- 1Department of Ecology and Coastal Management, Institute of Marine Sciences of Andalusia (ICMAN), Spanish National Research Council (CSIC), Puerto Real, Spain
- 2Department of Analytical Chemistry, Faculty of Marine and Environmental Sciences, Marine Research Institute (INMAR), University of Cadiz, Cadiz, Spain
Monitoring water systems under extreme conditions or in remote areas poses a risk to scientists and staff involved, and can also result in disturbances to the local flora and fauna. In order to overcome these limitations, new techniques are being developed with the aim to gain more insight into how geochemical pathways of trace elements regulate the functioning of the ocean. Here, we present the first trace metals and inorganic nutrients concentrations measured in dissolved (<0.22 µm) surface water samples collected in the Antarctic by using an Automatic Water Autosampler (AWA) system onboard an unmanned aerial vehicle (UAV). The AWA system has been used to chemically quantify the composition of the water masses (lake and coastal water) of Deception Island (South Shetland Islands, Antarctica), with, for example, Pb values ranging from 0.01 to 0.25 nM in seawater and 1.20 to 2.11 nM in lake water. The results demonstrate the effectiveness of the AWA system in environmental studies that require the sampling of trace metals and nutrients without sample contamination.
Introduction
Marine environmental monitoring requires sampling of surface waters for subsequent chemical analysis. Traditional water sampling methods involve manually collecting samples from the shore, or using grab sampling techniques (Noble et al., 2020). In coastal and offshore waters, however, there are numerous sampling devices and techniques that can be operated from vessels, including peristaltic pumps, CTD rosettes, and tow fish (e.g. Tovar-Sánchez, 2012). The use of these techniques is conditioned by environmental/climatological conditions, costs and logistics, and are more complicated to use, especially in protected, inaccessible or extreme environments, such as in the polar regions.
In marine geochemistry studies, sampling of trace metals (TMs) in seawater involves numerous challenges. Although some TMs form part of the Earth’s crust and are considered essential for marine organisms, their concentrations in ocean waters are extremely low, since low solubility and exposure to complex physical and chemical processes (i.e. organic complexation, sinking, scavenging or remineralization) (e.g. Lohan & Tagliabue, 2018) result in short residence times in surface water. Moreover, because of their low concentrations in seawater and high concentrations in sampling tools and devices, contamination of samples has historically been the main obstacle for accurate quantification of TMs (e.g. Grand et al., 2019). Therefore, the adoption of clean techniques and instrumentation is essential for the quantification of TMs in marine environments.
In the 1970s, the use of clean techniques resulted in the first accurate measurements of TMs, and a consequent increase in knowledge of the biogeochemistry of TMs in the ocean (Lam & Anderson, 2018). Nowadays, in order to avoid contamination several different standardized protocols exist for sampling, storage and analysis (e.g. USEPA, 1996; Cutter et al., 2017). Despite the huge progress that has been achieved in the knowledge of the distribution of TMs in the oceans, sampling methods make marine biogeochemical analysis a very laborious and time-consuming process. As a consequence, to overcome these limitations and gain a better understanding of the functioning of the ocean, marine research requires further development of new techniques and innovative tools.
Throughout the past decade, advances in unmanned aerial vehicles (UAVs, or drones) technology, a new era began in environmental monitoring, representing an innovative and continuously evolving tool (e.g. Anderson & Gaston, 2013; Ventura et al., 2016; Anweiler & Piwowarski, 2017; Konar & Iken, 2018; Tovar-Sánchez et al., 2021). The application of UAVs is suitable for a multidisciplinary studies approach, since they can be applied to a wide range of fields, using different sensors and devices (Park & Choi, 2020, and the references therein). Several new technologies of water sampling tools mounted on UAVs have been designed and successfully applied in different conditions; for example, wetland ecosystems (Schwarzbach et al., 2014), pit lakes (Castendyk et al., 2020) and freshwater (Koparan et al., 2020). Remote water samplers have been used for the determination of a wide range of chemical and physical parameters; for example, temperature, pH, alkalinity, dissolved oxygen, major ions, such as sulfate, chloride, calcium and manganese (Detweiler et al., 2015; Ore & Elbaun, 2015; Castendyk et al., 2020). However, to the best of our knowledge none of these devices have been designed exclusively for the determination of dissolved trace elements in marine environments.
Here, we present the Automatic Water Autosampler (AWA) system (Figure 1 and Supplementary Video SV-1), which, onboard a UAV, allows the collection of up to 2L of dissolved (<0.22 µm) surface water samples. The AWA system has been successfully applied for sampling surface water for dissolved trace metals (Ag, Cd, Co, Cu, Fe, Ni, Mo, Pb, V and Zn) and nutrients quantification in the harsh environment of the Antarctic continent (South Shetland Islands, Figure 2). We characterized the chemical composition of different water masses of Deception Island: Crater Lake (an Antarctic Specially Protected Area, ASPA 140) and the coastal waters of the Vapour Col (eastern part) and Baily Head (southwestern part) penguin colonies.
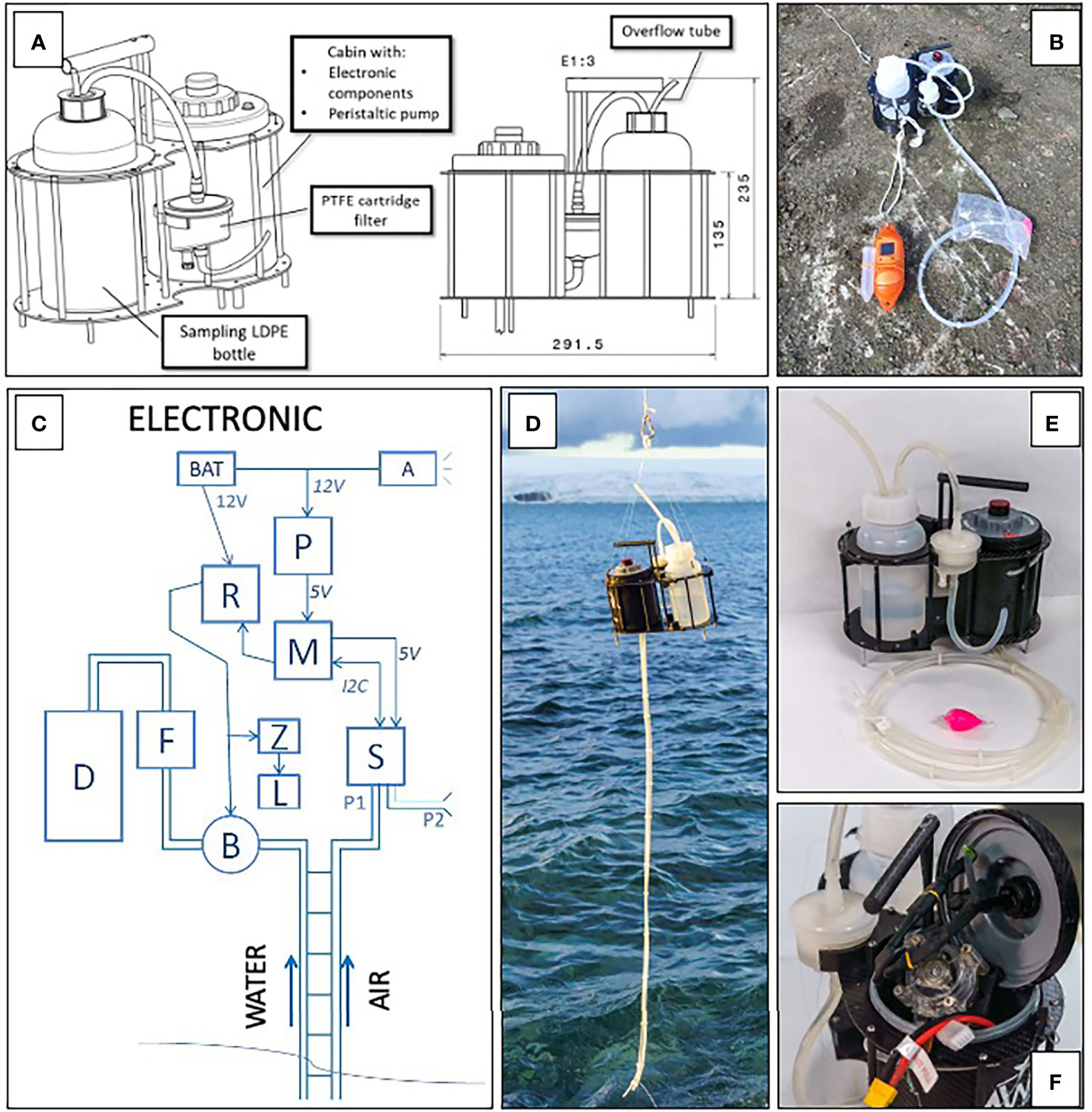
Figure 1 The AWA system: (A) schematic representation of the device components (Dronetools©) with sizes expressed in mm; (B, D, E) pictures of the whole system including, AWA and multiparameter probe [CastAway-CTD®, orange instrument: (B)]; (F) picture of components inside the cabin; (C) system components scheme (BAT, lipo battery; A, low battery alarm; P, DC-DC 5V power supply; R, relay 12 V; M, microcontroller; S, pressure sensor (P1, tube air pressure; P2, tube atmosphere pressure); Z, active pump buzzer; L, active pump flash light; B, peristaltic pump; F, PTFE Cartridge filter; D, sampling LDPE bottle; I2C, sensor communication protocol).
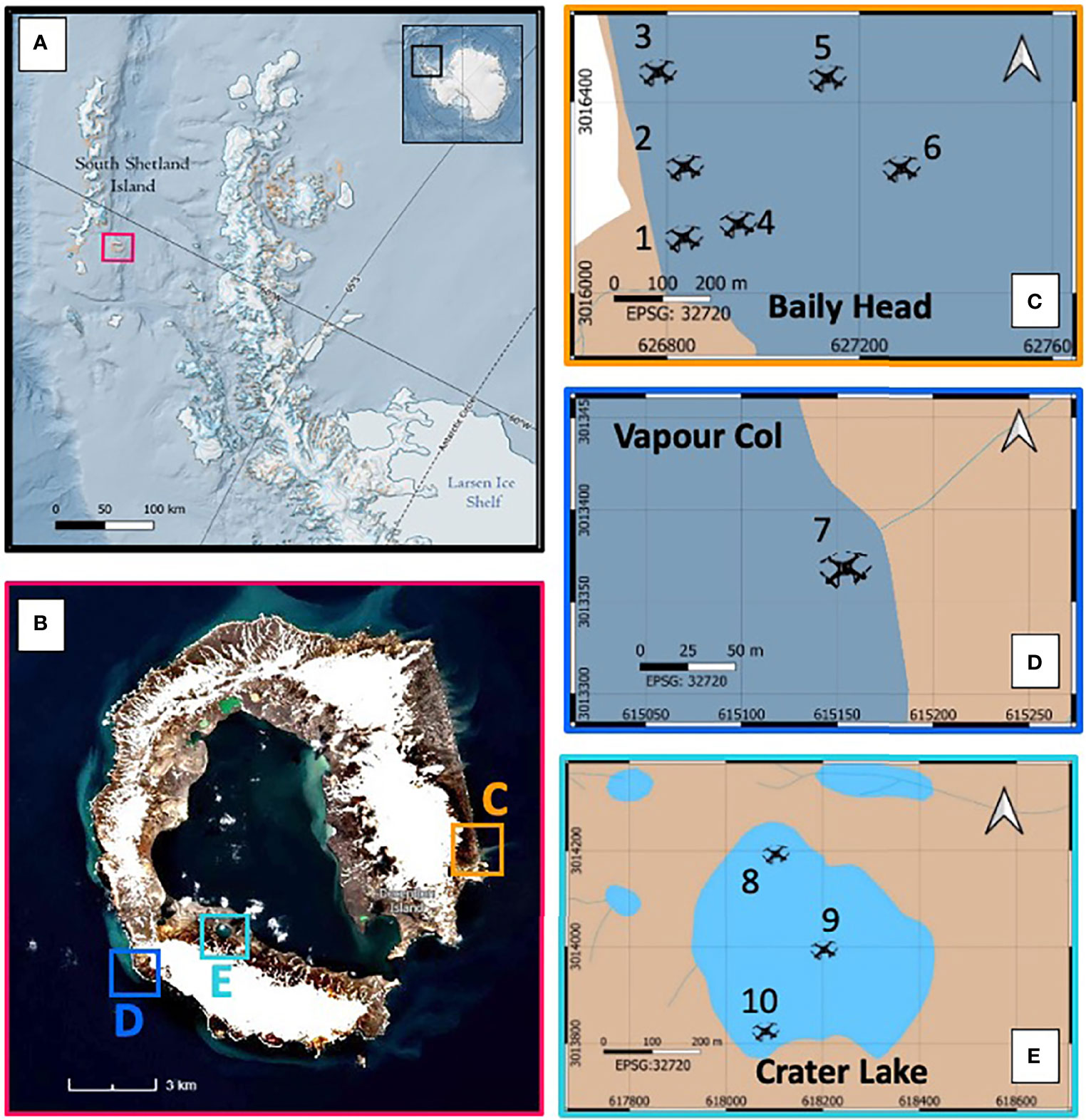
Figure 2 (A, B) Location of Deception Island in the South Shetland Islands. Sampling stations at: (C) Baily Head rockery, (D) Vapour Col and (E) Crater Lake.
Materials and Methods
The AWA System
The Automatic Water Autosampler device (Figure 1A), fulfils the requirements of the standards for TM analysis mentioned previously. It is composed of a hermetic carbon fiber cabin housing an electronic microcontroller and a relay for the control of the peristatic pump of three rollers (GOSO Technology, GB37-530), that works with a rechargeable LiPo battery, (12 V) and is equipped with a acid-washed silicone sampling tube (8mm external diameter and 5 mm internal diameter) connected to a PTFE cartridge filter (0.22 µm; Sartobran® P-300, Sartorius 5231307H5—OO-B, with 0.22 µm pore size and 300 cm2 filtration area) (Figure 1). A pressure sensor connected to another silicone tube activates the peristaltic pump when the tube is submerged. The system works at a constant flow rate of 150 mL/min and can collect up to 2 L of water using an acid cleaned LDPE bottle. The system has an overflow tube located in the cap of the bottle that allow discharge water once the bottle is full, allowing to condition the bottle and homogenize the sample (Figure 1A). The device includes visual (flashing red light) and audible (buzzer) elements to indicate that the pump is active and the device is sampling elements to indicate that the pump is active and the device is sampling. The system, with a size of 29.2 x 23.5 cm and an empty weight of approx. 300 g is attached to the UAV with a 25-metre rope (Supplementary Video SV-1), and connected with a release system activated by the pilot to collect the AWA device before landing the UAV. AWA hangs from a UAV at a minimum distance of 25 meters to ensure that: 1) UAV does not touch the surface water due any contingency such as fail during altitude operation by the pilot, waves, gusts of winds; 2) have the possibility to maneuver in the event of a possible hitch of the system and 3); avoid disturbance produced by the UAVs propellers on the surface water during sampling.
The AWA system uses the conventional validated TM sampling system based in the use of a peristaltic pump (e.g., USEPA, 1996; Cutter et al., 2017) but operated remotely from a UAV. The system was validated for TM analysis where the concentrations of samples collected with the AWA system were in accordance with the results of samples obtained with a conventional peristaltic pump handily operated from land (Tovar-Sánchez et al., 2021).
The UAV Platform
The UAV used in this study was a hexacopter with three-bladed propellers (Condor, Dronetools©), with a DJI6010 electric motor for each of the six blades, powered by four Li-ion batteries (7,000 mA each). The maximum takeoff weight (MTOW) was 14.9 kg, with maximum flight autonomy of up to 60 minutes (without payload). The UAV was equipped with a camera with an 30x optical zoom (DJI Zemuse Z30) to allow visualization of sample collection. The flight is programed with the DJI GO 4 software, which registers the coordinates out of the sampling location. Previous waypoints can also be set before sampling.
Study Site
Deception Island is one of the most active volcanos in Antarctica, belonging to the South Shetland Islands in the Bransfield Strait, Antarctic Peninsula (Figure 2). The volcano has a large submerged caldera 8-10 km in diameter, forming the natural harbor Port Foster (Cooper et al., 1998). Eight species of birds breed on the island, with the Chinstrap penguin (Pygoscelis antarcticus) representing the most abundant seabird species, with an estimated population of almost 200,000 breeding pairs (Deception Island Management Package, 2005). In this study, three sampling areas were selected: i) a coastal area of the largest chinstrap rookery at Baily Head, a rocky promontory on the southeastern coast of the island (Deception Island Management Package, 2005; Naveen et al., 2012) (Figure 2C), comprising approximately 100,000 breeding pairs; ii) a coastal area of the second largest Chinstrap colony, with ca. 12,000 breeding pairs, in Vapour Col, on the external west coast of the island (Naveen et al., 2012) (Figure 2D); and iii) Crater Lake, located northwest of Mount Kirkwood on the south side of the island. Crater Lake is part of the Antarctic Specially Protected Area (ASPA 140, Subsite B) due to its unique environmental characteristics (Figure 2E). Superficial water samples were collected from a total of 10 sample stations from the three different sites: i) six coastal stations from the Baily Head penguin colony ii) one station from the Vapour Col penguin colony and, iii) three stations on Crater Lake (Figure 2).
Chemical Analysis and Physical-Chemical Surveys
The filtered water samples (<0.22 µm) for the analysis of dissolved metals (Ag, Cd, Co, Cu, Fe, Ni, Mo, Pb, V and Zn) and nutrients were stored directly in 500 mL acid-washed low density polyethylene bottles. Once in the lab of the Spanish Research Base, samples for TM analysis were acidified to pH<2 with ultrapure-grade HCl in a class-100 HEPA laminar-flow hood and stored in dark conditions prior to analysis in the lab at Spain. For the analysis of nutrients, samples were stored in 13 ml PE tubes and maintained frozen until the analysis. For seawater TM quantification, samples were pre-concentrated using a liquid-organic extraction method (Bruland et al., 1979) and analyzed using inductively coupled plasma-mass spectrometry (ICP-MS, iCAP Thermo). For dissolved fresh water (lake), TMs (same as coastal water plus Mn) were measured directly by ICP-MS. The accuracy of the pre-concentration method and analysis for TMs was determined using Seawater Reference Material for Trace Elements (CASS 6, NRC-CNRC). The recoveries reported were: 74%, 97%, 84%, 92%, 91%, 89%, 93%, 121% and 92% for V, Fe, Co, Ni, Cu, Zn, Mo, Cd and Pb, respectively (Table 1). Concentrations of dissolved nutrients were determined with an autoanalyzer (Skalar San ++ System) using colorimetric techniques (Grasshoff, 1996). The accuracy of the nutrient analysis was checked using reference material for seawater (KANSO CRM Japan, Lot. No. CP 2020.3.31), with recoveries of 124%, 108%, 102% and 99% for , respectively. The Detection Limits (DL), based on 3 standard deviations of the lab blank, were: 0.11 nM, 0.13 nM, 8.20 nM, 0.03 nM, 0.03 nM, 0.05 nM, 1.64 nM, 0.05 nM, 0.04 nM, 0.01 nM and 0.01 nM for V, Mn, Fe, Co, Ni, Cu, Zn, Mo, Ag, Cd and Pb, respectively; and 0.4 µM, 0.4 µM, 0.32 µM, 2 µM and 2 µM for , respectively. Conductivity, temperature, salinity and depth and GPS position were measured in-situ using a hydrographic instrument (YSI CastAway-CTD®) attached to the UAV.

Table 1 Certified and measured concentrations of Standard Reference material for trace metals (CASS-6, NRC-CNRC) in µg/L and nutrients (KANSO, CRM Japan) in µM, in seawater.
Results and Discussion
This case study, using the AWA system water sampler mounted on an UAV, represents the first successful sampling survey of the outer coasts of Deception Island and of Crater Lake. The TM compositions of water samples are summarized in Table 2. The seawater surrounding the two penguin colonies were sampled with a maximum depth of 0.8 m, within a range of temperature of 1.8 – 2.3°C and salinity average of 33.5 ± 0.2 PSS (Table 4). The coastal water surrounding the two penguin colonies showed similar concentrations for Mo, Ni, Cd, and Pb (Table 2). Silver was below detection limits for both colonies. However, the Vapour Col coastal water showed higher concentrations for V (40.10 nM), Fe (5803.86 nM), Co (2.40 nM), Cu (8.56 nM) and Zn (14.93 nM) than the Baily Head seawater (Table 2). Unfortunately, only one station at Vapour Col was possible to sample and comparisons between the coastal water colonies is biased. The TM contents in coastal seawater are in agreement with the values found in a previous study (e.g. Croot et al., 2011; Baars et al., 2014). Sañudo-Wilhelmy et al. (2002) measured TMs dissolved surface seawater from the Antarctic Peninsula to the Weddell Sea in the same order of magnitud than our work, with the exception of Fe (with an average concentration of 12.95 ± 0.01 nM) considerably lower than the values found in this study. Grotti et al. (2001) presented concentrations of Cu (4.1 ± 3.3 nM) in superficial waters of the Ross Sea of the same order as our results; however, the average concentration of Fe was much lower (1.9 ± 1.2 nM). A previous study found that penguin excretion could be an important source of Fe to the surface coastal water (Sparaventi et al., 2021). The nutrient compositions of water samples are presented in Table 3. and total phosphorous (TP) concentrations were similar for the two penguin colonies, with values below detection limit for in Vapour Col was 18.1 ± 1.3 µM and Baily Head ranged from 21.5 to 32.5 µM, and values were 1.2 ± 0.1M at Vapour Col and 1.9 – 2.6 µM in Baily Head. However, concentrations were higher in Vapour Col (639.0 ± 4.7 µM) than in Baily Head (range: 24.8 ± 4.8 to 130.6 ± 8.6 µM). Silicate values were lower in Vapour Col than Baily Head (Table 3). Values of found in Port Foster (in the center of the island) in a previous study by Aracena et al. (2018), were 5.34 and 16.69 µM, showing lower concentrations than those found in the present study. Therefore, similar to TMs, penguin colonies are influencing the nutrient concentrations of coastal seawaters. However, other factors such as depth of each station or presence of animals at the moment of the sampling could also be influence the concentrations of the analyzed parameters.
Crater lake surficial (0 – 1 m depth) waters had an average temperature of 4 ± 0°C (Table 4). The average concentrations in nM for V, Fe, Co, Zn, Mo, Pb and Mn were 6185.5 ± 744.6, 281.1 ± 399.5, 7.9 ± 0.5, 196.3 ± 273.1, 73.1 ± 6.1, 1.5 ± 0.5 and 19.9 ± 30.9, respectively (Table 2). Concentrations of Ni, Ag and Cd were below detection limits, while Cu was detected only in one Crater Lake station with a concentration of 12.41 nM. Only one previous study reported TMs (i.e., Fe and Mn) in Crater Lake with Mn concentrations of 99.4 nM and Fe undetectable (Elderfield, 1972). This Mn concentration is of the same order of magnitude as our first station (55.61 nM), but of an order of magnitude higher than our other two stations (2.27 and 1.85 nM).
The and concentrations in the Crater Lake samples were below detection limits (Table 3); the level was detected only in one station with a concentration of 2.5 ± 0.7 µM. The phosphate level found ranged from 1.0 to 2.0 µM, the SiO2 concentration ranged from 5.7 to 303.3 µM and TP ranged from 1.9 to 2.2 µM. These results are in agreement with other nutrient values found in a lake on the James Ross Archipelago, Antarctic Peninsula (Lecomte et al., 2016) and Terra Nova Bay (Zelano et al., 2017); and whose concentrations are most likely linked to the scarce biological activity in inland water (Zelano et al., 2017).
The Automatic Water Autosampler onboard UAVs contributes towards improving the information about chemical composition of the water masses of Deception Island. The system represents a less invasive methodology, by avoiding disturbance to the local wildlife (penguins or seals) and ensures safer sampling in inaccessible and high-risk locations, such as coastal regions, by avoiding the risks highlighted in the Deception Island Management Package, (Deception Island Management Package, 2005); for example, the risk of landings due to the powerful wave motion resulting from the heavily sloping beach, the presence of submerged rocks and the glacier front. Crater Lake represents another environment that is difficult to sample by boat, due to the presence of strong slopes. Additionally, the bottom of the northern valley is characterized by the presence of the largest vegetation area on the island, composed of an extensive carpet of moss (Sanionia uncinata) of particular interest, and classified as protected environment with restricted access (Antarctic Specially Protected Area, ASPA 140, Antarctic Treaty Secretariat, 2017).
The AWA system helps overcome the main limitations indicated by Lally et al. (2019) in the recent review of UAV-mounted water sampler systems, which are: i) discrepancies between chemical water parameters collected by drones and traditional methods; data from Tovar-Sánchez et al., 2021 demonstrate that the chemical parameters resulting from our system are consistent with samples collected by traditional methods; therefore, the measurements are not biased. ii) They collect small volumes of water (≤ 500 mL); the AWA system allows collection of up to 2 liters of samples, since it can accommodate bottles of different volumes; furthermore, the limitation of the volume of water is not on the sampler system but rather the load capacity of the UAV. iii) An unequal rate of water collection due to operational accidents or environmental conditions; by using the AWA system mounted on an UAV, we were able to collect samples at the planned stations operated from land without the use of any auxiliary boat or platform.
This aerial water sampling technique could be used to provide a long-term series of physico-chemical parameters, with the aim of improving information about the biogeochemical cycles of TMs and nutrients in a harsh environment, such as the Antarctic continent. The use of this technology, which is faster and safer than traditional sampling methods, requires less staff, less infrastructure, resulting in lower costs, would improve research in this ecosystem.
Conclusions
Our results show that the AWA system mounted on an UAV is an effective sampling strategy for the determination of dissolved elements in coastal environments or other water systems, especially in harsh environments with difficult access. This technology can contribute towards advancing progress in the monitoring of ocean biogeochemistry. UAVs provide a spectrum of useful information at multi-disciplinary level, including biological, geological and chemical data within a short time scale. Moreover, they ensure that field operations are less invasive to the environment and the local flora and fauna and, in addition, increase safety of the staff, since UAVs can be operated from land.
Data Availability Statement
The original contributions presented in the study are included in the article/Supplementary Material. Further inquiries can be directed to the corresponding author.
Author Contributions
ES contributed to the conceptualization and visualization of the manuscript. AT-S and GN carried out the UAV flights, collecting the surface water samples. ES and AR-R analyzed the collected surface water samples. All authors contributed to the article and approved the submitted version.
Funding
This research has been funded by the Spanish Government projects PIMETAN (ref. RTI2018-098048-B-I00), EQC2018-004275-P and EQC2019-005721-P. ES is supported by the Spanish FPI grant (Ref: PRE2019-089679) and AR-R is supported by the Spanish grant, Juan de la Cierva Incorporación (Ref: IJC2018-037545-I).
Conflict of Interest
The authors declare that the research was conducted in the absence of any commercial or financial relationships that could be construed as a potential conflict of interest.
Publisher’s Note
All claims expressed in this article are solely those of the authors and do not necessarily represent those of their affiliated organizations, or those of the publisher, the editors and the reviewers. Any product that may be evaluated in this article, or claim that may be made by its manufacturer, is not guaranteed or endorsed by the publisher.
Acknowledgments
We thank Dronetools company for the developing of the AWA system and to D. Roque for supporting flight operations. We thank I. Carribero and M.C. Agulló for their support with the instrumental and methodological analyses and M. B. Dunbar for her English language edits. This research is part of the POLARCSIC research initiative. Permissions to work and collect guano samples in the study area were granted by the Spanish Polar Committee. We thank the hospitality of the Spanish Antarctic Stations “Gabriel de Castilla” and the transportation by the B/O Sarmiento de Gamboa. We also thank the logistic support by the Marine Technological Unit of CSIC.
Supplementary Material
The Supplementary Material for this article can be found online at: https://www.frontiersin.org/articles/10.3389/fmars.2022.879953/full#supplementary-material
Supplementary Video 1 | Automatic Water Autosampler operating from UAV in the Antarctic Environment.
References
Anderson K., Gaston K. J. (2013). Lightweight Unmanned Aerial Vehicles Will Revolutionize Spatial Ecology. Front. Ecol. Environ. 11 (3), 138–146. doi: 10.1890/120150
Antarctic Treaty Secretariat (2017). Management Plan for Antarctic Specially Protected Area No 140 Parts of Deception Island, South Shetland Islands 6, 140.
Anweiler S., Piwowarski D. (2017). Multicopter Platform Prototype for Environmental Monitoring. J. Clean. Prod. 155, 204–211. doi: 10.1016/j.jclepro.2016.10.132
Aracena C., González H. E., Vargas J. G., Lange C. B., Pantoja S., Muñoz F., et al. (2018). Influence of Summer Conditions on Surface Water Properties and Phytoplankton Productivity in Embayments of the South Shetland Islands. Pol. Biol. 41 (10), 2135–2155. doi: 10.1007/s00300-018-2338-x
Baars O., Abouchami W., Galer S. J. G., Boye M., Croot P. L. (2014). Dissolved Cadmium in the Southern Ocean: Distribution, Speciation, and Relation to Phosphate. Limnol. Oceanog. 59, 385–399. doi: 10.4319/lo.2014.59.2.0385
Bruland K. W., Franks R. P., Knauer G. A., Martin J. H. (1979). Sampling and Analytical Methods for the Determination of Copper, Cadmium, Zinc, and Nickel at the Nanogram Per Liter Level in Sea Water. Analytic. Chim. Acta 105 (C), 233–245. doi: 10.1016/S0003-2670(01)83754-5
Castendyk D., Voorhis J., Kucera B. (2020). A Validated Method for Pit Lake Water Sampling Using Aerial Drones and Sampling Devices. Min. Water Environ. 39 (3), 440–454. doi: 10.1007/s10230-020-00673-y
Cooper A. P. R., Smellie J. L., Maylin J. (1998). Evidence for Shallowing and Uplift From Bathymetric Records of Deception Island, Antarctica. Antarctic. Sci. 10 (4), 455–461. doi: 10.1017/s0954102098000558
Croot P. L., Baars O., Streu P. (2011). The Distribution of Dissolved Zinc in the Atlantic Sector of the Southern Ocean. Deep Sea Research Part Ii. Top. Stud. Oceanog. 58, 2707–2719. doi: 10.1016/j.dsr2.2010.10.041
Cutter G. A., Casciotti K., Croot P., Geibert W., Heimbürger L.-E., Maeve L., et al. (2017). Sampling and Sample-handling Protocols for GEOTRACES Cruises, Version 3.0. (Toulouse, France, GEOTRACES International Project Office) 139pp. doi: 10.25607/OBP-2
Deception Island Management Package (2005). Available at: http://www.deceptionisland.aq/package.php.
Detweiler C., Ore J. P., Anthony D., Elbaum S., Burgin A., Lorenz A. (2015). Environmental Reviews and Case Studies: Bringing Unmanned Aerial Systems Closer to the Environment. Environ. Pract. 17 (3), 188–200. doi: 10.1017/S1466046615000174
Elderfield H. (1972). Effects of Volcanism on Water Chemistry, Deception Island Antarctica. Mar. Geolog. 13 (c), M1–M6. doi: 10.1016/0025-3227(72)90066-7
Grand M. M., Laes-Huon A., Fietz S., Resing J. A., Obata H., Luther G. W., et al. (2019). Developing Autonomous Observing Systems for Micronutrient Trace Metals. Front. Mar. Sci. 6 (FEB). doi: 10.3389/fmars.2019.00035
Grasshoff K. (1996). Methods of Seawater AnalysisComprehensive Analytical Chemistry Vol. 30, 3–4. doi: 10.1016/S0166-526X(96)80004-3
Grotti M., Soggia F., Abelmoschi M. L., Rivaro P., Magi E., Frache R. (2001). Temporal Distribution of Trace Metals in Antarctic Coastal Waters. Mar. Chem. 76 (3), 189–209. doi: 10.1016/S0304-4203(01)00063-9
Konar B., Iken K. (2018). The Use of Unmanned Aerial Vehicle Imagery in Intertidal Monitoring. Deep-Sea Research Part Ii. Top. Stud. Oceanog. 147, 79–86. doi: 10.1016/j.dsr2.2017.04.010
Koparan C., Koc A. B., Sawyer C., Privette C. (2020). Temperature Profiling of Waterbodies With a Uav-Integrated Sensor Subsystem. Drones 4 (3), 1–10. doi: 10.3390/drones4030035
Lally H. T., O’Connor I., Jensen O. P., Graham C. T. (2019). Can Drones be Used to Conduct Water Sampling in Aquatic Environments? A Review. Sci. Total. Environ. 670, 569–575. doi: 10.1016/j.scitotenv.2019.03.252
Lam P. J., Anderson R. F. (2018). Geotraces: The Marine Biogeochemical Cycle of Trace Elements and Their Isotopes. Elements 14 (6), 377–378. doi: 10.2138/gselements.14.6.377
Lecomte K. L., Vignoni P. A., Córdoba F. E., Chaparro M. A. E., Chaparro M. A. E., Kopalová K., et al. (2016). Hydrological Systems From the Antarctic Peninsula Under Climate Change: James Ross Archipelago as Study Case. Environ. Earth Sci. 75 (7), 623. doi: 10.1007/s12665-016-5406-y
Lohan M. C., Tagliabue A. (2018). Oceanic Micronutrients: Trace Metals That are Essential for Marine Life. Elements 14 (6), 385–390. doi: 10.2138/gselements.14.6.385
Naveen R., Lynch H. J., Forrest S., Mueller T., Polito M. (2012). First Direct, Site-Wide Penguin Survey at Deception Island, Antarctica, Suggests Significant Declines in Breeding Chinstrap Penguins. Pol. Biol. 35 (12), 1879–1888. doi: 10.1007/s00300-012-1230-3
Noble A. E., Tuit C. B., Maney J. P., Wait A. D. (2020). A Review of Marine Water Sampling Methods for Trace Metals. Environ. Forensic. 21 (3–4), 267–290. doi: 10.1080/15275922.2020.1771629
Ore J., Elbaun S. (2015). Autonomous Aerial Water Sampling. Wiley. Periodic. Inc. 32 (8), 1095–1113. doi: 10.1002/rob.21591
Park S., Choi Y. (2020). Applications of Unmanned Aerial Vehicles in Mining From Exploration to Reclamation: A Review. Minerals 10 (8), 1–32. doi: 10.3390/min10080663
Sañudo-Wilhelmy S. A., Olsen K. A., Scelfo J. M., Foster T. D., Flegal A. R. (2002). Trace Metal Distributions Off the Antarctic Peninsula in the Weddell Sea. Mar. Chem. 77 (2–3), 157–170. doi: 10.1016/S0304-4203(01)00084-6
Schwarzbach M., Laiacker M., Mulero-Pazmany M., Kondak K. (2014). “Remote Water Sampling Using Flying Robots,” In International Conference on Unmanned Aircraft Systems, ICUAS 2014 - Conference Proceedings. (Orlando, FL, USA) 72–76. doi: 10.1109/ICUAS.2014.6842240
Sparaventi E., Rodríguez-Romero A., Barbosa A., Ramajo L., Tovar-Sánchez A. (2021). Trace Elements in Antarctic Penguins and the Potential Role of Guano as Source of Recycled Metals in the Southern Ocean. Chemosphere 285, 131423. doi: 10.1016/j.chemosphere.2021.131423
Tovar-Sánchez A. (2012). Sampling Approaches for Trace Element Determination in Seawater. Compr. Sampl. Sampl. Prep. 1, 317–334. doi: 10.1016/B978-0-12-381373-2.00017-X
Tovar-Sánchez A., Román A., Roque-Atienza D., Navarro G. (2021). Applications of Unmanned Aerial Vehicles in Antarctic Environmental Research. Sci. Rep. 11 (1), 1–8. doi: 10.1038/S41598-021-01228-Z
USEPA (1996). Method 1669: Sampling Ambient Water for Trace Metals at EPA Water Quality Criteria Levels. Environ. Prot., 37.
Ventura D., Bruno M., Jona Lasinio G., Belluscio A., Ardizzone G. (2016). A Low-Cost Drone Based Application for Identifying and Mapping of Coastal Fish Nursery Grounds. Estua. Coast. Shelf. Sci. 171, 85–98. doi: 10.1016/j.ecss.2016.01.030
Keywords: remote water sampling, Antarctic waters, dissolved trace element, unmanned aerial vehicle - UAV, biogeochemical cycle
Citation: Sparaventi E, Rodríguez-Romero A, Navarro G and Tovar-Sánchez A (2022) A Novel Automatic Water Autosampler Operated From UAVs for Determining Dissolved Trace Elements. Front. Mar. Sci. 9:879953. doi: 10.3389/fmars.2022.879953
Received: 20 February 2022; Accepted: 12 April 2022;
Published: 10 May 2022.
Edited by:
Christian Joshua Sanders, Southern Cross University, AustraliaReviewed by:
Peter Leslie Croot, National University of Ireland Galway, IrelandRobert Józef Bialik, Institute of Biochemistry and Biophysics (PAN), Poland
Copyright © 2022 Sparaventi, Rodríguez-Romero, Navarro and Tovar-Sánchez. This is an open-access article distributed under the terms of the Creative Commons Attribution License (CC BY). The use, distribution or reproduction in other forums is permitted, provided the original author(s) and the copyright owner(s) are credited and that the original publication in this journal is cited, in accordance with accepted academic practice. No use, distribution or reproduction is permitted which does not comply with these terms.
*Correspondence: Erica Sparaventi, ZXJpY2Euc3BhcmF2ZW50aUBjc2ljLmVz