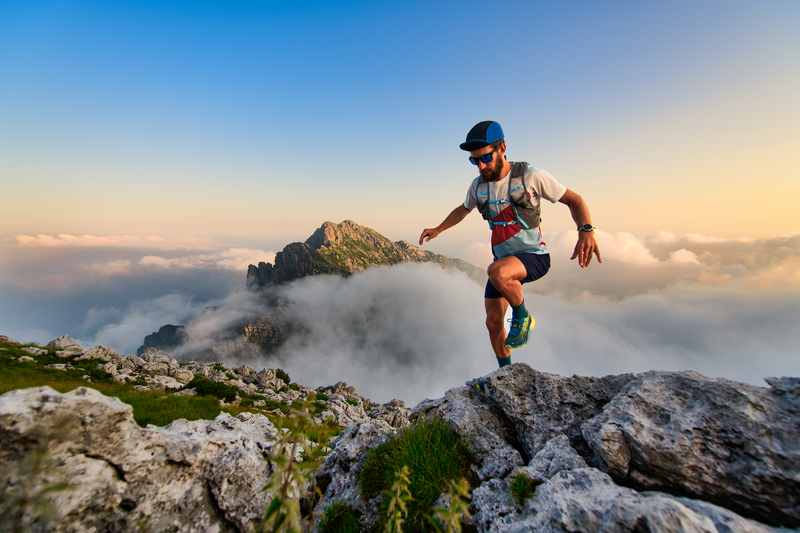
95% of researchers rate our articles as excellent or good
Learn more about the work of our research integrity team to safeguard the quality of each article we publish.
Find out more
ORIGINAL RESEARCH article
Front. Mar. Sci. , 13 May 2022
Sec. Marine Biology
Volume 9 - 2022 | https://doi.org/10.3389/fmars.2022.880112
This article is part of the Research Topic Effects of Environmental Stress on Marine Mollusks: Biomarkers, Physiological Responses and Underlying Mechanisms View all 7 articles
Extremely high temperature and hypoxia are mounting problems affecting the world’s coastal waters under the global warming environment, with severe consequences for marine life. Scallop is one of the most fragile species to hypoxia stress for their high respiration rate and lack of self-protection ability such as long-time shell closing. Circulatory physiology rhythm is sensitive to environmental stress and is an important means for shellfish to quickly respond to environmental stress. Therefore, monitoring the circulatory physiology indexes of Chlamys nobilis under hypoxia and high temperature stress is helpful to quickly diagnose its stress state and reveal the response process of circulatory system to the stress. In this study, using real-time Doppler ultrasonography technique, we continuously monitored the circulatory physiological indexes [heart rate (HR), blood flow volume (FV), blood velocity (PS and ED), resistance index (RI) and S/D ratio) of the scallop organs (gill, mantle and adductor muscle) under hypoxia (mild 4 mg/L DO, moderate 3 mg/L DO and severe 2 mg/L DO)] and fluctuating high temperature stress (29-31°C). Important metabolic function indexes [adenosine triphosphate (ATP), pyruvate kinase (PK) and cytochrome C oxidase (COX)] of various tissues were measured simultaneously. The results show that scallops are very sensitive to the changes of temperature and dissolved oxygen. Both high temperature and hypoxia will increase the HR, and severe hypoxia will bring greater load to the heart of the scallop. Hypoxia stress mainly caused the changes of PS, and the RI and S/D of the gill gradually increased. At 2mg/L DO level, the total blood flow of the mantle and adductor muscle decreased, but the blood flow supply in the gill was stable. The scallop is very sensitive to the change of high temperature and can make adjustments immediately. High temperature increased blood flow in various organs and preferentially supplied to the gill. The RI and S/D of branchial vessels under high temperature were lower than those under hypoxia. The ATP content of the mantle was continuous accumulated under high temperature and was significantly higher than that of the gill and adductor muscle, indicating the specificity of tissue metabolism. The overall circulatory physiological indexes of the gill showed a more positive response to the stress environment than the mantle and adductor muscle. All the results proved that the gill has the best resistance to the hypoxia and high temperature stress. Our study revealed the circulatory physiology regulation mechanism of the noble scallop C. nobilis under environmental stress, and provide effective data and technique supports for the timely diagnose of stress condition of the species, so as to formulate necessary handling strategies for the scallop farm.
The noble scallop Chlamys nobilis is an important cultivated bivalve in Asian coastal countries, with the advantages of fast growth, high profit and rich nutrition. Since the 1980s, it has gradually become an important economically cultured shellfish in the coastal areas of southern China (Tan et al., 2020; Ye et al., 2021). In recent years, the mortality of noble scallop C. nobilis from July to September is as high as 60-80% (Cheng et al., 2020), and high temperature and hypoxia are the main causes of large-scale death. The optimum growth temperature range of noble scallop C. nobilis is 23.3-27.9°C (Liu et al., 2011), while the water temperature in summer along the southeast coast of China frequently exceed 30°C from May to June. Yang et al., 2021 found that the dissolved oxygen concentration was linearly negatively correlated with the temperature of surface water and bottom water (P<0.01), which means that the stress of high temperature and hypoxia on scallops may occur simultaneously. Previous studies mainly focus on the effects of temperature gradient changes on shellfish physiology and metabolism, whereas detailed study of circulatory physiology and tissue metabolism response in noble scallop C. nobilis under fluctuating hyperthermia and acute hypoxia is lacking. The study is essential to provide data and technique supports for the timely diagnose of stress condition of the species, so as to formulate necessary handling strategies for the scallop farm.
Hypoxia has emerged as a major threat to the global coastal ecosystem (Vaquer-Sunyer and Duarte, 2008), and may form coastal hypoxia “dead zones” (Breitburg et al., 2018). Water with dissolved oxygen (DO) levels below 2.0 mg/L are generally defined as anoxic water bodies (Fennel and Testa, 2019). However, in many studies, water bodies with dissolved oxygen greater than 2.0 mg/L but not conducive to the survival of marine organisms are also called anoxic water bodies (Li, 2019). Grimes et al. (2021) described the dissolved oxygen concentration of 4.5 mg/L as mild hypoxia, 2.5 mg/L as moderate hypoxia and 1 mg/L as severe hypoxia in the study of corallivorous fireworms (Hermodice carunculata). Hypoxia affects shellfish behavior, metabolism, survival and growth (Zhang, 2020). Shen (2018) also pointed out that hypoxia will affect the metabolism and growth of abalone. In Sansha bay, Fujian Province, a reduction in dissolved oxygen led to mass mortality of farmed scallops (Wang et al., 2014). The noble scallop C. nobilis has poor tolerance to oxygen fluctuations, and most studies focus on the adaptation mechanism of shellfish under long-term hypoxia stress, and there are few studies on the physiological changes of it under the threat of acute hypoxia.
Water temperature increase in coastal areas are more pronounced than in open ocean waters (Alsterberg et al., 2011). High temperatures can accelerate microbial consumption of dissolved oxygen in water bodies, which result in severe hypoxia (Tomasetti & Gobler, 2020), subsequently lead to local extinctions and biodiversity loss at coastal areas (Godbold & Solan, 2013). The timely and effective physiological regulation response of shellfish is an important means to make it through the adverse period smoothly under the extreme stress environment. Among them, the physiological function response of heart and hemolymph circulation respond the fastest, and the adjustment of metabolic physiological balance is an important aspect to ensure the homeostasis of the body. The hemodynamics of Chlamys farreri under thermal stress revealed the quick response of the circulatory physiological function (Xu et al., 2019), and sustained high temperature can cause serious damage to the physiological and metabolic functions of Crassostrea virginica and Mytilus galloprovincialis (Anestis et al., 2007; Matoo et al., 2013). Xing et al. (2019) found that when the temperature increased from 5°C to about 30°C, the heart rate of C. farreri increased at a rate of 1.73 ± 0.04 bpm/°C, but decreased rapidly at 34°C; Götze et al., 2020 confirmed that high temperature stress can shift the metabolism of scallops to an anaerobic pathway; Hu et al., 2022 found that heat stress could affect the TCA cycle in shellfish.
The circulatory system includes the cardiovascular system and the lymphatic system (Li et al., 2006). The cardiovascular system consists of the heart, arteries, capillaries and veins. It provides oxygen, various nutrients and hormones to organs and tissues through blood flow, and transports tissue metabolic wastes to excretory organs to maintain the homeostasis of the internal environment, metabolism and normal life activities (Pittman, 2011). Heart rate and hemodynamics have been proved to be indicators of the overall physiological condition of the body. Heart rate in bivalves is an easily monitored physiological index, and environmental fluctuation is the main factor affecting heart rate (Trueman et al., 1973; DeFur and Mangum, 1979). Gurr et al. (2018) found that the heart rate of scallops fluctuated with oxygen level under circadian hypoxia. Invertebrates increase the rate at which the body acquires oxygen by increasing cardiac output (Grieshaber et al., 1993). Ultrasonography makes our observation of the internal structure of organisms more direct (Novelo and Tiersch, 2012). Doppler color ultrasonography can accurately monitor heart rate, blood flow direction, blood flow velocity, blood flow and vascular resistance without body hurt. Previously we used Doppler ultrasonography method and successfully revealed the effect of thermal stress on the hemodynamics of the scallop C. farreri (Hao et al., 2016; Xu et al., 2019). In this study, we also use the same technique to study the circulatory physiological changes of noble scallop C. nobilis under continuous high temperature and hypoxia stress.
In addition, the metabolism of organisms is tissue-specific, with different metabolic processes and responses to environmental stress in different parts (Le Moullac et al., 2007; Strahl et al., 2011; Tan et al., 2020). Key metabolic enzyme activity change can reflect the dynamics of vital metabolism process in tissue. Adenosine triphosphate (ATP) is the energy source of various metabolic reactions in cells (Hara & Kondo, 2015); Pyruvate kinase (PK) is an important rate limiting enzyme in anaerobic metabolism (Weber et al., 1996); Cytochrome C oxidase (COX) reflects the aerobic metabolic capacity of tissue (Ivanina et al., 2011). Liu et al. (2014) indicated that hypoxia may shift the metabolic pathway of animals to anaerobic metabolism. And hypoxia affects the rate and efficiency of ATP synthesis and suppresses COX gene expression in senna (Hochachka et al., 1996; Diaz and Breitburg, 2009; Woo et al., 2013). Heat stress can reduce the production of ATP (Hraoui et al., 2020), and the COX activity and ATP content of Dreisena polymorpha were significantly reduced under high temperature (Louis et al., 2020). Therefore, we selected three metabolic indicators (ATP, COX and PK) of the gills, mantle and adductor muscle of noble scallop C. nobilis and analyzed their level and activity, aiming to explore the impact of environmental stress on tissue metabolism and the possible correlation with the circulatory physiological dynamics.
The one-year old experimental scallops were obtained from a scallop raft-culture farm at Chengmai, north of Hainan Province. They were transported to the laboratory within 2 hours in cool and aerated seawater. Healthy scallops were cleaned and reared for acclimation in three 900 L circulating water culture system for a week (30-40 scallops in each system) under the conditions of natural light, water temperature of 25 ± 1°C and salinity of 31. Microalgae Isochrysis galbana (2×105 cell/L) was fed twice a day, and one-third of the seawater was replaced every two days to ensure water quality.
The Doppler ultrasonography system can measure a series of hemodynamic parameters (listed in Table 1). These indices can indicate several physiological situations of the circulatory system in different tissues, including the blood flow capacity, pumping capacity of the heart and whether blood vessels work well. The monitored ED values of the mantle and adductor muscle were discontinuous, so only the gill’s ED values were measured. Based on this, only the RI and S/D values of the gill were calculated.
Doppler ultrasonography system (FL-60181, Xuzhou Pyle Co., Ltd; Xuzhou, China) was equipped with an 8-12 MHz high frequency waterproof linear emission probe. The anterior and posterior auricle of the scallops were secured with plastic clips so that the opening was upward for easy monitoring. When measuring, the scanning mode was set to B mode (black & white), then the probe was put into the water with the position parallel to the two shells, carefully adjusted until clear image of the blood vessel was shown on the screen. Secondly, the scanning mode was set to C mode (color) to show the blood flow dynamic, then D mode (Doppler pulse) was switched on, and a 0.5mm sampling site was located at the middle of the vessel so as to acquire the most active blood flow dynamic image (Figure 1). One minute duration video was recorded for further detailed measurement.
Figure 1 Doppler ultrasonic imaging (pulse mode) of the scallop’s inner tissue and the branchial vessel blood flow dynamics. Red line shows the blood flow (upward) in afferent branchial vessel and blue line the blood flow (downward) in efferent branchial vessel. The short parallel lines (P) indicate the sampling site, at which the real-time blood flow dynamic is recorded at the lower part of the figure.
Adenosine triphosphate (ATP), cytochrome-C oxidase (COX) and pyruvate kinase (PK) were selected as metabolic indexes of scallop tissue under hypoxia and high temperature stress. The content of ATP in tissue reflects the state of energy supply. The activities of COX and PK reflect the aerobic and anaerobic metabolic capacity of tissue, respectively.
Three metabolic indexes were measured by commercial analyzing kits (Beijing Solarbio® biological company). After stress treatment, the scallops were dissected on ice, and the tissue samples (gill, mantle, adductor muscle) were quickly frozen in liquid nitrogen and thereafter stored in - 80°C refrigerator.
The experimental system for hypoxic stress effect comprised of a rearing tank (450L water volume, water temperature 25 ± 1°C, salinity 31 and 6.5 mg/L DO), a DO automatic control equipment (seawater acidification & hypoxia simulation system (WQ1002PD; Yantai Shenhong Measurement and Control Technology Co., Ltd.) and a nitrogen gas aerating system. We selected 20 scallops (shell height 8.35 ± 0.29; wet weight 60.69 ± 4.79 g) for the experiments. They were divided equally into four hypoxic stress treatment groups (five individuals each): mild (4.0 mg/L), moderate (3.0 mg/L), severe (2.0 mg/L), and extreme (1 mg/L) according to the ecologically defined hypoxic degree (Grimes et al., 2021). The scallops died quickly and no physiological index can be detected under the extreme hypoxia level (1 mg/L), so the other three treatments were tested. ‘Normal’ represent the scallop in the condition with the temperature of 26°C and 6.5 mg/L DO. Firstly, the normal circulating physiological values of the scallops were measured before aerating with nitrogen gas. Then, nitrogen aeration started and the dissolved oxygen level dropped within half an hour to the set DO concentration (the time was recorded as 0h). The circulatory physiological indexes of the scallop were measured at 2h, 4h, 6h, 8h and 10h. It was found that the circulatory physiological indexes of the scallop changed dramatically at severe hypoxia condition (2.0 mg/L DO), therefore, we then repeated the experiment with 2.0 mg/L DO to measure the metabolic indexes of three tissues.
According to the open sources mid-infrared SST data from NASA’s Earth Observing System Data and Information System (EOSDIS) (https://worldview.earthdata.nasa.gov), the highest SST at the scallop aquaculture sea area northeast of Beibu Gulf of China varied from 29 ± 0.5°C (night) to 31 ± 0.5°C (daytime) in May and June 2021, the hottest season. According to this, we set the thermal variation from 29 to 31 to simulate the in situ condition. Firstly, 35 scallops (shell height 5.31 ± 0.27cm, wet weight 20.96 ± 4.88 g) were put into the tank for experiment. The normal circulating physiological values and metabolic indexes of the scallop were measured. Then, the water was heated with a heating rod (200 W) from 26°C to 29°C at the rate of 1 °C per two days, another heating rod was put into the tank and the water temperature fluctuation experiment started. The heating time was automatically controlled by a timer. Water temperature began to rise at 7:30 from 29°C and reached 31 ± 0.5°C at 15:00, then maintained for 1 h. Then it dropped to 29 ± 0.5°C at 4:00 the next day and maintained until 7:30. The high temperature fluctuation experiment repeated for 3 days. We measured the circulatory physiological indexes of the scallop at 7:30 and 15:00 every day, randomly selected 5 scallops each time, and collected their tissues to measure metabolic indexes at the same time.
In order to better reflect the changes of physiological cycle indexes and metabolic indexes of scallops under stress, we classified the experimental results. In hypoxia stress experiment, HR and metabolic indexes (ATP, COX and PK) were expressed as relative rate of change, whereas the blood velocity (PS and ED), FV, RI and S/D were expressed as measured values. In high temperature stress experiments, blood velocity (PS and ED), FV, and metabolic indexes (ATP, COX and PK) were expressed as relative change rates, whereas the HR, RI, and S/D were expressed as measured values. The formula for calculating the relative rate of change in hypoxia and high temperature stress is shown in formula (1).
The experimental data were analyzed by SPSS 24.0 statistical software. The significant differences among each group were analyzed by one-way analysis of variance (ANOVA), combined with LSD test and independent T test, respectively (p<0.05). All variables were tested for normality of variance and homogeneity before analysis. Values are expressed as mean ± standard error (SE).
The results of the study showed that the heart rate of the scallops increased under all three dissolved oxygen conditions. The HR increase rate was the highest at 2.0 mg/L DO level (31.57-43.83%), and the lowest at 3 mg/L DO level (6.97-17.50%), indicating that scallops tend to increase the HR to elevate the circulatory efficiency to provide more oxygen under severe hypoxic condition (Figure 2). After 6 h, the increase rate of HR at 2mg/L showed a slightly decrease (Figure 2). Under the condition of 2mg/L DO, the overall increase of scallop HR was the largest, reaching the peak between 4h and 6h, and slightly decreased after 8h, but still increased by 35.91% compared with the normal value. However, it was strange that under the condition of 3mg/L DO, instead of 4 mg/L DO, the overall change range of scallop HR was the smallest, and was consistently elevated between 0 and 8h.
Figure 2 The relative change rate of HR with time under three hypoxia stress degrees (mean ± se, n = 5).
The changes of blood velocity in each organ under the three dissolved oxygen levels were basically consistent with the changes in HR, that is, the blood velocity was the highest at 2 mg/L DO, and the lowest at 3 mg/L DO (Figures 3A-C). The PS of adductor muscle was significantly higher than that of gill and mantle (P<0.05), but there was no significant difference between gill and mantle (Figures 3A-C). As dissolved oxygen decreased from 4 mg/L to 3 mg/L to 2 mg/L, compared to the respective normal PS values, the PS of the gills increased by an average of 52.70, 32.27 and 68.29 mm/s, the PS of adductor muscle increased by an average of 5.61, 21.54 and 116.75 mm/s, whereas the PS of mantle decreased by an average of -7.72 to -2.98 to -19.54 mm/s (Figures 3A-C). The ED of gill was less affected by the change of dissolved oxygen concentration and fluctuated steadily with time. As dissolved oxygen decreased, the ED of gill increased by an average of 5.61, 6.91 and 14.24 mm/s compared to the normal ED values (Figures 3A-C).
Figure 3 The changes of blood velocity of gill, mantle and adductor muscle under three dissolved oxygen levels [the solid line represents PS, dashed line represents ED, ED only monitored in the gill because the monitored ED values of the mantle and adductor muscle were discontinuous; (A) 4mg/L DO; (B) 3mg/L DO; (C) 2mg/L DO; means ± se, n = 5].
The Figure 4 shows the changes of blood supply and total blood supply in gill, mantle and adductor muscle tissues under different hypoxia levels. At the 4 mg/L DO level, the FV of three tissues and the total were relatively stable with time (Figure 4A). At the 3 mg/L DO level, the total FV decreased to 7.60 ml/min at 2 h and then gradually increased to 8.18 ml/min at 6 h. After 6 h, the total FV recovered gradually to 6.98 ml/min. The FV in the gill showed a similar dynamic to the total (from 2.51 to 2.02 ml/min with a peak value of 2.83 ml/min at 6h), whereas the FV change in the mantle and adductor muscle was not significant, which means that the FV increase in the gill contributes to the total FV elevation (Figure 4B). It implies that with the decrease of the DO, the scallop elevated the FV in the gill first to promote the DO acquisition to cope with the stress.
Figure 4 The stacked line chart of FV changes in gill, mantle, adductor muscle and total under three hypoxia stress degrees [(A) 4mg/L DO; (B) 3mg/L DO ; (C) 2mg/L DO ; means, n=5].
At the severe hypoxia treatment, the total FV and that in the mantle and adductor muscle reduced drastically (8.23 to 4.50 ml/min, 2.52 to 0.60 ml/min and 4.50 to 2.63 ml/min, respectively) during the acute DO drop from normal to 2 mg/L, and then they kept at a very low level during the following experiment period (Figure 4C). The elevation of FV in the gill was absent at 2 mg/L DO, which implied that the scallop couldn’t cope with the stress by elevating DO acquisition efficiency.
The results showed that under three hypoxia stress degrees, the RI and S/D of the gill had the same trend of change with time. At 4 mg/L DO level, the RI values of the gill increased significantly at 0h, then fluctuated increased and reached a peak at 10h (RI=0.70) (Figure 5A). At 3mg/L DO level, RI values increased as a whole but significantly decreased at 6 hours, the peak value also appeared at 10h (RI=0.70) (Figure 5B). At 2 mg/L DO level, the RI values decreased significantly at 2 h, and then rapidly increased and reached the peak value at 6h (RI=0.71), and then decreased slowly (Figure 5C). At 4 and 3 mg/L DO levels, the S/D values of the gill were the highest at 10 h, 3.38 and 3.35, respectively (Figures 5D, E). At 2 mg/L DO level, the S/D values at 6 h has reached 3.40 (Figure 5F). This means that severe hypoxia may damage the blood vessels in the gill earlier.
Figure 5 The changes resistance index (RI) and S/D ratio of gill, mantle and adductor muscle under three hypoxia stress degrees [(A) RI, 4mg/L DO; (B) RI, 3mg/L DO; (C) RI, 2mg/L DO; (D) S/D, 4mg/L DO; (E) RI, 3mg/L DO; (F) RI, 2mg/L DO; Normal: RI and S/D values under 6.5 mg/L DO level; means, n = 5].
According to the change process of circulating physiological indexes, the whole hypoxia stress process can be divided into three stages (0-2h) early stage, (4-6h) middle stage and late stage (8-10h). The scallop was under the greatest stress at 6 h, with the highest HR, the lowest total FV and the RI of gill vessels (Figure 2, Figure 4C and Figure 5C). Correspondingly, we found that the metabolic indexes of the three tissues were also remarkably different in the three periods, reflecting their response to hypoxic stress.
The study found that COX in the gill was always suppressed under hypoxic conditions (except for 6 h) (Figure 6B), and the PK value was significantly increased after 2 h, indicating that the anaerobic metabolic pathway was activated (Figure 6C). ATP was positively accumulated after 6h, indicating that the energy supply of gills was restored (Figure 6A). In the late state, the relative change rate of PK in gills decreased rapidly, from 314.06% (4h) to 31.25% (10h). COX activity also decreased by -56.34% (Figures 6B, C). It means that the aerobic and anaerobic metabolic capacity of gill tissue decreased at the same time.
Figure 6 The relative change rate of metabolic indexes [(A)ATP; (B)COX; (C)PK] of gill, mantle and adductor muscle under severe hypoxia (2mg/L DO) condition (means, n = 5).
The COX in the mantle kept at a negative state throughout the stress process, indicating a loss of aerobic capacity under hypoxic conditions (Figure 6B). The relative change rate of PK value increased by about 240% between 4 and 8 h, and the ATP content at this time also accumulated in the mantle, indicating that the energy supply recovered by the improvement of anaerobic metabolic capacity. However, at 10h, the relative change rate of PK value decreased to 30.73%, and the ATP was largely consumed in this period (Figures 6A, C). It indicated that the anaerobic metabolism of the mantle was inhibited and the energy supply tended to be unbalanced.
The relative change rates of COX and PK in the adductor muscle were significantly increased in the middle stage and significantly decreased in the late stage (P<0.05) (Figure 6). The ATP in adductor muscle was consistently consumed throughout the whole severe hypoxia stress process, and was largely consumed at 4-6h (Figure 6A). The simultaneous enhancement of COX and PK activities in the middle stage may mean that scallops are doing their best to improve their resistance to hypoxia stress (Figures 6B, C). However, although COX and PK activities increased simultaneously, the ATP in adductor muscle were still in depletion state, indicating that the metabolism in the muscle was completely disordered.
The normal HR of the scallop C. nobilis was 19 bpm at 26°C, which was significantly lower than that above 28°C (P<0.05) (Figure 7). Under the influence of fluctuating high temperature, the HR of scallops changes synchronously. HR peaked at around 15:00 (from 28 to 35 bpm) daily as the temperature reaching the highest, then along with the decreasing temperature, HR dropped to the minimum at 7:30 (from 35 to 28 bpm) daily (Figure 7). Even under high temperature stress, the circulatory rhythm of the scallop is still sensitively to the temperature fluctuations.
Figure 7 Changes in scallop HR under high temperature fluctuations (‘normal’ represent the scallop int the condition of 26°C and 6.5 mg/L DO) (mean ± SE, n=5).
FV increased in the gill, mantle and adductor muscle under the high temperature stress, with a marked decrease of the increase in FV on the third day, but still higher than that of normal state (26°C, 6.5 mg/L DO). The relative change rate of FV in the gill changed consistently with the fluctuations of high temperature, except at 15:00 on the third days, and the maximum increase of FV was 110.67%. On the third day, FV in the gill decreased significantly, but it was still 27.33% higher than that of scallop in the normal state (Figure 8). The FV of the mantle also changed with the fluctuation of temperature in the first two days, but the change rhythm was opposite to that of temperature. FV decreased from 83.31% to 61.60% on the third day, but the difference was not significant (Figure 8). However, different from the gill and mantle, FV in the adductor muscle continued to decrease with the fluctuation of temperature (from 78.72% to 20.03%), and only slightly increased to 25.52% at 15:00 on the third day (Figure 8).
Figure 8 The relative change rate of FV in the gill, mantle and adductor muscle under high temperature fluctuations (mean ± SE, n = 5).
PS and ED of the gill increased and decreased synchronously with the fluctuation of temperature, which was consistent with FV, and also decreased abnormally at 15:00 on the third day (Figure 9A). The increase of FV led to the improve of blood velocity in the gill, indicating the consistency of the changes of circulation physiological indexes under high temperature stress. The PS of the mantle was lower than the normal state on the first day, and increased by 30% compared with the normal state at 7:30 on the second day, and then decreased with the increase of temperature. The PS increased to 82.18% on the third day (Figure 9A). On the whole, the PS of the mantle also shows an opposite change rhythm with the temperature fluctuation (Figure 9A). Except that the PS of the adductor muscle decreased by - 15.14% compared with the normal state at 7:30 the second day, the PS of the adductor muscle was in a state of improvement at the rest of the experimental time, and the change trend was relatively stable, with an average increase of 38.46% (Figure 9A). Prolonged fluctuating high temperature disturbed blood velocity rhythms in the gill and mantle vessels.
Figure 9 (A) The relative change rate of blood velocity (PS and ED) of three tissues under high temperature fluctuations (mean, n = 5); (B) The resistance index (RI) of the gill under high temperature fluctuations (mean ± SE, n = 5); (C) The S/D ratio of the gill under high temperature fluctuations (mean ± SE, n = 5).
The results of the study showed that with the temperature increased from 26°C (normal state) to 29°C (at 7:30) and to 31°C (at 15:00) on the first day, the RI and S/D of the gill’s blood vessels increased rapidly, from 0.52 to 0.66 and 2.17 to 3.02, respectively (Figures 9B, C). With the temperature gradually dropped to 29°C at 7:30 in the second day, RI and S/D decreased significantly to 0.51 and 2.18, which were close to the normal state (Figures 9B, C). It shows that the gill has good physiological regulation function and is sensitive to temperature changes. However, in the following two days, RI and S/D continued to rise and did not change synchronously with temperature fluctuations (Figures 9B, C). The RI and S/D rose to 0.63 and 2.75 at the end of the experiment (Figures 9B, C). Although the RI and S/D values at this time were lower than those on the first day, their rising state means that prolonged high temperature may cause potential damage to the vascular function of the gill.
The ATP content of the mantle was continues accumulated during high temperature stress, which was significantly higher than the normal level, and the activity of COX continued to increase, indicating that the aerobic metabolism of the mantle was enhanced (Figures 10A, B). When the temperature increased from 26 to 29°C (at 7:30 on the first day), the change rate of ATP content was the largest (550.00%), and the mantle was very sensitive to temperature (Figure 10A). The change rate of ATP content in the mantle was significantly greater than that in the gill and adductor muscle (P<0.05) (Figure 10A).
Figure 10 The relative change rate of metabolic indexes (A ATP; B COX; C PK) of the gill, mantle and adductor muscle under high temperature fluctuations (means, n = 5).
The content of ATP in the adductor muscle also increased significantly by 126.67% at 7:30 on the first day (29°C), indicating that the adductor muscle can quickly generate ATP to cope with high temperature stress (Figure 10A). Subsequently, the increase rate of ATP gradually decreased, and dropped to -40.00% and -11.11 at 15:00 on the second day and 7:30 on the third day, respectively. ATP was consumed in large quantities, and the activity of COX was also remarkably reduced at this time. Subsequently, the activity of COX was significantly enhanced (P<0.05), and the supply of ATP was gradually restored (Figures 10A, B). The changes of ATP content in the gill under high temperature stress were small, and the significant decrease in ATP content on the second day indicated that the gill might be under high temperature stress at this time (Figure 10A).
Cardiac function is the most important in the circulatory system and is widely used as a representative of animal metabolism to reveal its immediate response to environmental changes (Trueman et al., 1973; Burnett et al., 2013; Chapperon et al., 2016). In our experiments, the relative change rates of the scallop’s HR under different dissolved oxygen levels were all positive, indicating that the HR would increase under hypoxic conditions to accelerate circulatory efficiency and obtain more oxygen. Under the condition of severe hypoxia (2 mg/L DO), the HR reached the highest, indicating that scallop was rapidly adjusting the circulatory rhythm to adapt to the stress. Extensive studies have been carried out on the cardiac response of bivalves under hypoxic stress (Bayne, 1971; Grieshaber et al., 1993). Gurr et al., 2018 found that heart rate and respiratory rates in bay scallop Argopecten irradians increased when dissolved oxygen was below 5 mg/L. This is consistent with our result.
The circulatory system transports blood to various organs to exchange nutrients and oxygen. Since the convective supply of oxygen is directly dependent on blood flow, the regulation of tissue oxygenation depends crucially on blood flow regulation (Pittman, 2011). In our study, moderate hypoxia (3mg/L DO) will stimulate the scallop to increase blood flow, especially to the gill, to acquire more oxygen from the water and transport to other tissues. Nicholson (2002) found that Perna viridis under hypoxia stress will increase blood output to maintain hemolymph circulation. But it is worth noting that the FV in each organ began to decrease after 6 h at 3mg/L DO, which indicates that prolonged moderate hypoxic stress brought a great load to the scallop’s circulatory system. FV in adductor muscle was remarkably higher than that in gills and mantle under three dissolved oxygen condition, which may be related to the resistance to the blood flow of different organs (Jorgensen et al., 1984).
At 2mg/L DO level, the overall blood flow of each organ decreased, the blood flow in the gill changed smoothly, whereas the blood flow of the mantle and adductor muscle decreased remarkably. This may be related to the change of blood vessel diameter. The blood vessel diameter of the gill increased by an average of 23.25%, whereas that of mantle and adductor muscle decreased by an average of 11.17% and 13.64%, respectively (unpublished data). Gill is the main respiratory organ of bivalves. Different from mantle and other organs, gill can maintain the respiratory stability of the whole body. Bivalves can obtain more oxygen by increasing heartbeat (perfusion) and expanding blood vessels in case of hypoxia (Bayne, 1971). The diameter of gill blood vessels of Mytilus edulis increases by 1.6-1.9 times in the face of hypoxia (González et al., 2019). The relationship between HR and FV was complex. Generally, the FV (ml/min) is decided by the HR, blood flow velocity and the diameter of the blood vessel. Under certain HR and blood flow velocity, if the vessel diameter increases, the FV will also elevate.
The blood flow velocity index of PS and ED can indicate the cardiac capacity and blood pumping ability and efficiency. ED is more sensitive to hemodynamic changes, while PS is less affected by Doppler artifacts, which is more suitable for dynamic blood flow regulation control system analysis (Rosengarten et al., 2001). PS is used as an index to judge whether the renal artery is damaged in medicine (Chen et al., 2014). Our study found that PS exhibited greater fluctuations under hypoxic stress, whereas ED was less affected. The PS of the gill elevated remarkably under mild hypoxia (4 mg/L DO), which shows that together with HR increment, the scallop was trying to increase its blood flow efficiency in the most important breathing organ to cope with hypoxia stress. Meanwhile, the PS fluctuation detected in the adductor muscle indicates that it is more vulnerable to the stress. Under moderate hypoxia conditions, the HR and PS in the three tissues didn’t elevate so much than mild condition (Figures 2, 3), yet FV in the gill and adductor tissue increased remarkably (Figure 4). It may be caused by the blood vessel expanding. More blood was redistributed to the gill for oxygen acquirement and to the adductor muscle to avoid tissue damage. When facing with severe hypoxia, although HR, PS of all tissues increased largely, the FV in the tissues were still inevitably reduced which caused by the shrinking of blood vessel (Figure 4). It means that the circulatory regulation failed and functional damage is inevitable under this stress degree.
The RI threshold and S/D threshold of the gill vessels of noble scallop C. nobilis under hypoxic conditions were slightly higher than that of C. farreri under normal conditions (RI: 0.50-0.63; S/D: 2.01-2.86) (Xu et al., 2019), but the resistance index (RI) and S/D ratio of gill increased over time, indicating potential tissue damage from prolonged exposure to hypoxic environments.
The results show that the metabolic regulation ability varied in different tissues under severe hypoxia (Venter et al., 2018). The gill has stronger self-regulation ability than others under hypoxia stress, and it can switch to anaerobic metabolic pathway timely, hence the energy supply ability is restored. This is also confirmed by the better RI and S/D indices of the gill under hypoxic stress. Moreover, the accumulation of ATP in the gill further alleviates the intensity of its anaerobic metabolism, further confirmed the better resistance of the gill to hypoxia stress among the three tissues.
The metabolic changes of the mantle are similar to that of the gill: the aerobic metabolic pathway is inhibited throughout the whole process, and the energy is completely supplied by anaerobic metabolic pathway. The self-regulation ability of the mantle is considered to be strong before 8h, yet the PK content in the mantle decreased rapidly at 10h, and the ATP accumulation also changed to consumption, which means that the long-term hypoxia brought great pressure on the anaerobic metabolism capacity of the mantle, and anaerobic metabolism could not provide enough energy. Continuous exposure to hypoxia may cause damage to the mantle, which corresponds to the greater potential damage of the mantle in cycling physiology experiments, but further research is needed.
When scallops avoid enemies and swim, the adductor muscle is responsible for strong and frequent opening and closing activities, and has a high demand for energy and oxygen (Chantler, 2006). Therefore, the adductor muscle may be more vulnerable to hypoxic stress. It is found that the tissue resistance of adductor muscle was the weakest and its self-regulation ability is very poor under hypoxia stress. It is proved by the results that the aerobic and anaerobic metabolic pathways didn’t effectively convert and the ATP content was always in depletion. Chantler (2006) found that adductor muscle of scallop mainly relies on anaerobic metabolism for energy supply in normal conditions, but our study didn’t find the effectively improved of anaerobic metabolism capacity. Under hypoxic conditions, the scallop showed an obvious escaping behavior with the shells clapping frequently, which probably responsible for the enormous energy in the adductor muscle. But the poor regulation ability of the tissue under severe hypoxia means that the scallop may lost its swimming ability to escape the “dead zone” to survive.
We found that the HR of scallops increased significantly when the water temperature increased from the optimum 26°C to 29°C. Moreover, the HR of the scallop fluctuated synchronously with temperature from 29°C to 31°C. This indicates that the noble scallop is also sensitive to temperature and can adjust the HR immediately, just like our previous study on the temperature scallop species C. farreri (Xu et al., 2019). Bivalves are very sensitive to temperature changes, and HR can be used as an indicator of their thermal tolerance (Xing et al., 2019). High HR relates to accelerated circulation rhythm and is essential to meet the need of faster body metabolism rate under thermal stress, yet if the duration of the stress exceed the limit, cardiac disorder caused by overburden may occur and would finally cause heart failure (Nicholson, 2002; Lannig et al., 2008; Xu et al., 2019). In present study, cardiac disorder or failure didn’t occur during our two and a half day’s experiment, and the reason may be that instead of a continuous extreme high temperature (31°C) stress, the water temperature fluctuated from 31°C to 29°C each day, just like the diurnal changing situation in the wild. It is vital for the scallop to have a breath and recover.
The blood velocity of each organ was also affected by the fluctuation of high temperature. Within the first two and a half days, the changes of the gill were consistent with temperature fluctuations, whereas the mantle blood velocity is negatively correlated with temperature fluctuation, which indicates that the regulation circulatory physiology is tissue-specific (Figure 9). At 15:00 on the third day, the blood velocity indexes of the gill and mantle appeared disorder at the same time, indicating that the regulation has lost effect. The relative change rate of PS in the adductor muscle was relatively stable as a whole, and only decreased significantly at 7:30 on the second day, indicating that high temperature fluctuations had little effect on it.
The FV in the gill, mantle and adductor muscle of the scallop will increase significantly under high temperature stress. The blood flow in the gill was significantly reduced on the third day, which may be related to the increase of RI and S/D of blood vessels, and the increase of vascular resistance will lead to the decrease of blood flow. The relative change rate of the FV in the gill was consistent with temperature fluctuations in the first two and a half days. It means that as the most important respiration organ, the body will preferentially provide sufficient blood flow to it to maintain the oxygen acquisition efficiency during thermal stress. This also explains why the changes of FV and PS in the mantle were opposite to those in the gill. Williams and Ponganis, 2021 also found that blood flow of marine mammals preferentially flows to vital organs such as the brain and heart when facing environmental stress. On the third day, the increase rate of FV in each organ decreased, which means that the scallop gradually adapted to fluctuating high temperature stress and do not need more blood flow to obtain oxygen.
The RI variation of scallop’s gill at 26°C ranged from 0.51 to 0.66, which is close to that of C. farreri under optimal water temperature of 8°C-26°C (Xu et al., 2019). On the first day, RI and S/D values were the highest, indicating that scallops were sensitive to temperature changes and had a stress response, and the increased blood flow brought great pressure to the vessels. The decrease in RI and S/D values at 7:30 in the second day proved that the regulation ability at this time was normal. However, the subsequent continuous increase of RI and S/D indicated that the pressure on the branchial vessels gradually increased and the possibility of potential injury increased. At the same time, the rising trend of RI and S/D also indicated that prolonged time of high temperature stress may cause more serious damage to the scallop. It is worth noting that the RI and S/D of the gill under 3-day high temperature stress were lower than those under 10 h hypoxia stress, indicating that hypoxia caused more serious damage to scallop.
The results of the study showed that with the temperature increased from 26°C (normal state) to 29°C (at 7:30) and to 31°C (at 15:00) on the first day, the RI and S/D of the gill’s blood vessels increased rapidly, from 0.52 to 0.66 and 2.17 to 3.02, respectively (Figures 9B, C). With the temperature gradually dropped to 29°C at 7:30 in the second day, RI and S/D decreased significantly to 0.51 and 2.18, which were close to the normal state (Figures 9B, C). It shows that the gill has good physiological regulation function and is sensitive to temperature changes. However, in the following two days, RI and S/D continued to rise and did not change synchronously with temperature fluctuations (Figures 9B, C). The RI and S/D rose to 0.63 and 2.75 at the end of the experiment (Figures 9B, C). Although the RI and S/D values at this time were lower than those on the first day, their rising state means that prolonged high temperature may cause potential damage to the vascular function of the gill.
Considering all the above results, we can infer that the scallop could gradually adapt to high temperature stress through circulatory physiological regulation, and the gill, as the main respiratory organ, was not seriously damaged. The scallops were maintained in good condition, indicating that the stress of the scallop caused by the fluctuating high temperature of 29-31°C was limited.
Under high temperature stress, the contents and activities of metabolites in various tissues of scallops changed continuously. The activity of COX in the mantle increased continuously under high temperature stress, and ATP also accumulated, indicating that the improvement of aerobic metabolism of the mantle could provide sufficient energy for it. The accumulation of ATP in the mantle was significantly higher than that in the gill and adductor muscle, which may be because the mantle is more sensitive to the changes of high temperature and requires a large amount of energy supply to withstand high temperature stress when it was continuously exposed to high temperature. This corresponded to the increase of circulating physiological indexes in the mantle. The improvement of circulatory physiological ability in the mantle requires a lot of energy support, so the body may give priority to meet its energy needs. Abele et al., 2002 found that the heat stress of organisms will enhance the ATP demand, which is consistent with our findings.
Adductor muscle mainly relies on anaerobic metabolism to provide energy. Under high temperature stress, its PK and COX activities were enhanced as a whole, indicating that its aerobic and anaerobic metabolism have been greatly improved (Figures 10B, C). The increase of PK activity in extremely warm environment reflects the enhancement of ATP demand (Feidantsis et al., 2009). However, the ATP content in the adductor muscle did not accumulate significantly (except at 7:30 on the first day), and was consumed at 15:00 on the second day and 7:30 on the third day. It shows that the energy demand of the adductor muscle under high temperature stress is greater, and the overall improved metabolic capacity can only maintain its energy consumption. The results show that the three tissues of the scallop can restore energy supply by regulating the metabolic capacity under the fluctuating high temperature stress of 29-31°C.
Under hypoxia stress, C. nobilis can increase heart rate and blood flow to obtain more oxygen. As the main respiratory organ, the gill will give priority to ensuring the stable supply of blood flow under the condition of severe hypoxia. Metabolic indexes also show that the regulation ability of the gill is stronger, whereas the regulation ability of the adductor muscle is the worst, which could not restore the energy supply. In the experiment of fluctuating high temperature stress, it is found that the scallop is very sensitive to the change of temperature and can make immediate regulation, which will also give priority to ensuring the blood flow supply of gill tissue. The mantle responds most strongly to temperature changes. The fluctuating high temperature of 29-31°C will not cause serious damage to the scallop, and the scallop can gradually adapt to high temperature stress through physiological and metabolic regulation. Therefore, during the cultivation of scallops, more attention should be paid to the change of dissolved oxygen concentration in water, because the damage caused by 10h hypoxia stress to scallop is more serious than three-day high temperature stress. High temperature stress left longer time to take countermeasures.
The raw data supporting the conclusions of this article will be made available by the authors, without undue reservation.
YH, conceptualization, methodology, validation, formal analysis, investigation, resources, data curation, writing - original draft, and visualization. YR, methodology, formal analysis, resources, investigation, and visualization. HW, methodology, validation, writing - review and editing, supervision. QX, funding acquisition, project administration, supervision, writing - review and editing, validation, methodology, and conceptualization. FG, conceptualization, validation, writing - review and editing, supervision. CS, Conceptualization, Methodology, Formal analysis, Investigation, Resources, Writing - Original Draft, Writing - Review & Editing, Visualization. AW, Conceptualization, Methodology, Project administration. All authors contributed to the article and approved the submitted version.
This study was sponsored by the National Natural Science Foundation of China (grant No. 31760757, 41766005) and the National Key R&D Project of China (No. 2019YFD0901304).
The authors declare that the research was conducted in the absence of any commercial or financial relationships that could be construed as a potential conflict of interest.
All claims expressed in this article are solely those of the authors and do not necessarily represent those of their affiliated organizations, or those of the publisher, the editors and the reviewers. Any product that may be evaluated in this article, or claim that may be made by its manufacturer, is not guaranteed or endorsed by the publisher.
Abele D., Heise K., Portner H. O., Puntarulo S. (2002). Temperature-Dependence of Mitochondrial Function and Production of Reactive Oxygen Species in the Intertidal Mud Clam Mya Arenaria. J. Exp. Biol. 205 (13), 1831–1841. doi: 10.1242/jeb.205.13.1831
Alsterberg C., Hulth S., Sundbäck K. (2011). Response of a Shallow-Water Sediment System to Warming. Limnol. Oceanogr. 56 (6), 2147–2160. doi: 10.4319/lo.2011.56.6.2147
Anestis A., Lazou A., Pörtner H. O., Michaelidis B. (2007). Behavioral, Metabolic, and Molecular Stress Responses of Marine Bivalve Mytilus Galloprovincialis During Long-Term Acclimation at Increasing Ambient Temperature. Am. J. Physiol-Regula. Integr. Comp. Physiol. 293 (2), R911. doi: 10.1152/ajpregu.00124.2007
Bayne B. L. (1971). Ventilation, the Heart Beat and Oxygen Uptake by Mytilus Edulis L. @ in Declining Oxygen Tension. Comp. Biochem. Physiol. Part A.: Physiol. 40 (4), 1065–1085. doi: 10.1016/0300-9629(71)90295-7
Breitburg D., Levin L. A., Oschlies A., Grégoire M., Chavez F. P., Conley D. J., et al. (2018). Declining Oxygen in the Global Ocean and Coastal Waters. Science, 359(6371). doi: 10.1126/science.aam7240
Burnett N. P., Seabra R., de Pirro M., Wethey D. S., Woodin S. A., Helmuth B., et al. (2013). An Improved Noninvasive Method for Measuring Heartbeat of Intertidal Animals. Limnology and Oceanography: Methods 11 (2), 91–100. doi: 10.4319/lom.2013.11.91
Chapperon C., Volkenborn N., Clavier J., Séité S., Seabra R., Lima L. P. (2016). “Exposure to solar radiation drives organismal vulnerability to climate: evidence from an intertidal limpet. J. Therm. Biol., 57, 92–100. doi: 10.1152/ajpregu.00499.2015
Cheng D., Liu H., Zhang H., Tan K., Ye T., Ma H., et al. (2020). Effects of Thermal Stress on Mortality and HSP90 Expression Levels in the Noble Scallops Chlamys Nobilis With Different Total Carotenoid Content. Cell Stress Chaperon. 25 (1), 105–117. doi: 10.1007/s12192-019-01052-5
Chen Q., He F., Feng X., Luo Z., Zhang J., Zhang L., et al. (2014). Correlation of Doppler Parameters With Renal Pathology: A Study of 992 Patients. Exp. Ther. Med. 7 (2), 439–442. doi: 10.3892/etm.2013.1442
DeFur P. L., Mangum C. P. (1979). The Effects of Environmental Variables on the Heart Rates of Invertebrates. Comp. Biochem. Physiol. Part A.: Physiol. 62 (2), 283–294. doi: 10.1016/0300-9629(79)90058-6
Diaz R. J., Breitburg D. L. (2009). “The Hypoxic Environment”, in Fish Physiology, vol. 27. (Academic Press), pp. 1–23. doi: 10.1016/S1546-5098(08)00001-0
Fennel K., Testa J. M. (2019). Biogeochemical Controls on Coastal Hypoxia. Annu. Rev. Mar. Sci. 11, 105–130. doi: 10.1146/annurev-marine-010318-095138
Feidantsis K., Pörtner H. O., Lazou A., Kostoglou B., Michaelidis B. (2009). Metabolic and Molecular Stress Responses of the Gilthead Seabream Sparus aurata During Long-Term Exposure to Increasing Temperatures. Mar. Biol. 156 (4), 797–809. doi: 10.1007/s00227-009-1135-z
Godbold J. A., Solan M. (2013). Long-Term Effects of Warming and Ocean Acidification are Modified by Seasonal Variation in Species Responses and Environmental Conditions. Philos. Trans. R. Soc. B.: Biol. Sci. 368 (1627), 20130186. doi: 10.1098/rstb.2013.0186
González P. M., Rocchetta I., Abele D., Rivera-Ingraham G. A. (2019). Hypoxically Induced Nitric Oxide: Potential Role as a Vasodilator in Mytilus Edulis Gills. Front. Physiol. 9. doi: 10.3389/fphys.2018.01709
Götze S., Bock C., Eymann C., Lannig G., Steffen J. B., Pörtner H. O. (2020). Single and Combined Effects of theDeadly TrioHypoxia, Hypercapnia and Warming on the Cellular Metabolism of the Great Scallop Pecten Maximus. Comp. Biochem. Physiol. Part B.: Biochem. Mol. Biol. 243, 110438. doi: 10.1016/j.cbpb.2020.110438
Grieshaber M. K., Hardewig I., Kreutzer U., Pörtner H. O. (1993). “Physiological and Metabolic Responses to Hypoxia in Invertebrates,” in Reviews of Physiology, Biochemistry and Pharmacology, vol. 125. (Berlin, Heidelberg: Springer). doi: 10.1007/BFb0030909
Grimes C. J., Petersen L. H., Schulze A. (2021). Differential Gene Expression Indicates Modulated Responses to Chronic and Intermittent Hypoxia in Corallivorous Fireworms (Hermodice Carunculata). Sci. Rep. 11 (1), 1–13. doi: 10.1038/s41598-021-90540-9
Gurr S. J., Goleski J., Lima F. P., Seabra R., Gobler C. J., Volkenborn N. (2018). Cardiac Responses of the Bay Scallop Argopecten Irradians to Diel-Cycling Hypoxia. J. Exp. Mar. Biol. Ecol. 500, 18–29. doi: 10.1016/j.jembe.2017.12.011
Hao J. H., Xu Q., Ru S. G., Yang H. S. (2016). Application of Digital Ultarsound Image to Copare the Circulation Physiology Between Chlamys Farrery and Patinopecten Yessoensis. Oceanolog. Limnol. Sin. 01), 29–35. doi: 10.11693/hyhz20150200044
Hara K. Y., Kondo A. (2015). ATP Regulation in Bioproduction. Microb. Cell Factor. 14 (1), 1–9. doi: 10.1186/s12934-015-0390-6
Hochachka P. W., Buck L. T., Doll C. J., Land S. C. (1996). Unifying Theory of Hypoxia Tolerance: Molecular/Metabolic Defense and Rescue Mechanisms for Surviving Oxygen Lack. Proc. Natl. Acad. Sci. 93 (18), 9493–9498. doi: 10.1073/pnas.93.18.9493
Hraoui G., Bettinazzi S., Gendron A. D., Boisclair D., Breton S. (2020). Mitochondrial Thermo-Sensitivity in Invasive and Native Freshwater Mussels. J. Exp. Biol. 223 (2), jeb215921. doi: 10.1242/jeb.215921
Hu Z., Feng J., Song H., Zhou C., Yang M. J., Shi P., et al. (2022). Metabolic Response of Mercenaria Mercenaria Under Heat and Hypoxia Stress by Widely Targeted Metabolomic Approach. Sci. Total. Environ. 809, 151172. doi: 10.1016/j.scitotenv.2021.151172
Jorgensen D. D., Ware S. K., Redmond J. R. (1984). Cardiac Output and Tissue Blood Flow in the Abalone, Haliotis Cracherodii (Mollusca, Gastropoda). J. Exp. Zoolog. 231 (3), 309–324. doi: 10.1002/jez.1402310303
Lannig G., Cherkasov A. S., Portner H. O., Bock C., Sokolova I. M. (2008). Cadmium-Dependent Oxygen Limitation Affects Temperature Tolerance in Eastern Oysters (Crassostrea Virginica Gmelin). Am. J. Physiol-Regula. Integr. Comp. Physiol. 294 (4), R1338–R1346. doi: 10.1152/ajpregu.00793.2007
Le Moullac G., Bacca H., Huvet A., Moal J., Pouvreau S., Van Wormhoudt A. (2007). Transcriptional Regulation of Pyruvate Kinase and Phosphoenolpyruvate Carboxykinase in the Adductor Muscle of the Oyster Crassostrea Gigas During Prolonged Hypoxia. J. Exp. Zoolog. Part A.: Ecol. Genet. Physiol. 307 (7), 371–382. doi: 10.1002/jez.390
Li Q. (2019). The Torlerance of Commercial Bivalves to Hypoxia-Taking the Ruditapes Philippinarun and the Chamys Farreri as Examples. phD thesis. Institute of Oceanology, Chinese Academy of Sciences.
Li Y., Park J. S., Deng J. H., Bai Y. (2006). Cytochrome C Oxidase Subunit IV is Essential for Assembly and Respiratory Function of the Enzyme Complex. J. Bioenerg. Biomembr. 38 (5-6), 283–291. doi: 10.1007/s10863-006-9052-z
Liu Z. G., Liu J. Y., Yang B. (2011). Interactions of Temperature and Salinity to the Survival and Growth of Chlamys nobilis (Reeve). Marine Science 35, 10, 75–80.
Liu C. C., Shin P. K., Cheung S. G. (2014). Comparisons of the Metabolic Responses of Two Subtidal Nassariid Gastropods to Hypoxia and Re-Oxygenation. Mar. Poll. Bull. doi: 10.1016/j.marpolbul.2014.03.013
Louis F., Rocher B., Barjhoux I., Bultelle F., Dedourge-Geffard O., Gaillet V., et al. (2020). Seasonal Monitoring of Cellular Energy Metabolism in a Sentinel Species, Dreissena Polymorpha (Bivalve): Effect of Global Change? Sci. Total. Environ. 725, 138450. doi: 10.1016/j.scitotenv.2020.138450
Matoo O. B., Ivanina A. V., Ullstad C., Beniash C., Sokolova I. M. (2013). Interactive Effects of Elevated Temperature and CO2 Levels on Metabolism and Oxidative Stress in Two Common Marine Bivalves (Crassostrea virginica and Mercenaria mercenaria). Comp. Biochem. Physiol. A Mol. Integr. Physiol. 164 (4), 545–553. doi: 10.1016/j.cbpa.2012.12.025
Nicholson S. (2002). Ecophysiological Aspects of Cardiac Activity in the Subtropical Mussel Perna Viridis (L.) (Bivalvia: Mytilidae). J. Exp. Mar. Biol. Ecol. 267 (2), 207–222. doi: 10.1016/S0022-0981(01)00362-8
Novelo N. D., Tiersch T. R. (2012). A Review of the Use of Ultrasonography in Fish Reproduction. North Am. J. Aquacult. 74 (2), 169–181. doi: 10.1080/15222055.2012.672370
Pittman R. N. (2011). “The Circulatory System and Oxygen Transport,” in Regulation of Tissue Oxygenation (Morgan & Claypool Life Sciences). doi: 10.4199/c00029ed1v01y201103isp017
Rosengarten B., Aldinger C., Kaufmann A., Kaps M. (2001). Comparison of Visually Evoked Peak Systolic and End Diastolic Blood Flow Velocity Using a Control System Approach. Ultras. Med. Biol. 27 (11), 1499–1503. doi: 10.1016/S0301-5629(01)00464-1
Shen Y. W. (2018). Effects of Hypoxia Stress on Surviva, Immune Physiology of Ablaone (Haliotis Discus Hannai and Its Hybrid With H.fulgens) and Primary Study on Molecular Basis(Master’s Thesis) (Xiamen University).
Strahl J., Dringen R., Schmidt M. M., Hardenberg S., Abele D. (2011). Metabolic and Physiological Responses in Tissues of the Long-Lived Bivalve Arctica Islandica to Oxygen Deficiency. Comp. Biochem. Physiol. Part A.: Mol. Integr. Physiol. 158 (4), 513–519. doi: 10.1016/j.cbpa.2010.12.015
Tan K., Zhang H., Lim L., Zheng H. (2020). Selection Breeding Program of Nan'ao Golden Scallop Chlamys Nobilis With Higher Nutritional Values and Less Susceptible to Stress. Aquaculture 517, 734769. doi: 10.1016/j.aquaculture.2019.734769
Tomasetti S. J., Gobler C. J. (2020). Dissolved Oxygen and Ph Criteria Leave Fisheries at Risk. Science 368 (6489), 372–373. doi: 10.1126/science.aba4896
Trueman E. R., Blatchford J. G., Jones H. D., Lowe G. A. (1973). Recordings of the Heart Rate and Activity of Molluscs in Their Natural Habitat. Malacologia 14 (1Á2), 377Á83. doi: 10.1016/0300-9629(71)90122-8
Vaquer-Sunyer R., Duarte C. M. (2008). Thresholds of Hypoxia for Marine Biodiversity. Proc. Natl. Acad. Sci. 105 (40), 15452–15457. doi: 10.1073/pnas.0803833105
Venter L., Loots D. T., Mienie L. J., Jansen van Rensburg P. J., Mason S., Vosloo A., et al. (2018). The Cross-Tissue Metabolic Response of Abalone (Haliotis Midae) to Functional Hypoxia. Biol. Open 7 (3), bio031070. doi: 10.1242/bio.031070
Wang H., Wei S. S., Yang Z., Zhang J., Wang A. J. (2014). Preliminary Study on DO Distributions and Hypoxia of Sansha Bay of FuJian in Summer. Trans. Oceanolog. Limnol. 3), 167–174. doi: 10.13984/j.cnki.cn37-1141.2014.03.023
Weber G., Convery H. J. H., Lea M. A., Stamm N. B. (1966). Feedback Inhibition of Key Glycolytic Enzymes in Liver: Action of Free Fatty Acids. Science 154 (3754), 1357–1360. doi: 10.1126/science.154.3754.1357
Williams C. L., Ponganis P. J. (2021). Diving Physiology of Marine Mammals and Birds: The Development of Biologging Techniques. Philos. Trans. R. Soc. B. 376 (1830), 20200211. doi: 10.1098/rstb.2020.0211
Woo S., Denis V., Won H., Shin K., Lee G., Lee T. K., et al. (2013). Expressions of Oxidative Stress-Related Genes and Antioxidant Enzyme Activities in Mytilus Galloprovincialis (Bivalvia, Mollusca) Exposed to Hypoxia. Zoolog. Stud. 52 (1), 1–8. doi: 10.1186/1810-522X-52-15
Xing Q., Zhang L., Li Y., Zhu X., Li Y., Guo H., et al. (2019). Development of Novel Cardiac Indices and Assessment of Factors Affecting Cardiac Activity in a Bivalve Mollusc Chlamys Farreri. Front. Physiol. 10. doi: 10.3389/fphys.2019.00293
Xu Q., Hao J., Gao F., Yang H. (2019). Effect of Temperature and Thermal Stress on the Hemodynamics of the Scallop Chlamys Farreri, as Indicated by Doppler Ultrasonography. J. Exp. Mar. Biol. Ecol. 510, 1–9. doi: 10.1016/j.jembe.2018.09.009
Yang B., Gao X., Zhao J., Liu Y., Xie L., Lv X., et al. (2021). Summer Deoxygenation in a Bay Scallop (Argopecten Irradians) Farming Area: The Decisive Role of Water Temperature, Stratification and Beyond. Mar. Poll. Bull. 173, 113092. doi: 10.1016/j.marpolbul.2021.113092
Ye T., Meng F., Tan K., Li L., Zhang G. F., Zheng H. P. (2021). Construction of Genetic Map and QTL Analysis of Carotenoid-Related Trait Based on EST Markers in Noble Scallop Chlamys Nobilis. Aquaculture 2021, 541. doi: 10.1016/j.aquaculture.2021.736775
Keywords: Chlamys nobilis, circulatory physiology, acute hypoxia, metabolism, thermal stress, scallop
Citation: Hao Y, Sun C, Rong Y, Wang H, Xu Q, Gao F and Wang A (2022) Circulatory and Metabolic Physiology Disorder in Different Organs of the Subtropical Scallop Species Chlamys nobilis Under Thermal and Hypoxia Stress, Revealed by Doppler Ultrasonography Technique. Front. Mar. Sci. 9:880112. doi: 10.3389/fmars.2022.880112
Received: 21 February 2022; Accepted: 18 April 2022;
Published: 13 May 2022.
Edited by:
Pengzhi Qi, Zhejiang Ocean University, ChinaReviewed by:
Muyan Chen, Ocean University of China, ChinaCopyright © 2022 Hao, Sun, Rong, Wang, Xu, Gao and Wang. This is an open-access article distributed under the terms of the Creative Commons Attribution License (CC BY). The use, distribution or reproduction in other forums is permitted, provided the original author(s) and the copyright owner(s) are credited and that the original publication in this journal is cited, in accordance with accepted academic practice. No use, distribution or reproduction is permitted which does not comply with these terms.
*Correspondence: Qiang Xu, eHVxaWFuZ2hudUBoYWluYW51LmVkdS5jbg==
†These authors have contributed equally to this work and share first authorship
Disclaimer: All claims expressed in this article are solely those of the authors and do not necessarily represent those of their affiliated organizations, or those of the publisher, the editors and the reviewers. Any product that may be evaluated in this article or claim that may be made by its manufacturer is not guaranteed or endorsed by the publisher.
Research integrity at Frontiers
Learn more about the work of our research integrity team to safeguard the quality of each article we publish.