- 1Institute for Chemistry and Biology of the Marine Environment, University of Oldenburg, Oldenburg, Germany
- 2Marine Biology Section, Department of Biology, University of Copenhagen, Helsingør, Denmark
- 3Department of Marine, Earth and Atmospheric Sciences, North Carolina State University, Raleigh, NC, United States
B-vitamins are essential micronutrients for marine plankton. Additionally, we now know many marine plankton cannot synthesize B-vitamins de novo (from scratch) and thus are reliant on external supplies. Details of B-vitamin exchange, whether ‘active’ or ‘passive’ (i.e. through cell secretion or mortality), are lacking and as a result we struggle to predict microbial physiology, community composition and biogeochemistry. We argue that significant advances in understanding of the impact of B-vitamin exchange and cycling on marine community structure and biogeochemistry can be made by focusing on unknowns related to the ‘in’s and out’s’ of B-vitamin transport, exchange between plankton, and ecosystem scale processing/transformation of B-vitamins. We point out that it is particularly necessary to reach beyond traditional categorization of populations as B-vitamin auxotrophs (requiring supplied vitamin) or prototrophs (de novo vitamin synthesizers) and begin addressing which populations are net ‘providers’ and/or ‘consumers’. This is a particularly interesting problem as organisms cannot be confidently categorized as net ‘providers’ and/or ‘consumers’ based on genome-based prediction, and it is possible the two roles may change over time and environmental conditions. We posit that greater knowledge of B-vitamin exchange, e.g. cross-feeding, acquisition and secretion systems, environmental triggers of ‘provision’ and ‘consumption’, will reveal unforeseen networking and novel niches across marine planktonic communities. Last, we advocate for further experiments tracking the responses of isolates or natural communities relative to vitamin availability, tracing flow of B-vitamins between cells using novel approaches (e.g. isotopic, fluorometric), and greater consideration of altered B-vitamin exchange and cycling under future climate scenarios.
Introduction
Exchange, cycling, and availability of B-vitamins in the ocean are poorly understood despite their necessity for marine life. In brief, B-vitamins are required in minute amounts (e.g. 10-1000’s of molecules) by cells and function as enzyme cofactors, riboswitch ligands, and/or antioxidants (Lukienko et al., 2000; Miranda-Ríos et al., 2001; Frank et al., 2007). Early cultivation experiments revealed B-vitamin auxotrophic (those unable to synthesize a vitamin de novo) and prototrophic (cells capable of de novo B-vitamin synthesis) marine plankton (Provasoli and Pintner, 1953; Burkholder, 1963; Provasoli and Carlucci, 1974). The development of molecular methods and continued cultivation efforts have revealed more about the steps in B-vitamin biosynthesis, salvage of vitamins from simpler fragments, and potential sources and sinks [see reviews by (Croft et al., 2006; Jurgenson et al., 2009; Gray and Escalante-Semerena, 2010; Butzin et al., 2013; Sañudo-Wilhelmy et al., 2014; Helliwell, 2017)]. After a half-century lull, interest in B-vitamins has revitalized largely following the recognition that prevalent and/or biochemically influential marine plankton are auxotrophic for one or more B-vitamins (Tang et al., 2010; Carini et al., 2014; Gutowska et al., 2017; Helliwell, 2017; Gómez-Consarnau et al., 2018; Paerl et al., 2018b) and the development of chemical methods to measure B-vitamins from seawater (Sañudo-Wilhelmy, 2012; Heal, 2014; Suffridge et al., 2017). Several new unknowns emerge from these recent findings and working to address them is expected to increase our ability to predict plankton composition, productivity, and plankton-driven cycling of elements (e.g. C, N, S) with climate and biological impact. Specifically, there are key knowledge gaps in the areas of: 1) cellular acquisition and provision; 2) microbe-microbe interactions; and 3) marine communities and ecosystem(s) that merit immediate attention. Soil and gut-microbiome related B-vitamin research (e.g. microbial vitamin salvage or vitamin remodeling) is ahead of oceanographic research and can provide perspectives on B-vitamin exchange and cycling potentially occurring in the ocean (Jenkins et al., 2007; Yi et al., 2012; Costliow and Degnan, 2017; Sharma et al., 2019; Sokolovskaya et al., 2020). We see B-vitamin exchange and cycling in the sea as ripe for exploration and offer several perspectives for filling near-term and future needs in this research area.
1. Investigating the “In’s and Out’s” of Transport at the Cellular Level
Most marine plankton are auxotrophic for one or more B-vitamins (Gómez-Consarnau et al., 2018; Paerl et al., 2018b) and thus rely on exogenous B-vitamins or vitamers (related compounds, e.g. precursors derived from de novo biosynthesis or degradation) to survive. Notably, some prototrophic plankton and those not requiring certain B-vitamins can also use exogenous B-vitamins/vitamers in seawater (Droop, 1968; Agarwal et al., 2019). These users of exogenous B-vitamins/vitamers are considered ‘consumers’ in the ocean. Accordingly, uptake transporters (importers) that enable B-vitamin/vitamer acquisition, and exporters that flux B-vitamin/vitamer to the public pool in seawater, influence B-vitamin/vitamer availability for diverse auxotrophic and prototrophic plankton that mediate elemental/energetic cycling and food web structure in the ocean.
Several importers are known based on experimentation with non-marine model microbes or are proposed based on proximity of transporter coding genes to B-vitamin biosynthesis genes or riboswitches in prokaryotes and eukaryotes (Gutiérrez-Preciado et al., 2015; Rodionova et al., 2015; Anderson et al., 2016; Rodionov et al., 2019). Nonetheless, key knowledge gaps remain. For example, most importer functionality is predicted based on comparative genomics (Carini et al., 2014; McRose et al., 2014; Gómez-Consarnau et al., 2018; Paerl et al., 2018b), leaving a current need for more direct experiments that clarify functionality (e.g. substrate binding affinity, uptake kinetics) of importers (Gray and Escalante-Semerena, 2010; Rodionova et al., 2015; Anderson et al., 2016; Rosnow et al., 2018). This is especially the case given that some importers may aid the uptake of vitamers or other nutrients, e.g. TonB-dependent transport systems facilitate uptake of B12 (cobalamin) and iron-chelating siderophores (Shultis et al., 2006) potentially intertwining iron and B-vitamin acquisition (Wienhausen et al., unpublished). Further, the number of importers per genome, types of protein subcomponents, and ATP/ion requirements vary across plankton (Vitreschak et al., 2003; Rodionov et al., 2008; Eitinger et al., 2011; Rodionov et al., 2019) and the advantages of different configurations is unclear due to a lack of experimental testing.
Compared to importers, virtually nothing is known about B-vitamin exporters, which is striking considering net ‘provision’ of B-vitamins by plankton – including bacteria and algae - was first described in the mid-1900’s (Burkholder, 1963; Carlucci and Bowes, 1970; Haines and Guillard, 1974b). Some importers are proposed to function as exporters based on their occurrence in prototrophs or their low B-vitamin affinity (Jaehme and Slotboom, 2015; Rodionov et al., 2019). ‘Dual-functioning’ transport systems are a fascinating possibility but would require strict regulation to effectively export or import under appropriate conditions. Fluorescent tagging of B-vitamin/vitamers, as used to recently study importers (Anderson et al., 2016; Rosnow et al., 2018), and experiments with genetically engineered strains coupled with bioassay-based or chemical-based measurements of exported B-vitamins (Carlucci and Silbernagel, 1966; Bonnet et al., 2010) could help identify exporters (also importers) and associated export (also import) of vitamins or vitamers.
We posit that an improved understanding of importer and exporter functionality will elevate genome-based predictions of which plankton are key net ‘providers’ (providers of exogenous B-vitamin/vitamer) or ‘consumers’ (users of exogenous B-vitamin/vitamer), but also involved in widespread exchanges between plankton. Notably, recent isolate experiments highlight that predicting net ‘provision’ or ‘consumption’ is not always possible even with complete genome sequences (Paerl et al., 2017; Shelton et al., 2019; Wienhausen et al. unpublished). For example, co-culture experiments with Escherichia coli K-12 and Vibrio anguillarum PF430-3, two isolates with complete and public genome sequences as well as arguably well-annotated B-vitamin metabolic pathways and transporters, showed exchange of vitamin B1 (thiamin) and a precursor HMP (4-amino-5-hydroxymethyl-2-methylpyrimidine) but not thiazole precursor – and this was genetically unexplainable (Sathe et al., 2022). Experiments with marine plankton and genome surveys similarly highlight the uncertainty in predicting B-vitamin uptake and efflux (Rodionov et al., 2008; McRose et al., 2014; Paerl et al., 2018a; Paerl et al., 2018b).
Overall, unraveling the details of vitamin and vitamer transport holds great promise to aid predictions of marine plankton physiology, metabolism and interactions. It should be a future priority to investigate the functionality of characterized and uncharacterized transporter systems, but also associated cellular responses, e.g. synthesis and salvage pathways, motility, etc., tied to consumption or provision of B-vitamins/vitamers. Experiments with ‘model’ strains with complete genomes as well as synthetic communities are the easiest path forward to obtain information in these areas and ultimately increase our power to predict changes in community composition and activity (Follows and Dutkiewicz, 2010; Casey et al., 2022), and resolving niches and interdependency amongst marine plankton (D’Souza et al., 2018).
2. Disentangling B-Vitamin/Vitamer Exchange Between Microbes
Numerous organic molecules are exchanged ‘actively’ (e.g. exported) or ‘passively’ (e.g. lost via cell lysis) between microbes (D’Souza et al., 2018), including B-vitamins. Exchanges involving vitamins/vitamers and macronutrients are known; for example, bacteria providing B12 to co-occurring algae and in turn receiving organic carbon (Haines and Guillard, 1974b; Croft et al., 2005; Kazamia et al., 2012; Durham et al., 2015).
Culture experiments have revealed the potential for plankton B-vitamin exchange while providing a ‘guiding light’ for investigating cellular level details behind B-vitamin exchange (see above) and interconnectivity between populations (Burkholder, 1963; Haines and Guillard, 1974a; Paerl et al., 2017; Cruz-López et al., 2018; Cooper et al., 2019; Wienhausen et al. unpublished) (Figure 1). Notably, it is unclear if specific vitamin/vitamer(s) are being shared in these experiments. Clarity is needed on whether vitamin or vitamer(s) or both are commonly exchanged and why, especially now that we know vitamers are useful for key marine plankton influencing key biogeochemistry and food webs (Helliwell et al., 2016; Gutowska et al., 2017; Paerl et al., 2018b; Wienhausen et al. in press).
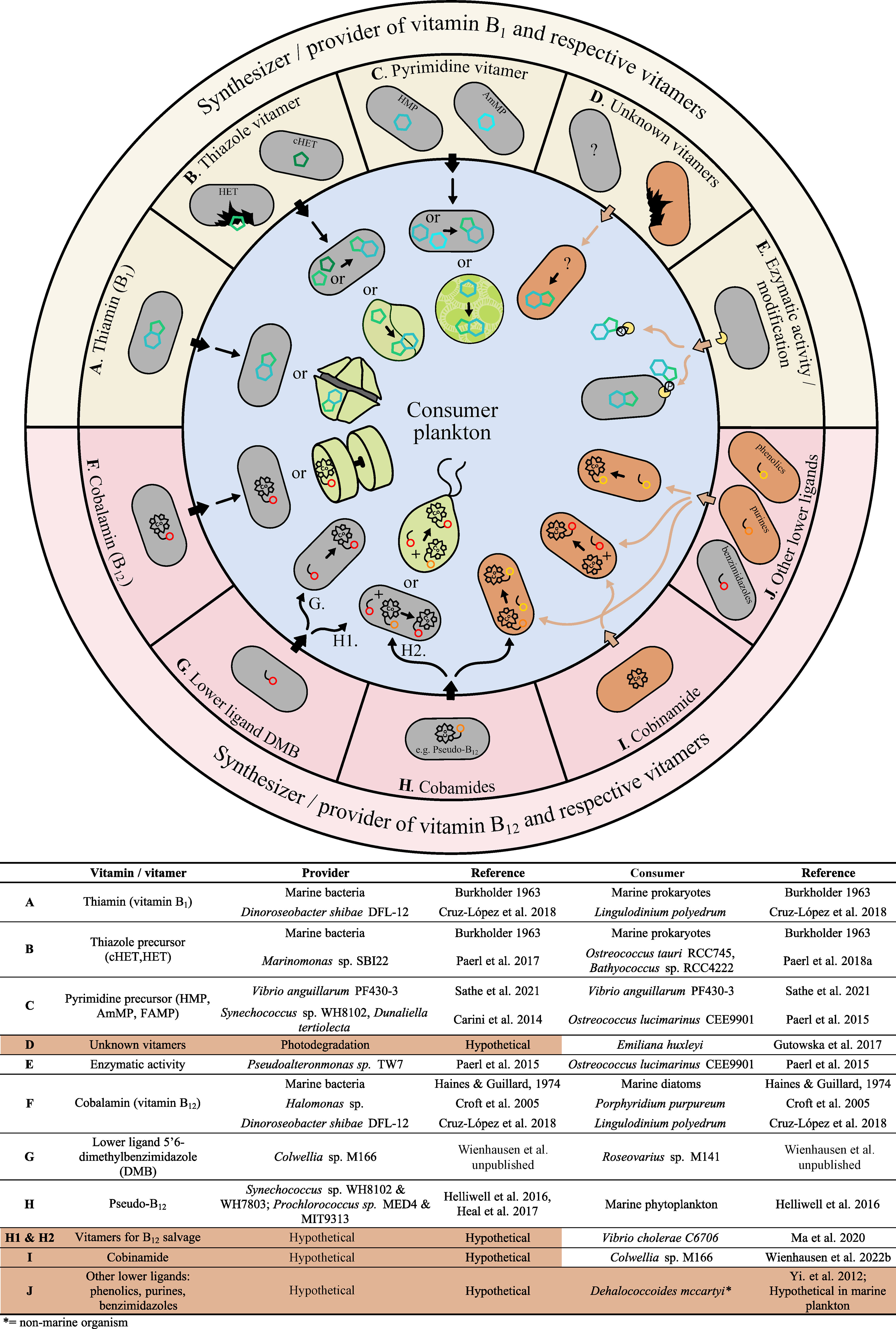
Figure 1 B-vitamin/vitamer exchange amongst marine plankton. The outer ring illustrates prokaryotes that synthesize and provide vitamins or vitamers. Examples of vitamin B1 and respective vitamers (A–E) are shown in the yellow semicircle and examples of vitamin B12 and respective vitamers (F–J) are shown in the red semicircle. The inner blue circle shows prokaryotic and eukaryotic plankton recipients that can use vitamin B1 and respective vitamers (A–E) or vitamin B12 and respective vitamers (F–J). Details on (A–J), including citations and representative organisms displaying each trait, are provided in the bottom grid. Provider and consumer microbial traits that are hypothetical or have not yet been verified in the marine environment are highlighted in orange and noted accordingly.
It is presently difficult to discern what cells are truly net ‘providers’ versus ‘consumers’ of B-vitamin(s) in the ocean. De novo synthesizers, auxotrophs salvaging vitamin from respective vitamers, and microbes modifying organics for other cellular needs (e.g. access to phosphate via alkaline phosphatase) all can be sources of vitamins/vitamers. Consumers are any cell capable of vitamin/vitamer uptake – whether or not they are B-vitamin auxotrophs (Figure 1). Genomic and transcriptomic efforts thus far have mainly focused on bacteria and algae, as well as vitamins B1 and B12, leaving many unknowns about other B-vitamins and the roles archaea and non-photosynthetic eukaryotes play as B-vitamin sources (Croft et al., 2006; Tang et al., 2010; Sañudo-Wilhelmy et al., 2014; Doxey et al., 2015; Poulson-Ellestad et al., 2016). Additionally, vitamin/vitamer ‘provision’ is not well predicted from genetic data (see section 1) (Sathe et al., 2022; Wienhausen et al., unpublished). Furthermore, the dynamic physical, biochemical, and biological variables in the ocean potentially alter B-vitamin exchange and concentrations (Carini et al., 2014; Gómez-Consarnau et al., 2018; Suffridge et al., 2020). Prokaryotes are traditionally considered B-vitamin ‘providers’, especially B12 (Haines and Guillard, 1974b; Provasoli and Carlucci, 1974); however, recent findings indicate they may also be key ‘consumers’ (Koch et al., 2012). To understand this conundrum of microbial function as B-vitamin providers or consumers, we need to further disentangle what exchanges are possible in situ.
Prototrophs are the source of ‘new’, versus salvaged, vitamin to the ocean and thus are logical potential B-vitamin ‘providers’. However, differences in the extent of provision is likely – for example vitamin B1 and pyrimidine precursors, rather than thiazole precursors, are shared among prokaryotes (Sathe et al., 2022) (Figure 1, see B, C). One complexity to ‘provision’ from prototrophs involves cyanobacteria, which are generally prototrophic for B-vitamins but importantly use and produce a specific form of B12, pseudo-B12, which is not bioavailable to most plankton (Figure 1, see H) (Helliwell et al., 2016; Heal et al., 2017). Prokaryotes and eukaryotes can remodel lower ligands of cobamides, a family of cobalt containing cofactors including B12 and pseudo-B12 (Helliwell et al., 2016; Ma et al., 2020). In this respect it is possible that the availability and cycling of lower ligands in the ocean (Figure 1, see H1, H2), e.g. benzimidazoles, purines and phenols, plays a more significant role than previously recognized (Yi et al., 2012; Men et al., 2014). We are only starting to understand how B12-vitamin/vitamers help to fulfill the needs of marine plankton and are involved in microbial exchange and how, for example, prophage-triggered lysis contributes to their release (Wienhausen et al., unplublished).
In the case of B12 in particular, eukaryotes and prokaryotes compete for the same rare, yet pivotal cofactor, with the latter outnumbering the former. To ensure sufficient uptake, some phototrophic eukaryotes can release proteins that effectively bind B12 (Provasoli and Carlucci, 1974; Pintner and Altmeyer, 1979; Bertrand et al., 2012). Protein binding has potentially far-reaching implications for B12 availability and microbial interactions dependent on B12 exchange, yet has received little recent attention. Non-marine studies also describe vitamin B1 binding proteins produced by non-marine microorganisms (Itokawa et al., 1982), thus binding proteins may impact the availability of other B-vitamins in the ocean (Figure 2B).
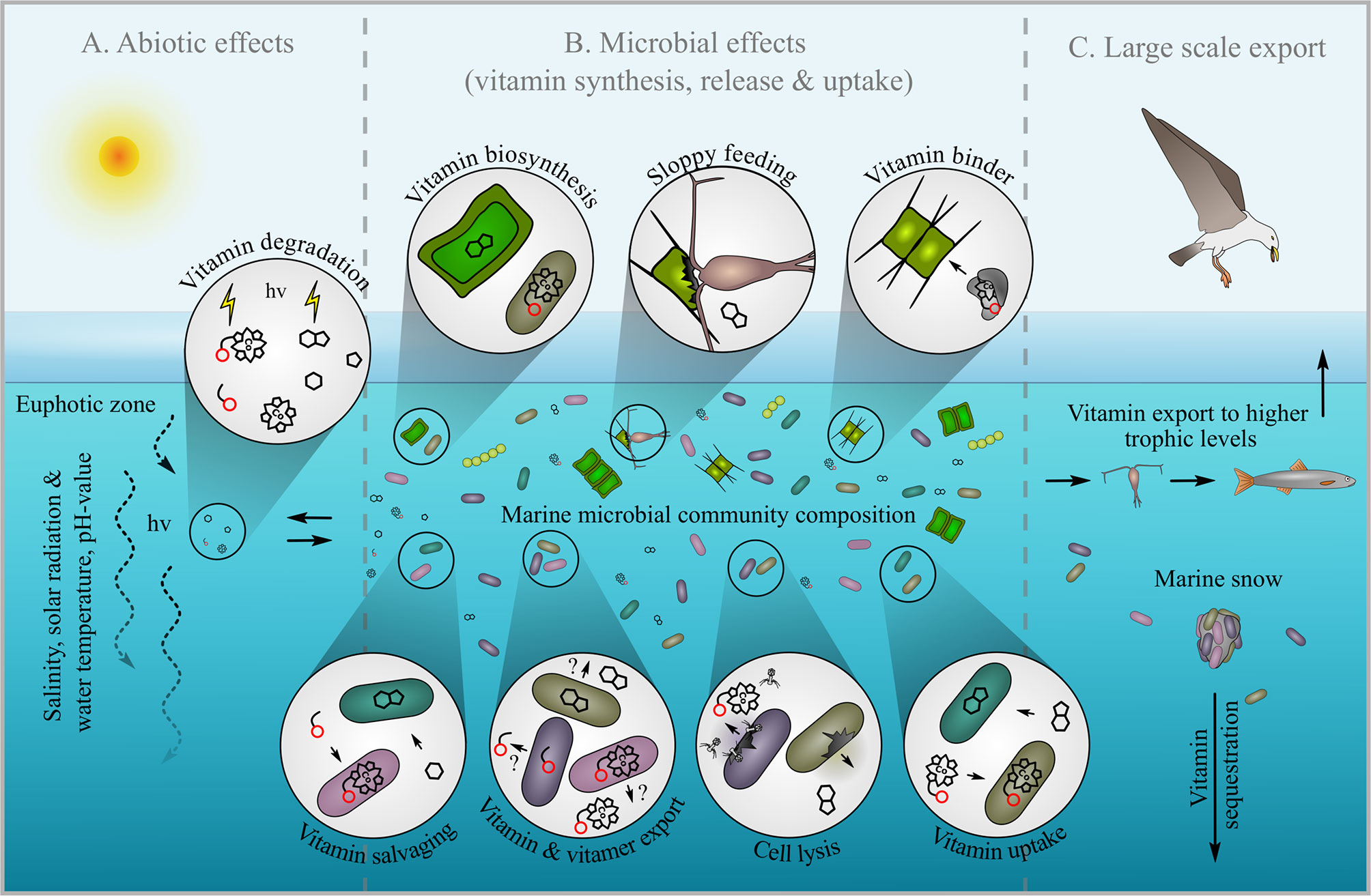
Figure 2 B-vitamin cycle in the pelagic ocean. The left section (A) illustrates abiotic factors that can affect the stability of B-vitamins including salinity, solar radiation, water temperature and pH. Microbial processes describing vitamin synthesis and salvaging as well as vitamin release and uptake are show in the middle section (B). Potential routes of large-scale B-vitamin export are depicted in the right section including transfer up the marine food web to higher trophic levels and sinking of B-vitamin rich marine snow (C).
Extracellular enzyme activity may also factor into recovering B-vitamins/vitamers and microbial exchange (Figure 1E). For example, phosphatase activity from co-occurring bacteria can give select algae access to phosphorylated vitamin B1 (via dephosphorylation), which is not useful for the algae alone (Paerl et al., 2015). Other B-vitamins (e.g. vitamin B6) and vitamers (e.g. HMP), and possibly yet to be identified vitamers are phosphorylated through cellular biosynthesis (Figure 2A) (Jurgenson et al., 2009; Parra et al., 2018); thus, their use and exchange could be similarly impacted by extracellular enzyme activity.
Future research should focus on disentangling vitamin/vitamer exchange in (1) environmentally relevant co-cultures, (2) defined plankton consortia with multiple B-vitamin requirements and (3) experiments considering the impacts of enzymatic activity. A more complete view of exchanges will likely advance understanding of co-evolution in the ocean, from tight-proximity symbioses (Croft et al., 2005; Blifernez-Klassen et al., 2021) or distant plankton exchanges, which are more prevalent in the ocean (Droop, 2007).
3. Marine B-Vitamin Cycling on the Ecosystem Scale
The physical, chemical and biological properties of the ocean are unique compared to other natural systems (e.g. soils, human gut), and both biotic and abiotic factors are anticipated to uniquely impact B-vitamin availability. Abiotic degradation of B-vitamins/vitamers is expected but at what rates? Experimental evidence suggests B-vitamin degradation products have a longer half-life than intact vitamins at least on the basis of light exposure (Carlucci et al., 1969; Gutowska et al., 2017). In non-marine experiments, B-vitamins degrade under elevated temperature, pH, irradiance, and metal concentrations (Dwivedi and Arnold, 1973; Combs and McClung, 2016). Light and temperature are known to degrade B1 and B12 in seawater (Gold, 1968; Carlucci et al., 1969). Intriguing recent evidence indicates some algae may be better adapted to use photodegraded vitamin versus non-degraded vitamin (Gutowska et al., 2017). Alkaline conditions degrade B-vitamins (Jenkins et al., 2007), but in the well-buffered pelagic ocean water, high pH (>10) would have to exist in particle or cell-associated microenvironments. In recent years considerable progress has been made to quantify vitamers (including degradation products) from seawater (Heal, 2014; Suffridge et al., 2017; Longnecker et al., 2018; Suffridge et al., 2020; Wienhausen et al. unpublished), but in several cases it is unclear if abiotic and/or biotic processes were responsible for the presence of vitamers in the ocean. A better understanding of the effects of various abiotic factors, including temperature, acidification or solar radiation on B-vitamin/vitamer stability is needed, especially under climate change scenarios (Figure 2A).
In the pelagic ocean, acquisition of dissolved B-vitamins is vital for microbial function and survival, but the influence of community composition on B-vitamin availability remains unclear. Mesocosm experiments indicate that microbial growth is limited due to B-vitamin deficiency (e.g., B12) in different oceanic provinces (Bertrand et al., 2007; Joglar et al., 2020; Wienhausen et al., in press) and B-vitamin (B1, B7, B12) concentrations in seawater can be below those required for microbial growth (Sañudo-Wilhelmy, 2012; Johnson et al., 2021). Putative microbial ‘providers’ and ‘consumers’ of individual B-vitamins have been identified based on genome-based predictions of auxotrophy/prototrophy (Sañudo-Wilhelmy et al., 2014), but as discussed above, these assumptions must be approached with caution. Greater understanding on these fronts is needed in order to begin classifying the availability of dissolved B-vitamins in different oceanic provinces (Longhurst, 2010; Reygondeau et al., 2018). For example, current knowledge suggests cyanobacteria are a vast source of pseudo-B12 in tropical to subtropical provinces and the fraction of B12 prototrophic bacteria decreases sharply towards the poles (Doxey et al., 2015; Heal et al., 2017; Wienhausen et al., unpublished). In contrast, archaea appear to be significant sources of B12 in the deep ocean (Doxey et al., 2015). There is much left to resolve in this area and some consideration of activity along with biogeography should be taken into account – e.g. transcript abundance as an indicator of metabolic activity (Gómez-Consarnau et al., 2018). Additionally, the amount of vitamins/vitamers synthesized and ‘provided’ per cell (or biomass) is crucial for estimating vitamin availability in the pelagic ocean. Limited estimates of vitamins present or released per cell are available (Bonnet et al., 2010; Carini et al., 2014; Paerl et al., 2015; Gutowska et al., 2017; Suffridge et al., 2017). Obtaining such information would help constrain estimates of B-vitamin exchange as well as change in plankton communities in situ (Teeling et al., 2012; Suffridge et al., 2018).
Beyond cell export, B-vitamins are expected to enter dissolved seawater via cell mortality, especially viral lysis and ‘sloppy feeding’ by grazers (Fuhrman, 1999; Wilhelm and Suttle, 1999; Møller et al., 2003; Poulson-Ellestad et al., 2016). About half of all marine bacteria are lysed by phages, resulting in the release of dissolved organic matter (DOM), including vitamins, via the ‘viral shunt’ (Fuhrman, 1999; Breitbart et al., 2018). Another caveat is that B-vitamin liberated via mortality may be bound to proteins (as in cells) or other organics (Provasoli and Carlucci, 1974; Itokawa et al., 1982) rendering it inaccessible (Figure 2B). How much B-vitamin in seawater is accounted for by viral lysis or grazing is not known, and will require chemical (mass spectrometry) (Suffridge et al., 2017) and biological assessments (bioassays) (Carlucci and Silbernagel, 1966) to fully address.
Zooplankton and ‘mixotrophic’ algae play an additional key role in B-vitamin cycling by directly contributing to transfer of vitamins to higher trophic levels, and this also requires greater exploration. In the case of B1 for example, intracellular concentrations within different zooplankton species can be species-specific, vary seasonally and spatially within organisms of the same genus (Fridolfsson et al., 2018). Differences in B-vitamin availability per biomass within steps of food webs can have dramatic consequences at higher trophic levels, such as birds and fish, where it has been shown that B1 deficiency can severely increase mortality and breeding failure (Balk, 2009; Keinänen et al., 2012). More biomass normalized B-vitamin production data for organisms at different trophic levels will enable better tuning of trophic transfer models and likely improved predictions of ecosystem-wide B-vitamin budgets (Ejsmond et al., 2019). Interestingly, grazing may be impacted by B-vitamin availability (Anderson et al., 2018), thus trophic transfer of vitamins as well as other nutrients may be impacted by vitamin availability. B-vitamin trophic transfer is potentially reduced via export of vitamin-rich particles, e.g. marine snow (Alldredge and Silver, 1988), to the ocean interior. Another point of future exploration is the B-vitamin content of recently formed particles, its importance in supporting free and particle-associated communities and metabolism, especially in the deep sea (Figure 2C).
Future Directions
We recommend culture or ‘bottle’ experiments that allow controlled examination of cell responses to changes in B-vitamin availability or exchange, abiotic degradation experiments, as well as implementing compound tracing (e.g. isotopic, fluorometric) methods and quantification methods (e.g. liquid chromatography mass spectrometry) to fill the key knowledge gaps highlighted above. A last suggestion is that future research view B-vitamin cycling more through a climate change ‘lens’ – especially given that predicted ocean change includes alteration of temperature, irradiance intensity and pH all of which are known to impact the stability of B-vitamins (Combs and McClung, 2016). Overall, numerous exciting discoveries are on the horizon related to marine B-vitamin cycling and are readily achievable with straightforward experimentation.
Author Contributions
GW, MB, and RP all contributed to writing of the perspective. GW constructed illustrations with feedback from MB and RP. All authors contributed to the article and approved the submitted version
Funding
GW acknowledges support by the German Research Foundation within the Transregional Collaborative Research Center Roseobacter (TRR51). MB received funding from the European Union’s Horizon 2020 research and innovation program under the Marie Skłodowska-Curie grant agreement No. 801199 and was supported via the Independent Research Fund Denmark grant No. 9040-00067B to Lasse Riemann and RP. RP acknowledges support from NSF OCE award #2049388.
Conflict of Interest
The authors declare that the research was conducted in the absence of any commercial or financial relationships that could be construed as a potential conflict of interest.
Publisher’s Note
All claims expressed in this article are solely those of the authors and do not necessarily represent those of their affiliated organizations, or those of the publisher, the editors and the reviewers. Any product that may be evaluated in this article, or claim that may be made by its manufacturer, is not guaranteed or endorsed by the publisher.
Acknowledgments
All acknowledge Hans W. Paerl for constructive criticisms on the manuscript and dedicate this perspective to the late Angelo F. Carlucci whose thought-provoking work was instrumental to the advancement of B-vitamin oceanographic research.
References
Agarwal S., Dey S., Ghosh B., Biswas M., Dasgupta J. (2019). Mechanistic Basis of Vitamin B12 and Cobinamide Salvaging by the Vibrio Species. Biochim. Biophys. Acta - Proteins Proteomics 1867, 140–151. doi: 10.1016/j.bbapap.2018.11.004
Alldredge A. L., Silver M. W. (1988). Characteristics, Dynamics and Significance of Marine Snow. Prog. Oceanogr. 20, 41–82. doi: 10.1016/0079-6611(88)90053-5
Anderson R., Charvet S., Hansen P. J. (2018). Mixotrophy in Chlorophytes and Haptophytes-Effect of Irradiance, Macronutrient, Micronutrient and Vitamin Limitation. Front. Microbiol. 9. doi: 10.3389/fmicb.2018.01704
Anderson L. N., Koech P. K., Plymale A. E., Landorf E. V., Konopka A., Collart F. R., et al. (2016). Live Cell Discovery of Microbial Vitamin Transport and Enzyme-Cofactor Interactions. ACS Chem. Biol. 11, 345–354. doi: 10.1021/acschembio.5b00918
Balk L., Hägerroth P-Åke, Åkerman G, Hanson M, Tjärnlund U, Hansson T. (2009). Wild Birds of Declining European Species are Dying From a Thiamine Deficiency Syndrome. Proc. Natl. Acad. Sci. U.S.A. 106, 12001–6. doi: 10.1073/pnas.0902903106
Bertrand E. M., Allen A. E., Dupont C. L., Norden-Krichmar T. M., Bai J., Valas R. E., et al. (2012). Influence of Cobalamin Scarcity on Diatom Molecular Physiology and Identification of a Cobalamin Acquisition Protein. Proc. Natl. Acad. Sci. 109, E1762 LP–E1771. doi: 10.1073/pnas.1201731109
Bertrand E. M., Saito M. A., Rose J. M. (2007). Vitamin B12 and Iron Colimitation of Phytoplankton Growth in the Ross Sea. Limnol. Ocean. 52, 1079–1093. doi: 10.4319/lo.2007.52.3.1079
Blifernez-Klassen O., Klassen V., Wibberg D., Cebeci E., Henke C., Rückert C., et al. (2021). Phytoplankton Consortia as a Blueprint for Mutually Beneficial Eukaryote-Bacteria Ecosystems Based on the Biocoenosis of Botryococcus Consortia. Sci. Rep. 11, 1726. doi: 10.1038/s41598-021-81082-1
Bonnet S., Webb E. A., Panzeca C., Karl D. M., Capone D. G., Wilhelmy S. A. S. (2010). Vitamin B12 Excretion by Cultures of the Marine Cyanobacteria Crocosphaera and Synechococcus. Limnol. Ocean. 5, 1959–1964. doi: 10.4319/lo.2010.55.5.1959
Breitbart M., Bonnain C., Malki K., Sawaya N. A. (2018). Phage Puppet Masters of the Marine Microbial Realm. Nat. Microbiol. 3, 754–766. doi: 10.1038/s41564-018-0166-y
Burkholder P. R. (1963). “Some Nutritional Relationships Among Microbes of Sea Sediments and Water,” in Symposium on Marine Microbiology. Ed. Oppenheimer C. H.. (Springfield, Illinois: CC Thomas Springfield), 133–150.
Butzin N. C., Secinaro M. A., Swithers K. S., Gogarten J. P., Noll K. M. (2013). Thermotoga Lettingae can Salvage Cobinamide to Synthesize Vitamin B12. Appl. Environ. Microbiol. 79, 7006–7012. doi: 10.1128/AEM.01800-13
Carini P., Campbell E. O., Morré J., Sañudo-Wilhelmy S. A., Cameron Thrash J., Bennett S. E., et al. (2014). Discovery of a SAR11 Growth Requirement for Thiamin’s Pyrimidine Precursor and its Distribution in the Sargasso Sea. ISME J. 8, 1727–1738. doi: 10.1038/ismej.2014.61
Carlucci A. F., Bowes P. M. (1970). Vitamin Production and Utlization by Phytoplankton in Mixed Culture. J. Phycol. 6, 393–400. doi: 10.1111/j.1529-8817.1970.tb02413.x
Carlucci A. F., Silbernagel S. B. (1966). Bioassay of Seawater. II. Methods for the Determination of Concentrations of Dissolved Vitamin B1 in Seawater. Can. J. Microbiol. 12, 1079–1089. doi: 10.1139/m66-147
Carlucci A. F., Silbernagel S. B., McNally P. M. (1969). Influence of Temperature and Solar Radiation on Persistence of Vitamin B12, Thiamine, and Biotin in Seawater. J. Phycol. 5, 302–305. doi: 10.1111/j.1529-8817.1969.tb02618.x
Casey J. R., Boiteau R. M., Engqvist M. K. M., Finkel Z. V., Li G., Liefer J., et al. (2022). Basin-Scale Biogeography of Marine Phytoplankton Reflects Cellular-Scale Optimization of Metabolism and Physiology. Sci. Adv. 8, eabl4930–eabl4930. doi: 10.1126/sciadv.abl4930
Combs G. F., McClung J. P. (2016). The Vitamins: Fundamental Aspects in Nutrition and Health (Boston, Massachusetts: Academic press).
Cooper M. B., Kazamia E., Helliwell K. E., Kudahl U. J., Sayer A., Wheeler G. L., et al. (2019). Cross-Exchange of B-Vitamins Underpins a Mutualistic Interaction Between Ostreococcus Tauri and Dinoroseobacter Shibae. ISME J. 13, 334–345. doi: 10.1038/s41396-018-0274-y
Costliow Z. A., Degnan P. H. (2017). Thiamine Acquisition Strategies Impact Metabolism and Competition in the Gut Microbe Bacteroides Thetaiotaomicron. mSystems 2. doi: 10.1128/mSystems.00116-17
Croft M. T., Lawrence A. D., Raux-Deery E., Warren M. J., Smith A. G. (2005). Algae Acquire Vitamin B12 Through a Symbiotic Relationship With Bacteria. Nature 438, 90–93. doi: 10.1038/nature04056
Croft M. T., Warren M. J., Smith A. G. (2006). Algae Need Their Vitamins. Eukaryot. Cell 5, 1175–1183. doi: 10.1128/EC.00097-06
Cruz-López R., Maske H., Yarimizu K., Holland N. A. (2018). The B-Vitamin Mutualism Between the Dinoflagellate Lingulodinium Polyedrum and the Bacterium Dinoroseobacter Shibae. Front. Mar. Sci. 5. doi: 10.3389/fmars.2018.00274
Doxey A. C., Kurtz D. A., Lynch M. D. J., Sauder L. A., Neufeld J. D. (2015). Aquatic Metagenomes Implicate Thaumarchaeota in Global Cobalamin Production. ISME J. 9, 461–471. doi: 10.1038/ismej.2014.142
Droop M. R. (1968). The Kinetics of Uptake Growth and Inhibition in Monochrysis Lutheri. J. Mar. Biol. 48, 686–733. doi: 10.1017/S0025315400019238
Droop M. R. (2007). Vitamins, Phytoplankton and Bacteria: Symbiosis or Scavenging? J. Plankton Res. 29, 107–113. doi: 10.1093/plankt/fbm009
D’Souza G., Shitut S., Preussger D., Yousif G., Waschina S., Kost C. (2018). Ecology and Evolution of Metabolic Cross-Feeding Interactions in Bacteria. Nat. Prod. Rep. 35, 455–488. doi: 10.1039/C8NP00009C
Durham B. P., Sharma S., Luo H., Smith C. B., Amin S. A., Bender S. J., et al. (2015). Cryptic Carbon and Sulfur Cycling Between Surface Ocean Plankton. Proc. Natl. Acad. Sci. U. S. A. 112, 453–457. doi: 10.1073/pnas.1413137112
Dwivedi B. K., Arnold R. G. (1973). Chemistry of Thiamine Degradation in Food Products and Model Systems: A Review. J. Agric. Food Chem. 21, 54–60. doi: 10.1021/jf60185a004
Eitinger T., Rodionov D. A., Grote M., Schneider E. (2011). Canonical and ECF-Type ATP-Binding Cassette Importers in Prokaryotes: Diversity in Modular Organization and Cellular Functions. FEMS Microbiol. Rev. 35, 3–67. doi: 10.1111/j.1574-6976.2010.00230.x
Ejsmond M. J., Blackburn N., Fridolfsson E., Haecky P., Andersson A., Casini M., et al. (2019). Modeling Vitamin B1 Transfer to Consumers in the Aquatic Food Web. Sci. Rep. 9, 10045. doi: 10.1038/s41598-019-46422-2
Follows M. J., Dutkiewicz S. (2010). Modeling Diverse Communities of Marine Microbes. Ann. Rev. Mar. Sci. 3, 427–451. doi: 10.1146/annurev-marine-120709-142848
Frank R. A. W., Leeper F. J., Luisi B. F. (2007). Structure, Mechanism and Catalytic Duality of Thiamine-Dependent Enzymes. Cell. Mol. Life Sci. 64, 892–905. doi: 10.1007/s00018-007-6423-5
Fridolfsson E., Lindehoff E., Legrand C., Hylander S. (2018). Thiamin (Vitamin B 1 ) Content in Phytoplankton and Zooplankton in the Presence of Filamentous Cyanobacteria. Limnol. Oceanogr 63, 2423–35. doi: 10.1002/lno.10949
Fuhrman J. A. (1999). Marine Viruses and Their Biogeochemical and Ecological Effects. Nature 399, 541–548. doi: 10.1038/21119
Gold K. (1968). Some Factors Affecting the Stability of Thiamine. Limnol. Ocean. 13, 185–188. doi: 10.4319/lo.1968.13.1.0185
Gómez-Consarnau L., Sachdeva R., Gifford S. M., Cutter L. S., Fuhrman J. A., Sañudo-Wilhelmy S. A., et al. (2018). Mosaic Patterns of B-Vitamin Synthesis and Utilization in a Natural Marine Microbial Community. Environ. Microbiol. 20, 2809–2823. doi: 10.1111/1462-2920.14133
Gray M. J., Escalante-Semerena J. C. (2010). A New Pathway for the Synthesis of α-Ribazole-Phosphate in Listeria Innocua. Mol. Microbiol. 77, 1429–1438. doi: 10.1111/j.1365-2958.2010.07294.x
Gutiérrez-Preciado A., Torres A. G., Merino E., Bonomi H. R., Goldbaum F. A., García-Angulo V. A. (2015). Extensive Identification of Bacterial Riboflavin Transporters and Their Distribution Across Bacterial Species. PloS One 10, e0126124. doi: 10.1371/journal.pone.0126124
Gutowska M. A., Shome B., Sudek S., McRose D. L., Hamilton M., Giovannoni S. J., et al. (2017). Globally Important Haptophyte Algae Use Exogenous Pyrimidine Compounds More Efficiently Than Thiamin. MBio 8, e01459-17. doi: 10.1128/mBio.01459-17
Haines K. C., Guillard R. R. L. (1974a). Growth of Vitamin B12-Requiring Marine Diatoms in Mixed Laboratory Cultures With Vitamin B12-Producing Bacteria. J. Phycol. 10, 245–252. doi: 10.1111/j.0022-3646.1974.00245.x
Haines K. C., Guillard R. R. L. (1974b). Growth of Vitamin B12-Requiring Marine Diatoms in Mixed Laboratory Cultures With Vitamin B12-Producing Marine Bacteria. J. Phycol. 10, 245–252. doi: 10.1111/j.0022-3646.1974.00245.x
Heal K. (2014). Determination of Four Forms of Vitamin B12 and Other B Vitamins in Seawater by Liquid Chromatography/Tandem Mass Spectrometry. Rapid Commun. Mass Spectrom. 28, 2398–2404. doi: 10.1002/rcm.7040
Heal K. R., Qin W., Ribalet F., Bertagnolli A. D., Coyote-Maestas W., Hmelo L. R., et al. (2017). Two Distinct Pools of B12 Analogs Reveal Community Interdependencies in the Ocean. Proc. Natl. Acad. Sci. 114, 369. doi: 10.1073/pnas.1608462114
Helliwell K. E. (2017). The Roles of B Vitamins in Phytoplankton Nutrition: New Perspectives and Prospects. New Phytol. 216, 62–68. doi: 10.1111/nph.14669
Helliwell K. E., Lawrence A. D., Holzer A., Kudahl U. J., Sasso S., Kräutler B., et al. (2016). Cyanobacteria and Eukaryotic Algae Use Different Chemical Variants of Vitamin B12. Curr. Biol. 26, 999–1008. doi: 10.1016/j.cub.2016.02.041
Itokawa Y., Kimura M., Nishino K. (1982). Thiamin-Binding Proteins. Ann. N. Y. Acad. Sci. 378, 327–336. doi: 10.1111/j.1749-6632.1982.tb31207.x
Jaehme M., Slotboom D. J. (2015). Diversity of Membrane Transport Proteins for Vitamins in Bacteria and Archaea. Biochim. Biophys. Acta - Gen. Subj. 1850, 565–576. doi: 10.1016/j.bbagen.2014.05.006
Jenkins A. H., Schyns G., Potot S., Sun G., Begley T. P. (2007). A New Thiamin Salvage Pathway. Nat. Chem. Biol. 3, 492–497. doi: 10.1038/nchembio.2007.13
Joglar V., Prieto A., Barber-Lluch E., Hernández-Ruiz M., Fernández E., Teira E. (2020). Spatial and Temporal Variability in the Response of Phytoplankton and Prokaryotes to B-Vitamin Amendments in an Upwelling System. Biogeosciences 17, 2807–2823. doi: 10.5194/bg-17-2807-2020
Johnson W. M., Kido Soule M. C., Longnecker K., Bhatia M. P., Hallam S. J., Lomas M. W., et al. (2021). Insights Into the Controls on Metabolite Distributions Along a Latitudinal Transect of the Western Atlantic Ocean. bioRxiv, 434501. doi: 10.1101/2021.03.09.434501
Jurgenson C., Begley T., Ealick S. (2009). The Structural and Biochemical Foundations of Thiamin Biosynthesis. Annu. Rev. Biochem. 78, 569–603. doi: 10.1146/annurev.biochem.78.072407.102340
Kazamia E., Czesnick H., Nguyen T. T., Van, Croft M. T., Sherwood E., Sasso S., et al. (2012). Mutualistic Interactions Between Vitamin B12-Dependent Algae and Heterotrophic Bacteria Exhibit Regulation. Environ. Microbiol. 14, 1466–1476. doi: 10.1111/j.1462-2920.2012.02733.x
Keinänen M., Uddström A., Mikkonen J., Casini M., Pönni J., Myllylä T., et al. (2012). The Thiamine Deficiency Syndrome M74, a Reproductive Disorder of Atlantic Salmon (Salmo Salar) Feeding in the Baltic Sea, is Related to the Fat and Thiamine Content of Prey Fish. ICES J. Mar. Sci. 69, 516–528. doi: 10.1093/icesjms/fss041
Koch F., Hattenrath-Lehmann T. K., Goleski J. A., Sañudo-Wilhelmy S., Fisher N. S., Gobler C. J. (2012). Vitamin B1 and B12 Uptake and Cycling by Plankton Communities in Coastal Ecosystems. Front. Microbiol. 3. doi: 10.3389/fmicb.2012.00363
Longnecker K., Sievert S. M., Sylva S. P., Seewald J. S., Kujawinski E. B. (2018). Dissolved Organic Carbon Compounds in Deep-Sea Hydrothermal Vent Fluids From the East Pacific Rise at 9°50′N. Org. Geochem. 125, 41–49. doi: 10.1016/j.orggeochem.2018.08.004
Lukienko P. I., Mel’nichenko N. G., Zverinskii I. V., Zabrodskaya S. V. (2000). Antioxidant Properties of Thiamine. Bull. Exp. Biol. Med. 130, 874–876. doi: 10.1007/BF02682257
Møller E. F., Thor P., Nielsen T. G. (2003). Production of DOC by Calanus Finmarchicus, C. Glacialis and C. Hyperboreus Through Sloppy Feeding and Leakage From Fecal Pellets. Mar. Ecol. Prog. Ser. 262, 185–191. doi: 10.3354/meps262185
Ma A. T., Tyrell B., Beld J. (2020). Specificity of Cobamide Remodeling, Uptake and Utilization in Vibrio Cholerae. Mol. Microbiol. 113, 89–102. doi: 10.1111/mmi.14402
McRose D., Guo J., Monier A., Sudek S., Wilken S., Yan S., et al. (2014). Alternatives to Vitamin B1 Uptake Revealed With Discovery of Riboswitches in Multiple Marine Eukaryotic Lineages. ISME J 8, 2517–29. doi: 10.1038/ismej.2014.146
Men Y., Seth E. C., Yi S., Allen R. H., Taga M. E., Alvarez-Cohen L. (2014). Sustainable Growth of Dehalococcoides Mccartyi 195 by Corrinoid Salvaging and Remodeling in Defined Lactate-Fermenting Consortia. Appl. Environ. Microbiol. 80, 2133–2141. doi: 10.1128/AEM.03477-13
Miranda-Ríos J., Navarro M., Soberón M. (2001). A Conserved RNA Structure (Thi Box) is Involved in Regulation of Thiamin Biosynthetic Gene Expression in Bacteria. Proc. Natl. Acad. Sci. 98, 9736–9741. doi: 10.1073/pnas.161168098
Paerl R. W., Bertrand E. M., Allen A. E., Palenik B., Azam F. (2015). Vitamin B1 Ecophysiology of Marine Picoeukaryotic Algae: Strain-Specific Differences and a New Role for Bacteria in Vitamin Cycling. Limnol. Oceanogr. 60, 215–28. doi: 10.1002/lno.10009
Paerl R. W., Bertrand E. M., Rowland E., Schatt P., Mehiri M., Niehaus T. D., et al. (2018a). Carboxythiazole is a Key Microbial Nutrient Currency and Critical Component of Thiamin Biosynthesis. Sci. Rep. 8, 5940. doi: 10.1038/s41598-018-24321-2
Paerl R. W., Bouget F. Y., Lozano J. C., Vergé V., Schatt P., Allen E. E., et al. (2017). Use of Plankton-Derived Vitamin B1 Precursors, Especially Thiazole-Related Precursor, by Key Marine Picoeukaryotic Phytoplankton. ISME J. 11, 753–765. doi: 10.1038/ismej.2016.145
Paerl R. W., Sundh J., Tan D., Svenningsen S. L., Hylander S., Pinhassi J., et al. (2018b). Prevalent Reliance of Bacterioplankton on Exogenous Vitamin B1 and Precursor Availability. Proc. Natl. Acad. Sci. U. S. A. 115, E10447–E10456. doi: 10.1073/pnas.1806425115
Parra M., Stahl S., Hellmann H. (2018). Vitamin B6 and Its Role in Cell Metabolism and Physiology. Cells 7, 84. doi: 10.3390/cells7070084
Pintner I. J., Altmeyer V. L. (1979). Vitamin B12-Binder and Other Algal Inhibitors. J. Phycol. 15, 391–398. doi: 10.1111/j.1529-8817.1979.tb00710.x
Poulson-Ellestad K. L., Harvey E. L., Johnson M. D., Mincer T. J. (2016). Evidence for Strain-Specific Exometabolomic Responses of the Coccolithophore Emiliania Huxleyi to Grazing by the Dinoflagellate Oxyrrhis Marina. Front. Mar. Sci. 3. doi: 10.3389/fmars.2016.00001
Provasoli L., Carlucci A. F. (1974). “Vitamins and Growth Regulators,” in Algal Physiology and Biochemistry. Ed. Stewart W. D. P.. (Berkeley and Los Angeles: University of California Press), 741–87.
Provasoli L., Pintner I. J. (1953). Ecological Implications of In Vitro Nutritional Requirements of Algal Flagellates. Ann. N. Y. Acad. Sci. 56, 839–851. doi: 10.1111/j.1749-6632.1953.tb30262.x
Reygondeau G., Guidi L., Beaugrand G., Henson S. A., Koubbi P., MacKenzie B. R., et al. (2018). Global Biogeochemical Provinces of the Mesopelagic Zone. J. Biogeogr. 45, 500–514. doi: 10.1111/jbi.13149
Rodionova I. A., Li X., Plymale A. E., Motamedchaboki K., Konopka A. E., Romine M. F., et al. (2015). Genomic Distribution of B-Vitamin Auxotrophy and Uptake Transporters in Environmental Bacteria From the Chloroflexi Phylum. Environ. Microbiol. Rep. 7, 204–210. doi: 10.1111/1758-2229.12227
Rodionov D. A., Arzamasov A. A., Khoroshkin M. S., Iablokov S. N., Leyn S. A., Peterson S. N., et al. (2019). Micronutrient Requirements and Sharing Capabilities of the Human Gut Microbiome. Front. Microbiol. 10, 1316. doi: 10.3389/fmicb.2019.01316
Rodionov D. A., Hebbeln P., Eudes A., ter Beek J., Rodionova I. A., Erkens G. B., et al. (2008). A Novel Class of Modular Transporters for Vitamins in Prokaryotes. J. Bacteriol. 191, 42–51. doi: 10.1128/JB.01208-08
Rosnow J. J., Hwang S., Killinger B. J., Kim Y.-M., Moore R. J., Lindemann S. R., et al. (2018). A Cobalamin Activity-Based Probe Enables Microbial Cell Growth and Finds New Cobalamin-Protein Interactions Across Domains. Appl. Environ. Microbiol. 84, e00955–e00918. doi: 10.1128/AEM.00955-18
Sañudo-Wilhelmy S., Cutter L. S., Durazo R., Smail E. A., Gómez-Consarnau L., Webb E. A. (2012). Multiple B-Vitamin Depletion in Large Areas of the Coastal Ocean. Proc. Natl. Acad. Sci. U.S.A. 109, 14041–5. doi: 10.1073/pnas.1208755109
Sañudo-Wilhelmy S. A., Gómez-Consarnau L., Suffridge C., Webb E. A.. (2014). The Role of B Vitamins in Marine Biogeochemistry. Ann. Rev. Mar. Sci. 6, 339–367. doi: 10.1146/annurev-marine-120710-100912
Sathe R. R., Paerl R. W., Hazra A. B. (2022). Exchange of Vitamin B1 and Its Biosynthesis Intermediates Shapes the Composition of Synthetic Microbial Cocultures and Reveals Complexities of Nutrient Sharing. J. Bacteriol. 0, e00503–e00521. doi: 10.1128/jb.00503-21
Sharma V., Rodionov D. A., Leyn S. A., Tran D., Iablokov S. N., Ding H., et al. (2019). B-Vitamin Sharing Promotes Stability of Gut Microbial Communities. Front. Microbiol. 10. doi: 10.3389/fmicb.2019.01485
Shelton A. N., Seth E. C., Mok K. C., Han A. W., Jackson S. N., Haft D. R., et al. (2019). Uneven Distribution of Cobamide Biosynthesis and Dependence in Bacteria Predicted by Comparative Genomics. ISME J. 13, 789–804. doi: 10.1038/s41396-018-0304-9
Shultis D. D., Purdy M. D., Banchs C. N., Wiener M. C. (2006). Outer Membrane Active Transport: Structure of the BtuB: TonB Complex. Science 312, 1396–1399. doi: 10.1126/science.1127694
Sokolovskaya O. M., Shelton A. N., Taga M. E. (2020). Sharing Vitamins: Cobamides Unveil Microbial Interactions. Science 369, eaba0165. doi: 10.1126/science.aba0165
Suffridge C. P., Bolaños L. M., Bergauer K., Worden A. Z., Morré J., Behrenfeld M. J., et al. (2020). Exploring Vitamin B1 Cycling and Its Connections to the Microbial Community in the North Atlantic Ocean. Front. Mar. Sci. 7. doi: 10.3389/fmars.2020.606342
Suffridge C., Cutter L., Sañudo-Wilhelmy S. (2017). A New Analytical Method for Direct Measurement of Particulate and Dissolved B-Vitamins and Their Congeners in Seawater. Front. Mar. Sci. 4, 175. doi: 10.3389/fmars.2017.00011
Suffridge C. P., Gómez-Consarnau L., Monteverde D. R., Cutter L., Arístegui J., Alvarez-Salgado X. A., et al. (2018). B Vitamins and Their Congeners as Potential Drivers of Microbial Community Composition in an Oligotrophic Marine Ecosystem. J. Geophys. Res. Biogeosciences 123, 2890–2907. doi: 10.1029/2018JG004554
Tang Y. Z., Koch F., Gobler C. J. (2010). Most Harmful Algal Bloom Species are Vitamin B1 and B12 Auxotrophs. Proc. Natl. Acad. Sci. 107, 20756–20761. doi: 10.1073/pnas.1009566107
Teeling H., Fuchs B. M., Becher D., Klockow C., Gardebrecht A., Bennke C. M., et al. (2012). Substrate-Controlled Succession of Marine Bacterioplankton Populations Induced by a Phytoplankton Bloom. Sci. (80-. ) 336, 608–611. doi: 10.1126/science.1218344
Vitreschak A. G., Rodionov D. A., Mironov A. A., Gelfand M. S. (2003). Regulation of the Vitamin B12 Metabolism and Transport in Bacteria by a Conserved RNA Structural Element. RNA 9, 1084–1097. doi: 10.1261/rna.5710303
Wienhausen G., Dlugosch L., Jarling R., Wilkes H., Giebel H. G., Simon M. (in press). Availability of Vitamin B12 and Its Lower Ligand Intermediate α-Ribazole Impact Prokaryotic and Protist Communities in Oceanic Systems. ISME J doi: 10.1038/s41396-022-01250-7
Wienhausen G., Moraru C., Bruns S., Den Q. T., Wilkes H., Dlugosch L., et al. (unpublished). Microbial Metabolic Patchwork – Vitamin B12 Auxotrophy of Two Bacteria Resolved by Ligand Crossfeeding and Prophage Induction.
Wilhelm S. W., Suttle C. A. (1999). Viruses and Nutrient Cycles in the Sea: Viruses Play Critical Roles in the Structure and Function of Aquatic Food Webs. Bioscience 49, 781–788. doi: 10.2307/1313569
Keywords: nutrient cycling, marine plankton, B-vitamin, vitamin B12, vitamin B1, microbial interactions, cross-feeding, auxotrophy
Citation: Wienhausen G, Bittner MJ and Paerl RW (2022) Key Knowledge Gaps to Fill at the Cell-To-Ecosystem Level in Marine B-Vitamin Cycling. Front. Mar. Sci. 9:876726. doi: 10.3389/fmars.2022.876726
Received: 15 February 2022; Accepted: 02 May 2022;
Published: 31 May 2022.
Edited by:
Paul Berube, Massachusetts Institute of Technology, United StatesReviewed by:
Samuel T. Wilson, University of Hawaii at Manoa, United StatesDanielle R. Monteverde, University of Southern California, United States
Copyright © 2022 Wienhausen, Bittner and Paerl. This is an open-access article distributed under the terms of the Creative Commons Attribution License (CC BY). The use, distribution or reproduction in other forums is permitted, provided the original author(s) and the copyright owner(s) are credited and that the original publication in this journal is cited, in accordance with accepted academic practice. No use, distribution or reproduction is permitted which does not comply with these terms.
*Correspondence: Ryan W. Paerl, cnBhZXJsQG5jc3UuZWR1