- 1Department of Life Sciences, National Chung Hsing University, Taichung, Taiwan
- 2The integrative Evolutionary Galliform Genomics (iEGG) and Animal Biotechnology Center, National Chung Hsing University, Taichung, Taiwan
The milkfish, Chanos chanos, is an important aquaculture species that can be cultured in freshwater (FW) and seawater (SW) ponds because of its high euryhalinity. In winter, cold snap leading to high mortality of this tropical species is a critical issue for the aquaculture industry in Taiwan. Under hypothermal stress, however, changes in energy supply for osmoregulation of this euryhaline species is intriguing. In this study, we used an antibody against glycogen phosphorylase (GP) to identify glycogen-rich (GR) cells distributed adjacent to the mitochondria-rich (MR) ionocytes in milkfish gills. Glucose transporter 1 (GLUT1), which plays a major role in energy supply, was also identified and localized in GR cells. Moreover, the expression of indicators of aerobic metabolism and energy production, citrate synthase (CS) and cytochrome c oxidase (COX), were analyzed in gills of FW- and SW-acclimated milkfish to reveal different strategies of energy utilization under hypothermal stress. When exposed to a low-temperature environment, SW individuals used branchial glycogen and lactate to match the energy demands of aerobic metabolism in ionocytes, and elevated aerobic capacity to support and maintain normal functions in gills. However, branchial glycogen mainly localized in the GR cells of FW milkfish was not utilized under hypothermal stress. Meanwhile, a similar level of branchial COX activity and COXIV protein abundance at low temperatures between FW and SW milkfish indicated similar energy requirements in gills. This suggested that another source, but not branchial glycogen, maintained the energy demand in FW milkfish. The present study illustrated differential energy supply strategies in gills between SW- and FW-acclimated milkfish for osmoregulation under low temperatures.
Introduction
Environmental temperatures and salinities are two critical abiotic factors that affect the physiological responses and behavior of aquatic organisms. Under optimal temperature and salinity conditions, individuals have reduced energy needs to satisfy normal physiological demands and demonstrate high growth performance (Jana et al., 2006; Kang et al., 2015; Dahlke et al., 2020). According to different salinity environments, energetic costs for ionic homeostasis, appetite and absorption ability, and swimming behavior are important factors affecting the growth rate of fishes. Studies on several euryhaline teleosts have suggested that improved growth performance was found in brackish water conditions (8–20‰) because the ingestion of feed with some external water affects the appetite and energy absorption in fishes (Boeuf and Payan, 2001; Deane and Woo, 2009). Additionally, fishes are aquatic ectotherms; therefore, water temperature fluctuations directly affect the activities of metabolic enzymes, which leads to physiological responses in the whole individual (Barnes et al., 2014). Responses to temperature changes also affect the appetite and hormone secretion, which are the major factors that affect the energy obtained (Donaldson et al., 2008; Deane and Woo, 2009).
Gills, a specialized organ in aquatic species, have several important roles, including osmoregulation, acid-base regulation, nitrogen extraction, and gas exchange. The ionocytes distributed widely in the gill epithelium are mitochondria-rich (MR) cells that contain a large abundance of Na+, K+-ATPase (NKA), which is the primary driving force for other transporters to maintain osmotic homeostasis in fishes (Hiroi and McCormick, 2012; Yan and Hwang, 2019). In euryhaline fishes, acclimation to biotic or abiotic fluctuations should utilize large amounts of energy to maintain several ion transporters in gills for normal physiological responses (De Boeck et al., 2013; Chang et al., 2016; Roa and Tresguerres, 2019). The glycogen-rich (GR) cell is an accessory cell for the acute energy supply to ionocytes in teleosts. Branchial glycogen is predominantly stored in GR cells and is utilized under acute salinity treatment (Chang et al., 2007; Tseng et al., 2007; Hwang et al., 2011). Glycogen phosphorylase is a rate-limiting enzyme in glycogenolysis and an ideal indicator for identifying the localization of GR cells in the gill epithelium (Tseng et al., 2007). Branchial glycogen utilization has been studied in other fish species under different stress conditions, including Amazonian oscars (Astronotus ocellatus) under hypoxia, (De Boeck et al., 2013), blue-spotted mudskippers (Boleophthalmus pectinirostris) under low-salinity conditions (Guo et al., 2020), leopard shark (Triakis semifasciata) during starvation (Roa and Tresguerres, 2019), and tilapia (Oreochromis mossambicus) during seawater acclimation (Chang et al., 2007) or under cadmium exposure (Lin et al., 2011). In addition, glucose transporters have an energy supply function from circulating plasma glucose to support GR cells for glycogen storage and energy demands of ion homeostasis of mitochondria-rich ionocytes (Tseng et al., 2008; Tseng and Hwang, 2008; Balmaceda-Aguilera et al., 2012). In zebrafish, glucose transporter isoforms, including GLUT1a, GLUT6, and GLUT13.1, have been identified in NKA-, GR-, and H+-ATPase-rich (HR)-immunoreactive cells, respectively. These glucose transporters play different physiological roles in energy metabolism in the gills (Tseng et al., 2011). Furthermore, GR cells produce lactate as an acute-phase energy source for ionocytes through monocarboxylate cotransporters (MCTs) (Hwang et al., 2011; Tresguerres et al., 2020).
Aerobic metabolism in mitochondria requires an oxygen supply for optimal energy release. Citrate synthase (CS) and cytochrome c oxidase (COX) are two important factors for assessing the aerobic capacity in the citrate acid cycle (CAC) and the electron transport chain, respectively. Because ionocytes are MR cells, the abundance of these aerobic markers is high in these cells. CS is the first and rate-limiting enzyme in the citric acid cycle in mitochondria matrix and relative to provide intermediate for electron transporter chain and precursors of certain amino acids. COX is the complex IV of the electron transport chain located in the mitochondrial membrane. Moreover, CS requires oxygen to generate a proton electrochemical potential, in order to supply ATP synthase for ATP synthesis (Gagnon and Holdway, 1999; Webb et al., 2005). Studies have reported the existence of an energy supply system for ionocytes in stenohaline zebrafish and euryhaline tilapia (Chang et al., 2007; Tseng et al., 2008). The energy supply system plays an important role via aerobic metabolism to be the major source of energy for ionic homeostasis in branchial ionocytes of fishes upon adverse environmental temperature and salinity conditions.
Milkfish (Chanos chanos), an important aquaculture species in Southeast Asia, can be cultured in environments ranging from fresh water (FW) to seawater (SW). The optimal culture temperature in milkfish ranges from 20°C to 33°C, with the best results observed at 28°C (Martinez et al., 2006). In Taiwan, a large number of milkfish die during cold snap in winter, leading to enormous economic losses (Liao, 1991). The cold-tolerance ability of SW-acclimated milkfish was found to be better than that of FW-acclimated milkfish (Kang et al., 2015). Previous studies have reported that under hypothermal stress (18°C), branchial NKA activity of FW- and SW-acclimated milkfish was significantly decreased, leading to an imbalance in plasma chloride and sodium concentrations (Kang et al., 2015). Hepatic glycogen depletion and hepatocyte apoptosis occurring in hypothermal FW-acclimated milkfish might be the reasons why FW-acclimated milkfish exhibit lower tolerance to cold than SW-acclimated milkfish (Chang et al., 2018; Chang et al., 2021). Therefore, the energy supply system in milkfish is critical to support the energy demands of ionocytes in gills for osmoregulation under hypothermal stress, even though ionic homeostasis also includes several cellular physiological requirements. In this study, the localization of GR and MR cells in milkfish gills was specifically identified, and differential strategies for energy supply in gills between FW- and SW-acclimated milkfish under hypothermal stress were described.
Materials And Methods
Experimental Animals
The juvenile milkfish (C. chanos; standard length, 10.2 ± 0.3 cm; body weight, 13.7 ± 1.2 g) were purchased from a local fish farm in Tainan, Taiwan. Milkfish (N=40) were raised in FW and SW (35‰) (20 individuals in each environment) at 28.0 ± 0.5°C with a 12 h light/12 h dark photoperiod for at least four weeks. SW was prepared from local tap water with the Reef Sea Salts (Blue Treasure, New South Wales, Australia). The water in the experimental tanks was continuously re-circulated through fabric-floss filters and was partially renewed every two weeks. All fishes were fed daily with commercial pellets (Fwuso Industry, Taichung, Taiwan) to satiation at 15:00–16:00 during acclimation period. The hypothermal treatment was equipped with a cooling system (PF-225 M, PRINCE, Tainan, Taiwan) with an electric controller. The experimental milkfish (N=40) were transferred to two FW and two SW tanks holding 10 fish in each tank and acclimated for at least two days to reduce handling stress. The experimental groups were cooled down at a constant rate (2°C h-1) from 28°C to 18°C and subsequently maintained at 18°C for one week. The experimental individuals were fasted for one day before sampling. All surgical procedures were performed after anesthesia with 0.5% 2-phenoxyethanol, and all effects were made to minimize suffering and distress. The protocol for the experimental fish was reviewed and approved by the Institutional Animal Care and Use Committee (IACUC) of the National Chung Hsing University, Taiwan (IACUC Approval No. 107-165 to T.H.L.).
Antibodies
The following primary antibodies were used in this study: (1) Na+, K+-ATPase α1 subunit (α6F, DSHB, Iowa City, IA, USA); (2) Na+, K+-ATPase α subunit (α5, DSHB, Iowa City, IA, USA); (3) citrate synthase (sc-390693, Santa Cruz Biotechnology, Dallas, TX, USA); (4) COX IV (#4850, Cell Signaling, Beverly, MA, USA); (5) β-actin (GTX109639, Genetex, Irvine, CA, USA); (6) glycogen phosphorylase (GTX124390, Genetex); and (7) glucose transporter 1 (ab128033, Abcam; Figure S1).
Cryosectioning and Immunofluorescence Staining
The gills were fixed in 10% formalin overnight at 4°C. Subsequently, samples were washed with phosphate-buffered saline (PBS) and fixed with methanol for 2 h at 4°C. Samples were embedded in OCT compound-embedding medium (Leica Biosystems, Richmond, IL, USA) at –20°C. Cryosections (5 μm) were prepared using a cryostat (CM3050 S, Leica Biosystems, Nussloch, Germany) and were placed on poly L-lysine-coated slides (P8920, Sigma, St. Louis, MO, USA). The slides were blocked with 1% bovine serum albumin (BSA; Sigma) for 30 min. The slides were incubated first with primary antibody (diluted 1:100–1:200) overnight at 4°C and then incubated with second primary antibody (diluted 1:100–1:200) for 2 h at room temperature (26°C). Then, the slides were incubated with Alexa Fluor secondary antibodies (Alexa Fluor-488, Alexa Fluor-546, Invitrogen, Carlsbad, CA, USA) for 1 h at room temperature. Finally, samples were mounted in DAPI Fluoromount-G (SouthernBiotech, Birmingham, AL, USA) and examined using an optical microscope (Olympus BX50, Tokyo, Japan). Micrographs were taken using a cooling charge-coupled device (CCD) camera (DP72, Olympus) and cellSens software (Olympus).
Protein Extraction and Immunoblotting
The gills of milkfish were sampled, and the branchial arches were removed. Sampled gills were dissected quickly, immersed in liquid nitrogen, and stored at –80°C. The samples were then placed in SEID solution (150 mM sucrose, 10 mM EDTA, 50 mM imidazole, 0.1% sodium deoxycholate, pH 7.5) containing proteinase inhibitor (vol/vol: 25:1; Roche, Mannheim, Germany) and homogenized with a Polytron PT1200E homogenizer (Lucerne, Switzerland) at maximum speed on ice. The protein concentration was determined using reagents from the Protein Assay Kit (Bio-Rad, Hercules, CA, USA) using BSA (Sigma) as a standard.
Immunoblotting was performed according to the method of Chang et al. (2016). Aliquots containing 40 µg of hepatic homogenates were heated at 95°C for 5 min with 6× sample buffer. They were then fractionated by electrophoresis on sodium dodecyl sulphate (SDS) containing 14% polyacrylamide gel. Pre-stained protein molecular weight marker (#26616, Termo Fisher Scientific, Waltham, MA, USA) was applied in the electrophoresis. The fractionated proteins were transferred to 0.45-µm polyvinylidene difluoride (PVDF) blotting membranes (Millipore, Bedford, MA, USA). Membranes were pre-incubated for 1 h in phosphate-buffered saline with Tween-20 (PBST) with 5% (w/v) nonfat dried milk to minimize nonspecific binding. Blots were incubated overnight at 4°C with primary antibodies including citrate synthase (1:3000 dilution), COX IV (1:3000 dilution), glycogen phosphorylase (1:10000 dilution), glucose transporter 1 (1:5000 dilution), and β-actin (1:5000 dilution). They were then incubated at 28°C for 1 h with HRP-conjugated secondary antibody goat anti-rabbit IgG (1:10000; GTX23110-01; GeneTex). The Western chemiluminescent HRP substrate (EMD Millipore, Billerica, MA, USA) was used to develop the immunoblots. Images were photographed with a cool-charge-coupled device (CCD) camera (ChemiDoc XRS+; Bio-Rad) and analyzed with Image Lab v. 3.0 (Bio-Rad) to compare the numerical values with the relative immunoreactive band intensities.
Determination of Carbohydrate Contents in Gills
To determine the glucose content in gills, 10 mg of fresh gills were used and assayed using the Glucose Colorimetric/Fluorometric Assay Kit (K606-100, BioVision, Milpitas, CA, USA) following the manufacturer’s instructions. The gill samples were homogenized in a glucose assay buffer (K606-100-1, BioVision) on ice. The absorbance was measured using a VersaMax microplate reader (Molecular Devices, San Jose, CA, USA) at 570 nm and measured by serial dilution of the glucose standard (100 nmol/μL; BioVision).
For quantification of glycogen, 10 mg of fresh gills were homogenized in ddH2O and boiled for 10 min to inactivate all enzyme activities. The boiled samples were homogenized on ice and centrifuged at 13,000 × g for 10 min at 4°C to remove insoluble materials. The glycogen content was determined using the Glycogen Colorimetric/Fluorometric Assay Kit (K646-100, BioVision) following the manufacturer’s instructions. Four microliters of boiled gill homogenates were added to each well of a 96-well microplate, and the volume was adjusted to 50 μL with the hydrolysis buffer. Then, samples were incubated with the hydrolysis enzyme mix at 28°C for 30 min. Then, 50 μL of the reaction mix buffer was added to each well containing the samples and then incubated at 28°C for 30 min. The absorbance (OD570) was measured using the VersaMax microplate reader (Molecular Devices), and the glycogen content was calculated according to the standard curve prepared by serial dilution of glycogen (0.04, 0.08, 0.12, 0.16, and 0.20 μg in 50 μL of hydrolysis buffer; K646-100-1).
The lactate content of milkfish gills was determined using the Lactate Colorimetric/Fluorometric Assay Kit (K607-100, BioVision) according to the manufacturer’s instructions. Gill samples (10 mg) were homogenized in lactate assay buffer (K607-100-1, BioVision). The absorbance (OD570) was measured using the VersaMax microplate reader (Molecular Devices), and the lactate content was calculated according to the standard curve prepared by serial dilution of L-lactate standard (100 nmol/μL; BioVision).
Determination of Enzymatic Activity
The gills from each milkfish were dissected quickly, immersed in liquid nitrogen, and stored at –80°C for analysis of CS and COX activities. The CS activity (EC 2.3.3.1) of milkfish gills was determined using the Citrate Synthase Activity Colorimetric Assay Kit (K318-100, BioVision) following the manufacturer’s instructions. One hundred milligrams of milkfish gill was homogenized in 500 μL of CS assay buffer (K318-100-1, BioVision). The protein concentration of the gills was determined using the Protein Assay Kit (Bio-Rad) following the manufacturer’s instructions. The gill samples were assayed following the formula compared with the GSH standard (K318-100-4, BioVision). A 96-well microplate was analyzed in kinetic mode at 28°C and 18°C for 20 min using a VersaMax microplate reader (Molecular Devices) at OD412. The formula for calculating the CS activity was (U/mg of protein) = (B/ΔT·V)·D, where B is the number of nanomoles of the S-H group from the standard curve, ΔT is the reaction time (min), V is the sample protein added to the reaction well (mg), and D is the dilution factor.
The COX activity (EC 1.9.3.1) was calculated by a cytochrome c oxidation reaction according to the decrease in OD550 value using the Cytochrome Oxidase Activity Colorimetric Assay Kit (K287-100, BioVision). All subsequent steps were performed according to the manufacturer’s instructions. The gill samples were homogenized in the COX assay buffer (K287-100-1, BioVision). A 96-well microplate was analyzed in kinetic mode at 28°C and 18°C using a VersaMax microplate reader (Molecular Devices). COX activity (U/mg) = ΔOD550/Time (ΔT)/(7.04·mg protein).
Statistical Analysis
The quantitative values from the hypothermal and control groups were compared using two-way analysis of variance followed by Tukey’s honestly significant difference (HSD) post-hoc method in R software (version 3.4.2). The values were expressed as the mean ± standard error of the mean (SEM), and statistical significance was set at P < 0.05.
Results
Detection of Mitochondria-Rich Cells (Ionocytes) and Glycogen-Rich Cells in Gills of Milkfish
Immunofluorescent staining was performed to identify MR cells (ionocytes) and GR cells in the gill filaments of SW-acclimated milkfish. Two mitochondrial indicators, COX and CS, were found co-localized in the basolateral membrane (Figures 1A–C). The signal patterns of COXIV immunoreaction in the gill filaments were detected in the basolateral membrane of NKA-immunoreactive cells (MR cells and ionocytes) (Figures 1D–H). Notably, GP, as a marker of GR cells, was detected in GR cells near MR cells (Figure 1G). Furthermore, serial cryosectioning revealed that GLUT1, a marker of glucose availability in the gills, was identified and shown to be situated between MR cells (Figure 1H).
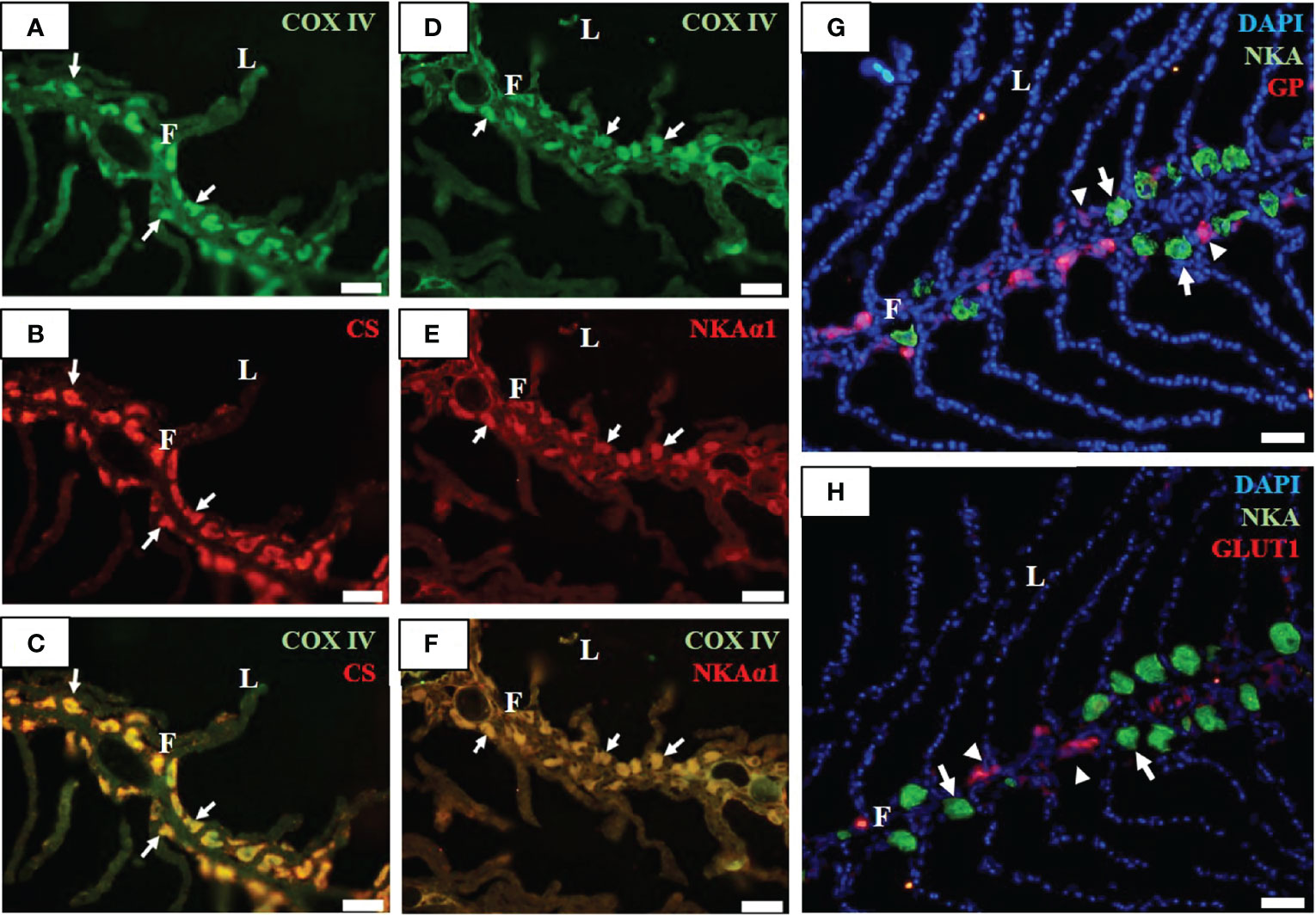
Figure 1 Immunofluorescence staining of gill filaments of milkfish stained with anti-cytochrome c oxidase (COX IV, green; (A, D), citrate synthase (CS, red; (B), sodium-potassium ATPase α1 subunit (NKAα1, red; (E), glycogen phosphorylase (GP, red; (G), sodium-potassium ATPase α subunit (NKA, green; (G, H), and glucose transporter 1 (GLUT1, red; (H) antibodies. Immunoreactions for the merged images (C) and (F) are shown from COX IV (A) with CS (B), and COX IV (D) with NKAα1 (E), respectively. F, filament; L, lamella. Scale bar, 50 μm.
Indicators of Energetic Supply Under Low-Temperature Treatment
To investigate the effect of low-temperature stress on the energy supply of milkfish gills, FW- and SW-acclimated milkfish were subjected to hypothermal treatment (18°C). GLUT1 and GP were used as indicators of glucose supply and glycogen utilization, respectively. The protein abundance of GLUT1 in both FW- and SW-acclimated milkfish gills was increased under hypothermal treatment (Figure 2A). Notably, compared to the FW group, the SW group exhibited significantly higher expression of GLUT1 at 18°C, whereas there was no obvious difference at 28°C. Under hypothermal treatment, the relative protein abundance of GP was upregulated in SW-acclimated milkfish gills; however, there was no significant change in FW-acclimated fish (Figure 2B).
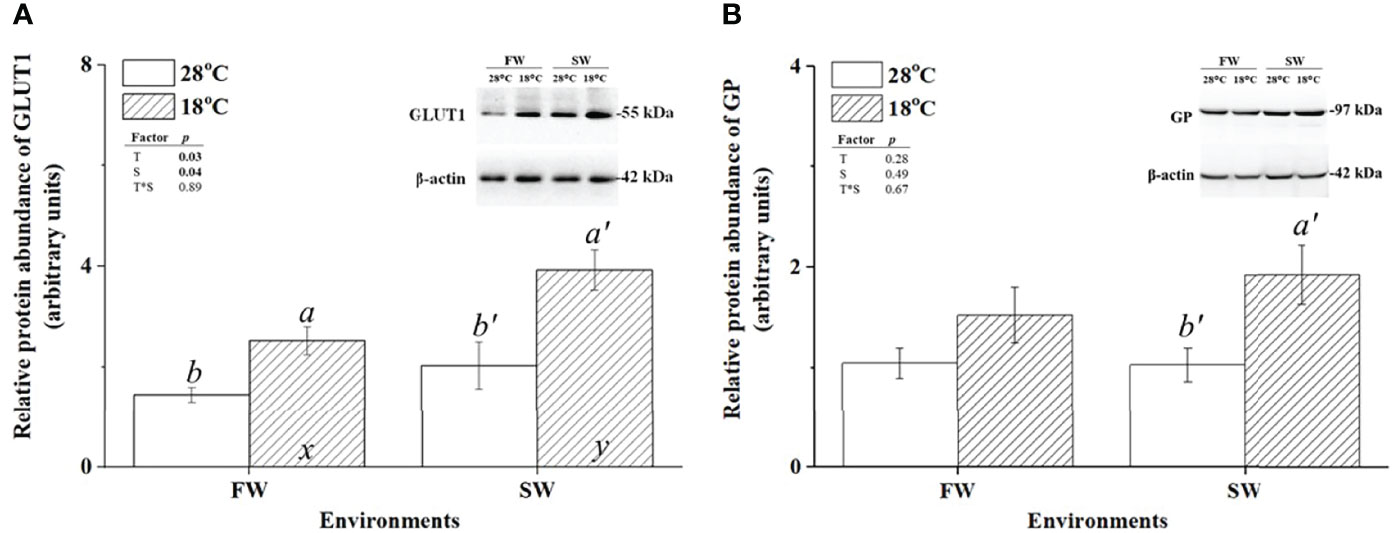
Figure 2 Relative protein abundance of branchial glucose transporter 1 (GLUT1) and glycogen phosphorylase (GP) in freshwater (FW)- and seawater (SW)-acclimated milkfish at 28°C (white bar) and 18°C (striped bar). Representative immunoblots of GLUT1 (A) and GP (B) show a single immunoreactive band. The single immunoreactive band of β-actin at 42 kDa was used as the loading control. Different letters in the FW milkfish (a, b) and SW milkfish (a’, b’) indicate significant differences between the normal and low-temperature groups. The x and y indicate significant differences between the FW and SW groups at 28°C or 18°C. Values represent the mean ± standard error of the mean (SEM), n = 9, P < 0.05.
To further confirm the hypothermal effects on glucose supply and glycogen utilization in gills of milkfish acclimated to different salinities, glycogen and glucose contents in gills were determined. The glucose content of SW-acclimated milkfish was increased under hypothermal stress, but no apparent change was observed in FW individuals (Figure 3A). Glycogen content was decreased in SW-acclimated milkfish under hypothermal stress but had no apparent effect on FW individuals (Figure 3B). Furthermore, we analyzed the lactate content in gills, which is another factor involved in energy supply and delivery under stressful conditions, according to a previous study (Chang et al., 2020). The lactate content in gills was downregulated in both FW and SW individuals under hypothermal stress (Figure 3C).
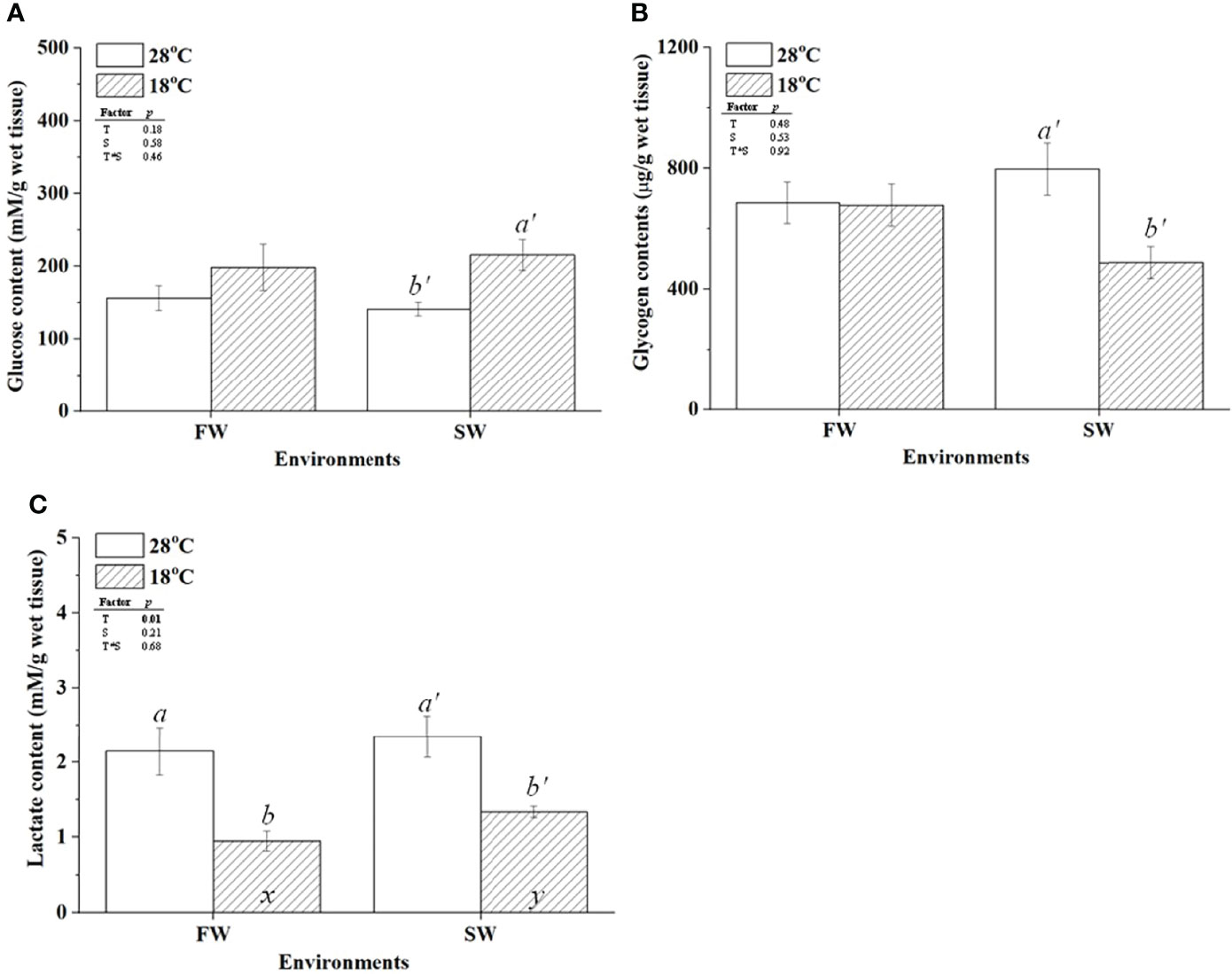
Figure 3 The contents of glucose (A), glycogen (B), and lactate (C) in freshwater (FW) and seawater (SW)-acclimated milkfish at 28°C (white bar) and 18°C (striped bar). Different letters in the FW milkfish (a, b) and SW milkfish (a’, b’) indicate significant differences between the normal and low-temperature groups. The x and y indicate significant differences between the FW and SW groups at 28°C or 18°C. Values represent the mean ± standard error of the mean (SEM), n = 9, P < 0.05.
Branchial Aerobic Metabolism
CS and COX were used as indicators of aerobic metabolism in the CAC cycle and the electron transporter chain of mitochondria, respectively. To investigate whether changes in energy supply were caused by branchial aerobic metabolism, the protein expression and activity of CS and COX in gills were analyzed. The protein abundance of CS was upregulated in FW-acclimated milkfish under hypothermal acclimation; however, CS levels were unchanged in SW individuals (Figure 4A). In addition, compared to the SW/18°C group, the FW/18°C group had a significantly higher expression level of CS. Nevertheless, the CS activity in FW-acclimated milkfish was downregulated under hypothermal stress, whereas no significant difference was found in SW individuals (Figure 5A). In both FW- and SW-acclimated milkfish, the protein abundance of COX IV was upregulated under hypothermal acclimation (Figure 4B). Meanwhile, the COX activity in FW-acclimated milkfish was higher than that in SW individuals at normal temperatures (28°C) (Figure 5B). However, COX activity in SW-acclimated milkfish was increased under hypothermal treatment, but that of FW-acclimated milkfish showed no apparent change (Figure 5B).
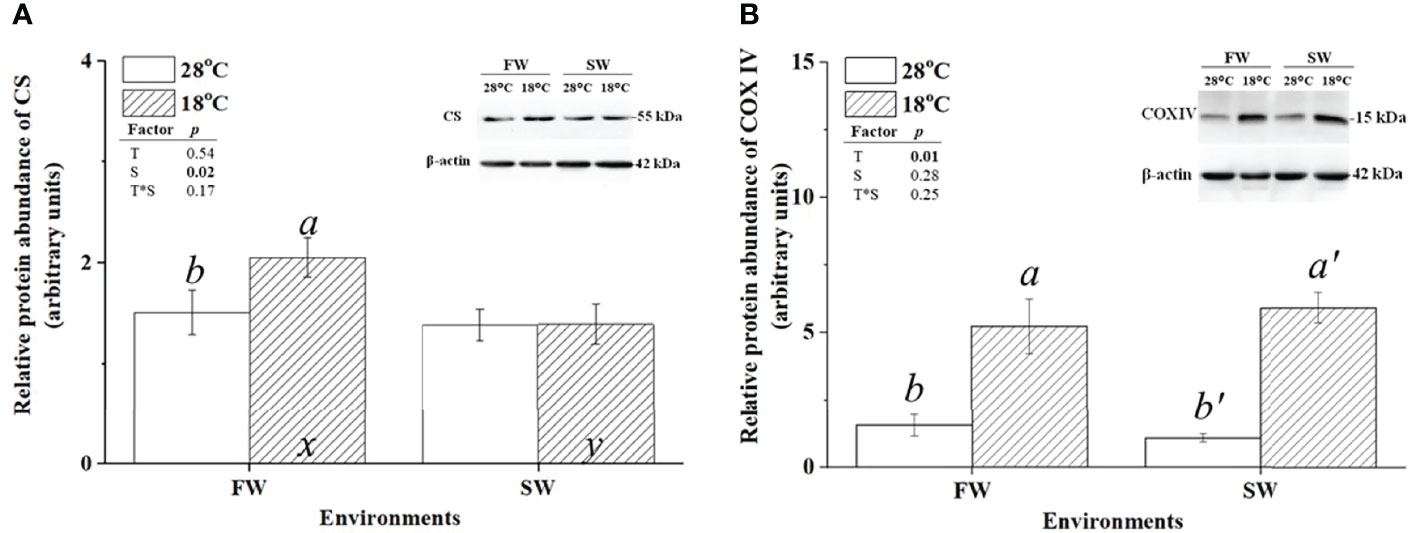
Figure 4 Relative protein abundance of branchial citrate synthase (CS) and cytochrome c oxidase IV (COX IV) in freshwater (FW)- and seawater (SW)-acclimated milkfish at 28°C (white bar) and 18°C (striped bar). Representative immunoblots of CS (A) and COX IV (B) show a single immunoreactive band. The single immunoreactive band of β-actin at 42 kDa was used as the loading control. Different letters in the FW milkfish (a, b) and SW milkfish (a’, b’) indicate significant differences between the normal and low-temperature groups. The x and y indicate significant differences between the FW and SW groups at 28°C or 18°C. Values represent the mean ± standard error of the mean (SEM), n = 9, P < 0.05.
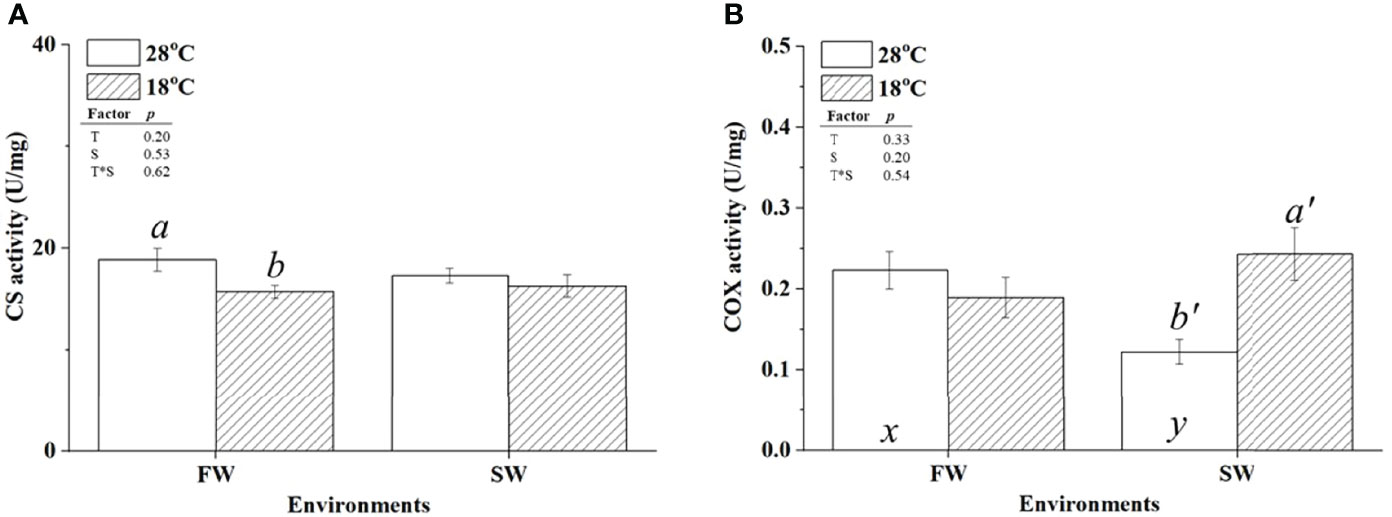
Figure 5 Activity levels of citrate synthase (CS) (A) and cytochrome c oxidase IV (COX IV) (B) in the gills of milkfish acclimated to 28°C (white bar) and 18°C (striped bar). Different letters in the FW milkfish (a, b) and SW milkfish (a’, b’) indicate significant differences between the normal and low-temperature groups. The x and y indicate significant differences between the FW and SW groups at 28°C or 18°C. Values represent the mean ± standard error of the mean (SEM), n = 9, P < 0.05.
Discussion
In this study, several indicators were used to identify MR and GR cells in milkfish gills. Among them, NKA is a traditional marker for MR cells (ionocytes). The mitochondrial markers COX and CS were co-localized with NKA in the gill filaments of milkfish. Immunolocalization of GP, a marker of GR cells, revealed that GR cells were localized next to the ionocytes. Notably, similar studies on immunostaining of glycogen particles in sharks showed that the content of glycogen was high in branchial NKA- and H+-ATPase-immunoreactive cells, indicating that glycogen was stored in MR cells in elasmobranch gills (Roa and Tresguerres, 2019). Compared to the findings in sharks, the accessory GR cells were found to supply energy to ionocytes in milkfish, zebrafish, and tilapia, which may be specific to teleostean gills (Chang et al., 2007; Tseng et al., 2011).
Glucose transporters (GLUTs) have glucose uptake capacity, as well as other functions that play a critical role in the energy supply system. Several isoforms of GLUTs are expressed in different cell types, such as NKA, HR, and GR cells, in gills. In zebrafish, the isoform GLUT6 identified in branchial GR cells had higher values of Michaelis constant (Km) and maximal velocity (Vmax) compared to those of the GLUT isoforms in ionocytes, GLUT1α and GLUT13.1. This result indicates that GLUT6 exhibits lower glucose absorption affinity to avoid competing with GLUT1α and GLUT13.1 for the energy substrate in ionocytes (Tseng et al., 2011). In this study, serial cryosectioning revealed the presence of the isoform GLUT1 in the gills of milkfish. GLUT1 was co-localized with GP and distributed in branchial GR cells (Figure S1A). In situ hybridization of glut1 in gill filaments was used to confirm the expression of GLUT1, and the results showed that glut1 mRNA was localized near NKA-IR cells (Figure S1B). Isoforms of GLUT have different distribution patterns in different species of teleosts. Interestingly, in mice, the Km and Vmax values of GLUT1 were higher than those of other GLUTs (Zhao and Keating, 2007). Further research is needed to assess if the isoforms of GLUTs in milkfish branchial cells have variable enzyme kinetics (Km and Vmax), indicating specific physiological importance.
Energy supply is required to support metabolic costs in the gills of teleosts when exposed to environmental fluctuations. Upon salinity challenge, glycogen utilization for energy demands of osmoregulation was identified in the gills of tilapia (Oreochromis mossambicus) and blue-spotted mudskippers (Boleophthalmus pectinirostris) (Chang et al., 2007; Tseng et al., 2007; Guo et al., 2020). Increasing requirements for glucose uptake and glycogen utilization in gills have been reported in moonlight gourami (Trichogaster microlepis), largemouth bass (Micropterus salmoides), and white shrimp (Litopenaeus vannamei) during the acute response to hypoxia (Martínez-Quintana et al., 2014; Hu et al., 2015; Yang et al., 2017). In this study, the increase in GLUT1 expression and glucose content in gills (Figures 2A and 3A) implied that branchial glucose uptake capacity in both FW- and SW-acclimated milkfish was upregulated under hypothermal stress. Moreover, the findings indicated that the branchial glucose uptake capacity in SW milkfish was greater than that in FW milkfish. Meanwhile, the increased expression of GP and degradation of branchial glycogen identified in SW-acclimated milkfish indicated that branchial glycogen was utilized in SW individuals, but not in FW individuals (Figures 2B, 3B). On the other hand, our previous study indicated that only FW individuals were found to utilize hepatic glycogen to maintain normal energy demands for the whole body under hypothermal stress (Chang et al., 2018). Taken together, these results suggested that when exposed to low-temperature condition, branchial and hepatic glycogen might be the source of energy supply for gills in SW-acclimated milkfish and FW-acclimated milkfish, respectively. Different environmental salinities could lead to diverse metabolic requirements of milkfish against hypothermal stress, thereby triggering different energy utilization strategies. Chang et al. (2018) also revealed that different environmental salinities did not influence the feed intake, which of both FW- and SW-acclimated milkfish was reduced under hypothermal stress, indicating that different energy utilization strategies between FW- and SW-acclimated milkfish were not induced by dietary intake. Meanwhile, this study showed that the lactate content in both FW and SW individuals under hypothermal stress was downregulated, suggesting that lactate was also used as an energy source in the branchial cells of milkfish. Moreover, Tseng et al. (Tseng et al., 2008) reported that lactate could be used as a fuel to maintain aerobic metabolism in the branchial ionocytes of tilapia upon salinity challenge. These findings with similar evidence illustrate that lactate is the form of energy transported to ionocytes from GR cells to supply energy demands for ionic homeostasis (Tseng and Hwang, 2008).
Changes in ambient temperatures affect the enzymatic activity of ectothermic teleosts. According to the Q10 temperature coefficient of the chemical reaction, enzyme activity should be reduced by half when the ambient temperature decreases by 10°C (Lefevre, 2016). Therefore, compensatory responses in protein abundance or enzyme activity are crucial for teleosts to maintain normal physiological functions and individual behavior upon hypothermal challenge (Hardewig et al., 1999). To enhance aerobic capacity in response to low-temperature stress, several species exhibit an increase in the mitochondrial density or aerobic enzyme content (Hardewig et al., 1999; Orczewska et al., 2010; Strobel et al., 2013). In addition, fluctuations in environmental salinities also affect aerobic metabolism and oxygen consumption of teleosts because less energy is required for the osmoregulation of various creatures under optimal habitat environments (Ern et al., 2014; Li et al., 2019). Our previous studies reported that although the oxygen consumption rate in SW milkfish exposed to 28°C was higher than that in FW individuals, SW milkfish exhibited lower branchial NKA protein expression and activity, which could reflect the energetic requirement for maintaining ionic homeostasis (Kang et al., 2015; Chang et al., 2019). In this study, CS and COX activities as indicators of aerobic metabolism and energy production were analyzed in the gills of milkfish under hypothermal stress. The branchial COX activity in gills of milkfish exposed to 28°C revealed similar patterns to NKA activity, indicating that the energy demands of ion regulation were lower in SW milkfish than in FW milkfish at normal temperature. The branchial COX protein abundance of both FW and SW milkfish was increased under hypothermal stress, whereas the branchial COX activity of SW milkfish was elevated to a similar capacity as that of FW milkfish, revealing that milkfish have compensatory responses in gills to increase the energy supply. However, a significant decrease in NKA activity was found in the gills of both FW and SW milkfish under hypothermal stress (Kang et al., 2015). The results reflected insufficient energy supply for ion regulation, which might be due to the enhanced energy requirement of gills to maintain other normal physiological functions, including antioxidant responses against low temperature-induced damages (Orczewska et al., 2010; Kang et al., 2015; Wu et al., 2015). Notably, in our previous study (Chang et al., 2019), we performed a similar analysis in the liver of milkfish and revealed different trends compared to those in gills. First, unlike in the gills of SW milkfish, COX activity and protein content in the liver remained stable under hypothermal stress. Next, in contrast to the stabilized CS protein abundance in livers of FW milkfish under hypothermal stress, CS protein abundance in FW milkfish gills was increased as a compensatory response to support activity in gills of hypothermal milkfish, despite its CS activity being slightly reduced. Furthermore, the CS : COX activity ratio, which reflects anabolic demand in mitochondria, increased in hypothermal FW milkfish livers but did not change in gills. Previous studies revealed that CS activity was upregulated in different tissues of teleosts at low temperatures, playing an important role in the biosynthesis of amino acids and lipids as well as maintaining aerobic metabolism to stabilize physiological mechanisms (McClelland et al., 2006; Orczewska et al., 2010; Strobel et al., 2013; Chang et al., 2019). Lipids and amino acids may be considered as supplementary fuels to support the normal demands of aerobic metabolism (Soengas et al., 2007; Speers-Roesch et al., 2016).
In conclusion, the present results suggested that compared to SW milkfish, FW milkfish have higher energy demands to maintain morphological features, mitochondria biosynthesis, and physiological functions in gills under hypothermal stress. The glycogen stored in livers and gills were found to be the source to support energy demands. However, under hypothermal stress, FW milkfish mainly used hepatic glycogen while SW milkfish used branchial glycogen for energy supply. The responses differed between FW and SW milkfish implied that FW- and SW-acclimated milkfish performed different energy utilization strategies under hypothermal stress. Notably, the muscle reserves in milkfish might be the other source of energy supply which was not analyzed under different conditions yet. Hence, more investigations are needed to check if the other source in milkfish, such as muscle reserves, was also involved in energy supply of gills.
Data Availability Statement
The original contributions presented in the study are included in the article/Supplementary Material. Further inquiries can be directed to the corresponding author.
Ethics Statement
The animal study was reviewed and approved by the Institutional Animal Care and Use Committee (IACUC) of the National Chung Hsing University, Taiwan (IACUC Approval No. 107-165 to T-HL).
Author Contributions
C-HC and C-JL contributed to manuscript writing. C-HC, W-JL, L-YW, and T-HL conceived discussed and designed this study. W-JL, L-YW, and Y-TL carried out the fish culture and experiments. C-JL and T-HL reviewed and edited the manuscript. T-HL supervised the projected. All authors read and approved the final manuscript for publication.
Funding
This research was partly supported by a grant to T-HL from the Ministry of Science and Technology (MOST) of Taiwan (108-2313-B-005-006-MY3) and in part by the integrative Evolutionary Galliform Genomics (iEGG) and Animal Biotechnology Center from The Feature Area Research Center Program within the framework of the Higher Education Sprout Project by the Ministry of Education (MOE) in Taiwan (109-S-0023-F).
Conflict of Interest
The authors declare that the research was conducted in the absence of any commercial or financial relationships that could be construed as a potential conflict of interest.
Publisher’s Note
All claims expressed in this article are solely those of the authors and do not necessarily represent those of their affiliated organizations, or those of the publisher, the editors and the reviewers. Any product that may be evaluated in this article, or claim that may be made by its manufacturer, is not guaranteed or endorsed by the publisher.
Supplementary Material
The Supplementary Material for this article can be found online at: https://www.frontiersin.org/articles/10.3389/fmars.2022.880103/full#supplementary-material
References
Balmaceda-Aguilera C., Cortés-Campos C., Cifuentes M., Peruzzo B., Mack L., Tapia J. C., et al. (2012). Glucose Transporter 1 and Monocarboxylate Transporters 1, 2, and 4 Localization Within the Glial Cells of Shark Blood-Brain-Barriers. PloS One 7 (2), e32409. doi: 10.1371/journal.pone.0032409
Barnes K. R., Cozzi R. R., Robertson G., Marshall W. S. (2014). Cold Acclimation of NaCl Secretion in a Eurythermic Teleost: Mitochondrial Function and Gill Remodeling. Comp. Biochem. Physiol. A Mol. Integr. Physiol. 168, 50–62. doi: 10.1016/j.cbpa.2013.11.004
Boeuf G., Payan P. (2001). How Should Salinity Influence Fish Growth? Comp. Biochem. Physiol. C Toxicol. Pharmacol. 130 (4), 411–423. doi: 10.1016/s1532-0456(01)00268-x
Chang C. H., Huang J. J., Yeh C. Y., Tang C. H., Hwang L. Y., Lee T. H. (2018). Salinity Effects on Strategies of Glycogen Utilization in Livers of Euryhaline Milkfish (Chanos Chanos) Under Hypothermal Stress. Front. Physiol. 9, 81. doi: 10.3389/fphys.2018.00081
Chang C. H., Liu Z. Z., Lee T. H. (2019). Changes in Hypothermal Stress-Induced Hepatic Mitochondrial Metabolic Patterns Between Fresh Water- and Seawater-Acclimated Milkfish, Chanos Chanos. Sci. Rep. 9 (1), 18502. doi: 10.1038/s41598-019-55055-4
Chang C. H., Wang Y. C., Lee T. H. (2021). Hypothermal Stress-Induced Salinity-Dependent Oxidative Stress and Apoptosis in the Livers of Euryhaline Milkfish, Chanos Chanos. Aquaculture 534, 736280. doi: 10.1016/j.aquaculture.2020.736280
Chang J. C., Wu S. M., Tseng Y. C., Lee Y. C., Baba O., Hwang P. P. (2007). Regulation of Glycogen Metabolism in Gills and Liver of the Euryhaline Tilapia (Oreochromis Mossambicus) During Acclimation to Seawater. J. Exp. Biol. 210 (Pt 19), 3494–3504. doi: 10.1242/jeb.007146
Chang C. H., Yang W. K., Lin C. H., Kang C. K., Tang C. H., Lee T. H. (2016). FXYD11 Mediated Modulation of Na+/K+-ATPase Activity in Gills of the Brackish Medaka (Oryzias Dancena) When Transferred to Hypoosmotic or Hyperosmotic Environments. Comp. Biochem. Physiol. A Mol. Integr. Physiol. 194, 19–26. doi: 10.1016/j.cbpa.2016.01.013
Chang C. H., Zhou X. W., Wang Y. C., Lee T. H. (2020). Differential Effects of Hypothermal Stress on Lactate Metabolism in Fresh Water- and Seawater-Acclimated Milkfish, Chanos Chanos. Comp. Biochem. Physiol. A Mol. Integr. Physiol. 248, 110744. doi: 10.1016/j.cbpa.2020.110744
Dahlke F. T., Wohlrab S., Butzin M., Pörtner H. O. (2020). Thermal Bottlenecks in the Life Cycle Define Climate Vulnerability of Fish. Science 369 (6499), 65–70. doi: 10.1126/science.aaz3658
Deane E. E., Woo N. Y. S. (2009). Modulation of Fish Growth Hormone Levels by Salinity, Temperature, Pollutants and Aquaculture Related Stress: A Review. Rev. Fish Biol. Fisheries 19 (1), 97–120. doi: 10.1007/s11160-008-9091-0
De Boeck G., Wood C. M., Iftikar F. I., Matey V., Scott G. R., Sloman K. A., et al. (2013). Interactions Between Hypoxia Tolerance and Food Deprivation in Amazonian Oscars, Astronotus Ocellatus. J. Exp. Biol. 216 (Pt 24), 4590–4600. doi: 10.1242/jeb.082891
Donaldson M. R., Cooke S. J., Patterson D. A., Macdonald J. S. (2008). Cold Shock and Fish. J. Fish Biol. 73 (7), 1491–1530. doi: 10.1111/j.1095-8649.2008.02061.x
Ern R., Huong D. T., Cong N. V., Bayley M., Wang T. (2014). Effect of Salinity on Oxygen Consumption in Fishes: A Review. J. Fish Biol. 84 (4), 1210–1220. doi: 10.1111/jfb.12330
Gagnon M. M., Holdway D. A. (1999). Metabolic Enzyme Activities in Fish Gills as Biomarkers of Exposure to Petroleum Hydrocarbons. Ecotoxicol Environ. Saf. 44 (1), 92–99. doi: 10.1006/eesa.1999.1804
Guo T., Yang Y., Meng F., Wang S., Xia S., Qian Y., et al. (2020). Effects of Low Salinity on Gill and Liver Glycogen Metabolism of Great Blue-Spotted Mudskippers (Boleophthalmus Pectinirostris). Comp. Biochem. Physiol. C Toxicol. Pharmacol. 230, 108709. doi: 10.1016/j.cbpc.2020.108709
Hardewig I., van Dijk P. L., Moyes C. D., Pörtner H. O. (1999). Temperature-Dependent Expression of Cytochrome-C Oxidase in Antarctic and Temperate Fish. Am. J. Physiol. 277 (2), R508–R516. doi: 10.1152/ajpregu.1999.277.2.R508
Hiroi J., McCormick S. D. (2012). New Insights Into Gill Ionocyte and Ion Transporter Function in Euryhaline and Diadromous Fish. Respir. Physiol. Neurobiol. 184 (3), 257–268. doi: 10.1016/j.resp.2012.07.019
Hu Y. C., Kang C. K., Tang C. H., Lee T. H. (2015). Transcriptomic Analysis of Metabolic Pathways in Milkfish That Respond to Salinity and Temperature Changes. PloS One 10 (8), e0134959. doi: 10.1371/journal.pone.0134959
Hwang P. P., Lee T. H., Lin L. Y. (2011). Ion Regulation in Fish Gills: Recent Progress in the Cellular and Molecular Mechanisms. Am. J. Physiol. Regul. Integr. Comp. Physiol. 301 (1), R28–R47. doi: 10.1152/ajpregu.00047.2011
Jana S. N., Garg S. K., Patra B. C. (2006). Effect of Inland Water Salinity on Growth Performance and Nutritional Physiology in Growing Milkfish, Chanos Chanos (Forsskal): Field and Laboratory Studies. J. Appl. Ichthyol 22 (1), 25–34. doi: 10.1111/j.1439-0426.2006.00698.x
Kang C.-K., Chen Y.-C., Chang C.-H., Tsai S.-C., Lee T.-H. (2015). Seawater-Acclimation Abates Cold Effects on Na+, K+-ATPase Activity in Gills of the Juvenile Milkfish, Chanos Chanos. Aquaculture 446, 67–73. doi: 10.1016/j.aquaculture.2015.04.022
Lefevre S. (2016). Are Global Warming and Ocean Acidification Conspiring Against Marine Ectotherms? A Meta-Analysis of the Respiratory Effects of Elevated Temperature, High CO2 and Their Interaction. Conserv Physiol. 4 (1), cow009. doi: 10.1093/conphys/cow009
Liao I. C. (1991). “Milkfish Culture in Taiwan,” in Handbook of Mariculture, Vol. II, Finfish Aquaculture. Ed. McVey J. P. (Boca Raton, FL: CRC Press), 91–115.
Lin Y. S., Tsai S. C., Lin H. C., Hsiao C. D., Wu S. M. (2011). Changes of Glycogen Metabolism in the Gills and Hepatic Tissue of Tilapia (Oreochromis Mossambicus) During Short-Term Cd Exposure. Comp. Biochem. Physiol. C Toxicol. Pharmacol. 154 (4), 296–304. doi: 10.1016/j.cbpc.2011.06.014
Li J., Xu X., Li W., Zhang X. (2019). Linking Energy Metabolism and Locomotor Variation to Osmoregulation in Chinese Shrimp Fenneropenaeus Chinensis. Comp. Biochem. Physiol. B Biochem. Mol. Biol. 234, 58–67. doi: 10.1016/j.cbpb.2019.05.006
Martínez-Quintana J. A., Peregrino-Uriarte A. B., Gollas-Galván T., Gómez-Jiménez S., Yepiz-Plascencia G. (2014). The Glucose Transporter 1 -GLUT1- From the White Shrimp Litopenaeus Vannamei Is Up-Regulated During Hypoxia. Mol. Biol. Rep. 41 (12), 7885–7898. doi: 10.1007/s11033-014-3682-8
Martinez F. S., Tseng M.-C., Yeh S.-P. (2006). Milkfish (Chanos Chanos) Culture: Situations and Trends. J. Fisheries Soc. Taiwan 33 (3), 229–244. doi: 10.29822/jfst.200609.0004
McClelland G. B., Craig P. M., Dhekney K., Dipardo S. (2006). Temperature- and Exercise-Induced Gene Expression and Metabolic Enzyme Changes in Skeletal Muscle of Adult Zebrafish (Danio Rerio). J. Physiol. 577 (Pt 2), 739–751. doi: 10.1113/jphysiol.2006.119032
Orczewska J. I., Hartleben G., O'Brien K. M. (2010). The Molecular Basis of Aerobic Metabolic Remodeling Differs Between Oxidative Muscle and Liver of Threespine Sticklebacks in Response to Cold Acclimation. Am. J. Physiol. Regul. Integr. Comp. Physiol. 299 (1), R352–R364. doi: 10.1152/ajpregu.00189.2010
Roa J. N., Tresguerres M. (2019). Differential Glycogen Utilization in Shark Acid- and Base-Regulatory Gill Cells. J. Exp. Biol. 222 (Pt 10). doi: 10.1242/jeb.199448
Soengas J., Sangiao-Alvarellos S., Laiz-Carrión R., Mancera J. (2007). “Energy Metabolism and Osmotic Acclimation in Teleost Fish,” in Fish Osmoregulation, 1st Edition. Eds. Baldisserotto B., Mancera J. M., Kapoor B. G. (Enfield, NH, USA: CRC Press), 277–307.
Speers-Roesch B., Callaghan N. I., MacCormack T. J., Lamarre S. G., Sykes A. V., Driedzic W. R. (2016). Enzymatic Capacities of Metabolic Fuel Use in Cuttlefish (Sepia Officinalis) and Responses to Food Deprivation: Insight Into the Metabolic Organization and Starvation Survival Strategy of Cephalopods. J. Comp. Physiol. B 186 (6), 711–725. doi: 10.1007/s00360-016-0991-3
Strobel A., Leo E., Pörtner H. O., Mark F. C. (2013). Elevated Temperature and PCO2 Shift Metabolic Pathways in Differentially Oxidative Tissues of Notothenia Rossii. Comp. Biochem. Physiol. B Biochem. Mol. Biol. 166 (1), 48–57. doi: 10.1016/j.cbpb.2013.06.006
Tresguerres M., Clifford A. M., Harter T. S., Roa J. N., Thies A. B., Yee D. P., et al. (2020). Evolutionary Links Between Intra- and Extracellular Acid-Base Regulation in Fish and Other Aquatic Animals. J. Exp. Zool A Ecol. Integr. Physiol. 333 (6), 449–465. doi: 10.1002/jez.2367
Tseng Y. C., Huang C. J., Chang J. C., Teng W. Y., Baba O., Fann M. J., et al. (2007). Glycogen Phosphorylase in Glycogen-Rich Cells Is Involved in the Energy Supply for Ion Regulation in Fish Gill Epithelia. Am. J. Physiol. Regul. Integr. Comp. Physiol. 293 (1), R482–R491. doi: 10.1152/ajpregu.00681.2006
Tseng Y. C., Hwang P. P. (2008). Some Insights Into Energy Metabolism for Osmoregulation in Fish. Comp. Biochem. Physiol. C Toxicol. Pharmacol. 148 (4), 419–429. doi: 10.1016/j.cbpc.2008.04.009
Tseng Y. C., Lee J., Chang J., Kuo C., Lee S.-J., Hwang P. P. (2008). Regulation of Lactate Dehydrogenase in Tilapia (Oreochromis Mossambicus) Gills During Acclimation to Salinity Challenge. Zool Stud. 47, 473–480.
Tseng Y. C., Lee J. R., Lee S. J., Hwang P. P. (2011). Functional Analysis of the Glucose Transporters-1α, -6, and -13.1 Expressed by Zebrafish Epithelial Cells. Am. J. Physiol. Regul. Integr. Comp. Physiol. 300 (2), R321–R329. doi: 10.1152/ajpregu.00144.2010
Webb D., Gagnon M. M., Rose T. (2005). Metabolic Enzyme Activities in Black Bream (Acanthopagrus Butcheri) From the Swan-Canning Estuary, Western Australia. Comp. Biochem. Physiol. C Toxicol. Pharmacol. 141 (4), 356–365. doi: 10.1016/j.cbpc.2005.07.010
Wu S. M., Liu J. H., Shu L. H., Chen C. H. (2015). Anti-Oxidative Responses of Zebrafish (Danio Rerio) Gill, Liver and Brain Tissues Upon Acute Cold Shock. Comp. Biochem. Physiol. A Mol. Integr. Physiol. 187, 202–213. doi: 10.1016/j.cbpa.2015.05.016
Yang S., Yan T., Wu H., Xiao Q., Fu H. M., Luo J., et al. (2017). Acute Hypoxic Stress: Effect on Blood Parameters, Antioxidant Enzymes, and Expression of HIF-1alpha and GLUT-1 Genes in Largemouth Bass (Micropterus Salmoides). Fish Shellfish Immunol. 67, 449–458. doi: 10.1016/j.fsi.2017.06.035
Yan J. J., Hwang P. P. (2019). Novel Discoveries in Acid-Base Regulation and Osmoregulation: A Review of Selected Hormonal Actions in Zebrafish and Medaka. Gen. Comp. Endocrinol. 277, 20–29. doi: 10.1016/j.ygcen.2019.03.007
Keywords: glycogen-rich cells, aerobic metabolism, lactate, GLUT1, milkfish, gills
Citation: Chang C-H, Liu C-J, Lu W-J, Wu L-Y, Lai K-J, Lin Y-T and Lee T-H (2022) Hypothermal Effects on Energy Supply for Ionocytes in Gills of Freshwater- and Seawater-Acclimated Milkfish, Chanos chanos. Front. Mar. Sci. 9:880103. doi: 10.3389/fmars.2022.880103
Received: 21 February 2022; Accepted: 28 March 2022;
Published: 21 April 2022.
Edited by:
Xiaodan Wang, East China Normal University, ChinaReviewed by:
Yung-Che Tseng, Academia Sinica, TaiwanHon Jung Liew, Universiti Malaysia Terengganu, Malaysia
Copyright © 2022 Chang, Liu, Lu, Wu, Lai, Lin and Lee. This is an open-access article distributed under the terms of the Creative Commons Attribution License (CC BY). The use, distribution or reproduction in other forums is permitted, provided the original author(s) and the copyright owner(s) are credited and that the original publication in this journal is cited, in accordance with accepted academic practice. No use, distribution or reproduction is permitted which does not comply with these terms.
*Correspondence: Tsung-Han Lee, dGhsZWVAZW1haWwubmNodS5lZHUudHc=
†These authors have contributed equally to this work