M.S. Challenger during the years 1873–76, vol 14, 429p.
Herlemann D. P., Labrenz M., Jürgens K., Bertilsson S., Waniek J. J., Andersson A. F. (2011). Transitions in Bacterial Communities Along the 2000 Km Salinity Gradient of the Baltic Sea. ISME. J. 5, 1571–1579. doi: 10.1038/ismej.2011.41
Hirotsu T., Tsujisaka T., Masuda T., Nakayama K. (2000). Plasma Surface Treatments and Biodegradation of Poly (Butylene Succinate) Sheets. J. Appl. Polymer. Sci. 78, 1121–1129. doi: 10.1002/1097-4628(20001031)78:5<1121::AID-APP210>3.0.CO;2-H
Hoffmann F., Radax R., Woebken D., Holtappels M., Lavik G., Rapp H. T., et al. (2009). Complex Nitrogen Cycling in the Sponge Geodia Barretti. Environ. Microbiol. 11, 2228–2243. doi: 10.1111/j.1462-2920.2009.01944.x
Hou D., Hong M., Wang K., Yan H., Wang Y., Dong P., et al. (2021). Prokaryotic Community Succession and Assembly on Different Types of Microplastics in a Mariculture Cage. Environ. Pollut. 268, 115756. doi: 10.1016/j.envpol.2020.115756
Howes S., Herbinger C., Darnell P., Vercaemer B. (2007). Spatial and Temporal Patterns of Recruitment of the Tunicate Ciona Intestinalis on a Mussel Farm in Nova Scotia, Canada. J. Exp. Marine. Biol. Ecol. 342, 85–92. doi: 10.1016/j.jembe.2006.10.018
Huerta-Cepas J., Serra F., Bork P. (2016). ETE 3: Reconstruction, Analysis, and Visualization of Phylogenomic Data. Mol. Biol. Evol. 33, 1635–1638. doi: 10.1093/molbev/msw046
Isobe A., Azuma T., Cordova M. R., Cózar A., Galgani F., Hagita R., et al. (2021). A Multilevel Dataset of Microplastic Abundance in the World’s Upper Ocean and the Laurentian Great Lakes. Microplastics. Nanoplastics. 1, 1–14. doi: 10.1186/s43591-021-00013-z
Jambeck J. R., Geyer R., Wilcox C., Siegler T. R., Perryman M., Andrady A., et al. (2015). Plastic Waste Inputs From Land Into the Ocean. Science 347, 768–771. doi: 10.1126/science.1260352
Jenkins S. R., Martins G. M. (2010). Succession on Hard Substrata. Biofouling 456, 60–72. doi: 10.1002/9781444315462.ch4
Kandlikar G. S., Gold Z. J., Cowen M. C., Meyer R. S., Freise A. C., Kraft N. J., et al. (2018). Ranacapa: An R Package and Shiny Web App to Explore Environmental DNA Data With Exploratory Statistics and Interactive Visualizations. F1000Research 7, 1734–1818. doi: 10.12688/f1000research.16680.1
Kandra P., Challa M. M., Jyothi H. K. P. (2012). Efficient Use of Shrimp Waste: Present and Future Trends. Appl. Microbiol. Biotechnol. 93, 17–29. doi: 10.1007/s00253-011-3651-2
Kelly J. J., London M. G., McCormick A. R., Rojas M., Scott J. W., Hoellein T. (2021). Wastewater Treatment Alters Microbial Colonization of Microplastics. PloS One 16, e0244443. doi: 10.1371/journal.pone.0244443
Kettner M. T., Rojas-Jimenez K., Oberbeckmann S., Labrenz M., Grossart H. P. (2017). Microplastics Alter Composition of Fungal Communities in Aquatic Ecosystems. Environ. Microbiol. 19, 4447–4459. doi: 10.1111/1462-2920.13891
Kim D., Rhee Y. (2003). Biodegradation of Microbial and Synthetic Polyesters by Fungi. Appl. Microbiol. Biotechnol. 61, 300–308. doi: 10.1007/s00253-002-1205-3
Klindworth A., Pruesse E., Schweer T., Peplies J., Quast C., Horn M., et al. (2013). Evaluation of General 16S Ribosomal RNA Gene PCR Primers for Classical and Next-Generation Sequencing-Based Diversity Studies. Nucleic Acids Res. 41, e1–10. doi: 10.1093/nar/gks808
Krug P. (2006). Defense of Benthic Invertebrates Against Surface Colonization by Larvae: A Chemical Arms Race. Antifouling. Compounds. 42, 1–53. doi: 10.1007/3-540-30016-3_1
Lakshmi K., Muthukumar T., Doble M., Vedaprakash L., Dineshram R., Jayaraj K., et al. (2012). Influence of Surface Characteristics on Biofouling Formed on Polymers Exposed to Coastal Sea Waters of India. Colloids. Surfaces. Biointerfaces. 91, 205–211. doi: 10.1016/j.colsurfb.2011.11.003
LeBlanc N., Davidson J., Tremblay R., McNiven M., Landry T. (2007). The Effect of Anti-Fouling Treatments for the Clubbed Tunicate on the Blue Mussel, Mytilus Edulis. Aquaculture 264, 205–213. doi: 10.1016/j.aquaculture.2006.12.027
Lee J.-W., Nam J.-H., Kim Y.-H., Lee K.-H., Lee D.-H. (2008). Bacterial Communities in the Initial Stage of Marine Biofilm Formation on Artificial Surfaces. J. Microbiol. 46, 174–182. doi: 10.1007/s12275-008-0032-3
Letunic I., Bork P. (2021). Interactive Tree Of Life (iTOL) V5: An Online Tool for Phylogenetic Tree Display and Annotation. Nucleic Acids Res. 49, W293–W296. doi: 10.1093/nar/gkab301
Li Z., Feng C., Wu Y., Guo X. (2020). Impacts of Nanoplastics on Bivalve: Fluorescence Tracing of Organ Accumulation, Oxidative Stress and Damage. J. Hazardous. Mater. 392, 122418. doi: 10.1016/j.jhazmat.2020.122418
Lin V. S. (2016). Research Highlights: Impacts of Microplastics on Plankton. Environ. Sci.: Processes. Impacts. 18, 160–163. doi: 10.1039/C6EM90004F
Lindquist N. (2002). Chemical Defense of Early Life Stages of Benthic Marine Invertebrates. J. Chem. Ecol. 28, 1987–2000. doi: 10.1023/A:1020745810968
Lionetto F., Esposito Corcione C. (2021). Recent Applications of Biopolymers Derived From Fish Industry Waste in Food Packaging. Polymers 13, 2337. doi: 10.3390/polym13142337
Liu Y., Xie J., Wu N., Wang L., Ma Y., Tong J. (2019). Influence of Silane Treatment on the Mechanical, Tribological and Morphological Properties of Corn Stalk Fiber Reinforced Polymer Composites. Tribol. Int. 131, 398–405. doi: 10.1016/j.triboint.2018.11.004
Lyons D. A., Lowen J. B., Therriault T. W., Brickman D., Guo L., Moore A. M., et al. (2020). Identifying Marine Invasion Hotspots Using Stacked Species Distribution Models. Biol. Invasions. 22, 3403–3423. doi: 10.1007/s10530-020-02332-3
Marin F., Luquet G. (2004). Molluscan Shell Proteins. Comptes. Rendus. Palevol. 3, 469–492. doi: 10.1016/j.crpv.2004.07.009
Marins F. O., Novaes R. L., Rocha R. M., Junqueira A. O. (2010). Non Indigenous Ascidians in Port and Natural Environments in a Tropical Brazilian Bay. Zoologia 27, 213–221. doi: 10.1590/S1984-46702010000200009
Martell L., Bracale R., Carrion S. A., Purcell J. E., Lezzi M., Gravili C., et al. (2018). Successional Dynamics of Marine Fouling Hydroids (Cnidaria: Hydrozoa) at a Finfish Aquaculture Facility in the Mediterranean Sea. PloS One 13, e0195352. doi: 10.1371/journal.pone.0195352
Martin M. (2011). Cutadapt Removes Adapter Sequences From High-Throughput Sequencing Reads. EMBnet. J. 17, 10–12. doi: 10.14806/ej.17.1.200
Martínez-Campos S., González-Pleiter M., Fernández-Piñas F., Rosal R., Leganés F. (2021). Early and Differential Bacterial Colonization on Microplastics Deployed Into the Effluents of Wastewater Treatment Plants. Sci. Total. Environ. 757, 143832. doi: 10.1016/j.scitotenv.2020.143832
McMurdie P. J., Holmes S. (2013). Phyloseq: An R Package for Reproducible Interactive Analysis and Graphics of Microbiome Census Data. PloS One 8, e61217. doi: 10.1371/journal.pone.0061217
Meng J., Zhang Q., Zheng Y., He G., Shi H. (2021). Plastic Waste as the Potential Carriers of Pathogens. Curr. Opin. Food Sci. 41, 224–230. doi: 10.1016/j.cofs.2021.04.016
Migotto A. E., Marques A. C., Flynn M. N. (2001). Seasonal Recruitment of Hydroids (Cnidaria) on Experimental Panels in the São Sebastião Channel, Southeastern Brazil. Bull. Marine. Sci. 68, 287–298.
Mincer T. J., Zettler E. R., Amaral-Zettler L. A. (2016). Biofilms on Plastic Debris and Their Influence on Marine Nutrient Cycling, Productivity, and Hazardous Chemical Mobility. Hazardous. Chem. Assoc. Plastics. Marine. Environ. 78, 221–233. doi: 10.1007/698_2016_12
Miralles L., Gomez-Agenjo M., Rayon-Viña F., Gyraitė G., Garcia-Vazquez E. (2018). Alert Calling in Port Areas: Marine Litter as Possible Secondary Dispersal Vector for Hitchhiking Invasive Species. J. Nat. Conserv. 42, 12–18. doi: 10.1016/j.jnc.2018.01.005
Miranda D., de Carvalho-Souza G. F. (2016). Are We Eating Plastic-Ingesting Fish? Marine. Pollut. Bull. 103, 109–114. doi: 10.1016/j.marpolbul.2015.12.035
Moore C. (2014). Rapidly Increasing Plastic Pollution From Aquaculture Threatens Marine Life. Tulane. Environ. Law J. 27(2), 205–217. http://www.jstor.org/stable/43294163
Morohoshi T., Ogata K., Okura T., Sato S. (2018). Molecular Characterization of the Bacterial Community in Biofilms for Degradation of Poly (3-Hydroxybutyrate-Co-3-Hydroxyhexanoate) Films in Seawater. Microbes Environ. 33, 19–25. doi: 10.1264/jsme2.ME17052
Muthukrishnan T., Al Khaburi M., Abed R. M. (2019). Fouling Microbial Communities on Plastics Compared With Wood and Steel: Are They Substrate-or Location-Specific? Microbial. Ecol. 78, 361–374. doi: 10.1007/s00248-018-1303-0
Nelson K. A., Leonard L. A., Posey M. H., Alphin T. D., Mallin M. A. (2004). Using Transplanted Oyster (Crassostrea Virginica) Beds to Improve Water Quality in Small Tidal Creeks: A Pilot Study. J. Exp. Marine. Biol. Ecol. 298, 347–368. doi: 10.1016/S0022-0981(03)00367-8
Nishioka M., Tuzuki T., Wanajyo Y., Oonami H., Horiuchi T. (1994). Biodegradation of BIONOLLE. Biodegradable. Plastics. Polymers. 12, 584–590. doi: 10.1016/B978-0-444-81708-2.50069-5
Oberbeckmann S., Kreikemeyer B., Labrenz M. (2018). Environmental Factors Support the Formation of Specific Bacterial Assemblages on Microplastics. Front. Microbiol. 8, 2709. doi: 10.3389/fmicb.2017.02709
Oberbeckmann S., Labrenz M. (2020). Marine Microbial Assemblages on Microplastics: Diversity, Adaptation, and Role in Degradation. Annu. Rev. Marine. Sci. 12, 209–232. doi: 10.1146/annurev-marine-010419-010633
Oberbeckmann S., Loeder M. G., Gerdts G., Osborn A. M. (2014). Spatial and Seasonal Variation in Diversity and Structure of Microbial Biofilms on Marine Plastics in Northern European Waters. FEMS Microbiol. Ecol. 90, 478–492. doi: 10.1111/1574-6941.12409
Oberbeckmann S., Osborn A. M., Duhaime M. B. (2016). Microbes on a Bottle: Substrate, Season and Geography Influence Community Composition of Microbes Colonizing Marine Plastic Debris. PloS One 11, e0159289. doi: 10.1371/journal.pone.0159289
O’Driscoll R., Booth J., Bagley N., Anderson O., Griggs L., Stevenson M., et al. (2003). Areas of Importance for Spawning, Pupping or Egg-Laying, and Juveniles of New Zealand Deepwater Fish, Pelagic Fish, and Invertebrates. New Z. Fisheries. Assess. Rep. 2008/30. 119, 1–377.
Oksanen J., Blanchet F. G., Kindt R., Legendre P., Minchin P. R., O’hara R., et al. (2013). Package ‘Vegan’. Community Ecology Package, Version 2. Community Ecology Package, Version 2 2, 1–295. https://cran.r-project.org/web/packages/vegan/index.html.
Paetzold S. C., Giberson D. J., Hill J., Davidson J. D., Davidson J. (2012). Effect of Colonial Tunicate Presence on Ciona Intestinalis Recruitment Within a Mussel Farming Environment. Manage. Biol. Invasions. 3, 15–23. doi: 10.3391/mbi.2012.3.1.02
Panti C., Baini M., Lusher A., Hernandez-Milan G., Rebolledo E. L. B., Unger B., et al. (2019). Marine Litter: One of the Major Threats for Marine Mammals. Outcomes From the European Cetacean Society Workshop. Environ. Pollut. 247, 72–79. doi: 10.1016/j.envpol.2019.01.029
Pawlowski J., Esling P., Lejzerowicz F., Cordier T., Visco J. A., Martins C. I., Cedhagen T., et al (2016). Benthic Monitoring of Salmon Farms in Norway Using Foraminiferal Metabarcoding. Aquaculture Environment Interactions, 8, 371–386.
Pearman J. K., von Ammon U., Laroche O., Zaiko A., Wood S. A., Zubia M., et al. (2021). Metabarcoding as a Tool to Enhance Marine Surveillance of Nonindigenous Species in Tropical Harbors: A Case Study in Tahiti. Environ. DNA 3, 173–189. doi: 10.1002/edn3.154
Peterson C. H., Grabowski J. H., Powers S. P. (2003). Estimated Enhancement of Fish Production Resulting From Restoring Oyster Reef Habitat: Quantitative Valuation. Marine. Ecol. Prog. Ser. 264, 249–264. doi: 10.3354/meps264249
Penca J. (2018). European Plastics Strategy: What Promise for Global Marine Litter? Marine Policy, 97, 197–201.
Pochon X., Wecker P., Stat M., Berteaux-Lecellier V., Lecellier G. (2019). Towards an in-Depth Characterization of Symbiodiniaceae in Tropical Giant Clams via Metabarcoding of Pooled Multi-Gene Amplicons. PeerJ 7, e6898. doi: 10.7717/peerj.6898
Pochon X., Zaiko A., Fletcher L. M., Laroche O., Wood S. A. (2017). Wanted Dead or Alive? Using Metabarcoding of Environmental DNA and RNA to Distinguish Living Assemblages for Biosecurity Applications. PloS One 12, e0187636. doi: 10.1371/journal.pone.0187636
Pollet T., Berdjeb L., Garnier C., Durrieu G., Le Poupon C., Misson B., et al. (2018). Prokaryotic Community Successions and Interactions in Marine Biofilms: The Key Role of Flavobacteriia. FEMS Microbiol. Ecol. 94, 1–13. doi: 10.1093/femsec/fiy083
Qian P.-Y., Lau S. C., Dahms H.-U., Dobretsov S., Harder T. (2007). Marine Biofilms as Mediators of Colonization by Marine Macroorganisms: Implications for Antifouling and Aquaculture. Marine. Biotechnol. 9, 399–410. doi: 10.1007/s10126-007-9001-9
Quast C., Pruesse E., Yilmaz P., Gerken J., Schweer T., Yarza P., et al. (2012). The SILVA Ribosomal RNA Gene Database Project: Improved Data Processing and Web-Based Tools. Nucleic Acids Res. 41, D590–D596. doi: 10.1093/nar/gks1219
Quell F., Schratzberger M., Beauchard O., Bruggeman J., Webb T. (2021). Biological Trait Profiles Discriminate Between Native and non-Indigenous Marine Invertebrates. Aquat. Invasions. 16, 571–600. doi: 10.3391/ai.2021.16.4.01
Rafiqah S., Khalina A., Harmaen A. S., Tawakkal I. A., Zaman K., Asim M., et al. (2021). A Review on Properties and Application of Bio-Based Poly (Butylene Succinate). Polymers 13, 1436. doi: 10.3390/polym13091436
Rinkevich B., Sakai K. (2001). Interspecific Interactions Among Species of the Coral Genus Porites From Okinawa, Japan. Zoology 104, 91–97. doi: 10.1078/0944-2006-00014
Rochman C. M., Browne M. A., Halpern B. S., Hentschel B. T., Hoh E., Karapanagioti H. K., et al. (2013). Classify Plastic Waste as Hazardous. Nature 494, 169–171. doi: 10.1038/494169a
Rochman C. M., Tahir A., Williams S. L., Baxa D. V., Lam R., Miller J. T., et al. (2015). Anthropogenic Debris in Seafood: Plastic Debris and Fibers From Textiles in Fish and Bivalves Sold for Human Consumption. Sci. Rep. 5, 1–10. doi: 10.1038/srep14340
Rodriguez L. F., Ibarra-Obando S. E. (2008). Cover and Colonization of Commercial Oyster (Crassostrea Gigas) Shells by Fouling Organisms in San Quintin Bay, Mexico. J. Shellfish. Res. 27, 337–343. doi: 10.2983/0730-8000(2008)27[337:CACOCO]2.0.CO;2
Romeo T., Pietro B., Pedà C., Consoli P., Andaloro F., Fossi M. C. (2015). First Evidence of Presence of Plastic Debris in Stomach of Large Pelagic Fish in the Mediterranean Sea. Marine. Pollut. Bull. 95, 358–361. doi: 10.1016/j.marpolbul.2015.04.048
Schoch C. L., Ciufo S., Domrachev M., Hotton C. L., Kannan S., Khovanskaya R., et al. (2020). NCBI Taxonomy: A Comprehensive Update on Curation, Resources and Tools. Database 1, 21. doi: 10.1093/database/baaa062
Schuchert P. (1998). The Marine Fauna of New Zealand: Athecate Hydroids and Their Medusae (Cnidaria: Hydrozoa). Oceanographic. Literature. Rev. 1, 102.
Poppe G. T., Goto Y. (1993). European Seashells: Scaphopoda. Bival-via, Cephalopoda, Verlag Christa Hemmen, Germany, 2, 221.
Seebens H., Gastner M., Blasius B., Courchamp F. (2013). The Risk of Marine Bioinvasion Caused by Global Shipping. Ecol. Lett. 16, 782–790. doi: 10.1111/ele.12111
Setälä O., Fleming-Lehtinen V., Lehtiniemi M. (2014). Ingestion and Transfer of Microplastics in the Planktonic Food Web. Environ. Pollut. 185, 77–83. doi: 10.1016/j.envpol.2013.10.013
Sharmila G., Muthukumaran C., Kumar N. M., Sivakumar V., Thirumarimurugan M. (2020). Food Waste Valorization for Biopolymer Production. Curr. Develop. Biotechnol. Bioeng., 233–249. doi: 10.1016/B978-0-444-64321-6.00012-4
Teeraphatpornchai T., Nakajima-Kambe T., Shigeno-Akutsu Y., Nakayama M., Nomura N., Nakahara T., et al. (2003). Isolation and Characterization of a Bacterium That Degrades Various Polyester-Based Biodegradable Plastics. Biotechnol. Lett. 25, 23–28. doi: 10.1023/A:1021713711160
Thiel M., Luna-Jorquera G., Álvarez-Varas R., Gallardo C., Hinojosa I. A., Luna N., et al. (2018). Impacts of Marine Plastic Pollution From Continental Coasts to Subtropical Gyres—Fish, Seabirds, and Other Vertebrates in the SE Pacific. Front. Marine. Sci. 5, 238. doi: 10.3389/fmars.2018.00238
Thompson J. R., Rivera H. E., Closek C. J., Medina M. (2015). Microbes in the Coral Holobiont: Partners Through Evolution, Development, and Ecological Interactions. Front. Cell. Infect. Microbiol. 4, 176. doi: 10.3389/fcimb.2014.00176
Utermann C. (2021). Ciona Intestinalis in the Spotlight of Metabolomics and Microbiomics: New Insights Into its Invasiveness and the Biotechnological Potential of its Associated Microbiota (Universitätsbibliothek Kiel, Faculty of Mathematics and Natural Sciences, Kiel University).
Utermann C., Blümel M., Busch K., Buedenbender L., Lin Y., Haltli B. A., et al. (2020). Comparative Microbiome and Metabolome Analyses of the Marine Tunicate Ciona Intestinalis From Native and Invaded Habitats. Microorganisms 8, 2022. doi: 10.3390/microorganisms8122022
Vannucci M., Rees W. (1961). A Revision of the Genus Bougainvillia (Anthomedusae) Vol. 11 (Boletim do Instituto Oceanográfico, University of Sao Paulo), 57–100.
Verlaque M. (1989). Contribution a La Flore Des Algues Marines De Méditerranée: Espèces Rares Ou Nouvelles Pour Les Côtes Françaises. Botanica Marina. 32, 101–113. doi: 10.1515/botm.1989.32.2.101
Vieira E. A., Flores A. A., Dias G. M. (2018). Persistence and Space Preemption Explain Species-Specific Founder Effects on the Organization of Marine Sessile Communities. Ecol. Evol. 8, 3430–3442. doi: 10.1002/ece3.3853
Vinod A., Sanjay M., Suchart S., Jyotishkumar P. (2020). Renewable and Sustainable Biobased Materials: An Assessment on Biofibers, Biofilms, Biopolymers and Biocomposites. J. Cleaner. Prod. 258, 120978. doi: 10.1016/j.jclepro.2020.120978
von Ammon U., Wood S. A., Laroche O., Zaiko A., Lavery S. D., Inglis G. J., et al. (2019). Linking Environmental DNA and RNA for Improved Detection of the Marine Invasive Fanworm Sabella Spallanzanii. Front. Marine. Sci. 6, 621. doi: 10.3389/fmars.2019.00621
von Ammon U., Wood S. A., Laroche O., Zaiko A., Tait L., Lavery S., et al. (2018). The Impact of Artificial Surfaces on Marine Bacterial and Eukaryotic Biofouling Assemblages: A High-Throughput Sequencing Analysis. Marine. Environ. Res. 133, 57–66. doi: 10.1016/j.marenvres.2017.12.003
Wang Q., Garrity G. M., Tiedje J. M., Cole J. R. (2007). Naive Bayesian Classifier for Rapid Assignment of rRNA Sequences Into the New Bacterial Taxonomy. Appl. Environ. Microbiol. 73, 5261–5267. doi: 10.1128/AEM.00062-07
Wilcox C., Van Sebille E., Hardesty B. D. (2015). Threat of Plastic Pollution to Seabirds is Global, Pervasive, and Increasing. Proc. Natl. Acad. Sci. 112, 11899–11904. doi: 10.1073/pnas.1502108112
Wood S. A., Smith K. F., Banks J. C., Tremblay L. A., Rhodes L., Mountfort D., et al. (2013). Molecular Genetic Tools for Environmental Monitoring of New Zealand's Aquatic Habitats, Past, Present and the Future. New Z. J. Marine. Freshwater. Res. 47, 90–119. doi: 10.1080/00288330.2012.745885
Wyatt A. S., Hewitt C. L., Walker D. I., Ward T. J. (2005). Marine Introductions in the Shark Bay World Heritage Property, Western Australia: A Preliminary Assessment. Diversity Distributions. 11, 33–44. doi: 10.1111/j.1366-9516.2005.00109.x
Xu J., Guo B. H. (2010). Poly (Butylene Succinate) and its Copolymers: Research, Development and Industrialization. Biotechnol. J. 5, 1149–1163. doi: 10.1002/biot.201000136
Zaiko A., Schimanski K., Pochon X., Hopkins G. A., Goldstien S., Floerl O., et al. (2016). Metabarcoding Improves Detection of Eukaryotes From Early Biofouling Communities: Implications for Pest Monitoring and Pathway Management. Biofouling 32, 671–684. doi: 10.1080/08927014.2016.1186165
Zettler E. R., Mincer T. J., Amaral-Zettler L. A. (2013). Life in the “Plastisphere”: Microbial Communities on Plastic Marine Debris. Environ. Sci. Technol. 47, 7137–7146. doi: 10.1021/es401288x
Zhang C., Garrison T. F., Madbouly S. A., Kessler M. R. (2017). Recent Advances in Vegetable Oil-Based Polymers and Their Composites. Prog. Polymer. Sci. 71, 91–143. doi: 10.1016/j.progpolymsci.2016.12.009
Keywords: bacterial and eukaryotic community structure, biosecurity, bio-polymer, DNA barcoding, marine plastic debris, non-indigenous species (NIS)
Citation: Audrézet F, Pochon X, Floerl O, Le Guen M-J, Trochel B, Gambarini V, Lear G and Zaiko A (2022) Eco-Plastics in the Sea: Succession of Micro- and Macro-Fouling on a Biodegradable Polymer Augmented With Oyster Shell. Front. Mar. Sci. 9:891183. doi: 10.3389/fmars.2022.891183
Received: 07 March 2022; Accepted: 13 May 2022;
Published: 22 June 2022.
Edited by:
Sedat Gundogdu, Çukurova University, TurkeyReviewed by:
Ana Luzia De Figueiredo Lacerda, UMR7093 Laboratoire d’océanographie de Villefranche (LOV), FranceSumer Singh Meena, Dr. B. R. Ambedkar National Institute of Technology Jalandhar, India
Arzu Karahan, Middle East Technical University, Turkey
Copyright © 2022 Audrézet, Pochon, Floerl, Le Guen, Trochel, Gambarini, Lear and Zaiko. This is an open-access article distributed under the terms of the Creative Commons Attribution License (CC BY). The use, distribution or reproduction in other forums is permitted, provided the original author(s) and the copyright owner(s) are credited and that the original publication in this journal is cited, in accordance with accepted academic practice. No use, distribution or reproduction is permitted which does not comply with these terms.
*Correspondence: François Audrézet, RnJhbmNvaXMuQXVkcmV6ZXRAY2F3dGhyb24ub3JnLm56