- 1Earth Dynamic System Research Center, National Cheng Kung University, Tainan, Taiwan
- 2Department of Earth Sciences, National Cheng Kung University, Tainan, Taiwan
- 3Institute of Earth Sciences, Academia Sinica, Taipei, Taiwan
- 4State Key Laboratory of Marine Geology, Tongji University, Shanghai, China
Boron (B) and B isotopic compositions (δ11B) in biogenic carbonates are useful proxies for pH reconstruction in the ocean. However, high-resolution archives are scarce due to associated sampling and analytical difficulty. In this study, a modern long-lived massive coral skeleton (Porites lobata) from Lanyu Islet off southeast Taiwan was drilled and used for high-resolution major/trace element analyses, including trace elements B and δ11B, as well as oxygen and carbon isotopes, to investigate the associated environmental changes during 1991–1997. To avoid complicated biological influence, the top-most tissue layer was excluded in this study. The coralline records show a clear temporal trend in metal/Ca-based sea surface temperatures (SSTs) on annual and monthly timescales. In particular, the Mg/Ca-SSTs, the most sensitive temperature proxy at the site, show a significant warming trend (+0.2°C year−1) during the study period. On the other hand, subtle changes in the annual δ11B record were identified, corresponding to ~0.2 pH unit, which is comparable with other coral records in the Pacific, e.g., the South China Sea (SCS), Guam Island, Flinders, and Arlington Reef, as well as the in-situ seawater pH measurement at Hawaii station. This corresponds to an acidification rate of ~0.25 pH unit 100 year−1, similar to other coralline data, in-situ pH/pCO2 measurement, or model predictions, and emphasizes the importance of ocean acidification due to anthropogenic activities. Combined with the Mg/Ca-SST, the intra-annual data show a clear seasonal cycle with higher pH in winter, consistent with the pCO2 at the oceanic surface. These chemical and isotopic results in corals conclude that marine biogenic carbonates are informative for oceanic pH reconstruction and can provide new insights into the relationships between climate changes and environmental responses on the coast of Taiwan.
Introduction
The evidence for climate change is unequivocal as the consensus in the Fourth Assessment Report of the Intergovernmental Panel on Climate Change (IPCC) indicated (Hoegh-Guldberg et al., 2007; Pachauri, 2008; IPCC, 2014; Liu et al., 2014). Various climatic model predictions and proxy reconstructions suggest that the increase in atmospheric CO2 relative to the pre-industrial era has already caused ocean acidification due to the uptake of anthropogenic CO2 (Caldeira and Wickett, 2003; Kasemann et al., 2009; Louvat et al., 2011). This acidification is predicted to decrease the level of carbonate saturation in seawater and lead to unfavorable living conditions for marine organisms (e.g., coccolithophorids, foraminifera, and corals) by disrupting the calcification processes necessary for shell/skeleton growth (Orr et al., 2005).
Many isotopic compositions in coral skeletons are primarily controlled by environmental parameters of ambient seawater, and thus, the patterns in trace elements and isotope compositions of fossil corals offer a robust approach to reconstruct the physical and chemical properties of the ocean (Quinn and Sampson, 2002; Cohen and McConnaughey, 2003; Pelejero et al., 2005; Ourbak et al., 2006; Shimamura et al., 2008). Most coralline trace elements are incorporated directly into aragonite skeletons from ambient seawater (Reuer et al., 2003). The trace element ratios of B, Mg, Sr, and U in coral skeletons can reflect faithfully the seawater compositions and physical conditions during calcification. The long residence times of B, Mg, Sr, and U (>1 Ma) cause relatively homogeneous distributions of these elements in the oceans (Allison and Finch, 2010). Also, temperature or other environmental variables may regulate trace element ratios in coral skeletons (Wei et al., 2000; Quinn and Sampson, 2002; Ourbak et al., 2006). On the other hand, the stable isotopes of oxygen (O) and carbon (C) in biogenic carbonates can provide an accurate reconstruction of past oceanic conditions (Hönisch et al., 2004; Reynaud et al., 2004; Omata et al., 2005; Shimamura et al., 2008; Moyer and Grottoli, 2011). Several factors may influence the stable isotope compositions in biogenic carbonates, such as isotopic equilibrium (e.g., temperature), kinetic isotope effects (e.g., growth rate), and metabolic isotope effects (i.e., photosynthesis and respiration) (Omata et al., 2005).
In the marine carbonate system, gaseous CO2 dissolves into surface seawater in proportion to the partial pressure of CO2 (pCO2). To understand any changes in the system, at least two of the co-varying parameters of pH, [CO2], [HCO3−], [CO32−], total alkalinity (TA), and total dissolved carbon (DIC) need to be constrained. By using these parameters, the state of the carbonate system can be reconstructed for the evaluation of past changes in saturation state with respect to atmospheric CO2. In this study, we focus on seawater pH reconstruction using boron isotopic composition in coral skeletons. There are many studies that used boron isotopic composition in biogenic carbonate to reconstruct past oceanic pH (Spivack et al., 1993; Gaillardet and Allègre, 1995; Pearson and Palmer, 2000; Sanyal et al., 2000; Hönisch et al., 2004; Hönisch et al., 2007; Foster, 2008; Liu et al., 2009). This application depends on the degree of isotopic fractionation between the two aqueous species, boric acid B(OH)3 and borate ion B(OH)4−, in seawater, as well as the pH dependence on the relative proportions of the two species (Figure 1), and 20‰ fractionation between the two dissolved boron species was experimentally calculated by Kakihana et al. (1977). An important assumption adopted in the calculation is that only the charged species, B(OH)4−, can be incorporated into calcium carbonates with insignificant fractionation during calcification (Hemming and Hanson, 1992; Hemming et al., 1995):
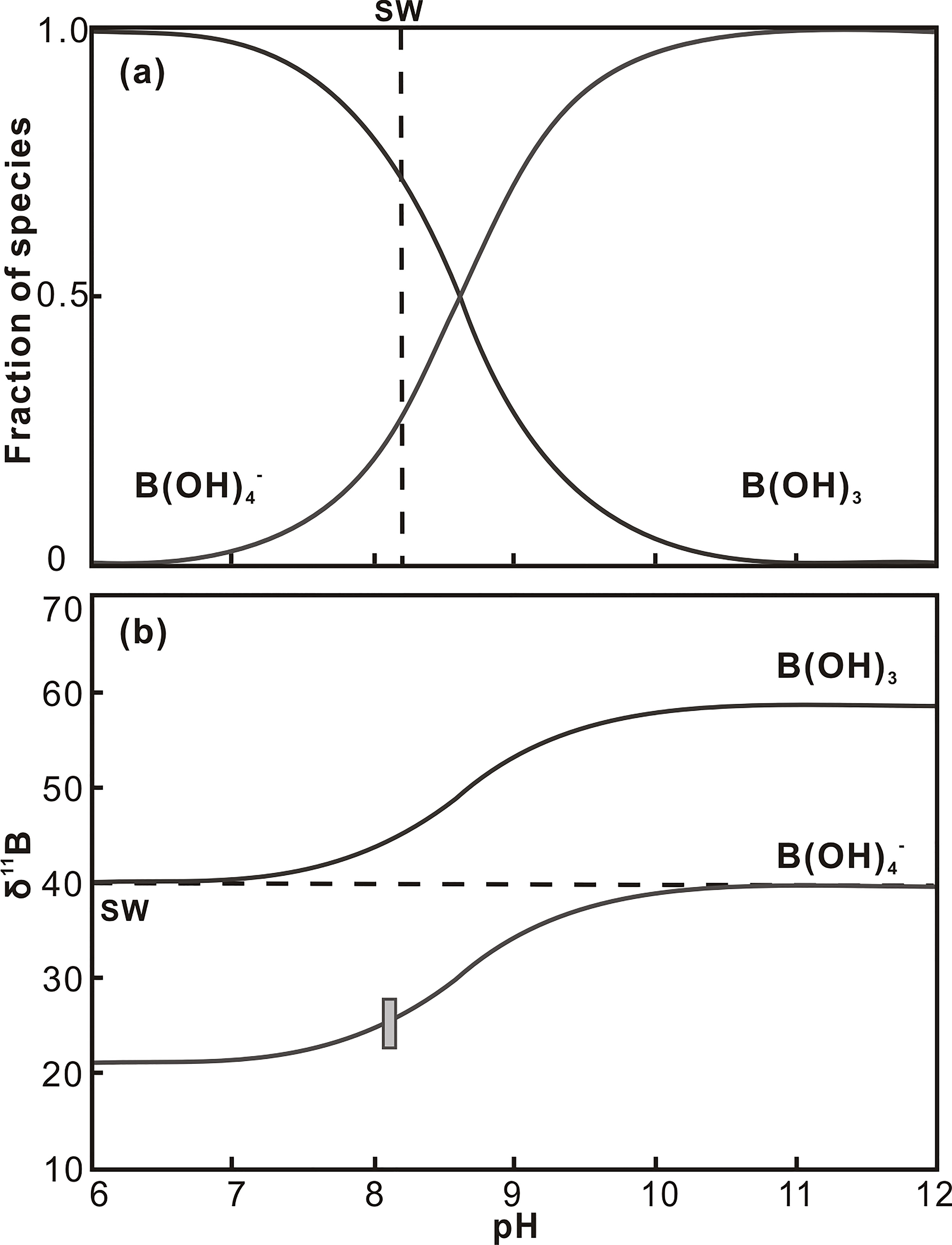
Figure 1 Distribution of (A) aqueous boron species and (B) boron isotopic composition as a function of pH in solutions. Note that the dashed line indicates modern seawater pH (~8.2), and the δ11B of modern carbonates falls near the theoretical line of B(OH)4− species.
It is critical to evaluate whether the boron isotopic composition of biogenic carbonates can serve as a reliable oceanic pH proxy. Several lines of evidence support the utility of this tracer based on synthetic (Hemming et al., 1995) and culture experiments (Sanyal et al., 2001; Hönisch et al., 2004), which have shown that the boron isotopic composition of biogenic carbonates accurately records variations in seawater pH. On the other hand, boron isotopes in marine carbonates are increasingly used to reconstruct seawater pH and atmospheric pCO2 through the Earth’s history. While isotope ratio measurements from individual laboratories are often of high quality, it is important that records generated in different laboratories can equally be compared (Gutjahr et al., 2021; Stewart et al., 2021). Also, there are recent advancements in studying biological effects using sensitive tracers in calcifying fluids (Venn et al., 2013; Cornwall et al., 2017; Comeau et al., 2018); however, those issues are beyond the scope of this study, as we have carefully avoided the tissue layer during our sampling or cleaning processes [see also McCulloch et al. (2017) for in-depth discussion].
In this study, we established a low blank technique for the precise measurement of δ11B in coralline skeleton, to establish a high-resolution temporal record of seawater pH, collected from Lanyu Islet, western Pacific. This represents the first use of inter-annual and intra-annual variations in surface ocean pH to understand seasonal CO2 variation in the atmosphere in this region.
Material and methods
Geological setting
Lanyu Islet, located offshore of southeast Taiwan (121.6°E, 22°N) (Figure 2), is an ideal location for high-resolution paleoclimatic study in the western Pacific. Lanyu is within the subtropical climatic zone and is strongly influenced by the East Asia monsoon system. The summer monsoon, a warm and wet season, begins in May with prevailing southwest winds. The winter monsoon, cold and dry, starts in November and is characterized by strong northeast winds originating from the Siberian High Pressure Zone. Heavy rainfall caused by typhoon events often occurs in Lanyu in summer, but no significant riverine discharge can reach Lanyu from Taiwan.
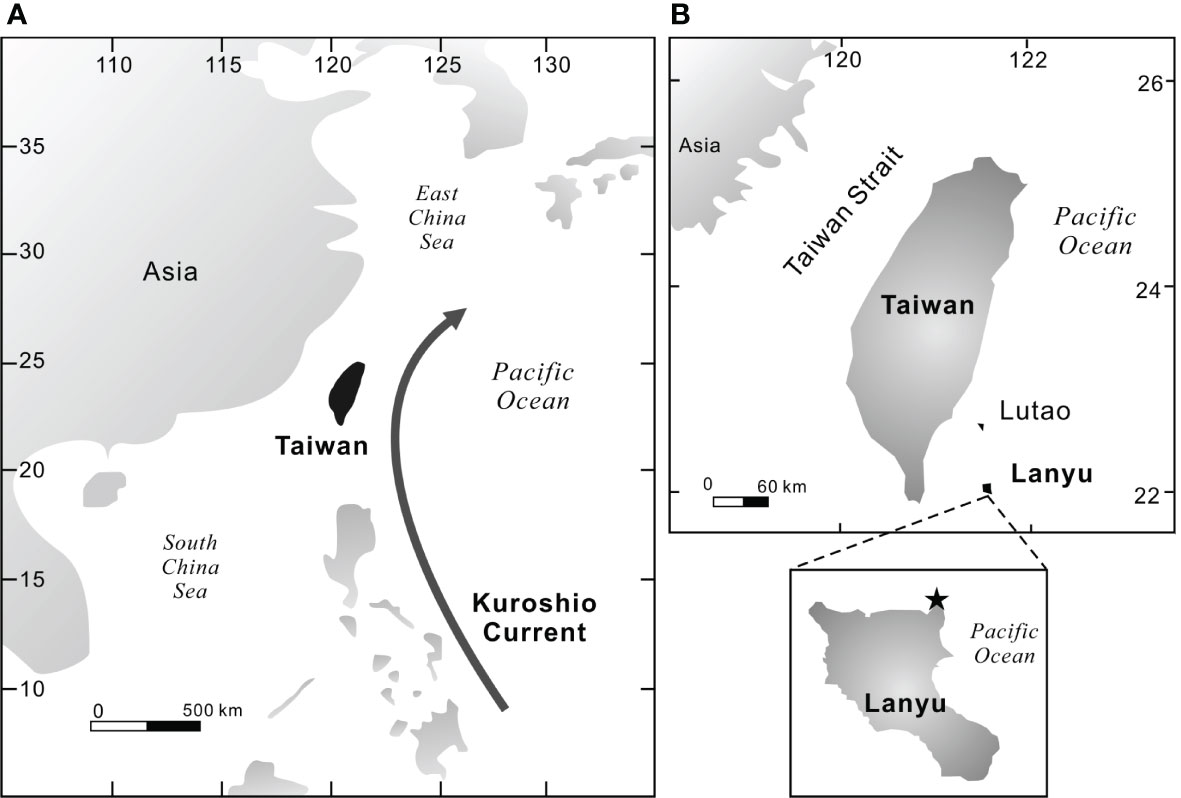
Figure 2 Location of the Lanyu Islet. This map shows (A) the path of the Kuroshio Current and the East Asian Monsoon and (B) the coral coring site off NE Lanyu Islet.
The Kuroshio Current, a major western boundary current in the western Pacific, is the most important surface current in the vicinity. It originates from the North Equatorial Current that bifurcates between 12 and 15°N in the western Pacific. The northward branch becomes the Kuroshio Current and the southward branch evolves into the Mindanao Current. The Kuroshio Current flows northward along the coasts of Luzon and Taiwan and continues to the shelf edge of the East China Sea. The Kuroshio Current transports warm water from the tropical ocean to mid-latitudes and is an important source of heat for global balance in the atmosphere (Chen et al., 2006). Its transport flux varies between 5 and 21 Sv (Sv = 106 m3 s−1) off western and eastern Lanyu Islet, respectively (Hsin et al., 2008).
Sampling and cleaning procedure
A 95-cm core of long-lived massive coral skeleton (Porites lobata) was drilled off northeast Lanyu in May 1997 and has been studied carefully for major/trace elements (Yu et al., 2015). The core was cut into a 1-cm-thick slab, cleaned with distilled water, and X-rayed. Monthly samples were cut along the major growth axis using a microtome, with 0.5-mm increments corresponding to a 1-month resolution (average 20 mg). All specimens were crushed using a PE rod and 1 mg of subsamples were used for oxygen and carbon isotopic analyses.
Its average extension rate is ~12 mm year−1. Mg/Ca in the Lanyu coral skeletons correlates strongly with sea surface temperature (SST) (R2 = 0.9) and provides better sensitivity than Sr/Ca for temperature reconstruction (Yu et al., 2015).
The chronology of this coral core is constructed by an SST calibration equation based on the Sr/Ca ratio and the satellite temperature record and corroborated by counting coral X-ray density bands, according to the relationship below:
Despite the potential bias induced by either the time resolution of each coral specimen or the spatial resolution of satellite-derived SST, the variation of temperature with the amplitude of 8°C is consistent with the instrumental record of a site nearby Lanyu (Shen et al., 2005). The slope in the calibration equation also agrees with literature-reported values using the same coral species (Corre`ge, 2006), with an estimated age uncertainty of<3 months (Charles et al., 1997).
All the cleaning and purification steps were carried out in a class-10 flow hood inside a class-10,000 cleanroom, and the main steps follow that of Hönisch and Hemming (2005). First, coral powders were bleached with 6%–14% sodium hypochlorite (NaOCl), repeatedly rinsed, and ultrasonicated with distilled water at least five times. Then, 0.0075 N of double-distilled HNO3 was added and this was repeated several times. The purified powder was dissolved with excess ultrapure HNO3 to ensure acidic conditions for the microsublimation technique.
Boron isotope analysis
Purification: microsublimation technique
It is necessary to separate B completely from the potential Ca matrix for isotopic determination. A microsublimation technique, modified from Gaillardet et al. (2001), was used to extract B from calcium carbonates to circumvent any column separation or complicate a chemical reagent. It is more effective for the purification and also for the elimination of potential contamination (Wang et al., 2020). Furthermore, this technique reduces the influence of organic matter from the ion exchange resin or the sample itself. A solution, ~40 µl, was placed on the cover of a 5-ml perfluoroalkoxy (PFA) conical beaker (Savillex, Eden Prairie, Minnesota USA), and heated on a dry bath system (Firefox Dry Bath 6100, Taipei City, Taiwan) for 12 h at 98°C. In order to eliminate matrix effects, a series of recovery experiments were conducted to assess our B separation under various matrices of Ca- and Na-added solutions (Wang et al., 2010).
Instrumental analysis
Precise analytical methods for boron isotopic measurements in natural materials have been discussed in detail (Spivack and Edmond, 1986; Guerrot et al., 2011). Despite the importance of marine biogenic carbonates for paleoceanographic studies, B isotopic applications are scarce owing to analytical difficulties, which require an accurate and high-precision technique for a small amount of sample (a few nanograms of B). Recent advances in multicollector inductively coupled plasma mass spectrometry (MC-ICP-MS) techniques are capable of precise isotopic analyses for a wide range of samples (Stewart et al., 2021).
In this study, a precise analytical technique for B isotopic determination in coral skeletons was developed using MC-ICP-MS (Thermo Fisher Neptune, Bremen, Germany) at the Earth Dynamic System Research Center (EDSRC), NCKU. The MC-ICP-MS has more advantages than the traditional thermal ionization mass spectrometry (TIMS). For instance, 1) the required sample amount is relatively small, 2) both the sample throughput and analytical precision are significantly higher than TIMS, and 3) the mass discrimination inherited from the instrument itself can be corrected by the sample-standard bracketing procedure.
A quartz spray dual chamber [Stable Sample Introduction (SSI), ESI] and a 50-μl PFA-ST nebulizer (ESI) were used to minimize boron blank and enhance signal stability. The Ni sampler and skimmer cones were used and the RF power was set at 1,200 W. Sample gas was adjusted to 1.05–1.10 L min−1, and the flow rates of the cooling gas and auxiliary gas were set at 15.0 and 0.7 L min−1, respectively. The typical sensitivity of B is ~0.7–1 V for 100 ppb at an uptake rate of 60 μl min−1. Ammonia solution was used to reduce the memory effect. Boron isotopes, 11B and 10B, were measured simultaneously on H3 and L3 Faraday cups, respectively, and 60 ratios with 6 blocks were collected with 2 s integration. Each analysis takes approximately 2 min. A quartz spray dual chamber coupled to a 50-μl PFA-ST nebulizer was used for sample introduction.
For most elements, memory effects can be resolved by washing with diluted HNO3 in the introduction system. In the case of boron, however, the interference cannot be reduced effectively using HNO3. As described by Al-Ammar et al. (1999), the addition of ammonia solution (NH4OH) to the spray chamber of ICP-MS can reduce the absorbability of B-bearing species, thus reducing memory effects more effectively.
Unlike the common internal or external correction for precise isotope determinations, B only has two isotopes and cannot be corrected for the mass bias by traditional normalization methods. The instrumental mass bias correction for B can be carried out by the sample-standard bracketing technique (Aggarwal et al., 2003), and δ11B values can be directly calculated by comparing the measured boron isotope ratios to standards. To establish a reliable isotope standard for boron using MC-ICP-MS, δ11B values of different standard materials were also analyzed by TIMS (Thermo Fisher, Triton T1), in positive ion mode at EDSRC, NCKU. Several reference materials, including synthetic aragonites (AS10, AS30, and AS50), KTP-1 (in-house coral standard), JA-A, and JA-B, were prepared for cross-calibration of δ11B values measured by MC-ICP-MS and P-TIMS.
Elemental ratios and other isotope analyses
The distribution of trace elements in coral skeletons was analyzed by HR-ICP-MS (Thermo Fisher, Element 2) at EDSRC, NCKU. Both the quartz spray chamber and the PFA nebulizer were used. The sensitivity of the instrument was optimized with a tuning solution by adjusting the operation parameters, such as the XYZ position of the plasma, gas flows, and lens voltages to attend to a maximum and stable signal for 7Li-115In-238U, and generally the sensitivity could reach ~0.8–1.2 × 106 cps ppb−1 for 238U. Carbon and oxygen isotopes were analyzed by a stable isotope mass spectrometer (Finnigan MAT252, Bremen, Germany) at the State Key Laboratory of Marine Geology, Tongji University.
Results
All analytical results obtained in this study, including trace element/Ca ratios and the isotopic compositions of B, O, and C, are summarized in the Supplementary Material Table S1. Analytical precision (2σ) of the coral standard (KTP-1) was 2.1% for B/Ca, 1.6% for Mg/Ca and Sr/Ca, and 6.2% for U/Ca. Carbon and oxygen isotopes were analyzed by a stable isotope mass spectrometer with a precision better than ±0.10‰.
These results show a large magnitude of Mg/Ca variation and apparent increases of Mg/Ca, Sr/Ca, and U/Ca, as well as a decreasing trend in δ13C record. The annual δ11B variation is around 2‰, ranging from 23.63‰ to 25.86‰, during the study period, and the estimated uncertainty of long-term δ11B measurement is ±0.25‰ (2SD). The average extension rate is ~12 mm/year for this core. As the Mg/Ca in the Lanyu coral skeletons correlates strongly with SST (R2 = 0.9), it provides a better sensitivity than Sr/Ca for temperature reconstruction.
Trace elements and isotope composition in corals
The coral temporal resolution in this study was approximately 1 month. All data are expressed as 3-month moving averages (Figure 3). The average growth rate is about 12 mm year−1 over the analyzed sections in coral skeletons collected from Lanyu. A comparison of the X-radiography with the instrumental temperature shows that the low- and high-density bands were formed in summer and winter, respectively. Seasonal variation of trace element ratios can be seen in the Lanyu corals for B/Ca, Mg/Ca, Sr/Ca, and U/Ca, based on the correlation between these trace elements and satellite temperature in a 7-year-old long-lived massive coral skeleton (P. lobata) (1991–1997 A.D.). δ18O, δ13C, and δ11B in the coral skeleton also show clear seasonal variability (Figure 6). Note that this plot shows a large magnitude of Mg/Ca variation and apparent upward slopes of Mg/Ca, Sr/Ca, and U/Ca, as well as a decreasing trend in δ13C record. The annual δ11B variation is around 2‰, 23.63‰–25.86‰, similar to recent results obtained from other coral reefs around the world (Pelejero et al., 2005; Liu et al., 2009; Wei et al., 2009).
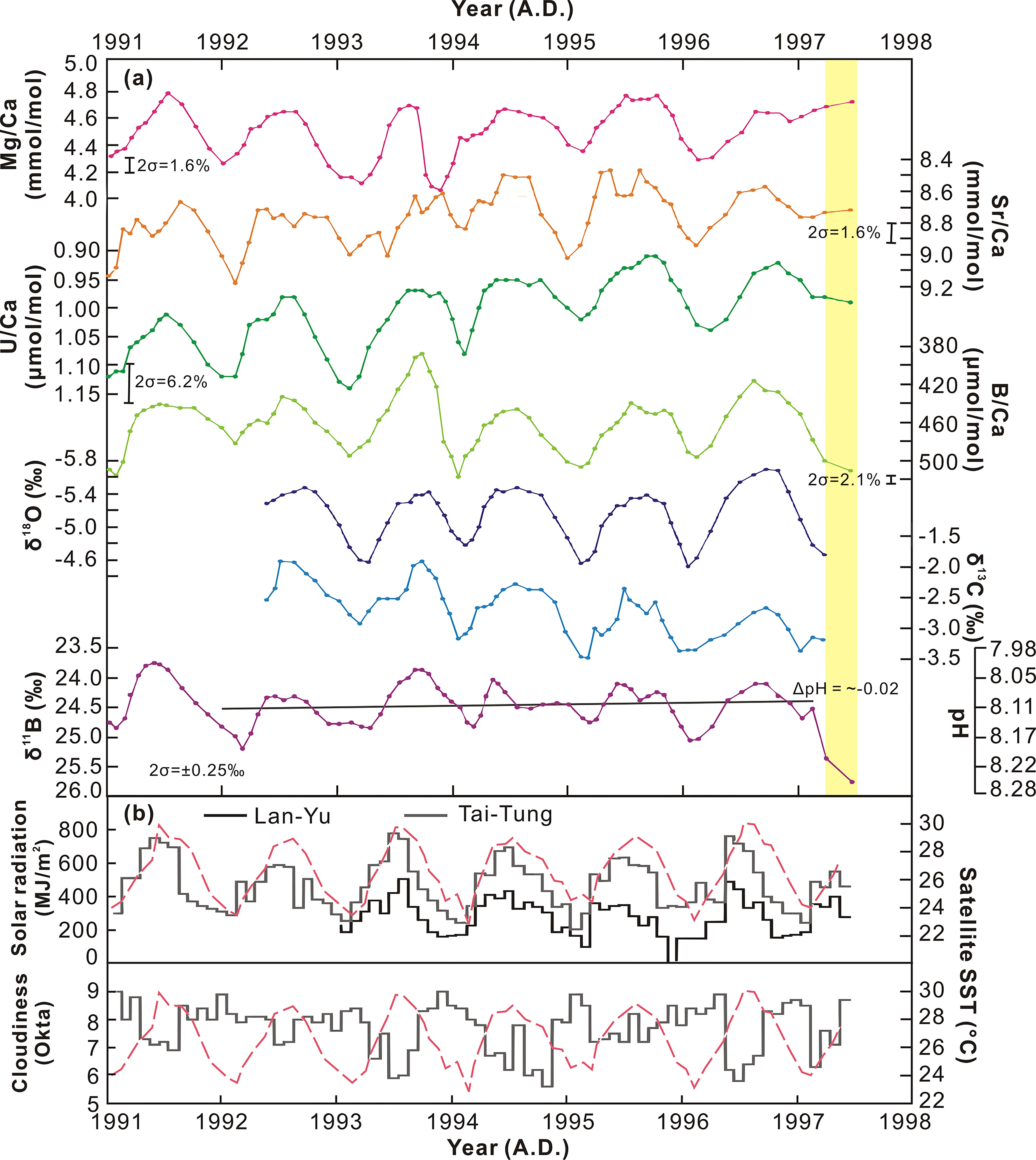
Figure 3 (A) Moving average (~3 months) of the seasonal variation in geochemical proxies (Mg/Ca, Sr/Ca, U/Ca, B/Ca, δ18O, δ13C, and δ11B) of the Lanyu coral records between 1991 and 1997. pH records are estimated from coralline δ11B. Yellow indicates the tissue layer. (B) Data of solar radiation and cloudiness collected from Lanyu and Tai-Tung are plotted with satellite temperature. Solar radiation in Lanyu Island has been recorded since 1993.
Reconstruction of sea surface temperature
Previous studies have reported that B/Ca, Mg/Ca, Sr/Ca, and U/Ca in corals can record SST (Sinclair, 2005). Figure 4 shows the relationships of the trace element ratios versus SST. The correlation coefficients (r) varied in a range between 0.90 and 0.64. The Mg/Ca ratios display the highest correlation with the satellite SST. In terms of temperature sensitivity, Mg/Ca variability is much larger than Sr/Ca, potentially a more reliable proxy for SST in the study region. Hence, the following equation was used to estimate SST variations:
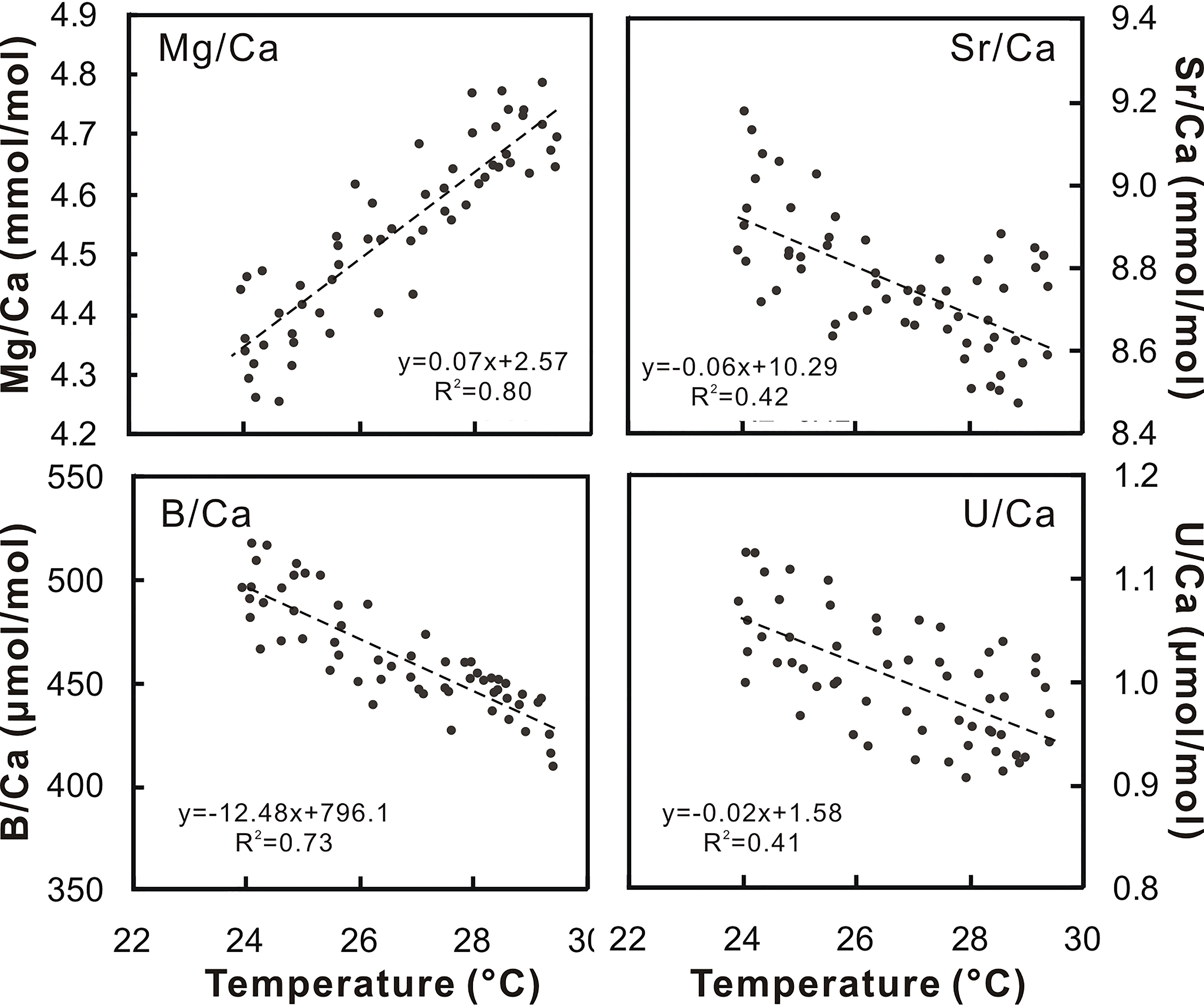
Figure 4 Scatter plots for the trace element ratios and SST. The correlation coefficients for these equations are significant, ranging from 0.90 to 0.64.
The satellite temperatures were plotted against the coralline Mg/Ca (Figure 5). The mean slope was +0.2°C year−1 and ΔMg/Ca-SST showed similar increasing trends, with a slope similar to the satellite temperature change of +0.17°C year−1.
On the other hand, the change of pCO2 in the Lanyu corals was also calculated by estimated TA based on coralline δ18O (equation II) (Tseng et al., 2007) and pH (derived from coralline δ11B) using the CO2sys.xls (version 12) program of Pelletier et al. (2005).
where pCO2 mean = ~354 μatm and Tmean = ~26.41°C
where S is the salinity estimated from δ18Ocoral and δ18OMg/Ca-SST using the listed equations below:
Discussion
Multiproxies approach
Factors controlling oxygen and carbon isotopic compositions
δ18O and δ13C of corals are common tools in environmental proxy studies. The δ18O of corals is mainly controlled by seawater temperature and salinity, whereas δ13C is related to skeletal extension rate, dissolved inorganic carbon, and light availability (Shimamura et al., 2008). In this study, δ13C ranged between −3.9‰ and −1.7‰ and δ18O varied from −5.7‰ to −4.3‰. A clear annual cycle can be seen in both δ18O and δ13C; however, the correlation is out-of-phase (Figure 3).
The Lanyu corals grew at a rate of 12 mm year−1, implying that kinetic isotope effects would be relatively constant due to the high skeletal extension rate, exceeding 5 mm year−1 (Suzuki et al., 2005). The negative relationships (r = −0.73) between δ18O and δ13C are consistent with the previous results by Omata et al. (2005), suggesting that high solar radiation corresponds to higher seawater temperature, resulting in the out-of-phase pattern for δ18O and δ13C in rapidly growing corals. This indicates that the coral skeleton was strongly affected by metabolic effects (photosynthesis) over kinetic effects at Lanyu. Despite the potential metabolic isotope effect playing a main factor to affect δ13C, the possible influence by seawater δ13CDIC was needed for further evaluation. Lin et al. (1999) pointed out that the δ13CDIC in surface seawater was slightly lower during the cold season than the warm season around southern Taiwan. It is possible that the δ13C was affected by seawater δ13C due to vital effect (Pelejero et al., 2005). However, more detailed studies are required to further evaluate the relationship between coralline δ13C and seawater δ13CDIC at decadal timescales.
δ11B in coralline carbonates
Previous studies have suggested that only [B(OH)4−] is incorporated into marine calcium carbonates (Hemming et al., 1995). Therefore, seawater pH values can be estimated from measured δ11B based on an equilibrium constant pKB, and an assumed fractionation factor αB, calculated from theoretical and experimental studies (Kakihana et al., 1977; Klochko et al., 2006). Then pH can be calculated by the following equation:
where δ11BSW = 39.5‰, pKB = 8.6 at 25°C, and αB = 1.0194.
The fractionation factor for boron isotopes ranges from 1.0176 (Sanchez-Valle et al., 2005) to 1.030 (Zeebe, 2005). The uncertainty in the fractionation factor limits the application of 11B for oceanic pH proxy. More recently, αB in seawater has been experimentally determined by Klochko et al. (2006). A value of 1.0272 ± 0.0006 was obtained using a spectrophotometric approach, and this value differs from the more commonly used value of 1.0194 in paleoceanography applications (Kakihana et al., 1977). In this study, αB was 1.0194 used to calculate seawater pH values from coralline δ11B because this value gives a better prediction of the borate isotopic composition in seawater in the study region. This scenario is similar with the observations depicted in McCulloch et al. (2018), where the coralline carbonates fit better with the Kakihana’s fractionation factor and foraminiferal shells or inorganic carbonates agree with the Klochko’s curve. In addition, the estimated pH values using αB = 1.0194 for the Lanyu coral records are consistent with modern instrumental measurement of seawater pH (~8.1), and seasonal variability in seawater pH is similar to modern conditions in the western Pacific (Chou et al., 2007). In the absence of knowledge regarding the physiological mechanisms controlling coralline δ11B, the in-situ calibration between δ11B in coral skeletons and ambient seawater pH needs to be evaluated. Unfortunately, continuous records of seawater pH in the area near Lanyu are lacking at this stage.
Comparison with published δ11B data in marine biogenic carbonates
Based on the close relationships between marine carbonate chemistry and pCO2 concentrations, boron isotopic composition of marine biogenic carbonates is the most important geochemical proxy for reconstructing paleo-pH in the ocean (Hönisch et al., 2007; De'ath et al., 2009). Here, we discuss δ11B values of different marine biogenic carbonates and interpret the boron isotope excursion in the Lanyu corals.
The δ11B in marine carbonates fall within the theoretical curve for the borate species in seawater (Figure 6). This observation has been supported by a number of empirical calibration works on planktonic foraminifers (Sanyal et al., 1996; Sanyal et al., 2001), scleractinian corals (Hemming et al., 1998; Hönisch et al., 2004; Pelejero et al., 2005), and inorganic precipitations (Sanyal et al., 2000) over a pH range of 7.7 to 9.0. Previous research has shown that variation of boron isotopic composition in marine carbonates was controlled by ambient seawater. Nevertheless, the systematic offset from the theoretical curve suggests that potential effects on the boron isotopic composition may have occurred, possibly due to variation in pH in calcification fluids (Hemming et al., 1998; Wei et al., 2009). This indicates that coralline δ11B may not record ambient seawater pH, but these processes only resulted in slight constant offset between corals and seawaters due to direct seawater supply to the calcification site in corals.
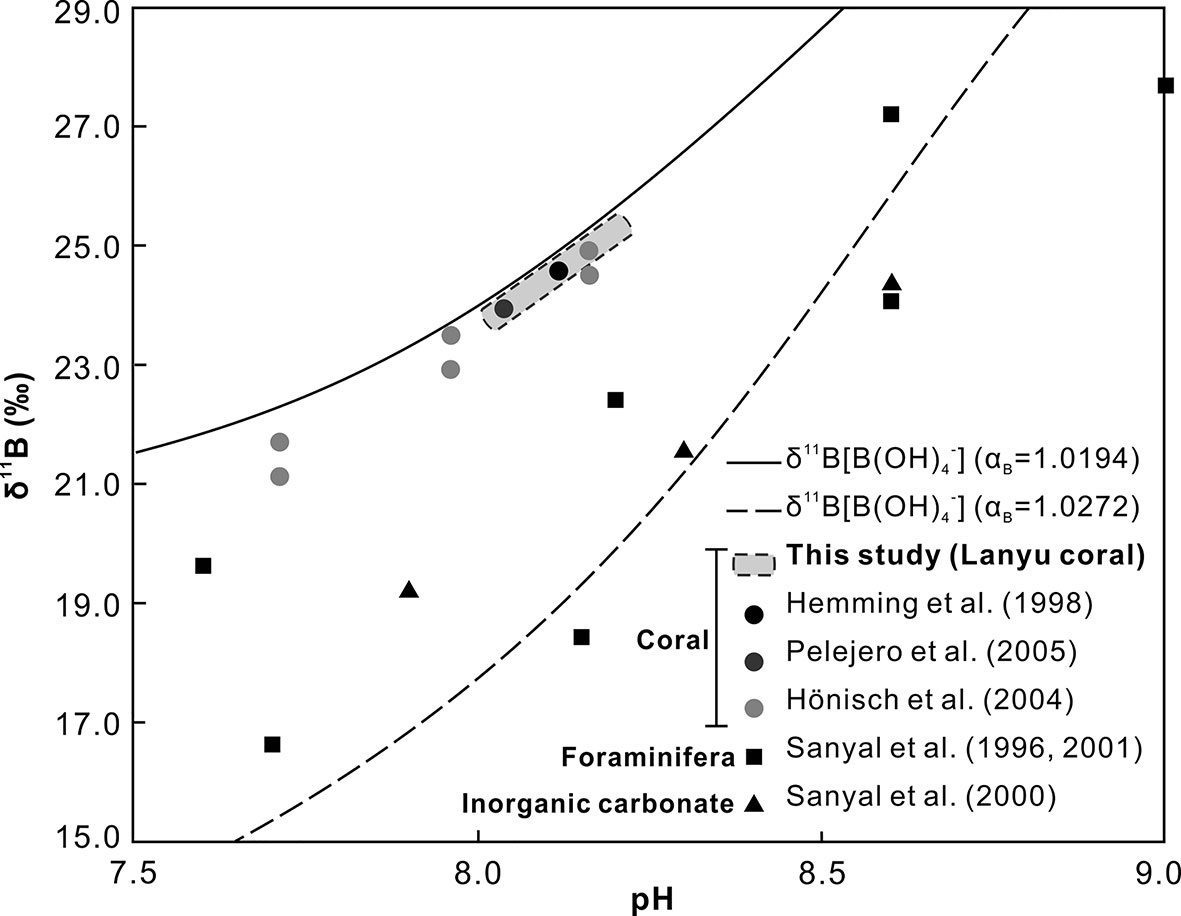
Figure 6 pH dependence of the boron isotopic composition of B(OH)4− based on theoretical αB = 1.0194 (blue) (Kakihana et al., 1977) and empirical αB = 1.0272 ± 0.0006 (red) (Klochko et al., 2006) at 25°C. The δ11B data of scleractinian corals (square), foraminifers (circle), and inorganic calcite precipitation (triangle) are also plotted. Note that most coralline specimens fit better with the Kakihana’s curve, but foraminifers and inorganic calcite precipitation agree more with the Klochko’s.
Controlling factors on coralline δ11B
Although the boron isotope in biogenic marine carbonates has been used for constructing seawater pH in the past, detailed studies about coralline δ11B are scarce, especially for high-resolution seasonal variation in δ11B records (Pelejero et al., 2005). It is necessary to understand the controlling factors on boron uptake and the isotopic fractionation before applying the coralline δ11B to reconstruct the seawater pH in the past.
Hemming et al. (1998) investigated the seasonal variation in the boron isotopic composition in coral skeletons. Paired analyses of carbon and boron isotopes revealed a clear seasonal variation, displaying the high-density bands having heavier δ11B and δ13C in the coral P. lobata from Fanning Island, probably reflecting high productivity during the period of high solar insolation. However, the boron and carbon isotope ratios in our corals did not show a similar correlation. Heavier δ11B together with lighter δ13C is exhibited in the high-density bands which grew in the cold season (Figure 3). Based on the different patterns of the seasonal fluctuation between two study areas, this effect cannot be used to explain the variation in coralline δ11B in Lanyu. Culture experiments with two surface corals, Porites and Acropora, have confirmed that coral skeletons can record the variation of seawater pH (Hönisch et al., 2004; Reynaud et al., 2004). The experimental studies showed that the δ13C in cultured corals can be significantly affected by symbiont photosynthetic activity, which is related to light intensity, feeding, and habitat depth. This result is inconsistent with the δ11B values in the same corals, suggesting that pH variations at the calcification site are rather small relative to the pH-dependent isotopic fractionation. As mentioned above, our coral samples do faithfully record the seawater signal on the seasonal timescale and support the potential use of coral skeleton boron isotopes for reconstructing seawater pH in the past.
High-resolution record of seawater pH around Lanyu Islet
Boron isotopic compositions of the Lanyu corals, ranging from 23.6‰ to 25.1‰, correspond to seawater pH of 8.0–8.2 (Figure 3). These δ11B-derived pH values from the Lanyu corals are identical to the modern surface seawater pH in the western Pacific. There is a clear decreasing δ11B trend of −0.16‰ if the El Niño 1991 data are excluded, and it implies that ocean acidification has occurred in the study area (pH change of −0.02 unit). The δ11B records obtained from the Lanyu corals provide the first detailed and high-resolution record of seawater pH in the region, which display low and high values during warm and cold seasons, respectively. This can be explained by seasonal variation in water masses with different pH values, caused by the monsoon climate (Liu et al., 2009; Wei et al., 2009) or pCO2 variations (Takahashi et al., 2002).
The Kuroshio Current is the most important current around Lanyu Islet. Major water masses at the west side and the east side of 121°E are the South China Sea surface water and Kuroshio Current (KC), respectively. The typical water properties of the two water masses are significantly different. The main water mass close to Lanyu Islet is Kuroshio waters, based on the θ–S plot (Chen et al., 2007). Chou et al. (2007) studied the carbon chemistry of Kuroshio waters off SE Taiwan. Seawater from stations off southeast Taiwan has intermediate values between stations at the West Philippine Sea and the SCS. The pH value of the surface seawater around Lanyu Islet is approximately 8.15, significantly higher than the pH at the SCS (pH = 7.96 to 8.10) (Chen et al., 2006).
The seasonal variability in KC can be linked to the East Asia Monsoon (EAM) climate in the study area. The seawater chemistry is affected by changes in the EAM, which causes SCS seawater entering the KC from the loop current around southern Taiwan. Nevertheless, most KC transport is associated with the path contributed by the east flow of the Lanyu (Hsin et al., 2008). The loop current probably carries the low pH seawater from the SCS (pH~8.0) to the main KC (West Philippine Sea water, pH ~ 8.2); thus, the pH value of surface seawater will decrease in the winter due to the greater contribution from the SCS. However, this is inconsistent with the seasonal variation in δ11B-based seawater pH, which is characterized by high pH (high δ11B) during the cold season. A more detailed assessment on the influence of the SCS waters is necessary for understanding the seawater pH variation around Lanyu.
A comparison of oceanic pCO2 in the western Pacific
Another possible reason for the change of seawater pH is seasonal pCO2 variation. Culture experiments on the boron isotopic composition of coral skeletons were carried out at two pCO2 conditions (~440 vs. ~725 ppm) (Reynaud et al., 2004). Their results indicated that seawater pH changes due to pCO2 variations can be recorded in coral skeletons. In comparison with the global sea–air CO2 flux study by Takahashi et al. (2002), low and high pCO2 were found during the cold and warm seasons, respectively, and pCO2 is mainly controlled by the solubility of CO2 and can be related to seawater temperature. For further evidence of the relationship between pCO2 and seawater temperature in tropical western Pacific, the variation of pCO2 caused by temperature effect was estimated using equation I (Takahashi et al., 2002).
The changes of the estimated pCO2 from the Lanyu coral record are plotted in Figure 7, showing the correlation of reconstructed pCO2 record in the study area on the basis of the two estimates derived from SST and TA. Seasonal variability in seawater pCO2, with higher pCO2 at warm seasons (higher SST), can also be found. This observation agrees well with the estimated seawater pH in the Lanyu corals, showing low pH value (or higher pCO2) in the warmer SST. Furthermore, the variation of seawater pH is approximately 0.2 units between the cold and warm seasons, which is similar to the previous studies in the western Pacific (Pelejero et al., 2005). This study suggests that the boron isotopic composition of coral skeletons can be used for studying the relationship between changes in surface seawater chemistry and climate change and further provide important information on ocean acidification and abrupt climate change.
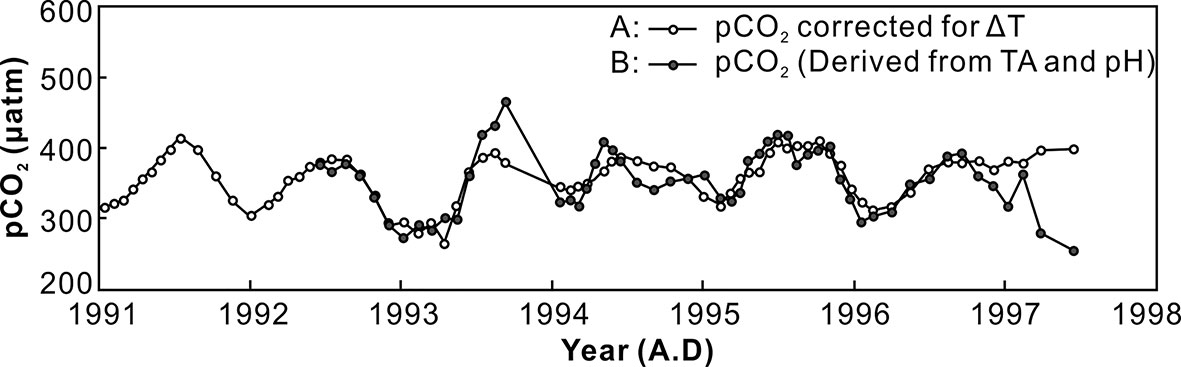
Figure 7 The correlation of reconstructed pCO2 records derived from SST (A) and TA (B) in Lanyu Island using the CO2sys.xls program of Pelletier et al. (2005). The seasonal variation in seawater pCO2 can also be observed, with higher pCO2 in warm seasons (higher SST) in this study.
Data availability statement
The original contributions presented in the study are included in the article/Supplementary Material. Further inquiries can be directed to the corresponding author.
Author contributions
C-FY contributed to the conception and design of the study and wrote the first draft of the manuscript with P-YL. P-YL and C-FY did the field work. C-FY, ZL, and K-FH provided the instrumental resources. P-YL performed the chemical and isotopic measurements. C-FY and P-YL wrote the manuscript. C-HC maintained the instruments. All authors contributed to manuscript revision and read and approved the submitted version.
Acknowledgments
We thank the State Key Laboratory of Marine Geology in Tongji University for analyzing the oxygen and carbon isotopes used in this study. This research was supported by MOST 109-2116-M-006-010 to C-FY.
Conflict of interest
The authors declare that the research was conducted in the absence of any commercial or financial relationships that could be construed as a potential conflict of interest.
Publisher’s note
All claims expressed in this article are solely those of the authors and do not necessarily represent those of their affiliated organizations, or those of the publisher, the editors and the reviewers. Any product that may be evaluated in this article, or claim that may be made by its manufacturer, is not guaranteed or endorsed by the publisher.
Supplementary material
The Supplementary Material for this article can be found online at: https://www.frontiersin.org/articles/10.3389/fmars.2022.877810/full#supplementary-material
Supplementary Figure 1 | Matrix effects in the microsublimation technique. Graphs illustrate (A) the recovery and (B) isotopic fractionation of boron at different concentration.
Supplementary Figure 2 | Average precision of δ11B measured by MC-ICP-MS (2σ = ± 0.25 ‰) in the IGL laboratory.
Supplementary Figure 3 | Cross-calibration of the δ11B of different inorganic carbonates, KTP-1, JA-A and JA-B, measured by MC-ICP-MS and P-TIMS
References
Aggarwal J., Böhm F., Foster G., Halas S., Hönisch B., Jiang S.-Y., et al. (2009). How well do non-traditional stable isotope results compare between different laboratories: results from the interlaboratory comparison of boron isotope measurements. J. Anal. At. Spectrom. 24, 825–831. doi: 10.1039/b815240c
Aggarwal J. K., Sheppard D., Mezger K., Pernicka E. (2003). Precise and accurate determination of boron isotope ratios by multiple collector ICP-MS: origin of boron in the ngawha geothermal system, new Zealand. Chem. Geol. 199, 331–342. doi: 10.1016/S0009-2541(03)00127-X
Al-Ammar A., Gupta R. K., Barnes R. M. (1999). Elimination of boron memory effect in inductively coupled plasma-mass spectrometry by addition of ammonia. Spectrochim. Acta Part B At. Spectrosc. 54, 1077–1084. doi: 10.1016/S0584-8547(99)00045-2
Allison N., Finch A. A. (2010). δ11Sr, Mg and B in a modern Porites coral: the relationship between calcification site pH and skeletal chemistry. Geocim. Cosmochim. Acta 74, 1790–1800. doi: 10.1016/j.gca.2009.12.030
Caldeira K., Wickett M. E. (2003). Oceanography: Anthropogenic carbon and ocean pH. Nature 425, 365. doi: 10.1038/425365a
Charles C. D., Hunter D. E., Fairbanks R. G. (1997). Interaction between the ENSO and the Asian monsoon in a coral record of tropical climate. Science 277, 925–928. doi: 10.1126/science.277.5328.925
Chen Y. L., Chen H. Y., Lin I. I., Lee M. A., Chang J. (2007). Effects of cold eddy on phytoplankton production and assemblages in Luzon strait bordering the south China Sea. J. Oceanogr. 63, 671–683. doi: 10.1007/s10872-007-0059-9
Chen C. T. A., Wang S. L., Chou W. C., Sheu D. D. (2006). Carbonate chemistry and projected future changes in pH and CaCO3 saturation state of the south China Sea. Mar. Chem. 101, 277–305. doi: 10.1016/j.marchem.2006.01.007
Chou W. C., Sheu D. D., Chen C., Wen L. S., Yang Y., Wei C. L. (2007). Transport of the south China Sea subsurface water outflow and its influence on carbon chemistry of kuroshio waters off southeastern Taiwan. J. Geophys. Res. Oceans 12008, 112. doi: 10.1029/2007JC004087
Cohen A. L., McConnaughey T. A. (2003). Geochemical perspectives on coral mineralization. Rev. Mineral. Geochem. 54, 151–187. doi: 10.2113/0540151
Comeau S., Cornwall C. E., DeCarlo T. M., Krieger E., McCulloch M. T. (2018). Similar controls on calcification under ocean acidification across unrelated coral reef taxa. Glob. Change Biol. 24, 4857–4868. doi: 10.1111/gcb.14379
Cornwall C. E., Comeau S., McCulloch M. T. (2017). Coralline algae elevate pH at the site of calcification under ocean acidification. Glob. Change Biol. 23, 4245–4256. doi: 10.1111/gcb.13673
Corre`ge T. (2006). Sea Surface temperature and salinity recon-struction from coral geochemical tracers. Palaeogeogr. Palaeoclimatol. Palaeoecol. 232, 408–428. doi: 10.1016/j.palaeo.2005.10.014
De'ath G., Lough J. M., Fabricius K. E. (2009). Declining coral calcification on the great barrier reef. Science 323, 116–119. doi: 10.1126/science.1165283
Foster G. L. (2008). Seawater pH, pCO2 and [CO2–3] variations in the Caribbean Sea over the last 130 kyr: A boron isotope and B/Ca study of planktic foraminifera. Earth Planet. Sci. Lett. 271, 254–266. doi: 10.1016/j.epsl.2008.04.015
Gaillardet J., Allègre C. J. (1995). Boron isotopic compositions of corals: Seawater or diagenesis record? Earth Planet. Sci. Lett. 136, 665–676. doi: 10.1016/0012-821X(95)00180-K
Gaillardet J., Lemarchand D., Göpel C., Manhès G. (2001). Evaporation and sublimation of boric acid: Application for boron purification from organic rich solutions. Geostand. Newsl. 25, 67–75. doi: 10.1111/j.1751-908X.2001.tb00788.x
Guerrot C., Millot R., Robert M., Négrel P. (2011). Accurate and high-precision determination of boron isotopic ratios at low concentration by MC-ICP-MS (Neptune). Geostand. Geoanal. Res. 35, 275–284. doi: 10.1111/j.1751-908X.2010.00073.x
Gutjahr M., Bordier L., Douville E., Farmer J., Foster G. L., Hathorne, et al. (2021). Sub-Permil interlaboratory consistency for solution-based boron isotope analyses on marine carbonates. Geostand. Newsl. 45, 59–75. doi: 10.1111/ggr.12364
Hemming N. G., Guilderson T. P., Fairbanks R. G. (1998). Seasonal variations in the boron isotopic composition of coral: A productivity signal? Global Biogeochem. Cycles 12, 581–586. doi: 10.1029/98GB02337
Hemming N. G., Hanson G. N. (1992). Boron isotopic composition and concentration in modern marine carbonates. Geocim. Cosmochim. Acta 56, 537–543. doi: 10.1016/0016-7037(92)90151-8
Hemming N. G., Reeder R. J., Hanson G. N. (1995). Mineral-fluid partitioning and isotopic fractionation of boron in synthetic calcium carbonate. Geocim. Cosmochim. Acta 59, 371–379. doi: 10.1016/0016-7037(95)00288-B
Hoegh-Guldberg O., Mumby P. J., Hooten A. J., Steneck R. S., Greenfield P., Gomez E., et al. (2007). Coral reefs under rapid climate change and ocean acidification. Science 318, 1737–1742. doi: 10.1126/science.1152509
Hönisch B., Hemming N. G. (2005). Surface ocean pH response to variations in pCO2 through two full glacial cycles. Earth Planet. Sci. Lett. 236, 305–314. doi: 10.1016/j.epsl.2005.04.027
Hönisch B., Hemming N. G., Grottoli A. G., Amat A., Hanson G. N., Bijma J. (2004). Assessing scleractinian corals as recorders for paleo-pH: Empirical calibration and vital effects. Geocim. Cosmochim. Acta 68, 3675–3685. doi: 10.1016/j.gca.2004.03.002
Hönisch B., Hemming N. G., Loose B. (2007). Comment on “A critical evaluation of the boron isotope-pH proxy: The accuracy of ancient ocean pH estimates” by m. pagani, d. lemarchand, a. spivack and j. gaillardet. Geocim. Cosmochim. Acta 71, 1636–1641. doi: 10.1016/j.gca.2006.07.045
Hsin Y. C., Wu C. R., Shaw P. T. (2008). Spatial and temporal variations of the kuroshio east of Taiwan 1982–2005: A numerical study. J. Geophys. Res. Oceans 113, C04002. doi: 10.1029/2007JC004485
IPCC (2014). Climate change 2014: Synthesis report. contribution of working groups I, II and III to the fifth assessment report of the intergovernmental panel on climate change [Core writing team. Eds. Pachauri R. K., Meyer L. A. (Geneva, Switzerland: IPCC), 151 pp.
Kakihana H., Kotaka M., Satoh S., Nomura M., Okamoto M. (1977). Fundamental studies on the ion-exchange separation of boron isotopes. Bull. Chem. Soc Jpn 50, 158–163. doi: 10.1246/bcsj.50.158
Kasemann S. A., Schmidt D. N., Bijma J., Foster G. L. (2009). In situ boron isotope analysis in marine carbonates and its application for foraminifera and palaeo-pH. Chem. Geol. 260, 138–147. doi: 10.1016/j.chemgeo.2008.12.015
Klochko K., Kaufman A. J., Yao W., Byrne R. H., Tossell J. A. (2006). Experimental measurement of boron isotope fractionation in seawater. Earth Planet. Sci. Lett. 248, 276–285. doi: 10.1016/j.epsl.2006.05.034
Lemarchand D., Gaillardet J., Gopel, Manhès G. (2002). An optimized procedure for boron separation and mass spectrometry analysis for river samples. Chem. Geol 182 (2-4), 323–333. doi: 10.1016/S0009-2541(01)00329-1
Lin H. L., Wang L. W., Wang C. H., Gong G. C. (1999). Vertical distribution of δ13C of dissolved inorganic carbon in the northeastern south China Sea. Deep Sea Res. Part I 46, 757–775. doi: 10.1016/S0967-0637(98)00091-0
Liu Y., Liu W., Peng Z., Xiao Y., Wei G., Sun W., et al. (2009). Instability of seawater pH in the south China Sea during the mid-late Holocene: Evidence from boron isotopic composition of corals. Geocim. Cosmochim. Acta 73, 1264–1272. doi: 10.1016/j.gca.2008.11.034
Liu Y., Peng Z., Zhou R., Song S., Liu W., You C. F., et al. (2014). Acceleration of modern acidification in the south China Sea driven by anthropogenic CO2. Sci. Rep. 4, 5148. doi: 10.1038/srep05148
Louvat P., Bouchez J., Paris G. (2011). MC-ICP-MS isotope measurements with direct injection nebulisation (d-DIHEN): Optimisation and application to boron in seawater and carbonate samples. Geostand. Geoanal. Res. 35, 75–88. doi: 10.1111/j.1751-908X.2010.00057.x
McCulloch M. T., D’Olivo J. P., Falter J., Georgiou P., Holcomb M., Montagna P., et al. (2018). “Chapter 6: Boron isotopic systematics in scleractinian corals and the role of pH up-regulation,” in Advance in isotope geochemistry series: Boron isotopes the fifth element. Eds. Marschall H., Foster G. (Springer International Publishing AG 2018), Cham. 145–162.
McCulloch M. T., D’Olivo J. P., Falter J., Holcomb M., Trotter J. (2017). Coral calcification in a changing world and the interactive dynamics of pH and DIC upregulation. Nat. Commun. 8, 15686. doi: 10.1038/ncomms15686
Moyer R. P., Grottoli A. G. (2011). Coral skeletal carbon isotopes (δ13C and Δ14C) record the delivery of terrestrial carbon to the coastal waters of Puerto Rico. Coral Reefs 30, 791–802. doi: 10.1007/s00338-011-0758-y
Omata T., Suzuki A., Kawahat H., Okamoto M. (2005). Annual fluctuation in the stable carbon isotope ratio of coral skeletons: The relative intensities of kinetic and metabolic isotope effects. Geocim. Cosmochim. Acta 69, 3007–3016. doi: 10.1016/j.gca.2004.12.018
Orr J. C., Fabry V. J., Aumont O., Bopp L., Doney S. C., Feely R. A., et al. (2005). Anthropogenic ocean acidification over the twenty-first century and its impact on calcifying organisms. Nature 437, 681–686. doi: 10.1038/nature04095
Ourbak T., Corrège T., Malaizé B., Le Cornec F., Charlier K., Peypouquet J (2006). A high-resolution investigation of temperature, salinity, and upwelling activity proxies in corals. P. Geochem. Geophys. Geosyst. 7, Q03013. doi: 1029/2005GC001064
Pachauri R. K. (2008). “Climate change 2007. synthesis report,” in Contribution of working groups I, II and III to the fourth assessment report (Switzerland: IPCC, Geneva (Switzerland).
Pearson P. N., Palmer M. R. (2000). Atmospheric carbon dioxide concentrations over the past 60 million years. Nature 406, 695–699. doi: 10.1038/35021000
Pelejero C., Calvo E., McCulloch M. T., Marshall J. F., Gagan M. K., Lough J. M., et al. (2005). Preindustrial to modern interdecadal variability in coral reef pH. Science 309, 2204–2207. doi: 10.1126/science.1113692
Pelletier G., Lewis E., Wallace D. (2005). A calculator for the CO2 system in seawater for Microsoft Excel/VBA (Brookhaven National Laboratory, Upton, NY: Washington State Department of Ecology, Olympia, WA).
Quinn T. M., Sampson D. E. (2002). A multiproxy approach to reconstructing sea surface conditions using coral skeleton geochemistry. Paleoceanography 17, 1062. doi: 10.1029/2000PA000528
Reuer M. K., Boyle E. A., Cole J. E. (2003). A mid-twentieth century reduction in tropical upwelling inferred from coralline trace element proxies. Earth Planet. Sci. Lett. 210, 437–452. doi: 10.1016/S0012-821X(03)00162-6
Reynaud S., Hemming N. G., Juillet-Leclerc A., Gattuso J. P. (2004). Effect of pCO2 and temperature on the boron isotopic composition of the zooxanthellate coral acropora sp. Coral Reefs 23, 539–546. doi: 10.1007/s00338-004-0399-5
Ries J.B., Cohen A. L., McCorkle D. C. (2009). Marine calcifiers exhibit mixed responses to CO2-induced ocean acidification. Geology 37, 1131–1134. doi: 10.1130/G30210A.1
Sanchez-Valle C., Reynard B., Daniel I., Lecuyer C., Martinez I., Chervin J. C. (2005). Boron isotopic fractionation between minerals and fluids: New insights from in situ high pressure-high temperature vibrational spectroscopic data. Geocim. Cosmochim. Acta 69, 4301–4313. doi: 10.1016/j.gca.2005.03.054
Sanyal A., Bijma J., Spero H., Lea D. W. (2001). Empirical relationship between pH and the boron isotopic composition of globigerinoides sacculifer: Implications for the boron isotope paleo-pH proxy. Paleoceanography 16, 515–519. doi: 10.1029/2000PA000547
Sanyal A., Hemming N. G., Broecker W. S., Lea D. W., Spero H. J., Hanson G. N. (1996). Oceanic pH control on the boron isotopic composition of foraminifera: Evidence from culture experiments. Paleoceanography 11, 513–517. doi: 10.1029/96PA01858
Sanyal A., Nugent M., Reeder R. J., Bijma J. (2000). Seawater pH control on the boron isotopic composition of calcite: evidence from inorganic calcite precipitation experiments. Geocim. Cosmochim. Acta 64, 1551–1555. doi: 10.1016/S0016-7037(99)00437-8
Shen C. C., Lee T., Liu K. K., Hsu H. H., Edwards R. L., Wang C. H., et al. (2005). An evaluation of quantitative reconstruction of past precipitation records using coral skeletal Sr/Ca and δ18O data. Earth Planet. Sci. Lett. 237, 370–386. doi: 10.1016/j.epsl.2005.06.042
Shimamura M., Irino T., Oba T., Xu G., Lu B., Wang L., et al. (2008). Main controlling factors of coral skeletal carbon isotopic composition and skeletal extension rate: High-resolution study at hainan island, south China Sea. Geochem. Geophys. Geosyst. 9, Q04024. doi: 10.1029/2007GC001789
Shinjo R., Asami R., Huang K. F., You C. F., Iryu Y. (2013). Ocean acidification trend in the tropical north pacific since the mid-20th century reconstructed from a coral archive. Mar. Geol. 342, 58–64. doi: 10.1016/j.margeo.2013.06.002
Sinclair D. J. (2005). Correlated trace element “vital effects” in tropical corals: A new geochemical tool for probing biomineralization. Geocim. Cosmochim. Acta 69, 3265–3284. doi: 10.1016/j.gca.2005.02.030
Spivack A. J., Edmond J. M. (1986). Determination of boron isotope ratios by thermal ionization mass spectrometry of the dicesium metaborate cation. Anal. Chem. 58, 31–35. doi: 10.1021/ac00292a010
Spivack A. J., You C. F., Smith H. J. (1993). Foraminiferal boron isotope ratios as a proxy for surface ocean pH over the past 21 myr. Nature 363, 149–151. doi: 10.1038/363149a0
Stewart J. A., Day R. D., Christopher S. J., Kucklick J. R., Bordier L., Chalk T. B., et al. (2021). New carbonate reference material for boron isotope and trace element geochemistry: NIST RM 8301 boron isotopes in marine carbonate (Simulated coral and foraminifera solutions) inter-laboratory comparison exercise. Geostand. Geoanal. Res. 45, 77–96. doi: 10.1111/ggr12364
Suzuki A., Hibino K., Iwase A., Kawahata H. (2005). Intercolony variability of skeletal oxygen and carbon isotope signatures of cultured porites corals: Temperature-controlled experiments. Geocim. Cosmochim. Acta 69, 4453–4462. doi: 10.1016/j.gca.2005.05.018
Takahashi T., Sutherland S. C., Sweeney C., Poisson A., Metzl N., Tilbrook B., et al. (2002). Global sea–air CO2 flux based on climatological surface ocean pCO2, and seasonal biological and temperature effects. Deep Sea Res. Part II 49, 1601–1622. doi: 10.1016/S0967-0645(02)00003-6
Takeshita Y., Cyronak T., Martz T. R., Kindeberg T., Andersson A. J. (2018). Coral reef carbonate chemistry variability at different functional scales. Front. Mar. Sci. 5, 175. doi: 10.3389/fmars.2018.00175
Trotter J., Montagna P., McCulloch M., Silenzi S., Reynaud S., Mortimer G., et al. (2011). Quantifying the pH ‘vital effect’ in the temperate zooxanthellate coral cladocora caespitosa: Validation of the boron seawater pH proxy. Earth Planet. Sci. Lett. 303, 163–173. doi: 10.1016/j.epsl.2011.01.030
Tseng C. M., Wong G. T. F., Chou W. C., Lee B. S., Sheu D. D., Liu K. K. (2007). Temporal variations in the carbonate system in the upper layer at the SEATS station. Deep Sea Res. Part II 54, 1448–1468. doi: 10.1016/j.dsr2.2007.05.003
Venn A. V., Tambutte E., Holcomb M., Laurent J., Allemand D. (2013). Impacts of seawater acidification on pH at the tissue-skeleton interface. PNAS 110, 1634–1639. doi: 10.1073/pnas.1216153110
Wang T. H., You C. F., Chung C. H., Liu H. C., Lin Y. P. (2020). Macro-sublimation: purification of boron in low-concentration geological samples for isotopic determination by MC-ICPMS. Microchem J. 152, 104424. doi: 10.1016/j.microc.2019.104424
Wang B. S., You C. F., Huang K. F., Wu S. F., Aggarwal S. K., Chung C. H., et al. (2010). Direct separation of boron from Na- and Ca-rich matrices by sublimation for stable isotope measurement by MC-ICP-MS. Talanta 82, 1378–1384. doi: 10.1016/j.talanta.2010.07.010
Wei G., McCulloch M. T., Mortimer G., Deng W., Xie L. (2009). Evidence for ocean acidification in the great barrier reef of Australia. Geocim. Cosmochim. Acta 73, 2332–2346. doi: 10.1016/j.gca.2009.02.009
Wei G., Sun M., Li X., Nie B. (2000). Mg/Ca, Sr/Ca and U/Ca ratios of a porites coral from sanya bay, hainan island, south China Sea and their relationships to sea surface temperature. Palaeogeogr. Palaeoclimatol. Palaeoecol. 162, 59–74. doi: 10.1016/S0031-0182(00)00105-X
Yu T. L., Wang B. S., You C. F., Burr G. S., Chung C. H., Chen Y. G. (2015). Geochemical effects of biomass burning and land degradation on lanyu islet, Taiwan, limnol. Oceanogr. 60, 411–418. doi: 10.1002/lno.10039
Keywords: boron isotope, coral, pCO2, Lanyu, ocean acidification
Citation: You C-F, Lin P-Y, Huang K-F, Chung C-H and Liu Z (2022) Ocean acidification in the Western Pacific: Boron isotopic composition recorded in a tropical massive coral core from Lanyu Islet SE Taiwan. Front. Mar. Sci. 9:877810. doi: 10.3389/fmars.2022.877810
Received: 17 February 2022; Accepted: 01 July 2022;
Published: 27 July 2022.
Edited by:
Michele Giani, Istituto Nazionale di Oceanografia e di Geofisica Sperimentale, ItalyReviewed by:
Xiubao Li, Hainan University, ChinaSambuddha Misra, Indian Institute of Science (IISc), India
Copyright © 2022 You, Lin, Huang, Chung and Liu. This is an open-access article distributed under the terms of the Creative Commons Attribution License (CC BY). The use, distribution or reproduction in other forums is permitted, provided the original author(s) and the copyright owner(s) are credited and that the original publication in this journal is cited, in accordance with accepted academic practice. No use, distribution or reproduction is permitted which does not comply with these terms.
*Correspondence: Chen-Feng You, Y2Z5MjBAbWFpbC5uY2t1LmVkdS50dw==