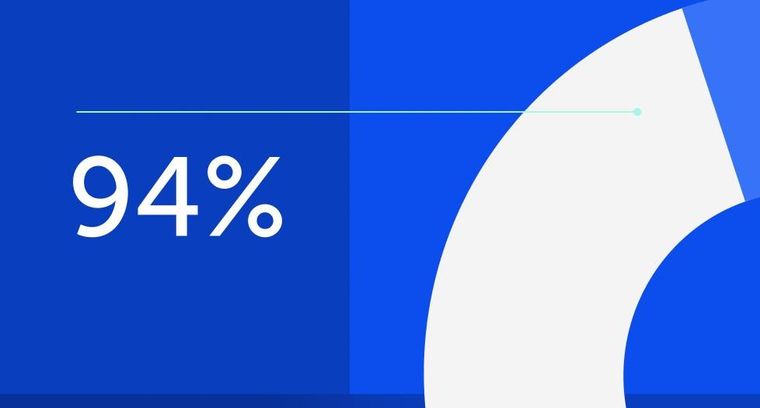
94% of researchers rate our articles as excellent or good
Learn more about the work of our research integrity team to safeguard the quality of each article we publish.
Find out more
ORIGINAL RESEARCH article
Front. Mar. Sci., 16 June 2022
Sec. Marine Ecosystem Ecology
Volume 9 - 2022 | https://doi.org/10.3389/fmars.2022.877904
This article is part of the Research TopicThe Physiology, Ecology and Biogeochemistry of Marine Zooplankton in a Changing Arctic OceanView all 15 articles
In the Arctic shelf seas, the mesozooplankton biomass is dominated by the arctic copepod Calanus glacialis, but its boreal congeneric C. finmarchicus is expanding northwards. Even though it is already there, C. finmarchicus may not be able to truly establish itself in the Arctic seas and potentially replace C. glacialis. We compared metabolic and digestive enzyme activities of sympatric C. glacialis and C. finmarchicus from Isfjorden, Svalbard and off-shelf north of Svalbard. The seasonal regulation of anabolic and catabolic enzyme activities was generally similar for the two species, but with some interspecific differences corresponding to their ontogeny. Wake-up from overwintering started earlier in adults of C. glacialis than in C. finmarchicus, while the onset of dormancy started early in the overwintering stages of both species. Furthermore, C. glacialis showed an earlier and higher mobilization of lipase enzyme activities, indicating higher efficiency in assimilating dietary lipids compared to C. finmarchicus. Similar population sizes and population structures for C. finmarchicus off-shelf north of Svalbard and in Isfjorden support a similar origin. Still, C. finmarchicus was able to match regulation of enzyme activities to the bloom even though the bloom peaked approximately a month later off-shelf north of Svalbard, indicating that food availability is an important signal for the final step of termination of diapause. Even though the two species largely follow the same patterns of metabolic enzyme activities, the more efficient lipid anabolism of C. glacialis may give it an advantage over C. finmarchicus in high-Arctic unpredictable environments with short-pulsed primary production regimes.
Calanoid copepods of the genus Calanus dominate the mesozooplankton community in Arctic and sub-Arctic seas in terms of biomass (>60%) (Jaschnov, 1970; Blachowiak-Samolyk et al., 2008; Kosobokova et al., 2011) and constitute a key link between primary producers and higher trophic levels (Falk-Petersen et al., 2007). In large calanoid copepods, lipids may comprise up to 70% of their dry weight (Sargent and Falk-Petersen, 1988; Lee et al., 2006), which they acquire mainly during short periods of primary production lasting from two to eight weeks at high latitudes (reviewed by Leu et al., 2015). The conversion of their food to energy-rich lipids is key to the rapid and efficient transfer of energy and essential fatty acids from primary producers to higher trophic levels in arctic marine ecosystems (Falk-Petersen et al., 1990; Dahl et al., 2003). For the copepods, the accumulated lipid stores are necessary to fuel molting, gonad maturation and egg production in the following season (Falk-Petersen et al., 2009; Banas et al., 2016). In the most extreme environment – the deep Arctic Ocean – these copepods must be capable of surviving up to 10-11 months without food. The lack of sun during the 4-month polar night as well as the ice cover may efficiently block the incoming solar light into the summer. They also utilize the stored lipids for their basic metabolism during the extended periods without food through the arctic autumn and winter (Falk-Petersen et al., 2009). To preserve the lipids in this period, Calanus spp. perform seasonal vertical migrations, descending to depth and reducing their metabolism to a minimum when winter approaches, a physiological state named diapause or dormancy (Hirche, 1996; Freese et al., 2017).
Three species of the genus Calanus co-occur in the European Arctic: the two arctic species Calanus hyperboreus and C. glacialis, and the North Atlantic C. finmarchicus (Conover, 1988; Choquet et al., 2017), of which the two latter are the focus in this study. Calanus glacialis is considered an arctic species, though isolated populations have been identified along the Norwegian coast (Choquet et al., 2017). It has a 1–2-year life cycle and exhibits a mixed breeding strategy, meaning that it can mature and produce eggs at low rates, likely fueled by internal resources (capital breeding) early in the season, and then egg production rates increase when food becomes available with the bloom (income breeding, Hirche and Kattner, 1993). The boreal C. finmarchicus, which is smaller than C. glacialis, has a 1-year life cycle at its northernmost distribution and is considered to be an income breeder (Plourde and Runge, 1993; Kjellerup et al., 2012). Their difference in life cycle is also reflected in their overwintering stages. Calanus glacialis overwinters from CIV on and C. finmarchicus overwinters primarily as CV (Falk-Petersen et al., 2009).
Diapause is controlled endogenously and is a physiological reaction to reoccurring adverse environmental conditions such as low food availability during winter (Dahms, 1995). Diapause is commonly performed by specific ontogenetic stages in crustaceans (Guppy and Withers, 1999), such as the overwintering stages in Calanus spp. (see above). Five phases of diapause have been described in copepods: 1) the preparatory phase when the organisms accumulate lipids (energy reserves) and arrest further development and growth, 2) the induction phase when the organisms stop feeding and reduce their metabolism, 3) the refractory phase when the organisms are torpid and reach minimum metabolic activity, 4) the activation phase when organisms regain their ability to develop, and, finally 5) the termination phase when the organisms reach full metabolic activity again (Hirche, 1996). Depending on environment, latitude and season, dormancy strategies and ontogenetic migrations may vary profoundly among species and even within populations of the same species (Hirche, 1996; Hirche, 1998; Darnis and Fortier, 2014).
In the Svalbard-Barents Sea region, C. finmarchicus and C. glacialis are regarded as important beacons of climate change (Wassmann et al., 2015). A higher proportion of C. finmarchicus compared to C. glacialis reflects a strong influence of Atlantic water and a relatively warm arctic climate while the opposite indicates a colder arctic climate. A substantial borealization of the arctic zooplankton community is predicted with global warming (Hays et al., 2005). In the surrounding path of the North Atlantic Current into the Fram Strait and the Barents Sea, an increase in abundances of the boreal C. finmarchicus and other North Atlantic species has already been recorded (Wassmann et al., 2006; Aarflot et al., 2017; Hop et al., 2019a; Hop et al., 2019b). To better predict the consequences of the rapid warming for these key species in the Arctic, better knowledge on physiological adaptations of zooplankton species is needed. Baseline information on the natural seasonal variability in metabolism is important in order to identify physiological responses directly linked to the ongoing climate change (e.g., Møller et al., 2016).
Seasonal changes in metabolic activity can be determined by measuring the relative activity level of enzymes of central metabolic pathways (e.g., Freese et al., 2017). For instance, citrate synthase (CS) and malate dehydrogenase (MDH), which catalyze different reactions in the citric acid cycle, are regarded as good indicators of the aerobic potential of an organism and thus its overall metabolism (Torres and Somero, 1988; Meyer et al., 2002; Teschke et al., 2007). MDH is strongly correlated with respiration (Meyer et al., 2010) and the advantage of measuring MDH rather than respiration by incubations is the minimum laboratory handling effect (Ohman et al., 1998). CS on the other hand has also been found to correlate with egg production (Kreibich et al., 2008). Catabolism of stored lipids, which occurs mainly in January-March for C. glacialis (Freese et al., 2017), is positively correlated to activity of 3-hydroxyacyl-CoA dehydrogenase (HOAD), a key enzyme of the β-oxidation of fatty acids (Auerswald and Gäde, 1999; Hassett, 2006). Further, the HOAD activity is inversely related to digestive enzyme activities (Freese et al., 2016; Freese et al., 2017). The major components of the copepods´ diet are proteins and lipids, which are processed by proteinase and lipase/esterase, respectively. Peak digestive enzyme activities in C. glacialis are found when algal food is plentiful and then gradually decrease throughout the autumn-winter (Freese et al., 2016). Overall, the metabolism of C. glacialis is reduced to half during winter, which suggests that this species is not going into a proper diapause as defined by Hirche (1996) (Freese et al., 2017). However, respiration rates of both species in winter have been found to be reduced by a similar amount [40–65%; C. finmarchicus Hirche (1983); C. glacialis: Morata and Søreide (2015)] and do not suggest large inter-species differences in the overwintering metabolic states. How Calanus spp. regulate their physiology to best schedule their complex life history to the strong seasonality at high latitudes is still poorly understood.
In this study, we were particularly interested in the timing of physiological changes in co-occurring C. glacialis and C. finmarchicus, and how such changes are related to life history strategies and the environmental conditions. Possible inter-species differences may provide new insights into why C. glacialis is specially adapted to the Arctic while C. finmarchicus thrives under more temperate North Atlantic conditions.
We performed monthly sampling of C. glacialis and C. finmarchicus over a 15-month period in Isfjorden, a high-Arctic (78°N) fjord in Svalbard with relatively high water temperatures (>1°C) and no seasonal ice. Samples were collected to assess general metabolism (CS and MDH), digestion of incoming food (proteinase and lipase) and utilization of stored lipid resources (HOAD). For comparison, we also collected samples off-shelf north of Svalbard. For comparing the timing of changes in co-occurring C. glacialis and C. finmarchicus, our major research questions were (1) Do the metabolic and catabolic enzyme activities follow the same seasonal patterns in the two Calanus species? (2) Do the seasonal patterns in digestive enzyme activity differ between the species? And (3) How do enzyme activity patterns compare between off-shelf and fjord individuals?
Calanus glacialis and C. finmarchicus were collected monthly from June 2015 to August 2016 at Karlskronadjupet (78°19’N; 015°10’E) (Figure 1), a 274 m deep basin in the central part of the Isfjorden system, Svalbard. The mouth of Isfjorden is open towards the shelf and slope area along west Spitsbergen, with no sill limiting the inflow of Atlantic water via the West Spitsbergen Current (WSC) and of Arctic water via the East Spitsbergen Current. Both these currents are flowing from south to north along the shelf break and shelf respectively (Nilsen et al., 2008).
Figure 1 Study area with main station in Isfjorden, Svalbard marked with star. Off-shelf sampling locations marked with dots (N-ICE campaign in white, other campaigns in grey with white circles). [Map data: (GADM, 2015; NPI, 2015; GEBCO, 2020)].
Samples were also collected off-shelf north of Svalbard (Figure 1 and Supplementary Table S1). The main sampling period off-shelf north of Svalbard was from February to June during the N-ICE 2015 campaign when RV Lance was frozen into the ice (Granskog et al., 2018), and additional off-shelf sampling north of Svalbard was conducted with RV Helmer Hanssen in late summer 2015 and 2016.
Sampling in Isfjorden was conducted from larger vessels (RV Helmer Hanssen, KV Svalbard, RV Dalnie Zelentsy) and smaller boats (RV Viking Explorer and UNIS Polaris). Data on water column properties were collected using a SAIV SD204 CTD or an SBE Seabird Electronics CTD; and identification of water masses was based on Skogseth et al. (2020) for the fjord station, and on Meyer et al. (2017) and Rudels et al. (2000) for off-shelf north of Svalbard. Data from fluorometers attached to the CTDs were used to estimate the average Chlorophyll a (Chl a) concentration corresponding to the depth intervals where zooplankton was sampled (see below). The fluorescence sensor data were verified by comparison with Chl a measured fluorometrically. Water samples were collected at several depths and filtered on GF/F filters. The samples were extracted in methanol for 24 h at 4°C before Chl a concentration was measured with an AU10 Turner Fluorometer (Turner Design, Inc.).
Stratified zooplankton samples were collected with a MultiNet Midi (0.250 m2 opening, mesh size 200 µm, Hydro-Bios) or a WP2 closing net (0.255 m2 opening, mesh size 200 µm, Hydro-Bios). Copepods for enzyme analyses were collected from those layers in which the majority of the population resided. On 15 January 2016, off-shelf, and 4 April 2016, in Isfjorden, samples were collected both from surface and depth (Table S1). Sorting was conducted either immediately after sampling on board or within 12 h after sampling at return to the University Centre in Svalbard (UNIS). Previous studies on calanoid copepods have showed that it takes at least a week for changes to occur in digestive (Landry, 1983; Head and Conover, 1983) and metabolic (Freese, 2015) enzyme activities when conditions are altered, thus the effect of the up to 12-hour delay should be negligible. In either case, sorting was conducted in a temperature-controlled room at 2-4°C to avoid temperature stress on the copepods. The dominating stages of C. glacialis and C. finmarchicus were sorted in triplicates à 10 and 15 individuals for enzyme activity analyses and single individuals of the same stages were collected in tin caps to determine dry mass (Supplementary Table S1). Individuals were quickly rinsed in distilled water, blotted briefly by touching them to a tissue paper and transferred to cryo vials before being snap frozen in liquid nitrogen. Samples were stored at -80°C and transported to the Alfred Wegener Institute, Bremerhaven, Germany, either in a dry shipper or on dry ice for further analyses.
The different species of Calanus were initially distinguished, while alive, by their prosome length according to Daase and Eiane (2007) with adjustments made for CV and adult females after Gabrielsen et al. (2012), as presented in Daase et al. (2018). From copepodite stage CIV and older, C. hyperboreus was also distinguished by the presence of an acute spine on the fifth thoracic segment. Pigmentation was used as an additional indicator of alive specimens since C. glacialis, from these regions, tend to have more red pigmentation on the antennules and genital segment of the urosome (females only) compared to C. finmarchicus (Nielsen et al., 2014; Choquet et al., 2018; Trudnowska et al., 2020).
Enzyme activities in both C. glacialis and C. finmarchicus were measured at the Alfred Wegener Institute in Bremerhaven, Germany. Measurements were conducted in triplicates for two digestive enzymes (proteinase and lipase/esterase), and for three metabolic enzymes [Malate dehydrogenase (MDH), Citrate synthase (CS), and 3-Hydroxyacyl-CoA dehydrogenase (HOAD)]. Proteinase, lipase/esterase and CS activities were all measured in the same extract of 10 individuals, from the sorted triplicates à 10 individuals (section 2.1). Individuals for MDH and HOAD measurements were collected from each of the triplicates à 15 individuals. Two of these were pooled and extracted for MDH activity analyzes, three of these were pooled and extracted for HOAD activity, and the remaining 10 in each vial were kept as back up.
Measurements of the Malate dehydrogenase activity (EC 1.1.1.37) were modified after Teschke et al. (2007) as described in Freese et al. (2017). The frozen copepod samples were transferred to 1.5 ml Eppendorf tubes in triplicates of two individuals each and homogenized by hand with a micropestle in 40 µl 0.1 M potassium phosphate buffer at pH 7.0. The homogenates were centrifuged for 15 min at 15,000 g at 4°C and the liquid phase was transferred to a new 1.5 ml Eppendorf tube. The sample was diluted 1:10 by adding 6 µl of the sample to a vial with 54 µl of buffer. Measurements of enzyme activity were done in a 96 well plate. Buffer (180 µl), 6.7 µl NADH (Roche Diagnostics 10107735001) and 6.7 µl sample were mixed and incubated at 25°C for 5 min. The reaction was started by adding 6.7 µl oxaloacetic acid and the absorbance was measured for 5 min at 25°C and 340 nm in a Synergy HTX Multi-Mode Reader using the software KC4 3.4 Rev.21. The slope of the curve from 1-4 min was used to calculate the enzyme activity.
Citrate synthase activity (EC 4.1.3.7.) was measured after Stitt (1984) and Saborowski et al. (2002), but with a different buffer system. Enzymes were extracted from the frozen copepods as described below (see “Digestive Enzymes”). In a semi-microcuvette, 20 µL 5,5’-dithiobis-(2-nitrobenzoic acid) (DTNB, Sigma-Aldrich, D8130), 20 µL Acetyl-CoA (Acetyl-Coenzyme A trilithium salt, Roche Diagnostics, 13893324), 20 µL sample extract, or buffer for controls, and 520 µL 0.1 M Tris/HCl (supplemented with 10 mM CaCl2) buffer at pH 7.0 were mixed. The mixture was pre-incubated in the spectrophotometer (Thermo Scientific, UV1), kept at 25°C using a Peltier element (Krüss Optronic), for 5 min before 20 µL oxaloacetic acid (Sigma-Aldrich, O4126) was added to start the reaction. The absorbance was measured regularly in the spectrophotometer for 3 min at 405 nm, and the measurements were recorded with the software VisionLite (version 2.2).
Measurements of 3-Hydroxyacyl-CoA dehydrogenase activity (EC 1.1.1.35) were modified after Auerswald and Gäde (1999) as described in Freese et al. (2017). The frozen copepod samples were transferred to 1.5 ml micro centrifuge tubes in triplicates á three individuals and homogenized by hand with a micropestle in 180 µl 107 mM triethanolamine/HCl buffer (supplemented with 5.3 mM EDTA) at pH 7.0. The homogenates were centrifuged at 15 000 g at 4°C for 15 min and the liquid phase was transferred to a new 1.5 ml Eppendorf tube. Measurements of enzyme activity were done in a 96 well plate. 180 µl buffer, 6.7 µl NADH (see above) and 6.7 µl sample were mixed and incubated at 25°C for 5 min. The reaction was started by adding 6.7 µl Acetoacetyl-CoA (Sigma A-1625) and the absorbance was measured for 8 min at 25°C and 340 nm in a Synergy HTX Multi-Mode Reader using the software KC4 3.4 Rev.21. The slope of the curve from 3-7 min was used to calculate the enzyme activity. Unfortunately, we experienced technical difficulties when measuring HOAD in C. glacialis from winter and thus were not able to obtain consistent HOAD measurements throughout the year.
The frozen copepod samples were transferred to 1.5 ml Eppendorf tubes in triplicates á 10 individuals and homogenized by hand with a micropestle in 200 µl 0.1 M Tris/HCl (supplemented with 10 mM CaCl2) buffer at pH 7.0. The homogenates were centrifuged at 15 000 g at 4°C for 15 min. The liquid phase was transferred to a new 1.5 ml Eppendorf tube and the remaining tissue was stored at -80°C for later molecular verification of species. This extract was used for measurements of proteinase, lipase/esterase and CS.
Total proteinase activity (EC 3.4.21-24) was measured after Saborowski et al. (2004), modified after Kreibich et al. (2008). Sample (20 µL), or buffer for controls, was pipetted into 1.5 ml Eppendorf tubes and pre-incubated at 30°C for 5 min on a thermo shaker at 400 rpm. To each vial, five µl azocasein (1% in deionized water, Fluka BioChemika, 11615) was added before incubation for another 60 min. The reaction was stopped by adding 50 µl trichloroacetic acid (TCA, 8%) and the vials were centrifuged at 15 000 g at 4°C for 15 min. The supernatant was transferred to an ultra-microcuvette (Hellma 105.203-QS). The optical density of the supernatant was measured with a spectrophotometer (Thermo Scientific, UV1) at 366 nm (dE366) and recorded with the software VisionLite (version 2.2).
Lipase/esterase activity (EC 3.1.1.) was measured after Knotz et al. (2006). Sample (20 µl), or buffer for controls, was diluted in 470 µL 0.1 M Tris/HCl (supplemented with 10 mM CaCl2) buffer at pH 7.0. Ten µl 4-methylumbelliferyl butyrate dissolved in dimethyl sulfoxide (5 mmol L-1, MUF-butyrate, Fluka BioChemica, 19362; DMSO, AppliChem A3608) were added to each vial and incubated in the dark at 25°C for 30 min on a thermo shaker at 400 rpm. Fluorescence was measured with a NanoDrop 3300 at 360 nm (excitation) and 450 nm (emission) and recorded with the software ND-3300 V 2.7.0.
Because of the uncertainty of differentiating between C. glacialis and C. finmarchicus based on morphology (Choquet et al., 2018), the remaining tissue pellets after the first batch of enzyme extraction were kept and stored at -80°C for molecular species identification. The pellets were transported in a dry shipper to Nord University in Bodø (Norway) where molecular analyses were conducted.
The species composition of the tissue pellets, i.e., from pooled individuals, was genetically assessed by analysis of species-specific profiles of length polymorphism in six nuclear molecular markers characterized by motifs of insertion or deletion (i.e., InDels) (Smolina et al., 2014), following Choquet et al. (2017). In short, DNA was extracted from the remaining pellets of Calanus tissue, following the HotSHOT DNA extraction method (Montero-Pau et al., 2008). Six InDel markers were amplified in a multiplexed Polymerase Chain Reaction (PCR). The resulting amplified markers were sized on a 3500xL Genetic Analyzer (Applied Biosystems), generating either a “pure” species-specific profile characteristic of Calanus finmarchicus or C. glacialis (see Smolina et al., 2014) when only one species was present within a pool, or a profile containing mixed signals from both species when two species were present within a pool.
At each sampling, 24 individuals of both C. glacialis and C. finmarchicus were collected for dry mass (DM) and Carbon and Nitrogen (CN) analyses. The animals were rinsed quickly in distilled water before being placed individually in pre-weighed tin capsules (IVA analysentechnik, SA76981102) and dried at 60°C for 24 h. DM was determined by weighing the samples in the tin capsules and subtracting the pre-weight. After weighing, the tin capsules were packed tight and the samples were analyzed for CN content with an element analyzer (vario EL cube, Elementar).
Weighted mean depth (WMD) was calculated for the total population of C. glacialis and C. finmarchicus following equation 1:
Where ai is the number of individuals per m3 of species a in depth stratum i, di is the sampled distance in depth stratum i, D is average depth in depth stratum i and n is the number of depth strata at a station.
Statistical analyses were done in Sigmaplot (14.0, Systat Software, San Jose, CA). T-tests were applied to investigate potential differences in enzyme activities among species, stages and dates. Correlations between changes in enzyme activities and physical (temperature, light) and biological (Chl a, Weighted Mean Depth (WMD) of the community) factors, as well as among enzyme activities, were done with Pearson correlations when linear relationships and normally distributed data occurred, and Spearman’s rank-order correlation when not. The significance level was set to α=0.05 in all tests.
The fluorescence sensor data, as verified with Chl a data measured fluorometrically and described in detail by Eide (2016) for Isfjorden and Assmy et al. (2017) for off-shelf area north of Svalbard, captured well the seasonality in Chl a concentrations.
In Isfjorden, the spring bloom had commenced (1.5 µg Chl a L-1) at the start of the sampling campaign in June 2015. Relatively high Chl a concentrations persisted throughout July and August (2.2 and 2.4 µg L-1, respectively; Figure 2). From October 2015 to April 2016, typically low winter Chl a values (< 0.01 µg Chl a L-1) were found. The first increase was recorded in early May (2.1 µg Chl a L-1), and peak Chl a concentrations were found between mid-May and early June 2016 (>7.0 µg Chl a L-1). In July 2016, Chl a concentrations were found to be in the same range as in the previous summer (2.1 µg Chl a L-1). In late August, the Chl a concentration dropped to 0.73 µg Chl a L-1 (Figure 2). Off-shelf north of Svalbard, low winter algal biomasses (< 0.01 µg Chl a L-1) prevailed until mid-May in 2015 during the N-ICE drift campaign (Figure 2). Peak phytoplankton bloom concentrations (8 µg Chl a L-1) were recorded in early June and remained relatively high (4.7-6.3 µg L-1) until late June when the last measurement was done (2.4 µg L-1, Figure 2). North of Svalbard in September 2015 and August 2016, the Chl a concentrations were elevated in the upper 20 m with respective 1.4 µg Chl a L-1 (15 m) and 1.3 µg Chl a L-1 (14 m). In January 2016, no fluorescence was detectable (Figure 2).
Figure 2 Enzyme activities for Calanus finmarchicus (red) and C. glacialis (blue). Data from off-shelf are shown in the left panels and data from Isfjorden are shown in the right panels. The background presents data from the Chl a max ranging from <0.01 (lightest green) to 8 µg Chl a L-1 (darkest green). White background with diagonal lines means no data on Chl a max. Specific activities are indicated for (A) Malate dehydrogenase, (B) Citrate synthase, (C) 3-hydroxyacyl-CoA dehydrogenase (HOAD), (D) proteinase, and (E) lipase. Each point represents one measurement, and the line connects the averaged levels of activity. For dates with sampling both in the surface and at depth, the surface samples are highlighted with grey fill.
In Isfjorden during the sampling period, the water column was mostly dominated by transformed Atlantic water. However, stratification was observed during both summers with warmer (> 4°C) and less saline (< 33) Surface Water in the upper ~25 m compared to the waters below. There was also an influx of Atlantic water in December 2015, observed as an increase in both temperature and salinity at around 100 m depth (Figure 3).
Figure 3 Seasonal changes in water temperature (Top, °C) and salinity (bottom) Off-shelf during the N-ICE 15 campaign in 2015 (left) and in Isfjorden from June 2015 to August 2016 (right).
Off-shelf north of Svalbard during the drift with RV Lance in the N-ICE campaign, we passed over the Yermak Plateau, an area influenced by the north-flowing West Spitsbergen Current (Meyer et al., 2017). In the consolidated pack ice north of Svalbard, the hydrography was characterized by a cold (<0°C) relatively fresh (<34.5) and deep mixed surface layer of Polar Surface Water down to 100 m, and warmer (>2°C) and more saline (>34.5) Atlantic Water and Modified Atlantic Water were located between 100-500 m (Meyer et al., 2017). Hydrographic conditions changed significantly after 25 May, when the Atlantic Water was found closer to the surface and the mixed surface layer became thinner, fresher, and warmer (Figure 3).
After extraction of enzymes from the samples á 10 individuals (section 2.3.2), the remaining tissue pellets provided sufficient material for molecular identification (section 2.4). 65% of these tissue pellets were sequenced, with resulting success rate of morphological identification at 97% for Isfjorden and at 85% for the off-shelf stations. The enzyme measurements from the six extracts of mixed samples were discarded. In total, 49 extracts were not tested because of a shipping issue; 29 from Isfjorden and 20 from off-shelf. Applying the same success rate of identification as the tested samples indicate that among the untested extracts, one from Isfjorden and three from off-shelf may be mixed. Based on the high success rate of the tested samples and the fact that each sample is made up of several individuals that will mitigate the impact of the occasional misidentified individual, these samples and two extracts with inconclusive molecular ID were treated as correctly identified in further analyses. The samples of mixed ID, inconclusive and not tested are listed in Table S2.
The MDH and CS activities followed the same seasonal trend for both species in Isfjorden, with high levels in spring and summer and low levels in autumn and winter (Figure 2). For both enzymes, the specific activity (activity mg DM-1) was low prior to the bloom and increased rapidly for a short period, peaking in mid-June (Figure 2).
The timing of peak MDH activity and the CS activity levels differed between the two species. For MDH, the specific activities in the two species were in the same range. However, peak MDH activities were reached one month earlier in C. glacialis than in C. finmarchicus (Figure 2A). Maximum MDH levels were recorded in early May (23.3 U mg DM-1) in C. glacialis and June (24.4 U mg DM-1) in C. finmarchicus (Figure 2A). For both species, minimum MDH activity levels were recorded in January (3.7-4.9 U mg DM-1). For CS, Calanus finmarchicus had higher activity (2.6 U mg DM-1) in spring-summer than C. glacialis (1.6 U mg DM-1), while the CS activity levels decreased to similarly low levels (~1 U mg DM-1) during autumn and winter for both species (Figure 2B). The CS and MDH activities in Isfjorden were strongly positively correlated to day length (as well as to Chl a) in C. finmarchicus, but not in C. glacialis (Table 1).
Table 1 Correlation between the enzyme activity and the environmental factors Chlorophyll a max in the water column (Chl a), Weighted mean depth of community (WMD), temperature at weighted mean depth (WMD temp.) and Day length.
Peak HOAD activity occurred in March in both C. glacialis (1.98 U mg DM-1) and C. finmarchicus (1.63 U mg DM-1). Minimum activities were measured in late August in C. glacialis (0.02 U mg DM-1) and in mid-September in C. finmarchicus (0.08 U mg DM-1) (Figure 2C). In C. finmarchicus, HOAD was negatively correlated (Pearson, r = -0.57, p<0.05) with Chl a, whereas no significant correlation was found for C. glacialis (Pearson, r = -0.04, Table 1).
Strong positive correlations between Chl a concentration and digestive enzyme activities were found for both species (Table 1 and Figures 2D, E). In Isfjorden, the proteinase activity of C. finmarchicus varied between a minimum of 1.59 dE366 h-1 mg DM-1 in October and maximum of 15.36 dE366 h-1 mg DM-1 in early May (Figure 2D). In C. glacialis in Isfjorden, the highest proteinase activity was also observed in early May (8.47 dE366 h-1 mg DM-1) and minimum proteinase activity was measured in early November with 0.2 dE366 h-1 mg DM-1 (Figure 2D).
The lipase activity of C. finmarchicus was at its minimum in February (28.94 nmol h-1 mg DM-1) and its maximum in May (280.51 nmol h-1 mg DM-1). For C. glacialis, the minimum lipase activity was found in March (33.96 nmol h-1 mg DM-1) and maximum in May, as for C. finmarchicus, but with three times higher maximum lipase activities (927.22 nmol h-1 mg DM-1) than recorded for C. finmarchicus (t-test, p<0.001) (Figure 2E).
Off-shelf, a positive correlation, similarly as in Isfjorden, between the Chl a maximum and the activity of both proteinase and lipase was observed for C. finmarchicus (Table 1). Off-shelf proteinase activity of C. finmarchicus was at a minimum in early March (1.0 dE366 h-1mg DM-1) and increased to peak activity on May 31st (10.21 dE366 h-1 mg DM-1). The lipase activity in off-shelf C. finmarchicus had a similar pattern as proteinase activities, with a minimum value measured in early March (189.65 dE366 h-1 mg DM-1) and peak activities in mid-June (1458.98 dE366 h-1 mg DM-1) (Figure 2). Calanus glacialis was only sampled during the pre-bloom, and at that time proteinase and lipase activities remained stable at 1.2-1.6 dE366 h-1 mg DM-1 and 109.3-136.8 nmol h-1 mg DM-1, respectively (Figure 2).
Both C. glacialis and C. finmarchicus followed similar and clear seasonal patterns of metabolic enzyme activities, with high activity in spring and summer and low activity during autumn and winter. In both species, MDH, a valid proxy for respiration and thus overall metabolism (Meyer et al., 2010), correlated well with day length, suggesting that light is an important factor regulating the seasonal pattern of the metabolic activity.
The seasonal pattern of metabolic enzyme activities also corresponded with the ontogenetic migration patterns of Calanus spp. (Figure S1; Hatlebakk et al., this issue). In Isfjorden, from which we have the longest (15 months) and most consistent data set, we found a negative correlation between MDH and WMD for both C. glacialis and C. finmarchicus supporting the assumption that Calanus spp. reduce their overall metabolism as they descend to depth (Hirche, 1996). Following the five phases of diapause described by Hirche (1996), we can assign the preparatory and induction phases of diapause to start in July-August corresponding to their seasonal descent. The refractory phase, i.e., when the lowest anabolic enzyme activities were recorded, occurred between September and January, followed by the activation phase in January-April, dependent on species, ontogenetic stage and presumably also body size (see below). The final termination phase was shorter and took place in April-May. In Isfjorden, minimum MDH activities, found in winter for both C. glacialis and C. finmarchicus, were 80% lower compared to specimen with maximum activity. In a previous study on C. glacialis in Billefjorden, Svalbard, Freese et al. (2017) showed a similar seasonal pattern, but their winter minimum activities were only 60% lower than summer maximum activities. Billefjorden is a colder, seasonally ice-covered branch of Isfjorden, and it is possible that differences in environmental conditions may have led to the differences in relative minimum enzyme activities while the general activity patten was similar. It is, however, also possible that we missed the deepest state of dormancy since there was a gap in sampling between early November and mid-January (Figure 2B), and C. glacialis may reach the refractory phase only for a brief period (Varpe and Ejsmond, 2018). Also, the variability in MDH was high among the samples, and this may relate to differences in individual fitness (Hassett, 2006). In order to clarify whether environmental or physiological factors can explain differences in minimum activities in winter, sampling with a higher resolution would be necessary.
We still have a poor understanding of which external and internal cues trigger and regulate the metabolism of Calanus, and particularly the onset and termination of diapause. However, recent studies on the seasonal variability in ion concentration (Freese et al., 2015), enzymes (e.g., Freese et al., 2016; Freese et al., 2017) and gene expressions (Häfker et al., 2018), together with life history modelling (Banas et al., 2016; Ejsmond et al., 2018; Varpe and Ejsmond, 2018) have provided new insights into diapause regulation. While most studies assume that deep-dwelling copepods enter a dormant state (i.e., diapause), there does not seem to be a simple on-off switch for diapause (e.g., Häfker et al., 2018). Specimens are gradually lowering and increasing their metabolic rates throughout the year (Freese et al., 2017), which has been well described by Hirche (1996) when identifying the five different phases of diapause in copepods. Diapause may be endogenously regulated (Häfker et al., 2018), or a gradual response to ceased feeding as suggested for C. glacialis by Freese et al. (2017), although it is most likely a complex combination of several factors.
There was an increase in abundance of adults over a period of 2-3 months for both species, but they appeared roughly two months earlier in C. glacialis than in C. finmarchicus, which confirms that C. glacialis reproduces earlier than C. finmarchicus, (Hatlebakk et al., this issue). This was further supported by an earlier peak of abundance in both C. glacialis eggs and young copepodite stages (CI-CIII, Hatlebakk et al., 2022). Gonad maturation is even more energy demanding than egg production (Jónasdóttir, 1999). In accordance, particularly high CS activities was found in C. glacialis males in January likely suggesting sperm formation. At that time, the male:female sex ratio was relatively high (0.4) and 9.2% of the females carried spermatophores (Daase et al., 2018), supporting that January was the time of male gonad maturation and mating.
The increase in metabolic enzyme activity would be expected to be coupled with increased HOAD activity, indicating utilization of stored lipid resources since external food is scarce in January. Accordingly, Freese et al. (2017) found the highest HOAD activity during mid-winter and decreasing activities when ice algae became present from mid-March on, providing food early in the season (Freese et al., 2016). We also found some indication of higher HOAD activity in CV C. glacialis in winter as compared to summer, but unfortunately, we experienced technical difficulties when measuring HOAD activities. In the period of low food availability, we were only able to obtain measurements of HOAD in October and one measurement in February and, thus, we are lacking data from mid-winter. However, HOAD activity was higher at these times compared to measurements for the spring and summer, and thus it is fair to assume the HOAD activity was increased in mid-winter.
In C. finmarchicus, the HOAD activity during winter was elevated compared to summer, and reached the highest levels just prior to the spring bloom. The relative abundance of adult C. finmarchicus also increased in March-April indicating molting and wake up from dormancy (Hatlebakk et al., this issue). Isfjorden is ice free during winter, and thus no ice algae can develop. Besides, C. finmarchicus is not known to feed on ice algae to fuel reproduction (Niehoff et al., 2002 (Disko Bay)). Thus, at that time of the year, the increased energy demand cannot be fueled by food uptake as the spring bloom is weeks ahead. The seasonal pattern in HOAD activities exhibited by C. finmarchicus thus differed from that of C. glacialis (Freese et al., 2017)
As MDH, CS is a proxy for the overall metabolism, and for this enzyme similar seasonal activity patterns were found for C. glacialis in Isfjorden and the colder Billefjorden, supporting the 50% reduction in metabolism in winter as previously estimated by Freese et al. (2017). CS has also been found to be positively correlated to egg production (Kreibich et al., 2008), which is further supported by our study. We found that peak CS activity in C. finmarchicus was correlated strongly to Chl a concentration, but not in C. glacialis. Both C. glacialis and C. finmarchicus reproduced over an extended period, but with different timing (Hatlebakk et al., 2022). Calanus glacialis males and females appeared in early January and produced eggs from late March on, with a strong peak at the onset of the spring bloom. Calanus finmarchicus adults appeared in February-March and started egg production at the onset of the spring bloom in early May and maintained egg production well into the summer (Eide, 2016; Hatlebakk et al., 2022). Thus, the correlation between Chl a and CS activity for C. finmarchicus likely mirrors that C. finmarchicus is an income breeder. Calanus glacialis, on the other hand, can start reproduction earlier based on internal reserves (capital breeding) before Chl a builds up in the water column of Isfjorden. Thus, when CS increases with egg production, this is not directly linked to the spring bloom in C. glacialis, as it is in C. finmarchicus.
Lipase and proteinase reached peak activities during the spring bloom, and earlier in C. glacialis than C. finmarchicus. Calanus finmarchicus have been found to be torpid and have reduced gut epithelium during overwintering (Hallberg and Hirche, 1980). Interestingly, specific lipase activities increased much more than proteinase in C. glacialis, while the opposite was the case in C. finmarchicus in which proteinase increased more than lipase. This may suggest that the two species differ with regard to their potential of using these two dietary enzymes. The higher proteinase activity in C. finmarchicus could be part of the adaptation to income-breeder strategy, utilizing food for final oocyte maturation, while C. glacialis invests substantial amounts of stored lipids into early egg production (Hirche and Kattner, 1993; Jónasdóttir, 1999; Hatlebakk, 2014). Calanus finmarchicus only needs sufficient lipids to sustain basic metabolism through diapause. The higher lipase mobilization in C. glacialis could be a physiological adaptation of this arctic species to fully utilize the generally short primary production window to fuel lipid accumulation.
Sampling off-shelf was focused on the period from March to June 2015, when the N-ICE 2015 campaign allowed for frequent sampling in the area. Then, the community was strongly dominated by C. finmarchicus (Hop et al., 2021), and thus, there are too few data for C. glacialis to compare with Isfjorden. The MDH and CS activities of C. finmarchicus showed similarly low activity levels prior to the bloom and similarly high levels in the bloom as in Isfjorden. Off-shelf, however, enzyme activities were not correlated with day length, which can be explained by the consolidated sea ice with snow on top and concomitantly low irradiances in the water column until late May (Assmy et al., 2017). In the Arctic Ocean, the ice algae and phytoplankton blooms are initiated later, and are of lower magnitude with shorter duration than in Svalbard fjords further south (Leu et al., 2011; Leu et al., 2015), which also was the case in 2015. Off-shelf, food availability increased not before the beginning of June (Assmy et al., 2017), while in Isfjorden, maximum Chl a was already recorded at the end of April (University Centre in Svalbard, 2020). Correspondingly, also the digestive enzyme activities increased later off-shelf, and thus, similar to Isfjorden, corresponded well to the Chl a development.
There are still questions to resolve regarding mechanisms controlling initiation and termination of diapause in Calanus. Based on gene expression patterns and synchronized ascent despite lack of light in their study, Häfker et al. (2018) suggest that an endogenous circannual clock is involved in the termination of diapause. Gene expression was not involved in our study, and unfortunately the community data are not overlapping for the two sites at the time of ascent (Hop et al., 2021, Hatlebakk et al., 2022). However, we note that the ascent of Calanus spp. was recorded in early May both off-shelf in 2015 (Hop et al., 2021) and in Isfjorden in 2016 (Hatlebakk et al., 2022), and whilst this is not sufficient to state synchronized ascent across the two sampling sites, it is in line with the hypothesis of Häfker et al. (2018). Even if the ascent to the surface occurred at the same time of the year, the increases in metabolic enzymes as well as digestive enzyme activities were first observed in connection with the increase in Chl a concentration. This was the case in early May in Isfjorden, but a month later off-shelf (Assmy et al., 2017). A previous study by Morata and Søreide (2015) has also demonstrated a link between termination of diapause and food availability. In their study, C. glacialis CV collected in winter (November) increased respiration when exposed to light. If food was not present, the respiration rates decreased again, while with the presence of food, the respiration remained elevated. Also, feeding during winter initiated an increase in the lipolytic activity of C. glacialis CV (Freese et al., 2012). This along with our findings that peak metabolic enzyme activity was measured alongside peak Chl a concentrations both in the fjord and off-shelf, indicate that food availability is an important signal for the final step of termination of diapause.
In our study, the seasonal regulation of anabolic and catabolic enzyme activities was overall similar for C. glacialis and C. finmarchicus, but differed in the variation of digestive enzymes throughout the seasons. Calanus glacialis may utilize dietary lipids more efficiently, as reflected by an earlier and higher increase in lipase enzyme activities, while C. finmarchicus showed higher increase in proteinase for digestion of proteins. Even small differences in the timing of life-history events can have large impacts on a species success (e.g., Varpe, 2012). A more efficient lipid anabolism of C. glacialis may provide an advantage over C. finmarchicus in high-Arctic unpredictable environments with short-pulsed primary production regimes. The accumulation of lipids allows for flexibility by providing an internal energy source, while the strategy of C. finmarchicus of relying on external food becomes a limitation with regard to the short-pulsed bloom which is typical for the high Arctic.
The strong seasonality in incoming solar radiation is predictable and will not change with global warming. However, sea ice, snow cover and open leads can strongly modulate the underwater light regime, which impacts the timing of the blooms. The arrival of Calanus in surface waters under the ice well before the bloom (Hop et al., 2021), the connection between enzyme activity and Chl a as shown in our study, and the increase in catabolism of stored lipids observed in C. finmarchicus prior to the spring bloom, indicate that external triggers such as food are important for the final steps of terminating diapause. Thus, while endogenous mechanisms, like a circannual clock, may be important for initiating termination of diapause, there appears to be mechanisms for tuning the timing between the producers and consumers in the planktonic food web to ensure a good match, even with interannual and spatial variability in bloom timing.
The raw data supporting the conclusions of this article will be made available by the authors, without undue reservation.
MH has conducted sampling, sample analyses, compiling first draft and edited the manuscript based on feedback from co-authors. BN has contributed with initial planning, guidance for enzyme analyses and interpretation of data, and extensive feedback to the manuscript. MC has contributed with molecular analyses, interpretation of this data and feedback to the manuscript. HH and AW have contributed with sampling off-shelf and feedback on the manuscript. GH has contributed with initial planning of the study, guidance for the molecular part of the study and feedback to the manuscript. JS has contributed with initial planning of the study, sampling in Isfjorden, interpretation of data, compiling the first draft and extensive feedback in the editing process. All authors contributed to the article and approved the submitted version.
This study was supported by the Centre for Ice, Climate and Ecosystems (ICE) at the Norwegian Polar Institute, the Ministry of Climate and Environment, Norway, the Research Council of Norway (projects COPPY no. 227139 IMOS no. 246747 and Boom or Bust no. 24464) and FRAM - High North Research Centre for Climate and the Environment, Flagship Arctic Ocean, project FADE no. 66009.
The authors declare that the research was conducted in the absence of any commercial or financial relationships that could be construed as a potential conflict of interest.
All claims expressed in this article are solely those of the authors and do not necessarily represent those of their affiliated organizations, or those of the publisher, the editors and the reviewers. Any product that may be evaluated in this article, or claim that may be made by its manufacturer, is not guaranteed or endorsed by the publisher.
We kindly acknowledge the captains and crew of RV Lance, RV Helmer Hanssen, RV Dalnie Zelentsy, RV Viking Explorer and UNIS logistics for making it possible to conduct this study’s year-round sampling. We are very grateful to A. Baily during the N-ICE campaign and other fellow colleagues for all their support and help during fieldwork. Thanks are also due to S. Murawski for her support with enzyme analyses, and H. Eide for help with sampling in Isfjorden.
The Supplementary Material for this article can be found online at: https://www.frontiersin.org/articles/10.3389/fmars.2022.877904/full#supplementary-material
Supplementary Figure 1 | Weighted mean depth of Calanus glacialis and C. finmarchicus (A) during the N-ICE 2015 campaign spring 2015 and (B) in Isfjorden from June 2015 to August 2016. Error bars indicate standard deviation.
Aarflot J. M., Skjoldal H. R., Dalpadado P., Skern-Mauritzen M. (2017). Contribution of Calanus Species to the Mesozooplankton Biomass in the Barents Sea. ICES J. Mar. Sci. 75, 2342–2354. doi: 10.1093/icesjms/fsx221
Assmy P., Fernández-Méndez M., Duarte P., Meyer A., Randelhoff A., Mundy C. J., et al. (2017). Leads in Arctic Pack Ice Enable Early Phytoplankton Blooms Below Snow-Covered Sea Ice. Sci. Rep. 7, 40850. doi: 10.1038/srep40850
Auerswald L., Gäde G. (1999). The Fate of Proline in the African Fruit Beetle Pachnoda sinuata. Insect Biochem. Molec. Biol. 29, 687–700. doi: 10.1016/S0965-1748(99)00045-4
Banas N. S., Møller E. F., Nielsen T. G., Eisner L. B. (2016). Copepod Life Strategy and Population Viability in Response to Prey Timing and Temperature: Testing a New Model Across Latitude, Time, and the Size Spectrum. Front. Mar. Sci. 3, 225. doi: 10.3389/fmars.2016.00225
Blachowiak-Samolyk K., Søreide J. E., Kwasniewski S., Sundfjord A., Hop H., Falk-Petersen S., et al. (2008). Hydrodynamic Control of Mesozooplankton Abundance and Biomass in Northern Svalbard Waters (79–81 N). Deep. Sea. Res. II. 55, 2210–2224. doi: 10.1016/j.dsr2.2008.05.018
Choquet M., Hatlebakk M., Dhanasiri A. K. S., Kosobokova K., Smolina I., Søreide J. E., et al. (2017). Genetics Redraws Pelagic Biogeography of Calanus. Biol. Lett. 13, 20170588. doi: 10.1098/rsbl.2017.0588
Choquet M., Kosobokova K., Kwaśniewski S., Hatlebakk M., Dhanasiri A. K., Melle W., et al. (2018). Can Morphology Reliably Distinguish Between the Copepods Calanus finmarchicus and C. Glacialis, or is DNA the Only Way? Limnol. Oceanogr.: Methods 16, 237–252. doi: 10.1002/lom3.10240
Conover R. J. (1988). Comparative Life Histories in the Genera Calanus and Neocalanus in High Latitudes of the Northern Hemisphere. Hydrobiologia 167, 127–142. doi: 10.1007/bf00026299
Daase M., Eiane K. (2007). Mesozooplankton Distribution in Northern Svalbard Waters in Relation to Hydrography. Polar Biol. 30, 969–981. doi: 10.1007/s00300-007-0255-5
Daase M., Kosobokova K., Last K. S., Cohen J. H., Choquet M., Hatlebakk M., et al. (2018). New Insights Into the Biology of Calanus spp. (Copepoda) Males in the Arctic. Mar. Ecol. Prog. Ser. 607, 53–69. doi: 10.3354/meps12788
Dahl T. M., Falk-Petersen S., Gabrielsen G. W., Sargent J. R., Hop H., Millar R.-M. (2003). Lipids and Stable Isotopes in Common Eider, Black-Legged Kittiwake and Northern Fulmar: A Trophic Study From an Arctic Fjord. Mar. Ecol. Prog. Ser. 256, 257–269. doi: 10.3354/meps256257
Dahms H.-U. (1995). Dormancy in the Copepoda—An Overview. Hydrobiologia 306, 199–211. doi: 10.1007/BF00017691
Darnis G., Fortier L. (2014). Temperature, Food and the Seasonal Vertical Migration of Key Arctic Copepods in the Thermally Stratified Amundsen Gulf (Beaufort Sea, Arctic Ocean). J. Plankton Res. 36, 1092–1108. doi: 10.1093/plankt/fbu035
Eide H. (2016). “Fate of Calanus spp. Reproduction and Development Under Different Environmental Stressors,” [Master's thesis]. (Tromsø, Norway: Department of Arctic and Marine Biology, UiT the Arctic University of Norway).
Ejsmond M. J., Mcnamara J. M., Søreide J., Varpe Ø. (2018). Gradients of Season Length and Mortality Risk Cause Shifts in Body Size, Reserves and Reproductive Strategies of Determinate Growers. Funct. Ecol. 32, 2395–2406. doi: 10.1111/1365-2435.13191
Falk-Petersen S., Hopkins C. C. E., Sargent J. R. (1990). “Trophic Relationships in the Pelagic, Arctic Food Web,” in Trophic Relationships in the Marine Environment. Eds. Barnes M., Gibson R. N. (Aberdeen: Aberdeen University Press), 315–333.
Falk-Petersen S., Mayzaud P., Kattner G., Sargent J. R. (2009). Lipids and Life Strategy of Arctic Calanus. Mar. Biol. Res. 5, 18–39. doi: 10.1080/17451000802512267
Falk-Petersen S., Pavlov V., Timofeev S., Sargent J. R. (2007). “Climate Variability and Possible Effects on Arctic Food Chains: The Role of Calanus,” in Arctic Alpine Ecosystems and People in a Changing Environment. Eds. Ørbæk J. B., Kallenborn R., Tombre I., Hegseth E. N., Falk-Petersen S., Hoel A. H. (Berlin, Heidelberg, Germany: Springer), 147–161.
Freese D., Niehoff B., Søreide J. E., Sartoris F. J. (2015). Seasonal Patterns in Extracellular Ion Concentrations and pH of the Arctic Copepod Calanus glacialis. Limnol. Oceanogr. 60, 2121–2129. doi: 10.1002/lno.10158
Freese D. (2015). Life History Traits of Copepods in a Changing Arctic – Seasonal Patterns in the Physiology of C. glacialis (Bremerhaven, Germany: Bremerhaven, Germany Alfred-Wegener-institute).
Freese D., Kreibich T., Niehoff B. (2012). Characteristics of Digestive Enzymes of Calanoid Copepod Species From Different Latitudes in Relation to Temperature, pH and Food. Comp. Biochem. Physiol. B.: Biochem. Molec. Biol. 162, 66–72. doi: 10.1016/j.cbpb.2012.04.007
Freese D., Søreide J. E., Graeve M., Niehoff B. (2017). A Year-Round Study on Metabolic Enzymes and Body Composition of the Arctic Copepod Calanus glacialis: Implications for the Timing and Intensity of Diapause. Mar. Biol. 164, 3. doi: 10.1007/s00227-016-3036-2
Freese D., Søreide J. E., Niehoff B. (2016). A Year-Round Study on Digestive Enzymes in the Arctic Copepod Calanus glacialis: Implications for its Capability to Adjust to Changing Environmental Conditions. Polar Biol. 39, 2241–2252. doi: 10.1007/s00300-016-1891-4
Gabrielsen T. M., Merkel B., Søreide J. E., Johansson-Karlsson E., Bailey A., Vogedes D., et al. (2012). Potential Misidentifications of Two Climate Indicator Species of the Marine Arctic Ecosystem: Calanus glacialis and C. finmarchicus. Polar Biol. 35, 1621–1628. doi: 10.1007/s00300-012-1202-7
GADM. (2015). Data Extracted from the GADM Database (https://www.gadm.org), Version 2.8, November 2015.
Granskog M. A., Fer I., Rinke A., Steen H. (2018). Atmosphere-Ice-Ocean-Ecosystem Processes in a Thinner Arctic Sea Ice Regime: The Norwegian YoungSea Ice (N-ICE2015) Expedition. J. Geophys. Res. Oceans 123, 1586—1594. doi: 10.1002/2017JC013328
Guppy M., Withers P. (1999). Metabolic Depression in Animals: Physiological Perspectives and Biochemical Generalizations. Biol. Rev. 74, 1–40. doi: 10.1017/S0006323198005258
Häfker N. S., Teschke M., Last K. S., Pond D. W., Hüppe L., Meyer B. (2018). Calanus finmarchicus Seasonal Cycle and Diapause in Relation to Gene Expression, Physiology, and Endogenous Clocks. Limnol. Oceanogr. 63, 2815–2838. doi: 10.1002/lno.11011
Hallberg E., Hirche H.-J. (1980). Differentiation of Mid-Gut in Adults and Over-Wintering Copepodids of Calanus finmarchicus (Gunnerus) and Calanus helgolandicus Claus. J. Exper. Mar. Biol. Ecol. 48, 283–295. doi: 10.1016/0022-0981(80)90083-0
Hassett R. P. (2006). Physiological Characteristics of Lipid-Rich 'Fat' and Lipid-Poor 'Thin' Morphotypes of Individual Calanus finmarchicus C5 Copepodites in Nearshore Gulf of Maine. Limnol. Oceanogr. 51, 997–1003. doi: 10.4319/lo.2006.51.2.0997
Hatlebakk M. (2014). Capital or Income Breeder: The Role of Lipids and Fatty Acid Composition for Successful Reproduction in Calanus glacialis [Master's thesis]. Trondheim, Norway: Norwegian University of Science and Technology and University Centre in Svalbard
Hatlebakk M., Kosobokova K., Daase M., Søreide J. E. (2022). Contrasting Life Traits of Sympatric Calanus glacialis and C. finmarchicus in a Warming Arctic Revealed by a Year-Round Study in Isfjorden, Svalbard. Front. Mar. Sci. 9, 877910. doi: 10.3389/fmars.2022.877910
Hays G. C., Richardson A. J., Robinson C. (2005). Climate Change and Marine Plankton. Trends Ecol. Evol. 20, 337–344. doi: 10.1016/j.tree.2005.03.004
Head E. J. H., Conover R. J. (1983). Induction of Digestive Enzymes in Calanus hyperboreus. Mar. Biol. Lett. 4, 219–231.
Hirche H.-J. (1983). Overwintering of Calanus finmarchicus and Calanus helgolandicus. Mar. Ecol. Prog. Ser. 11, 281–290. doi: 10.3354/meps011281
Hirche H.-J. (1996). Diapause in the Marine Copepod, Calanus finmarchicus—a Review. Ophelia 44, 129–143. doi: 10.1080/00785326.1995.10429843
Hirche H.-J. (1998). Dormancy in Three Calanus Species (C. finmarchicus, C. glacialis and C. hyperboreus) From the North Atlantic. Archiv. Hydrobiol. Spec. Iss. Adv. Limnol. 52, 359–369.
Hirche H.-J., Kattner G. (1993). Egg Production and Lipid Content of Calanus glacialis in Spring: Indication of a Food-Dependent and Food-Independent Reproductive Mode. Mar. Biol. 117, 615–622. doi: 10.1007/BF00349773
Hop H., Wold A., Vihtakari M., Daase M., Kwasniewski S., Gluchowska M., et al. (2019a). “Zooplankton in Kongsfjorden, (1996–2016) in Relation to Climate Change,” in The Ecosystem of Kongsfjorden, Svalbard: Advances in Polar Ecology, vol. 2 . Eds. Hop H., Wiencke C. (Cham: Springer Verlag), 229–303. doi: 10.1007/978-3-319-46425-1_7
Hop H., Assmy P., Wold A., Sundfjord A., Daase M., Duarte, et al. (2019b). Pelagic Ecosystem Characteristics Across the Atlantic Water Boundary Current From Rijpfjorden, Svalbard, to the Arctic Ocean During Summer, (2010-2014). Front. Mar. Sci. 6. doi: 10.3389/fmars.2019.00181
Hop H., Wold A., Meyer A., Bailey A., Hatlebakk M., Kwasniewski S., et al. (2021). Winter-Spring Development of the Zooplankton Community Below Sea Ice in the Arctic Ocean. Front. Mar. Sci. 8, 551. doi: 10.3389/fmars.2021.609480
Jaschnov W. (1970). Distribution of Calanus Species in the Seas of the Northern Hemisphere. Internat. Rev. Hydrobiol. Hydrogr. 55, 197–212. doi: 10.1002/iroh.19700550203
Jónasdóttir S. H. (1999). Lipid Content of Calanus finmarchicus During Overwintering in the Faroe–Shetland Channel. Fish. Oceanogr. 8, 61–72. doi: 10.1046/j.1365-2419.1999.00003.x
Kjellerup S., Dunweber M., Swalethorp R., Nielsen T. G., Møller E. F., Markager S., et al. (2012). Effects of a Future Warmer Ocean on the Coexisting Copepods Calanus finmarchicus and C. glacialis in Disko Bay, Western Greenland. Mar. Ecol. Prog. Ser. 447, 87–108. doi: 10.3354/meps09551
Knotz S., Boersma M., Saborowski R. (2006). Microassays for a Set of Enzymes in Individual Small Marine Copepods. Comp. Biochem. Physiol. A.: Molec. Integr. Physiol. 145, 406–411. doi: 10.1016/j.cbpa.2006.07.019
Kosobokova K. N., Hopcroft R. R., Hirche H.-J. (2011). Patterns of Zooplankton Diversity Through the Depths of the Arctic’s Central Basins. Mar. Biodiv. 41, 29–50. doi: 10.1007/s12526-010-0057-9
Kreibich T., Saborowski R., Hagen W., Niehoff B. (2008). Short-Term Variation of Nutritive and Metabolic Parameters in Temora longicornis Females (Crustacea, Copepoda) as a Response to Diet Shift and Starvation. Helgol. Mar. Res. 62, 241. doi: 10.1007/s10152-008-0112-0
Landry M. R. (1983). The Development of Marine Calanoid Copepods With Comment on the Isochronal Rule. Limnol. Oceanogr. 28, 614–624. doi: 10.4319/lo.1983.28.4.0614
Lee R. F., Hagen W., Kattner G. (2006). Lipid Storage in Marine Zooplankton. Mar. Ecol. Prog. Ser. 307, 273–306. doi: 10.3354/meps307273
Leu E., Mundy C. J., Assmy P., Campbell K., Gabrielsen T. M., Gosselin M., et al. (2015). Arctic Spring Awakening–Steering Principles Behind the Phenology of Vernal Ice Algal Blooms. Progr. Oceanogr. 139, 151–170. doi: 10.1016/j.pocean.2015.07.012
Leu E., Søreide J. E., Hessen D., Falk-Petersen S., Berge J. (2011). Consequences of Changing Sea-Ice Cover for Primary and Secondary Producers in the European Arctic Shelf Seas: Timing, Quantity, and Quality. Progr. Oceanogr. 90, 18–32. doi: 10.1016/j.pocean.2011.02.004
Møller E. F., Bohr M., Kjellerup S., Maar M., Møhl M., Swalethorp R., et al. (2016). Calanus finmarchicus Egg Production at its Northern Border. J. Plankton Res. 38, 1206–1214. doi: 10.1093/plankt/fbw048
Meyer B., Auerswald L., Siegel V., Spahić S., Pape C., Fach B. A., et al. (2010). Seasonal Variation in Body Composition, Metabolic Activity, Feeding, and Growth of Adult Krill Euphausia superba in the Lazarev Sea. Mar. Ecol. Prog. Ser. 398, 1–18. doi: 10.3354/meps08371
Meyer B., Saborowski R., Atkinson A., Buchholz F., Bathmann U. (2002). Seasonal Differences in Citrate Synthase and Digestive Enzyme Activity in Larval and Postlarval Antarctic Krill, Euphausia superba. Mar. Biol. 141, 855–862. doi: 10.1007/s00227-002-0877-7
Meyer A., Sundfjord A., Fer I., Provost C., Villacieros Robineau N., Koenig Z., et al. (2017). Winter to Summer Oceanographic Observations in the Arctic Ocean North of Svalbard. J. Geophys. Res. Ocean. 122, 6218–6237. doi: 10.1002/2016JC012391
Montero-Pau J., Gómez A., Muñoz J. (2008). Application of an Inexpensive and High-Throughput Genomic DNA Extraction Method for the Molecular Ecology of Zooplanktonic Diapausing Eggs. Limnol. Oceanogr. Met. 6, 218–222. doi: 10.4319/lom.2008.6.218
Morata N., Søreide J. E. (2015). Effect of Light and Food on the Metabolism of the Arctic Copepod Calanus glacialis. Polar Biol. 38, 67–73. doi: 10.1007/s00300-013-1417-2
Niehoff B., Madsen S. D., Hansen B. W., Nielsen T. G. (2002). Reproductive Cycles of Three Dominant Calanus Species in Disko Bay, West Greenland. Mar. Biol. 140, 567–576. doi: 10.1007/s00227-001-0731-3
Nielsen T. G., Kjellerup S., Smolina I., Hoarau G., Lindeque P. (2014). Live Discrimination of Calanus glacialis and C. finmarchicus Females: Can We Trust Phenological Differences? Mar. Biol. 161, 1299–1306. doi: 10.1007/s00227-014-2419-5
Nilsen F., Cottier F., Skogseth R., Mattsson S. (2008). Fjord–shelf Exchanges Controlled by Ice and Brine Production: The Interannual Variation of Atlantic Water in Isfjorden, Svalbard. Cont. Shelf Res. 28, 1838–1853. doi: 10.1016/j.csr.2008.04.015
Ohman M. D., Drits A. V., Clarke M. E., Plourde S. (1998). Differential Dormancy of Co-Occurring Copepods. Deep. Sea. Res. II. 45, 1709–1740. doi: 10.1016/S0967-0645(98)80014-3
Plourde S., Runge J. A. (1993). Reproduction of the Planktonic Copepod Calanus finmarchicus in the Lower St. Lawrence Estuary: Relation to the Cycle of Phytoplankton Production and Evidence for a Calanus Pump. Mar. Ecol. Prog. Ser. 102, 217–227. doi: 10.3354/meps102217
Rudels B., Meyer R., Fahrbach E., Ivanov V., Østerhus S., Quadfasel D., et al. (2000). Water Mass Distribution in Fram Strait and Over the Yermak Plateau in Summer 1997. Annal. Geophys. 18, 687–705. doi: 10.1007/s00585-000-0687-5
Saborowski R., Bröhl S., Tarling G., Buchholz F. (2002). Metabolic Properties of Northern Krill, Meganyctiphanes norvegica, From Different Climatic Zones. I. Respiration and Excretion. Mar. Biol. 140, 547–556. doi: 10.1007/s00227-001-0730-4
Saborowski R., Sahling G., Del Toro M. N., Walter I., Garcıa-Carreno F. (2004). Stability and Effects of Organic Solvents on Endopeptidases From the Gastric Fluid of the Marine Crab Cancer pagurus. J. Molec. Catal. B.: Enzymat. 30, 109–118. doi: 10.1016/j.molcatb.2004.04.002
Sargent J. R., Falk-Petersen S. (1988). “The Lipid Biochemistry of Calanoid Copepods,” in Biology of Copepods (Dordrecht, Netherlands: Springer).
Skogseth R., Olivier L. L. A., Nilsen F., Falck E., Fraser N., Tverberg V., et al. (2020). Variability and Decadal Trends in the Isfjorden (Svalbard) Ocean Climate and Circulation - a Thermometer for Climate Change in the Arctic Ocean. Progr. Oceanogr. 187, 102394. doi: 10.1016/j.pocean.2020.102394
Smolina I., Kollias S., Poortvliet M., Nielsen T. G., Lindeque P., Castellani C., et al. (2014). Genome-And Transcriptome-Assisted Development of Nuclear Insertion/Deletion Markers for Calanus Species (Copepoda: Calanoida) Identification. Molec. Ecol. Res. 14, 1072–1079. doi: 10.1111/1755-0998.12241
Stitt M. (1984). “Citrate Synthase (Condensing Enzyme),” in In Methods of Enzymatic Analysis, vol. 4 . Ed. Bergmeyer H. U.(Chemie, Weinheim: Elsevier Inc), 353–358.
Teschke M., Kawaguchi S., Meyer B. (2007). Simulated Light Regimes Affect Feeding and Metabolism of Antarctic Krill, Euphausia superba. Limnol. Oceanogr. 52, 1046–1054. doi: 10.4319/lo.2007.52.3.1046
Torres J., Somero G. (1988). Metabolism, Enzymic Activities and Cold Adaptation in Antarctic Mesopelagic Fishes. Mar. Biol. 98, 169–180. doi: 10.1007/BF00391192
Trudnowska E., Balazy K., Ston-Egiert J., Smolina I., Brown T., Gluchowska M. (2020). In a Comfort Zone and Beyond—Ecological Plasticity of Key Marine Mediators. Ecol. Evol. 10, 14067–14081. doi: 10.1002/ece3.6997
University Centre in Svalbard (2020) ISA_Svalbard_Chlorophyll_a_2011_2019. Available at: https://doi.org/10.11582/2020.00063.
Varpe Ø. (2012). Fitness and Phenology: Annual Routines and Zooplankton Adaptations to Seasonal Cycles. J. Plankton Res. 34, 267–276. doi: 10.1093/plankt/fbr108
Varpe Ø., Ejsmond M. J. (2018). Trade-Offs Between Storage and Survival Affect Diapause Timing in Capital Breeders. Evol. Ecol. 32, 623–641. doi: 10.1007/s10682-018-9961-4
Wassmann P., Kosobokova K. N., Slagstad D., Drinkwater K. F., Hopcroft R. R., Moore S. E., et al. (2015). The Contiguous Domains of Arctic Ocean Advection: Trails of Life and Death. Progr. Oceanogr. 139, 42–65. doi: 10.1016/j.pocean.2015.06.011
Keywords: calanoid copepods, overwintering, metabolism, enzyme activities, Svalbard
Citation: Hatlebakk M, Niehoff B, Choquet M, Hop H, Wold A, Hoarau G and Søreide JE (2022) Seasonal Enzyme Activities of Sympatric Calanus glacialis and C. finmarchicus in the High-Arctic. Front. Mar. Sci. 9:877904. doi: 10.3389/fmars.2022.877904
Received: 17 February 2022; Accepted: 26 April 2022;
Published: 16 June 2022.
Edited by:
Sigrun Huld Jonasdottir, Technical University of Denmark, DenmarkReviewed by:
Mie Hylstofte Sichlau Winding, Greenland Climate Research Centre, GreenlandCopyright © 2022 Hatlebakk, Niehoff, Choquet, Hop, Wold, Hoarau and Søreide. This is an open-access article distributed under the terms of the Creative Commons Attribution License (CC BY). The use, distribution or reproduction in other forums is permitted, provided the original author(s) and the copyright owner(s) are credited and that the original publication in this journal is cited, in accordance with accepted academic practice. No use, distribution or reproduction is permitted which does not comply with these terms.
*Correspondence: Maja Hatlebakk, bWFqYS5rLnYuaGF0bGViYWtrQG50bnUubm8=
Disclaimer: All claims expressed in this article are solely those of the authors and do not necessarily represent those of their affiliated organizations, or those of the publisher, the editors and the reviewers. Any product that may be evaluated in this article or claim that may be made by its manufacturer is not guaranteed or endorsed by the publisher.
Research integrity at Frontiers
Learn more about the work of our research integrity team to safeguard the quality of each article we publish.