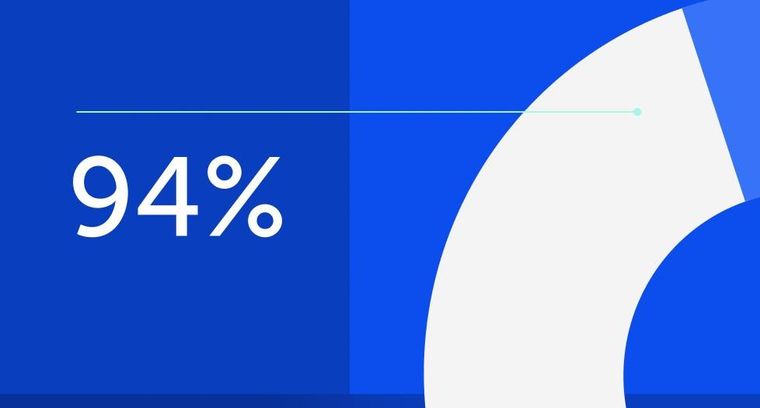
94% of researchers rate our articles as excellent or good
Learn more about the work of our research integrity team to safeguard the quality of each article we publish.
Find out more
ORIGINAL RESEARCH article
Front. Mar. Sci., 13 June 2022
Sec. Marine Conservation and Sustainability
Volume 9 - 2022 | https://doi.org/10.3389/fmars.2022.875541
This article is part of the Research TopicTracking Marine Megafauna for Conservation and Marine Spatial PlanningView all 35 articles
One direct consequence of Arctic warming is the expansion of navigable portions of the Arctic Ocean. As a result, vessel traffic and the accompanying threats of spills, strikes and disturbance is intensifying throughout the Arctic. In the Bering Sea, these threats to the environment, wildlife and to the people who rely on marine resources for food and cultural continuity, are acute. We examined the spatial relevance of an Area To Be Avoided (ATBA), a shipping-risk mitigation measure, established around St. Lawrence Island with respect to seabirds, as sentinel species, habitat use. We studied four seabird species (common murre Uria aalge, thick-billed murre U. lomvia, crested auklet Aethia cristatella, black-legged kittiwake Rissa tridactyla) breeding at St. Lawrence Island in the northern Bering Sea. GPS tracking data from 47 at-sea foraging trips showed that both murre species and crested auklets distributed outside the ATBA, during at least one stage of the breeding season. A larger dataset based on the birds’ red blood cell isotopic signatures confirmed that for murres, the tracked individuals covered the broad niche exploited by these species. Habitat modelling further showed that the birds’ most suitable marine habitats were associated with seasonal surface chlorophyll blooms, and largely extended beyond the ATBA on the shelf north of the island. Data on the murres’ diet and diving behavior emphasized the importance of the shelf as a foraging habitat for these birds. We suggest that extending the ATBA to the north by only 35 km, would include areas of maximal habitat suitability. This extension would better protect seabirds, their foraging habitats and the cultural continuity of St. Lawrence Islanders, against growing threats stemming from Arctic warming.
Decreased Arctic sea ice extent has opened up new northern shipping routes (AMSA, 2009; Yumashev et al., 2017). Climate modelling indicates that these ice-free routes in the Arctic Ocean will continue to expand spatially and seasonally (Smith and Stephenson, 2013; Aksenov et al., 2017). Accordingly, vessel-based commerce has developed, and by 2025 Arctic vessel traffic is projected to triple compared to what it was a decade ago (International Council on Clean Transportation, 2015).
In particular, all vessel traffic between the Arctic and Pacific Oceans must pass through the Bering Strait, a maritime bottleneck that has become a focal point for Arctic shipping regulation and the conservation of biodiversity (AMSA, 2009; Hauser et al., 2018). The Bering Sea is known for its remarkable biological richness fuelled by high levels of surface primary productivity, and high carbon export to the benthos due to limited zooplankton grazing (Hunt et al., 2002; Grebmeier et al., 2006; Grebmeier, 2012). This results in a benthic-dominated system that supports large populations of seabirds and marine mammals (Grebmeier et al., 2015), which in turn support ancient and vibrant Indigenous cultures (Fall et al., 2013). Thus, with the increasing vessel traffic in the region, there is a potential for magnified threats to both the local marine environment and to the people who rely on this marine wildlife for food and cultural resources. Vessels in transit pose several environmental risks, such as exposure to oil, emission of gases and particles from exhausts (sulphur dioxide, nitrous oxide, ozone, black carbon), direct disturbance to wildlife including displacement and collision risks (ship strikes), noise-related impacts, discharge of contaminants and the introduction of invasive species (Huntington et al., 2015; Stevenson et al., 2019; Dehnhard et al., 2020), which may translate into the loss of biodiversity and cultural heritage (Huntington et al., 2015; Tempel et al., 2021).
In view of the magnitude, severity and rapidity of the growth of threats to the marine environment in this region, the existing network of marine areas implemented for the conservation of species and habitats is seen as inadequate and insufficient (Harris et al., 2018). Recent research in the Arctic has focused on marine mammals (e.g., Hauser et al., 2018); however, seabirds also are at risk of conflict with human activities in the Arctic (Humphries and Huettmann, 2014; Wong et al., 2018), and represent a considerable ecological and cultural component in the Bering Sea (Huntington et al., 2015). Seabirds are major secondary consumers in this ecosystem (Hunt et al., 2002; Grebmeier et al., 2015), and are a critical aspect of food security and cultural continuity for indigenous Arctic peoples in the region (Fall et al., 2013; Huntington et al., 2015; Naves, 2018; Will et al., 2020a). In particular, the importance of seabirds as subsistence resources is the highest (81%) for peoples from St. Lawrence and Diomede Islands, islands that are within or adjacent to the Bering Strait. Seabird harvesting on these islands largely relies on auklets Aethia spp. and murres Uria spp., which are among the most abundant seabird species in the region (Kuletz et al., 2015), and occurs in spring and summer (Naves, 2018).
In this context, there is an urgent need to protect both biological resources and subsistence activities from the potential impacts of increasing vessel traffic in the Bering Strait region. Implementing Areas To Be Avoided (ATBAs) for shipping offers one mechanism to help achieve these goals. Three ATBAs have recently been adopted by the International Maritime Organization (IMO) in this region. These are around King, Nunivak, and St. Lawrence islands (Huntington et al., 2019). However, there is concern that the implemented ATBA around St. Lawrence Island may not be wide enough based on initial consultation with the public (Huntington et al., 2019). This situation warrants scientific evaluation on the relevance of the current boundaries of the St. Lawrence Island ATBA to seabird habitat use. Tracking marine animals, and seabirds in particular, has strong potential to inform policy and management (Thaxter et al., 2012; Hays et al., 2019). In this paper, we use the first tracking data collected on seabird species breeding at St. Lawrence Island (three Alcidae: the common murre Uria aalge, the thick-billed murre U. lomvia, the crested auklet Aethia cristatella and one Laridae: the black-legged kittiwake Rissa tridactyla), to examine whether these species’ foraging areas distributed inside the adopted ATBA’s boundaries. During breeding, seabirds behave as central-place foragers (Orians and Pearson, 1979), thus their relatively restricted foraging range should provide a conservative estimate of their habitat use around the island (Oppel et al., 2018). This would ensure that we evaluated the ATBA boundaries for the seabird populations that use the island to breed, and which may be locally harvested by the islanders. We characterized habitat use by both GPS tracking, for all species, and dive behaviour and chick-diet observations, for murres only. We supplemented these data with a larger dataset from stable isotope data on the birds’ blood, to evaluate how representative the individual tracking data may be. Based on these results, we discussed the options to improve the design and management of the St. Lawrence ATBA.
St. Lawrence Island (Sivuqaq in Siberian Yupik) is a large island (4,640 km2) situated in the northern Bering Sea, directly south of the Bering Strait (Figure 1). It is surrounded by an extensive shelf, providing shallow depths (less than 30 m) around the island, especially to the east. St. Lawrence Island sits in a very dynamic hydrographic system, with two strong currents flowing northward (the Anadyr Current on the West side of the island, and the Alaska Coastal Current on the East) which supply nutrient-rich waters from the southern Bering Sea basin all the way up into the Chukchi Sea (Springer et al., 1987; Hunt et al., 1990; Piatt and Springer, 2003). The regional topography funnelling the northward flow of these currents also creates a locally longitudinal current along the north coast of St. Lawrence Island, and particularly near the north-west and north-east capes (Danielson et al., 2014).
Figure 1 Map of the study area showing St. Lawrence Island in the northern Bering Sea, surrounded by the Area To Be Avoided for shipping (ATBA, blue shade). Isobaths -20, -30 and -40 m are drawn in grey dotted lines. Study colonies are indicated with filled circles: from west to east: Kitnik (gold), Myughi (red), Tuqaghuk (blue).
We studied seabirds from three colonies conveniently accessible from Savoonga and located on the northern coast, where the ATBA is the narrowest, a situation which may put these northern colonies at higher risk of adverse impacts from shipping. Four seabird species were studied on the island. Most of our effort focused on murres: common and thick-billed, which breed sympatrically in large numbers on seaside cliffs around the island. Current estimates of numbers on St. Lawrence Island are 337,447 and 279,219 individuals for the common and thick-billed murres, respectively (from the Circumpolar Seabird Data Portal, http://axiom.seabirds.net/). In the Bering Sea, common murres primarily consume pelagic schooling forage fish (Kokubun et al., 2016; Barger et al., 2016), while thick-billed murres are more generalist foragers that consume a mix of benthic prey, pelagic forage fish and large invertebrates (Harding et al., 2013; Barger et al., 2016). Earlier reports at St. Lawrence Island (Piatt et al., 1988) noted that these two species are markedly synchronous in their breeding cycle: the median laying date was between late June and early July for both species, with hatching occurring in early August and chicks fledging in late August. Murres were studied at Tuqaghuk (63.54°N; -170.06°E) and Cape Myughi (63.66°N; -170.22°E) colonies on the north shore of the island. Besides murres, two other seabird species were surveyed. First, the crested auklet was studied from Kitnik colony (63.71°N; -170.42°E). Auklets are planktivorous alcids relying predominantly on Neocalanus copepods during the breeding season (Gall et al., 2006; Sheffield Guy et al., 2009). They nest in burrows underneath boulders, and lay eggs on St. Lawrence around late June; hatching occurs between late July and early August, and the chicks fledge at the end of August (Gall et al., 2006). A total of 1,528,455 crested auklets are estimated to breed on St. Lawrence Island. Last, we studied the black-legged kittiwake, which similarly to murres is a cliff-nesting and piscivorous species, but feeds at the surface (Bayer, 1983); it also breeds synchronously to the murres. Kittiwakes were studied from Cape Myughi colony, and 53,937 individuals are estimated to breed on the island. Across their differences in foraging ecology, the four species studied here provide a synoptic indication of the at-sea ecology of the seabird community breeding at St. Lawrence Island.
Fieldwork was conducted in the summers of 2016 to 2019. GPS loggers were attached onto breeding birds that were caught either using a noose pole (for the cliff-nesting species) or by hand under the boulders (auklets). The loggers were attached to the back feathers on the dorsal midline using mastic and waterproof Tesa © tape; the ends of the tape were locked using Loctite © glue. Two different types of GPS loggers were used: first, to track murres (2016 and 2017) and kittiwakes we used CatTraq loggers (CatLogTM, 16 Mb memory, 380 mA lithium-ion battery, Catnip Technologies, USA). These GPS loggers were housed in waterproof heat-shrink tubes before being deployed on the birds (final mass: 15 g, final size: 14 mm × 35 mm × 70 mm). Second, we used Ecotone GPS-UHF short-range download loggers (Alle and Uria series, Ecotone Telemetry, Gdynia, Poland) to track murres in 2019 (model Uria-100; dimensions: 35 × 16 × 11 mm; mass: 8.5 g) and auklets in all years (model Alle-60; dimensions: 26 × 16 × 10 mm; mass: 4.5 g). The attachment or recovery of loggers and associated body measurements and/or physiological samples took 10 to 20 minutes per bird. Tracking data per year, species and breeding stages are detailed in Table 1; no tracking data were collected in 2018 when very low breeding success was observed in all species (Will et al., 2020b).
Table 1 Summary of tracks available per seabird species, study year, breeding stage and model of tracking unit.
The location acquisition was set with a 2-minute interval on the CatTraq loggers, and the loggers were retrieved after the birds made several foraging trips to sea. The Ecotone loggers were set to communicate with a base station positioned near the study nest(s) using ultra-high frequency signal; in this case the loggers were not retrieved and the signal was acquired until the batteries died or the logger was shed. The location acquisition was set with a 5-minute interval in 2016, a 10-minute interval in 2017, and a 15-minute interval in 2019 on the Ecotone loggers. Retrieval was challenged by the fact that birds were extremely shy to human presence.
In addition, the diving behaviour of murres was surveyed using time-depth recorders. Cylindrically-shaped loggers (Little Leonardo, Tokyo, Japan; model ORI400-D3GT; dimensions: 12 mm diameter × 45 mm length; mass: 9 g) were deployed ventrally and retrieved together with GPS units in 2016, providing depth and temperature records every second; and tri-axial acceleration data every 0.25 second. However, temperature and acceleration data were not used in this study.
Data were downloaded from the loggers or the base station in the field to a computer. The first phase of analysis was to identify within each dataset the start and end points of the different trips made by the bird. Through a detailed inspection of the locations, at-sea foraging trips were separated from isolated erroneous locations or small-scale movements away from the nest reflecting recapture attempts of skittish birds. This approach identified all trips as lasting > 1 h, similar to previous studies (e.g., Kokubun et al., 2015). Second, periods at the nest between trips were removed, keeping only the start and end points of each trip at this location (or the last point recorded in the case of incomplete tracks caused by battery failure). Third, movement metrics were derived from each trip: maximum range reached from the nest (km), trip duration (h), and bearing to the furthest point (degrees, clockwise with North as 0). Fourth, each trip was linearly re-interpolated with an exact 2-minute interval, because locations were occasionally missing or were not recorded at constant intervals. The incomplete tracks were not re-interpolated past the last recorded point. This analysis was performed using the R packages “sp” and “trip”. Preliminary distribution maps derived from these tracks have been presented in Will et al. (2020b); nevertheless, these data provide the first crested auklet GPS tracks. Data on diving behaviour were analysed with Igor Pro (WaveMetrics, Oregon), with maximum dive depth and bottom time of each dive calculated as in Takahashi et al. (2008).
An analysis of pseudo-replication was undertaken to evaluate whether successive trips made by one individual could be considered as independent trips made by distinct individuals. We followed the standard “toolkit” script provided by BirdLife International in Lascelles et al. (2016), specifically designed to account for this point, consistently across tracking analyses for conservation purposes. The pseudo-replication analysis, based on iterative Mann-Whitney U-tests, compared the intra-individual versus inter-individual proximity in core distribution areas among trips made by individuals of a same species and breeding stage. This analysis was performed only when at least two trips were collected for each of at least two individuals, during a given breeding stage and study colony. This analysis showed that in all examined datasets (common murres during incubation, and thick-billed murres during incubation and chick-rearing), the core areas from one individual’s multiple trips were not closer to each other than that of trips from different individuals (Mann–Whitney U tests, p-value = 0.48 for common murres during incubation, 0.50 for thick-billed murres during incubation, and 0.56 for thick-billed murres during chick-rearing). Hence, individual pseudo-replication was not a significant issue and all the GPS tracks were treated as independent observations.
To count the number of tracking locations included within the ATBA for each bird trip, we used the function “over” in the R package “sp”. This function examines whether a spatial polygon (the St. Lawrence ATBA in this case) overlaps with point coordinates (the GPS tracks).
Trips were compared between species or breeding stages according to their maximum range, duration, and proportion extending beyond the ATBA. Variables were first tested for normal distribution using a Shapiro-Wilk Normality Test, and depending on the result, parametric (one-way ANOVA, t-test) or non-parametric (Wilcoxon and Kruskal-Wallis rank sum tests) comparison tests were conducted. The threshold for significance was set at p = 0.05. When significant differences occurred among more than two factor levels, we undertook a Tukey’s post-hoc multiple comparison test to identify which group(s) significantly differed from others. All analyses were performed using R 3.4.2 (R Core Team, 2022). Unless otherwise stated, values reported are mean ± standard deviation. The effect of sex was not examined here, due to low sample sizes for each combination of species, year, colony and breeding stage. However, studies confirm that although the partners may segregate in time for at-sea provisioning, habitat selection and diet is similar among sexes in murres (Huffeldt et al., 2021).
We examined whether the current ATBA limits encompassed the potential foraging distribution of seabirds from all breeding locations known around St. Lawrence Island, based on the foraging ranges measured for each species tracked in this study. To this end, we used the Circumpolar Seabird Data Portal to locate all colonies known on St. Lawrence Island for the four studied seabird species (http://axiom.seabirds.net/maps/js/seabirds.php?app=circumpolar#z=8&ll=64.54111,-170.24739). Using the maximum foraging range observed for the tracked birds in each species, we projected simple buffers of the seabirds’ potential distribution around the island.
Using the Mahalanobis Distance Factorial Analysis (MADIFA, Calenge et al., 2008) approach, we quantified habitat suitability levels around the island based on habitat covered by bird tracks. Habitat selection was identified according to six environmental variables: seafloor depth (bathymetry), seafloor slope (bathymetry gradient), sea-surface temperature, sea-surface chlorophyll concentration, and zonal and meridional current velocity. The MADIFA approach is adequate for this purpose using presence-only data, such as GPS tracking, and performs particularly well in a situation where environmental variables may be highly correlated (for a comparison of methods see Tsoar et al., 2007). In the MADIFA, two principal component analyses (PCAs) respectively summarize the information on (a) the environment described by spatial variables; and (b) the relationship (distance) between the animals’ habitat niche (inferred from GPS locations) and the hypervolume described by the environmental covariates (R package “adehabitatHS”). Bathymetry data were downloaded from NOAA’s ETOPO1 1 Arc-Minute Global Relief Model (https://ngdc.noaa.gov/mgg/global/global.html), from which the seafloor gradient was computed. For sea-surface temperature and chlorophyll concentration, monthly data were downloaded from NOAA’s ERDDAP server (https://coastwatch.pfeg.noaa.gov/erddap/griddap/jplMURSST41mday and https://coastwatch.pfeg.noaa.gov/erddap/griddap/erdVHNchlamday, respectively). Specifically, the datasets were the Multi-scale Ultra-high Resolution (MUR, global, 0.01° resolution, 2002-present) temperature data, and the monthly composite rasters of chlorophyll concentration for the North Pacific (NOAA VIIRS, 750m resolution, 2015-present). Current velocity data were downloaded from the Ocean Surface Current Analysis Real-time project (OSCAR; https://podaac-opendap.jpl.nasa.gov/opendap/allData/oscar/preview/L4/oscar_third_deg/). In these datasets the currents’ horizontal velocity (zonal and meridional) is directly estimated from sea surface height, surface vector wind and sea surface temperature. These data are available at a 5-day temporal resolution and one third spatial degree grid. All datasets were subsequently aggregated at this latter (coarser) spatial resolution; and the environmental variable files were subsampled to cover the time span of the tracking dataset.
Using MADIFA, we modelled the habitat suitability levels separately for each species, breeding stage and year. However, in the case of kittiwakes, the extremely small distribution range observed from the tracks precluded estimating habitat suitability, because of the insufficient number of cells used across the environmental grid. Hence, no niche modelling analysis is shown for kittiwakes. The predictions from these models were merged following three successive steps, consistently giving a similar weight to each component in the combination (following Thiers et al., 2017). First, the mapped predictions (% values in each cell) were averaged between years for the same species and breeding stage (e.g., common murres tracked during chick-rearing in 2016 versus 2017). Second, the results within each species were combined across breeding stages and years (case of the common murres in 2016/2017 versus 2019, the thick-billed murres in 2016 versus 2019, and the crested auklets in 2016 versus 2017). This step provided one prediction map per species. In the third step, the predictions were merged across species, to produce a single habitat suitability map for the locally-harvested seabird community.
We did not include distance to the colony as a spatial constraint to the potentially suitable habitats in the region. This approach was chosen deliberately so that the analysis based on the tracked birds’ observed selection may also reveal habitats deemed potentially suitable to birds from other colonies, assuming the selected habitat is comparable across colonies. Second, in this analysis our objective was to identify areas where birds may preferentially distribute at sea, but not strictly for foraging. Accordingly, all available tracking locations were included in the model, potentially reflecting any activity by the birds: commuting or resting, in addition to foraging.
The results from stable isotope analysis provide a complementary approach to the individual movement tracking data. Although it is not possible to directly translate these results into spatial context, isotopic ratios were sampled in larger numbers across species and years, and thus provided a convenient perspective to examine the representativeness of the limited individual GPS tracking data among the broader foraging niche revealed by the isotopic signatures. Thus, this comparison is used to assess how confident we may be in using the GPS tracks to represent the species’ foraging strategies. In this study we re-analyzed the data presented in Will et al. (2020b), by aggregating the data across years, in contrast to the previous report which focused on interannual variability.
Blood samples were taken throughout the breeding season from 2016 to 2019, from the birds’ alar vein using a heparinized syringe or a needle and heparinized capillary tubes. Samples were kept cool until centrifuged (within 12 hours of collection, as much as possible), and separated into plasma and red blood cell components. Samples were stored frozen (-80°C) until analyzed. Analysis of the samples was performed as described in Will et al. (2020b). Red blood cell samples were thawed, subsampled, and dried at 35°C in an isotemp vacuum oven. These samples have a relatively lower turnover rate than plasma, and reflect the isotopic niche of birds 1-2 months prior to the sampling date, hence during the early breeding season (Hobson and Clark, 1993). Dried red blood cell samples of 0.4 to 0.8 mg were weighed, placed into individual tin capsules, and submitted to the University of Alaska Fairbanks Stable Isotope Facility for analysis. There, they were processed in a continuous-flow isotope ratio mass spectrometer. A peptone standard was used to calibrate the 13C:12C and 15N:14N ratios (inter-tray SD: δ13C = 0.02 ‰, δ15N = 0.03 ‰). Sample stable isotope ratios are expressed as δ13C δ15N = [(Rsample/Rstandard) - 1] × 1000, where R represents the ratio of 13C:12C or 15N:14N. These results are reported as delta values in parts per thousand (‰).
Isotopic niches (from δ13C and δ15N) are presented as relative values to the average for the year and species, and shown as Bayesian standard ellipses computed in the R package “SIAR” (Jackson et al., 2011).
To further investigate the murres’ habitat use, direct observations of the prey species brought back to the nest in bill loads (whole fish) were conducted as frequently as possible on the colonies of Tuqaghuk and Cape Myughi, in 2016 and 2017, using either 10 × 42 binoculars, a telescope, or photographs (Canon © digital camera with 300 mm lens). These observations were conducted over several hours during a total of 21 days: five days in 2016 (from 14 through 20 August), and in 2017 during four days in July (from 19 through 26 July) and twelve days in August (from 2 through 21 August). Identification of observed prey species was conducted to the lowest possible taxonomic level. This information on the captured prey is pertinent in our study to assess how much the birds may rely on benthic prey from the shelf to provision their offspring, and therefore, how important it may be to ensure the protection of these key foraging habitats from the development of human activities. Birds relying predominantly on pelagic prey would indicate a lower importance of the shelf habitat itself to the birds (Springer et al., 1987; Hunt et al., 1990; Piatt and Springer, 2003).
In total, 47 tracks were available from 19 birds: 16 tracks from 7 common murres, 20 tracks from 7 thick-billed murres, 6 tracks from 4 crested auklets and 5 tracks from 1 black-legged kittiwake (Table 1).
All tracks extended to more than two km away from the nest, suggesting these were actually at-sea trips (Table 2). In all surveyed years and stages, the murres of both species moved to the northeast or east from their nest, towards the shallower area of the shelf (Figure 2). The auklets moved more northwardly towards areas with deeper waters, and performed large-scale looping or sinuous trips during incubation and more directional trips during chick-rearing. In contrast, the surveyed kittiwake remained very close to the coast throughout its five similarly small-scale trips during chick-rearing. This small-scale distribution during that stage confirmed the authors’ observations in the field, feeding flocks of kittiwakes were commonly visible to the naked eye from the coast in all study years.
Figure 2 At-sea distribution of the birds tracked from St. Lawrence Island (common murres U. aalge: n = 16 tracks, thick-billed murres U. lomvia: n = 20 tracks, black-legged kittiwake Rissa tridactyla: n = 5 tracks, and crested auklets Aethia cristatella: n = 6 tracks), relative to the contour of the Area To Be Avoided (black line). The tracks collected during the incubation versus chick-rearing stages are shown in darker versus lighter colors, respectively. The -30 m isobath is drawn in grey dotted line.
There was an overall significant difference in the maximum foraging range observed between species (Kruskal-Wallis χ23 = 13.201, p = 0.004), however no inter-specific difference was significant between pairs of species, with only auklets’ versus kittiwake’s range approaching the significance threshold (Tukey’s test, adjusted p = 0.063; and > 0.17 in all other cases). There was no overall significant difference in the maximum foraging range between breeding stages (Wilcoxon test, W = 325, p = 0.27), even when excluding the kittiwake trips (W = 290, p = 0.08). However, within species, the maximum foraging range differed significantly between breeding stages in common murres (greater during chick-rearing than during incubation; Wilcoxon test, W = 58, p = 0.003). It was not significantly different between stages in thick-billed murres (Wilcoxon test, W = 41, p = 0.55) and due to very low sample sizes this was not statistically tested in crested auklets. Overall, in five out of the seven cases of species/breeding stages, average trip bearings were comprised between 15.3-90.5 degrees (north-east sector).
In total, ten bird tracks (>21% of all tracks) extended beyond the boundaries of the ATBA: three of the 16 tracks of common murres (19%; all of them during chick-rearing), four of the 20 tracks of thick-billed murres (20%; all of them during incubation), and half of the six auklet tracks. There was no significant difference between the proportion of common versus thick-billed murre trips extending outside the ATBA (Fisher’s exact test for count data, p = 1). However, there was a significant effect of the breeding stage in murres on the proportion of trips extending outside the ATBA, with significantly more trips extending outside the ATBA during chick-rearing in common murres (Fisher’s exact test for count data, p = 0.036), versus more during incubation in thick-billed murres (p = 0.026). Auklets were the only tracked birds to extend trips beyond the ATBA during both incubation and chick-rearing stages. None of the kittiwake tracks extended beyond the ATBA.
The proportion of locations falling outside the ATBA for each trip varied between 0 and 84.2% for common murres, and between 0 and 73.7% for thick-billed murres. This represented an average portion of 10.2% ± 26.4 of each trip’s locations for common murres (both stages pooled), and 10.0% ± 22.6 for thick-billed murres (no significant difference between species, Wilcoxon test, W = 159, p = 0.98). In common murres, the three trips extending outside the ATBA had 54.6% ± 39.8 (range: 9.4 – 84.2) of their locations outside the area; in thick-billed murres, that was 50.1% ± 23.6 (range: 24.8 – 73.7) for the four trips extending outside the area.
There are 25 sites where the four seabird species surveyed in this study are known to breed on St. Lawrence Island. Projected foraging ranges around these locations extended beyond the boundaries of the ATBA in virtually all directions: in the north, west, south and east (Figure 3). However, only in the north, where the ATBA boundary is closer to the shore, did the three species (the common and thick-billed murres and the crested auklet) have their foraging range extend markedly beyond this limit. The foraging distribution of black-legged kittiwakes, based on the maximum foraging range measured for single individual, did not seem to extend outside the ATBA.
Figure 3 Projected foraging range of seabirds, based on foraging tracks collected in this study, for 25 colonies (yellow crosses) around St. Lawrence Island for common murres (green areas), thick-billed murres (blue areas), crested auklets (gold areas) and black-legged kittiwakes (red areas). The contour of the ATBA around the island is indicated in black.
The MADIFA adequately summarized on a single principal component the relationship between the birds’ habitat use and the environmental covariates, with generally 80–90% of variance explained by the first component (Supplementary Figure 1). Sea-surface chlorophyll concentration was the highest scoring variable in most models (5 out of 7, with absolute scores on the first principal component >0.90 in four out of these five cases), and was the second-best variable in another case (thick-billed murre data from 2019, absolute score: 0.49). In the latter case, bathymetry was the best explanatory variable (absolute score: 0.65), and this variable was the second-best in three other cases: common murre data from 2017 and 2019, and crested auklets from 2017 (absolute scores: 0.45, 0.63, and 0.47, respectively). Zonal current velocity (longitudinal, noted U) was the best explanatory variable in one case (common murre data from 2016, score: 0.96), when both sea-surface chlorophyll concentration and bathymetry showed extremely low scores. Zonal current velocity was the second-best score for thick-billed murre data from 2016 (score: 0.65). These three variables (sea-surface chlorophyll concentration, bathymetry and zonal current velocity) were the only ones with predominant scores on the first principal component in at least one instance, and had low scores <0.10 occurring in only one model for each of these variables. SST was never the highest scoring variable in any model, but showed absolute scores ranging from 0.31–0.58 except in two cases when it was <0.1. SST was only the second-best explanatory variable in two cases: for common murre and crested auklet data, both from 2016. The two other variables used in the habitat model (meridional current velocity and the bathymetry gradient) were never among the higher-scoring factors. Meridional current velocity showed absolute scores ranging 0.18–0.35 except in two cases when it was <0.1; for bathymetry gradient, the absolute scores were 0.10 or less in three models, and reached only 0.17 at most.
In each case-specific projection (Supplementary Figure 1), the highest levels of habitat suitability were found off the north-eastern coasts of the island, whereas lower levels usually appeared off the southern coast.
This pattern was particularly evident in the years 2016 and 2019 across species, when high levels of suitability were generally found from 63 to 66°N; whereas this was less marked in 2017 (common murre and crested auklet datasets), when the distribution of high suitability areas tended to be more balanced off the north and south coasts.
When merging predictions at the species level (Figure 4), it was clear that highly suitable foraging habitats were available for the birds off the north-east coasts of the island. However, projections for thick-billed murres were unique in predicting large favourable areas to the north up to the Bering Strait, whereas suitable areas for common murres were in a relatively narrow band north-east from the island to the continent. For crested auklets, the marine areas predicted as suitable did not extend as far to the north as it was predicted for the murres, however these areas spread more to the western sector of the island.
Figure 4 Results of the Mahalanobis Distance Factorial niche analysis, showing the projected habitat suitability levels (expressed in %) of common murres (A); thick-billed murres (B); crested auklets (C), and for the three species combined (D). The boundaries of the ATBA around St. Lawrence Island is shown in black lines, and the white dashed lines indicate the suggested 35 km extension of the ATBA. These projection maps do not account for distance from the tracked colony as a covariate to evaluate regional habitat suitability.
Overall, across species, the most suitable marine habitats for the seabird community breeding on St. Lawrence Island were projected to be concentrated off the north-east coasts of the island. However, this relatively small patch of high suitability (>90%) extended from the island to about 50 km offshore, more than twice as much as the ATBA.
Data collected through time-depth recorders retrieved from two thick-billed murres indicated shallow diving behaviour during daytime, up to 41 m depth (Figure 5), and shallower than 10 m during the Arctic summer twilight. Dive bouts exhibited by murres during daytime often showed typical benthic dive behaviour (constant maximal dive depth to specific depths between 37 and 41 m). These benthic dives were not numerous (7.6 and 4.0% of 236 and 330 dives recorded), nevertheless contributed disproportionally to total bottom time recorded by the two birds (17.0 and 9.5% of 101 and 143 min. of total bottom time, respectively). These results indicate that the birds likely relied, at least partially, on the benthic shelf habitat for provisioning.
Figure 5 Time-depth records collected from two thick-billed murres U. lomvia (TM16180 and TM16187) (A, C) surveyed from Tuqaghuk colony during the chick-rearing stage in 2016. The arrows indicate trip departure (up arrow) and return (down arrow) based on acceleration and temperature. The middle panel (B) is detailed profiles of dives reaching to constant depth (37.6–37.8 m), showing characteristic benthic diving behaviour.
This was supported by the direct observations made on the murres’ diet. Prey brought to the nest by both species of murres consisted of fish species only (Table 3). Capelin Mallotus villosus and Pacific sand lance Ammodytes hexapterus were the only identified species of pelagic prey; whereas three identified taxa of benthic prey were observed, among which the Pricklebacks (Stichaeidae) were the most often found. A substantial proportion of the observed prey could not be identified, in both murre species and years (35.5 ± 19.6%). Considering only the identified prey species, the diet of common murres consisted of benthic fish at 33.3 and 77.9% in 2016 and 2017, respectively, and that was 70.0 and 94.9% for the thick-billed murres.
Table 3 Food items delivered to the chicks by common and thick-billed murres at St. Lawrence Island in 2016 and 2017.
Isotopic data were available for all studied species and years, with 52–96 samples available per species (see Table 4 for sample sizes per species and year). In common and thick-billed murres, the stable isotope signatures of birds with GPS data overlapped the broad spectrum of year-species-specific isotopic niches (Figure 6). This indicates that the diets and foraging behaviour of GPS-sampled birds were representative of species-specific foraging patterns typical for the breeding murre populations sampled on the island. However, in black-legged kittiwakes, the one bird that had both GPS and isotopic samples, appeared outside the ellipses (Figure 6D); indicating that the behaviour of that single individual likely represents a smaller portion of the population foraging trips in the nearshore habitat, while the majority of birds are likely to have different foraging patterns that we were not able to document through GPS tracking. Finally, no crested auklet with isotopic sample had concomitant GPS data, and thus it was not possible to assess representativity in this case.
Figure 6 Standard Bayesian ellipse areas (solid lines) corrected for small sample sizes ((A): common murres; (B): thick-billed murres; (C): crested auklets; (D): black-legged kittiwake). The axes show the δ13C and δ15N isotopic ratios relative to the average values per species and year. Points show individual values; the larger points represent isotopic signatures of birds for which GPS data was also available (no GPS data available among crested auklets with isotopic samples).
Using the first individual GPS tracking data collected on four seabird species breeding on St. Lawrence Island, our study revealed that three of these species exploited at-sea habitats outside the ATBA: the common and thick-billed murres, and the crested auklet. Movements extending beyond the ATBA boundaries were repeatedly observed in these species despite the limited number of tracks that were collected.
The results from murres are particularly illustrative of a mismatch between ATBA and birds foraging distributions. Specifically, the murre foraging ranges measured in our study are relatively small compared to other study sites. Common murres from the Bering and Chukchi Seas showed foraging ranges reaching up to more than double the values measured in our study (e.g., Hatch et al., 2000; Kokubun et al., 2016), whereas values from the Atlantic sites were more similar to those recorded in our study (e.g., Cairns et al., 1987; Gulka et al., 2019; Delord et al., 2020). In thick-billed murres, foraging ranges were greater both in the Atlantic (e.g., Benvenuti et al., 2002; Gaston et al., 2013) and Pacific (Hatch et al., 2000; Kokubun et al., 2016) regions than we recorded at St. Lawrence Island. Yet, despite their comparatively small foraging ranges, the ATBA north to the island was too narrow to contain the at-sea distribution of both species of murres breeding at St. Lawrence Island (Figure 2). Whether habitat use from the other colonies on the island are the same as predicted here, or remain within the ATBA is not known. Tracking studies on murres from the south-eastern Bering Sea showed dramatically divergent foraging distribution and habitat selection, depending on the colony location on the same island (Paredes et al., 2015). Thus, the fact that areas predicted as the most suitable (>90%) extend from the ATBA to the north only (Figure 4), should be considered a minimum region to be included in the ATBA.
For auklets, the high levels of predicted suitability expanded more widely than murres, to the west on the northern side of the island. These predictions match the density of at-sea observations of this species in this sector (Kuletz et al., 2020), probably mirroring the existence of large auklet colonies on the west side of the island (Piatt et al., 1988). Again, this indicates that at least a fraction of the auklets breeding on the north and west sides of the island, may largely distribute outside the ATBA during the breeding season (Figure 3; Figure 4), while this may not be true for the southern colonies.
Areas selected by the three bird species were characterized by chlorophyll blooms, high longitudinal current, as well as deeper depths and slightly colder waters. These characteristics are congruent with the oceanographic conditions associated with higher densities of foraging seabirds observed around St. Lawrence Island in recent years (Nishizawa et al., 2020), providing observational support for the model prediction that the area north of St. Lawrence Island is an important habitat for these three seabird species during the breeding season.
Our study did not provide a sufficient record to evaluate whether foraging black-legged kittiwakes distributing beyond the ATBA during the breeding season. The foraging range measured here for a single individual breeding at St. Lawrence Island appears very small compared to values recorded elsewhere in the region. For example, tracking studies on this species in the Pribilof Islands in the Bering Sea have repeatedly measured bimodal foraging ranges averaging 27.2 and 201.4 km, respectively, during the chick-rearing period (Paredes et al., 2014). While our results correspond with repeated field observations of flocks of birds feeding a few hundred meters from the island during the breeding season, it is likely they do not represent the full range of kittiwake foraging movements at this colony, as inferred from the isotopic niches occupied by kittiwakes breeding on St. Lawrence I. For instance, tracking data collected from breeding kittiwakes on Middleton Island in the Gulf of Alaska, also exhibit bimodal foraging ranges (Kotzerka et al., 2010): about half of the foraging trips recorded by GPS (n=7 among 15) ranged within 10 km of the breeding site (average 5.0 km, similarly to our study), but the others were 45.0 km on average (up to 92 km). At-sea surveys conducted concurrently with our at-colony work also indicate large numbers of kittiwakes (of unknown breeding status and colony of origin) during the breeding season outside the St. Lawrence Island ATBA (Nishizawa et al., 2020), hence suggesting potentially greater foraging ranges than what we measured from a single bird. However, in the absence of further quantitative evidence that kittiwakes breeding on St. Lawrence Island might forage outside of the current ATBA boundaries, we conservatively report that during chick-rearing at least a portion of their foraging trips occur within the current boundaries.
Despite fundamental differences in their at-sea ecology, the four studied species all highlighted the utilization of the shelf area immediately north-east of the island. For murres, the importance of this habitat was further emphasized by the high ratio of benthic prey in their diet, and coherently by a clear benthic-diving behaviour for the thick-billed murres. However, we argue that the importance of these benthic foraging areas to the St. Lawrence Island seabird community may not be as consistently important as it was suggested here.
First, murre diet observations were conducted in 2016 and 2017, which are considered relatively “warm” years (above the long-term survey mean) in the northern Bering Sea, with low winter sea-ice extent and warm summer water temperatures affecting the latitudinal distribution of high-biomass seabird prey species as well as their recruitment success (Stevenson and Lauth, 2019). This situation may have caused the low occurrence of pelagic fish in the diet observations, especially in 2017 which is considered the first extreme anomalous year in the recent regime shift (Huntington et al., 2020; Table 3), and this would in turn over-emphasize the importance of benthic habitats for murres. Common and thick-billed murres, as well as black-legged kittiwakes previously exhibited increased foraging on shelf-based prey during warm oceanographic conditions in the southeastern Bering Sea, in contrast to a higher reliance on pelagic prey during cold conditions (Will and Kitaysky, 2018). In this context, it is remarkable that 2017 is the only year in our habitat suitability model predictions where there is no clear segregation between the habitat suitability levels north and south of St. Lawrence Island (Supplementary Figure 1). This situation occurred at a time when common murres from this island showed a significant increase in their nutritional stress levels, and a shift in their isotopic niche indicating higher reliance on benthic prey coincided with a decline in the abundance of pelagic forage fish in the region (Will et al., 2020b). The other two summer seasons in our survey, 2018 and 2019, were even warmer in the northern area, and are among historical lows in winter sea-ice formation (Duffy-Anderson et al., 2019; Huntington et al., 2020). These years were associated with increased nutritional stress in all seabird species studied here (Will et al., 2020b). Hence, the recent regime shift to warmer conditions in the northern Bering Sea may cause additional nutritional stress to seabirds, with an observed response to further rely on benthic food sources for the common murre.
Second, multiple studies have shown that murres and kittiwakes from the Bering Sea exhibit variable foraging ranges in response to changes in food resources availability in their immediate environment. For instance, in years of low levels of local food resource, black-legged kittiwakes switched to substantially longer-distance foraging trips to the oceanic basin (Paredes et al., 2014). Similarly, thick-billed murres prolonged their foraging trip duration and enlarged foraging radius in years when they fed less on pelagic fish (Kokubun et al., 2018). This comparison, together with the result that sea-ice loss in the northern Bering Sea was associated with increased nutritional stress in all seabird species studied from St. Lawrence Island (Will et al., 2020b), indicates that seabirds from this island may also show variable foraging habitats and ranges (and thus, variable propension to reach outside the ATBA), in different food resource conditions. Considering the shift to a warm regime during our study years (Stevenson and Lauth, 2019; Huntington et al., 2020; Will et al., 2020b), and thus potentially to further reliance on benthic foraging habitat for murres, it is possible that the seabird community of St. Lawrence Island may have shown different foraging ranges than what may occur under other environmental regimes. Specifically, with the long-term trend to northward distribution shifts in marine benthic resources under the effect of regional warming (Grebmeier et al., 2015; Zhang et al., 2022), and a general reduction in the availability of Arctic Cod (Boreogadus saida) for seabirds in the region (apparently unavailable to murres in our study), it would be plausible that such unusually warm conditions may drive the St. Lawrence Island birds at-sea distribution further north too. However, none of the murre species’ foraging range increased from 2016 to 2019, and it even decreased in common murres. This indicates that these birds may be switching to benthic foraging, after reaching the limits of their ability to target north-shifting pelagic resources in the current warm regime. Thus, they may not be primarily benthic specialists, and instead may rely less on benthic resources during colder oceanographic regimes. Further tracking studies under different regimes would be needed to formally test this point and quantify how the foraging range variability may affect the exploitation of habitats beyond the ATBA.
The at-sea distribution of breeding murres and other seabirds including kittiwakes has been used as an indicator to quantify the impact of anthropogenic marine activities on the environment (e.g., Eastern Canadian Arctic: Gaston et al., 2013; southern North Sea: Peschko et al., 2020). Using tracking techniques, it has also been possible to guide the implementation of ATBAs based on distribution hotspots of seabird communities (e.g. in the South Atlantic: Requena et al., 2020). According to the seabird tracking data collected from St. Lawrence Island, the current ATBA boundaries do not fully encompass the marine habitats used by seabirds during the breeding season. Instead, the tracking data provide support for the initial boundaries based on recommendations from St. Lawrence Islanders (Huntington et al., 2019). During the review process at the IMO these boundaries were reduced for fear that a large ATBA would hinder navigation in the region, an argument used to reduce ATBA’s elsewhere as well (e.g., Peninsula de Osa off the Pacific coast of Costa Rica, Huntington et al., 2019). Specifically, the current ATBA polygon coordinates were chosen to accommodate for the neighboring shipping routes, as well as the limits of the contiguous Exclusive Economic Zones of the USA and Russia (Huntington et al., 2019). Based on the results presented here, in order to adequately protect important ecological and cultural marine resources, the ATBA around St. Lawrence Island should be extended further to the north-northeast, by about 35 km (18.9 nautical miles), to capture most of the predicted area of maximal habitat suitability (Figure 4). Such an extension would address the following shortcomings in the current ATBA boundaries.
First, the current ATBA design inadequately sets boundaries close to the island’s north-eastern shores, where most of the seabird breeding sites are located (Piatt et al., 1988; Figure 3), making it more likely that seabirds from these northern colonies may distribute beyond the ATBA. Moving this northern boundary further offshore, would maximize conservation benefits on these bird-rich northern shores which are a focal point for murre egg harvesting by the islanders (Will et al., 2020a).
Second, an extended ATBA would encompass the entirety of the high suitability (>90%) area predicted for the breeding seabird community that is immediately north of the current ATBA boundary. This high suitability area highlighted from our model relates mostly to alcids (murres and auklets), which, as diving birds, may be particularly susceptible to impacts from oiling (Wiese et al., 2004; Hedd et al., 2011), as they spend more time in contact with seawater (more so than the kittiwakes, King et al., 2021).
Besides, and more generally, the wider the regulation zone, the stronger the buffer effect to dilute acute threats (including noise, displacement, atmospheric pollution, oil, discharge) in a wildlife-rich area. Such an approach involving buffer zones along shipping routes has recently been suggested to better protect Arctic marine megafauna (Pirotta et al., 2019), and is particularly useful near areas with large aggregations of animals. Similarly, a general outcome of extending the ATBA into an area where shipping traffic is reduced may also improve safety at sea for local fishing activities operated from small boats (Huntington et al., 2015).
And finally, this highlighted area of maximal habitat suitability for seabirds is similar to an area of high ecological importance to other harvested resources (marine mammals, fish) computed for St. Lawrence Island in the absence of tracking data on seabirds (Krenz, 2014). Hence, this area is not specific to seabirds but is also an important one for fish and marine mammals, adding support to including this key region in the ATBA.
To balance marine conservation with shipping safety, a hybrid solution is to implement a dynamic ATBA (Huntington et al., 2019). In the present case, the most practical version of this would be a seasonal extension of the ATBA, as described above, such that during the seabird breeding season (May–August) foraging areas are well-protected, given that this same time-frame is when shipping traffic is likely to be the most intense (Hauser et al., 2018; Stevenson et al., 2019). Then, during the rest of the year when sailing conditions are more hazardous the boundaries would be reduced, giving ships more room to maneuver. Seasonal adjustments could also be made for the presence of spectacled eiders, whales and walruses in winter and early spring at the documented aggregation sites (Noongwook et al., 2007; Lovvorn et al., 2009; Grebmeier, 2012; Grebmeier et al., 2015). Alternatively, if a vessel management system were in place, a more adaptive ATBA could be implemented which would be able to account for the variability in habitat use indicated by the stable isotope standard ellipses in this study (Maxwell et al., 2015; Maxwell et al., 2020). Natural environmental variability and current transformations underway in the Pacific Arctic (Grebmeier et al., 2015; Stabeno and Bell, 2019; Huntington et al., 2020) may result in shifts in habitat suitability, corresponding to interannually different seabird distributions (e.g., Nishizawa et al., 2020; Kuletz et al., 2020). For instance, we suggest that a dynamic management of the St. Lawrence ATBA could be based on surface isotherms to reflect ecological habitat distributions (Stevenson and Lauth, 2019). Such mobile areas have been implemented effectively elsewhere and allow managers to quickly adjust boundaries based on near-real time information (hazardous sea-ice conditions for example, or quick feedback on recent ship strikes or other accidents; Maxwell et al., 2015; Huntington et al., 2019; Maxwell et al., 2020).
This study’s primary objective was to evaluate the effectiveness of the current St. Lawrence Island ATBA boundaries at separating vessel traffic from seabird foraging habitat. Here we identify the shortcomings of the study and discuss whether or how they may affect our ability to draw conclusions about seabird habitat use during the breeding season as it relates to the ATBA boundary north of St. Lawrence Island. Although our tracking data set is based on a small number of individuals, we repeatedly observed individuals from three species and across two breeding stages travel beyond the ATBA boundaries, indicating that birds from these northern colonies may come into contact with increased shipping-related threats in the region. To evaluate how representative these few individuals were of their respective species we used stable isotopic data, dive behavior and diet observations to complement our knowledge on the birds’ foraging niche. While these approaches do not directly translate into spatial distribution or inform us on the proportion of birds reaching beyond the ATBA boundaries; the details gained on habitat use at their foraging locations are crucial elements to evaluate population sensitivity to threats and ultimately to adjust conservation policies.
Representation of seabird colonies as well as seabird species may also be considered somewhat limited in our study. Foraging range may vary across colonies due to competition-induced effects, habitat availability, and colony size (e.g., Ballance et al., 2009; Gaston et al., 2013). Hence the island-wide extrapolations shown in Figure 3 should be taken with caution. However, by keeping the observed distribution radius constant across colonies, we highlight that the current ATBA’s design with inconsistent width from the shore is likely to create a discrepancy in the birds’ protection level across the island. We acknowledge that because we tracked seabirds from colonies situated on the island’s northern coasts only, our results do not necessarily imply that a similar proportion of birds reach outside the ATBA boundaries at all colonies around the island. Rather, we make the case that where the ATBA is the narrowest (northern side), it does not cover the relatively small at-sea distribution radius measured for three species during the breeding season. Thus, in line with this limitation in our sampling set-up, our recommendation to extend the ATBA applies only to the northern shore of the island. Although our study focused on some of the most numerous and culturally important species, several other species may remain to be studied to fully encompass the seabird community. These include other locally abundant species (e.g., the least auklet Aethia pusilla), or those that also have traditionally been or still are harvested by the islanders (pigeon guillemot Cepphus colomba, horned puffin Fratercula corniculata, pelagic cormorant, Phalacrocorax pelagicus; Naves, 2018). Thus, further tracking surveys may still be warranted, however smaller GPS tags would need to be developed (e.g., for least auklets), and the issue of small sample sizes, due both to low accessibility to the colony and high sensitivity of St. Lawrence birds to human disturbance, would still persist.
As sea ice is rapidly lost in the Arctic, the region is threatened by risks from emerging industrial activity, such as the development of commercial shipping traffic. Proactive strategies are necessary to safeguard the marine environment for the people and wildlife that rely on it. Implementing area-based management tools such as ATBAs based on ecological and culturally important wildlife areas requires reliable information on animals’ at-sea distribution (Hays et al., 2019). The scientific data from our study contributes concrete quantitative and conservative measurements of the St. Lawrence Island seabird community’s habitat use that were missing at the time of implementing the local ATBA. Based on these data and our modelling results, we recommend extending the northern boundary of this ATBA 35 km to the north-northeast. Due to the variability observed in isotopic niches and in environmental conditions during our study period, we further advise that a more dynamic approach to the St. Lawrence Island ATBA boundaries be considered. Depending on the region’s capacity these boundaries could be based on key environmental features monitored in real-time, or be seasonally proscriptive to maximize wildlife protection and minimize risks to ships. Regular re-evaluation of the boundaries should be undertaken given the rapid environmental changes occurring in this region.
The datasets presented in this study can be found in online repositories. The names of the repository/repositories and accession number(s) can be found below: ADS (Arctic Data archive System) of the National Institute of Polar Research:.
Behavioral and ecological data on seabirds in St. Lawrence Island, 2016: https://ads.nipr.ac.jp/data/meta/A20191227-002/
Behavioral and ecological data on seabirds in St. Lawrence Island, 2017:https://ads.nipr.ac.jp/data/meta/A20191227-003/
Behavioral and ecological data on seabirds in St. Lawrence Island, 2018:https://ads.nipr.ac.jp/data/meta/A20191227-004/
Behavioral and ecological data on seabirds in St. Lawrence Island, 2019:https://ads.nipr.ac.jp/data/meta/A20191227-005/.
The animal study was reviewed and approved by University of Alaska Fairbanks Institutional Animal Care and Use Committee protocol #471022, USFWS scientific collection permit #MB70337A, Alaska Department of Fish and Game’s permits #19–140, 18–131, 17–104, 16–089.
J-BT, AW, AK, and AT lead the writing. J-BT, AW, and AT conducted the analysis. AK, AT, and AW organized the funding. J-BT, AW, ST, AK, and AT conducted field work. All contributed writing and final edits.
This research was funded by the North Pacific Research Board (projects # 1410 & 1612, publication number 1612–5), the Arctic Challenge for Sustainability (ArCS) Project (Program Grant Number JPMXD1300000000) of Japan Ministry of Education, Culture, Sports, Science and Technology (MEXT), and Japan Society for the Promotion of Science KAKENHI Grant Number JP16H02705.
The authors declare that the research was conducted in the absence of any commercial or financial relationships that could be construed as a potential conflict of interest.
All claims expressed in this article are solely those of the authors and do not necessarily represent those of their affiliated organizations, or those of the publisher, the editors and the reviewers. Any product that may be evaluated in this article, or claim that may be made by its manufacturer, is not guaranteed or endorsed by the publisher.
The authors thank the Native Village of Savoonga, in particular Michael Toolie and Punguk Shoogukwruk who greatly helped with all colony-based sampling. Wendi Pillars, supported by the Arctic Research Consortium of the United States’ PolarTREC program, Larisa Kava, David Akeya, Carl Burnside and Lucy Kingeekuk also assisted with field work. Lodging was provided by the Alowa, Akeya, and Pelowook families. J-BT also thanks Ben Raymond for his advices on data analysis.
The Supplementary Material for this article can be found online at: https://www.frontiersin.org/articles/10.3389/fmars.2022.875541/full#supplementary-material
Supplementary Figure 1 | Results of the second Principal Component Analysis in the MADIFA computed from bird tracks for each dataset (species: COMU: common murre, TBMU: thick-billed murre, CRAU: crested auklet; and year and breeding stage). Results show % of variance explained by the two first principal components, and correlation coefficients between the MADIFA components and the variables (bathymetry, bathymetry gradient, sea surface temperature, sea surface chlorophyll concentration, and zonal and meridional current velocity). In each case, the two variables with the highest scores are highlighted in red. A map with projected habitat suitability (expressed as %; the boundaries of the ATBA around St Lawrence Island is shown in black lines); and histograms of the available (open bars) versus utilized (shaded bars) range of relative values for each environmental variable are presented for each species and stage.
Supplementary Figure 2 | A flowchart detailing the steps followed in this study from the case-by-case habitat suitability models to the final projections accounting for all studied species, years and breeding stages.
Aksenov Y., Popova E. E., Yool A., Nurser A. G., Williams T. D., Bertino L., et al. (2017). On the Future Navigability of Arctic Sea Routes: High-Resolution Projections of the Arctic Ocean and Sea Ice. Mar. Policy 75, 300–317. doi: 10.1016/j.marpol.2015.12.027
Ballance L. T., Ainley D. G., Ballard G., Barton K. (2009). An Energetic Correlate Between Colony Size and Foraging Effort in Seabirds, an Example of the Adélie Penguin Pygoscelis Adeliae. J. Avian Biol. 40 (3), 279–288. doi: 10.1111/j.1600-048X.2008.04538.x
Barger C. P., Young R. C., Will A., Ito M., Kitaysky A. S. (2016). Resource Partitioning Between Sympatric Seabird Species Increases During Chick-Rearing. Ecosphere 7, 1–15. doi: 10.1002/ecs2.1447
Bayer R. D. (1983). Black-Legged Kittiwake Feeding Flocks in Alaska: Selfish Reciprocal Altruistic Flocks? J. Field Ornithol. 52, 196–199.
Benvenuti S., Dall'Antonia L., Falk K. (2002). Diving Behaviour Differs Between Incubating and Brooding Brünnich's Guillemots, Uria Lomvia. Polar. Biol. 25, 474–478. doi: 10.1007/s00300-002-0372-0
Cairns D. K., Bredin K. A., Montevecchi W. A. (1987). Activity Budgets and Foraging Ranges of Breeding Common Murres. Auk 104 (2), 218–224. doi: 10.1093/auk/104.2.218
Calenge C., Darmon G., Basille M., Loison A., Jullien J. M. (2008). The Factorial Decomposition of the Mahalanobis Distances in Habitat Selection Studies. Ecology 89, 555–566. doi: 10.1890/06-1750.1
Danielson S. L., Weingartner T. W., Hedstrom K., Aagaard K., Woodgate R., Curchitser E., et al. (2014). Coupled Wind-Forced Controls of the Bering–Chukchi Shelf Circulation and the Bering Strait Through-Flow: Ekman Transport, Continental Shelf Waves, and Variations of the Pacific-Arctic Sea Surface Height Gradient. Prog. Oceanogr. 125, 40–61. doi: 10.1016/j.pocean.2014.04.006
Dehnhard N., Skei J., Christensen-Dalsgaard S., May R., Halley D., Ringsby T. H., et al. (2020). Boat Disturbance Effects on Moulting Common Eiders Somateria Mollissima. Mar. Biol. 167, 1–11. doi: 10.1007/s00227-019-3624-z
Delord K., Barbraud C., Pinaud D., Letournel B., Jaugeon B., Goraguer H., et al. (2020). Movements of Three Alcid Species Breeding Sympatrically in Saint Pierre and Miquelon, Northwestern Atlantic Ocean. J. Ornithol. 161, 359–371. doi: 10.1007/s10336-019-01725-z
Duffy-Anderson J. T., Stabeno P., Andrews A. G. III, Cieciel K., Deary A., Farley E., et al. (2019). Responses of the Northern Bering Sea and Southeastern Bering Sea Pelagic Ecosystems Following Record-Breaking Low Winter Sea Ice. Geophys. Res. Lett. 46, 9833–9842. doi: 10.1029/2019GL083396
Fall J. A., Braem N. S., Brown C. L., Hutchinson-Scarbrough L. B., Koster D. S., Krieg T. M. (2013). Continuity and Change in Subsistence Harvests in Five Bering Sea Communities: Akutan, Emmonak, Savoonga, St. Paul, and Togiak. Deep. Sea. Res. Part II. 94, 274–291. doi: 10.1016/j.dsr2.2013.03.010
Gall A. E., Roby D. D., Irons D. B., Rose I. C. (2006). Differential Response in Chick Survival to Diet in Least and Crested Auklets. Mar. Ecol. Prog. Ser. 308, 279–291. doi: 10.3354/meps308279
Gaston A. J., Elliott K. H., Ropert-Coudert Y., Kato A., Macdonald C. A., Mallory M. L., et al. (2013). Modeling Foraging Range for Breeding Colonies of Thick-Billed Murres Uria Lomvia in the Eastern Canadian Arctic and Potential Overlap With Industrial Development. Biol. Conserv. 168, 134–143. doi: 10.1016/j.biocon.2013.09.018
Grebmeier J. M. (2012). Shifting Patterns of Life in the Pacific Arctic and Sub-Arctic Seas. Annu. Rev. Mar. Sci. 4, 63–78. doi: 10.1146/annurev-marine-120710-100926
Grebmeier J. M., Bluhm B. A., Cooper L. W., Danielson S. L., Arrigo K. R., Blanchard A. L., et al. (2015). Ecosystem Characteristics and Processes Facilitating Persistent Macrobenthic Biomass Hotspots and Associated Benthivory in the Pacific Arctic. Prog. Oceanogr. 136, 92–114. doi: 10.1016/j.pocean.2015.05.006
Grebmeier J. M., Cooper L. W., Feder H. M., Sirenko B. I. (2006). Ecosystem Dynamics of the Pacific-Influenced Northern Bering and Chukchi Seas in the Amerasian Arctic. Prog. Oceanogr. 71, 331–361. doi: 10.1016/j.pocean.2006.10.001
Gulka J., Ronconi R. A., Davoren G. K. (2019). Spatial Segregation Contrasting Dietary Overlap: Niche Partitioning of Two Sympatric Alcids During Shifting Resource Availability. Mar. Biol. 166, 1–17. doi: 10.1007/s00227-019-3553-x
Harding A., Paredes R., Suryan R., Roby D., Irons D., Orben R., et al. (2013). Does Location Really Matter? An Inter-Colony Comparison of Seabirds Breeding at Varying Distances From Productive Oceanographic Features in the Bering Sea. Deep. Sea. Res. Part II. 94, 178–191. doi: 10.1016/j.dsr2.2013.03.013
Harris P. T., Macmillan-Lawler M., Kullerud L., Rice J. C. (2018). Arctic Marine Conservation is Not Prepared for the Coming Melt. ICES J. Mar. Sci. 75, 61–71. doi: 10.1093/icesjms/fsx153
Hatch S. A., Meyers P. M., Mulcahy D. M., Douglas D. C. (2000). Seasonal Movements and Pelagic Habitat Use of Murres and Puffins Determined by Satellite Telemetry. Condor 102, 145–154. doi: 10.1093/condor/102.1.145
Hauser D. D., Laidre K. L., Stern H. L. (2018). Vulnerability of Arctic Marine Mammals to Vessel Traffic in the Increasingly Ice-Free Northwest Passage and Northern Sea Route. Proc. Natl. Acad. Sci. U.S.A. 115, 7617–7622. doi: 10.1073/pnas.1803543115
Hays G. C., Bailey H., Bograd S. J., Bowen W. D., Campagna C., Carmichael R. H., et al. (2019). Translating Marine Animal Tracking Data Into Conservation Policy and Management. Trends Ecol. Evol. 34, 459–473. doi: 10.1016/j.tree.2019.01.009
Hedd A., Montevecchi W. A., McFarlane Tranquilla L., Burke C. M., Fifield D. A., Robertson G. J., et al. (2011). Reducing Uncertainty on the Grand Bank: Tracking and Vessel Surveys Indicate Mortality Risks for Common Murres in the North-West Atlantic. Anim. Conserv. 14, 630–641. doi: 10.1111/j.1469-1795.2011.00479.x
Hobson K. A., Clark R. G. (1993). Turnover of 13C in Cellular and Plasma Fractions of Blood: Implications for Nondestructive Sampling in Avian Dietary Studies. Auk 110, 638–641. doi: 10.2307/4088430
Huffeldt N. P., Linnebjerg J. F., Fort J., Merkel F. R., Frederiksen M. (2021). Habitat When Foraging Does Not Explain Temporal Segregation by Sex in a Breeding Seabird. Mar. Biol. 168, 1–18. doi: 10.1007/s00227-021-03958-0
Humphries G. R., Huettmann F. (2014). Putting Models to a Good Use: A Rapid Assessment of Arctic Seabird Biodiversity Indicates Potential Conflicts With Shipping Lanes and Human Activity. Divers. Distrib. 20, 478–490. doi: 10.1111/ddi.12177
Hunt J. G.L., Harrison N. M., Cooney R. T. (1990). The Influence of Hydrographic Structure and Prey Abundance on Foraging of Least Auklets. Stud. Avian Biol. 14, 7–22.
Hunt J. G.L., Stabeno P., Walters G., Sinclair E., Brodeur R. D., Napp J. M., et al. (2002). Climate Change and Control of the Southeastern Bering Sea Pelagic Ecosystem. Deep. Sea. Res. Part II. 49, 5821–5853. doi: 10.1016/S0967-0645(02)00321-1
Huntington H. P., Bobbe S., Hartsig A., Knight E. J., Knizhnikov A., Moiseev A., et al. (2019). The Role of Areas to be Avoided in the Governance of Shipping in the Greater Bering Strait Region. Mar. Policy 110, 103564. doi: 10.1016/j.marpol.2019.103564
Huntington H. P., Daniel R., Hartsig A., Harun K., Heiman M., Meehan R., et al. (2015). Vessels, Risks, and Rules: Planning for Safe Shipping in Bering Strait. Mar. Policy 51, 119–127. doi: 10.1016/j.marpol.2014.07.027
Huntington H. P., Danielson S. L., Wiese F. K., Baker M., Boveng P., Citta J. J., et al. (2020). Evidence Suggests Potential Transformation of the Pacific Arctic Ecosystem is Underway. Nat. Clim. Change 10, 342–348. doi: 10.1038/s41558-020-0695-2
International Council on Clean Transportation (2015). A 10-Year Projection of Maritime Activity in the US Arctic Region (Washington DC, USA: US Committee on the Marine Transportation System).
Jackson A. L., Inger R., Parnell A. C., Bearhop S. (2011). Comparing Isotopic Niche Widths Among and Within Communities: SIBER-Stable Isotope Bayesian Ellipses in R. J. Anim. Ecol. 80, 595–602. doi: 10.1111/j.1365-2656.2011.01806.x
King M. D., Elliott J. E., Williams T. D. (2021). Effects of Petroleum Exposure on Birds: A Review. Sci. Total. Environ. 755, 142834. doi: 10.1016/j.scitotenv.2020.142834
Kokubun N., Takahashi A., Paredes R., Young R. C., Sato N. N., Yamamoto T., et al. (2018). Inter-Annual Climate Variability Affects Foraging Behavior and Nutritional State of Thick-Billed Murres Breeding in the Southeastern Bering Sea. Mar. Ecol. Prog. Ser. 593, 195–208. doi: 10.3354/meps12365
Kokubun N., Yamamoto T., Kikuchi D. M., Kitaysky A., Takahashi A. (2015). Nocturnal Foraging by Red-Legged Kittiwakes, a Surface Feeding Seabird That Relies on Deep Water Prey During Reproduction. PLos One 10, e0138850. doi: 10.1371/journal.pone.0138850
Kokubun N., Yamamoto T., Sato N., Watanuki Y., Will A., Kitaysky A. S., et al. (2016). Foraging Segregation of Two Congeneric Diving Seabird Species Breeding on St. George Island, Bering Sea. Biogeosciences 13, 2579–2591. doi: 10.5194/bg-13-2579-2016
Kotzerka J., Garthe S., Hatch S. A. (2010). GPS Tracking Devices Reveal Foraging Strategies of Black-Legged Kittiwakes. J. Ornithol. 151, 459–467. doi: 10.1007/s10336-009-0479-y
Krenz C. (2014). Bering Strait Marine Life and Subsistence Use Data Synthesis (Juneau, USA: Oceana and Kawerak).
Kuletz K., Cushing D., Labunski E. (2020). Distributional Shifts Among Seabird Communities of the Northern Bering and Chukchi Seas in Response to Ocean Warming During 2017–2019. Deep. Sea. Res. Part II. 181, 104913. doi: 10.1016/j.dsr2.2020.104913
Kuletz K. J., Ferguson M. C., Hurley B., Gall A. E., Labunski E. A., Morgan T. C. (2015). Seasonal Spatial Patterns in Seabird and Marine Mammal Distribution in the Eastern Chukchi and Western Beaufort Seas: Identifying Biologically Important Pelagic Areas. Prog. Oceanogr. 136, 175–200. doi: 10.1016/j.pocean.2015.05.012
Lascelles B. G., Taylor P. R., Miller M. G. R., Dias M. P., Oppel S., Torres L., et al. (2016). Applying Global Criteria to Tracking Data to Define Important Areas for Marine Conservation. Divers. Distrib. 22, 422–431. doi: 10.1111/ddi.12411
Lovvorn J. R., Grebmeier J. M., Cooper L. W., Bump J. K., Richman S. E. (2009). Modeling Marine Protected Areas for Threatened Eiders in a Climatically Changing Bering Sea. Ecol. Appl. 19, 1596–1613. doi: 10.1890/08-1193.1
Maxwell S. M., Gjerde K. M., Conners M. G., Crowder L. B. (2020). Mobile Protected Areas for Biodiversity on the High Seas. Science 367, 252–254. doi: 10.1126/science.aaz9327
Maxwell S. M., Hazen E. L., Lewison R. L., Dunn D. C., Bailey H., Bograd S. J., et al. (2015). Dynamic Ocean Management: Defining and Conceptualizing Real-Time Management of the Ocean. Mar. Policy 58, 42–50. doi: 10.1016/j.marpol.2015.03.014
Naves L. C. (2018). Geographic and Seasonal Patterns of Seabird Subsistence Harvest in Alaska. Polar. Biol. 41, 1217–1236. doi: 10.1007/s00300-018-2279-4
Nishizawa B., Yamada N., Hayashi H., Wright C., Kuletz K., Ueno H., et al. (2020). Timing of Spring Sea-Ice Retreat and Summer Seabird-Prey Associations in the Northern Bering Sea. Deep. Sea. Res. Part II. 181, 104898. doi: 10.1016/j.dsr2.2020.104898
Noongwook G., Native Village of Savoonga, Native Village of Gambell, Huntington H. P., George J. C. (2007). Traditional Knowledge of the Bowhead Whale (Balaena Mysticetus) Around St. Lawrence Island, Alaska. Arctic 60, 47–54.
Oppel S., Bolton M., Carneiro A. P., Dias M. P., Green J. A., Masello J. F., et al. (2018). Spatial Scales of Marine Conservation Management for Breeding Seabirds. Mar. Policy 98, 37–46. doi: 10.1016/j.marpol.2018.08.024
Orians G. H., Pearson N. E. (1979). “On the Theory of Central Place Foraging”, in Analysis of Ecological Systems. Eds. Horn D. J., Mitchell R. D., Stairs G. R. (Columbus, OH: Ohio State Univ. Press), 154–177.
Paredes R., Orben R. A., Roby D. D., Irons D. B., Young R., Renner H., et al. (2015). Foraging Ecology During Nesting Influences Body Size in a Pursuit-Diving Seabird. Mar. Ecol. Prog. Ser. 533, 261–276. doi: 10.3354/meps11388
Paredes R., Orben R. A., Suryan R. M., Irons D. B., Roby D. D., Harding A. M., et al. (2014). Foraging Responses of Black-Legged Kittiwakes to Prolonged Food-Shortages Around Colonies on the Bering Sea Shelf. PLos One 9, e92520. doi: 10.1371/journal.pone.0092520
Peschko V., Mendel B., Müller S., Markones N., Mercker M., Garthe S. (2020). Effects of Offshore Windfarms on Seabird Abundance: Strong Effects in Spring and in the Breeding Season. Mar. Environ. Res. 162, 105157. doi: 10.1016/j.marenvres.2020.105157
Piatt J. F., Hatch S. A., Roberts B. D., Lidster W. W., Wells J. L., Haney J. C. (1988). Populations, Productivity, and Feeding Habits of Seabirds on St. Lawrence Island, Alaska (Alaska Fish and Wildlife Research Center: US Fish and Wildlife Service).
Piatt J. F., Springer A. M. (2003). Advection, Pelagic Food Webs and the Biogeography of Seabirds in Beringia. Mar. Ornithol. 31, 141–154.
Pirotta V., Grech A., Jonsen I. D., Laurance W. F., Harcourt R. G. (2019). Consequences of Global Shipping Traffic for Marine Giants. Front. Ecol. Environ. 17, 39–47. doi: 10.1002/fee.1987
R Core Team (2022). R: A Language and Environment for Statistical Computing (Vienna, Austria: R Foundation for Statistical Computing). Available at: https://www.R-project.org/.
Requena S., Oppel S., Bond A. L., Hall J., Cleeland J., Crawford R. J., et al. (2020). Marine Hotspots of Activity Inform Protection of a Threatened Community of Pelagic Species in a Large Oceanic Jurisdiction. Anim. Conserv. 23 (5), 585–596. doi: 10.1111/acv.12572
Sheffield Guy L. M., Roby D. D., Gall A. E., Irons D. B., Rose I. C. (2009). The Influence of Diet and Ocean Conditions on Productivity of Auklets on St. Lawrence Island, Alaska. Mar. Ornithol. 37, 227–236.
Smith L. C., Stephenson S. R. (2013). New Trans-Arctic Shipping Routes Navigable by Midcentury. Proc. Natl. Acad. Sci. U.S.A. 110, E1191–E1195. doi: 10.1073/pnas.1214212110
Springer A. M., Murphy E. C., Roseneau D. G., McRoy C. P., Cooper B. A. (1987). The Paradox of Pelagic Food Webs in the Northern Bering Sea—I. Seabird Food Habits. Cont. Shelf. Res. 7, 895–911. doi: 10.1016/0278-4343(87)90005-7
Stabeno P. J., Bell S. W. (2019). Extreme Conditions in the Bering Sea, (2017–2018): Record-Breaking Low Sea-Ice Extent. Geophys. Res. Lett. 46, 8952–8959. doi: 10.1029/2019GL083816
Stevenson T. C., Davies J., Huntington H. P., Sheard W. (2019). An Examination of Trans-Arctic Vessel Routing in the Central Arctic Ocean. Mar. Policy 100, 83–89. doi: 10.1016/j.marpol.2018.11.031
Stevenson D. E., Lauth R. R. (2019). Bottom Trawl Surveys in the Northern Bering Sea Indicate Recent Shifts in the Distribution of Marine Species. Polar. Biol. 42, 407–421. doi: 10.1007/s00300-018-2431-1
Takahashi A., Matsumoto K., Hunt G. L. Jr., Shultz M. T., Kitaysky A. S., Sato K., et al. (2008). Thick-Billed Murres Use Different Diving Behaviors in Mixed and Stratified Waters. Deep. Sea. Res. Part II. 55, 1837–1845. doi: 10.1016/j.dsr2.2008.04.005
Tempel J. T. L., Wise S., Osborne T. Q., Sparks K., Atkinson S. (2021). Life Without Ice: Perceptions of Environmental Impacts on Marine Resources and Subsistence Users of St. Lawrence Island. Ocean Coast. Manage. 212, 105819. doi: 10.1016/j.ocecoaman.2021.105819
Thaxter C. B., Lascelles B., Sugar K., Cook A. S., Roos S., Bolton M., et al. (2012). Seabird Foraging Ranges as a Preliminary Tool for Identifying Candidate Marine Protected Areas. Biol. Conserv. 156, 53–61. doi: 10.1016/j.biocon.2011.12.009
Thiers L., Delord K., Bost C. A., Guinet C., Weimerskirch H. (2017). Important Marine Sectors for the Top Predator Community Around Kerguelen Archipelago. Polar. Biol. 40, 365–378. doi: 10.1007/s00300-016-1964-4
Tsoar A., Allouche O., Steinitz O., Rotem D., Kadmon R. (2007). A Comparative Evaluation of Presence-Only Methods for Modelling Species Distribution. Divers. Distrib. 13, 397–405. doi: 10.1111/j.1472-4642.2007.00346.x
Wiese F. K., Robertson G. J., Gaston A. J. (2004). Impacts of Chronic Marine Oil Pollution and the Murre Hunt in Newfoundland on Thick-Billed Murre Uria Lomvia Populations in the Eastern Canadian Arctic. Biol. Conserv. 116, 205–216. doi: 10.1016/S0006-3207(03)00191-5
Will A. P., Kitaysky A. S. (2018). Variability in Trophic Level and Habitat Use in Response to Environmental Forcing: Isotopic Niche Dynamics of Breeding Seabirds in the Southeastern Bering Sea. Mar. Ecol. Prog. Ser. 593, 247–260. doi: 10.3354/meps12471
Will A., Takahashi A., Thiebot J. B., Martinez A., Kitaiskaia E., Britt L., et al. (2020b). The Breeding Seabird Community Reveals That Recent Sea Ice Loss in the Pacific Arctic Does Not Benefit Piscivores and is Detrimental to Planktivores. Deep. Sea. Res. Part II. 181, 104902. doi: 10.1016/j.dsr2.2020.104902
Will A., Thiebot J. B., Ip H. S., Shoogukwruk P., Annogiyuk M., Takahashi A., et al. (2020a). Investigation of the 2018 Thick-Billed Murre (Uria Lomvia) Die-Off on St. Lawrence Island Rules Out Food Shortage as the Cause. Deep. Sea. Res. Part II. 181, 104879. doi: 10.1016/j.dsr2.2020.104879
Wong S. N., Gjerdrum C., Gilchrist H. G., Mallory M. L. (2018). Seasonal Vessel Activity Risk to Seabirds in Waters Off Baffin Island, Canada. Ocean Coast. Manage. 163, 339–351. doi: 10.1016/j.ocecoaman.2018.07.004
Yumashev D., van Hussen K., Gille J., Whiteman G. (2017). Towards a Balanced View of Arctic Shipping: Estimating Economic Impacts of Emissions From Increased Traffic on the Northern Sea Route. Clim. Change 143, 143–155. doi: 10.1007/s10584-017-1980-6
Zhang R., Song P., Li H., Wang R., Li Y., Miao X., et al. (2022). Spatio-Temporal Characteristics of Demersal Fish Community in the Chukchi and Northern Bering Seas: Significant Distributional Records and Interannual Variations in Species Composition and Biodiversity. Polar. Biol. 45, 259–273. doi: 10.1007/s00300-021-02980-8
Keywords: Arctic, warming, GPS tracking, habitat suitability, marine spatial planning, alcids, kittiwake, subsistence harvesting
Citation: Thiebot J-B, Will AP, Tsukamoto S, Kitaysky AS and Takahashi A (2022) The Designated Shipping Avoidance Area Around St. Lawrence Island, Northern Bering Sea, Is not Sufficient to Protect Foraging Habitat of the Island’s Breeding Seabird Community. Front. Mar. Sci. 9:875541. doi: 10.3389/fmars.2022.875541
Received: 14 February 2022; Accepted: 17 May 2022;
Published: 13 June 2022.
Edited by:
Ryan Rudolf Reisinger, University of Southampton, United KingdomReviewed by:
David Ainley, H.T. Harvey & Associates, United StatesCopyright © 2022 Thiebot, Will, Tsukamoto, Kitaysky and Takahashi. This is an open-access article distributed under the terms of the Creative Commons Attribution License (CC BY). The use, distribution or reproduction in other forums is permitted, provided the original author(s) and the copyright owner(s) are credited and that the original publication in this journal is cited, in accordance with accepted academic practice. No use, distribution or reproduction is permitted which does not comply with these terms.
*Correspondence: Jean-Baptiste Thiebot, amJ0aGllYm90QGZpc2guaG9rdWRhaS5hYy5qcA==
†Present address: Jean-Baptiste Thiebot, Graduate School of Fisheries Sciences, Hokkaido University, Hakodate, Japan
Disclaimer: All claims expressed in this article are solely those of the authors and do not necessarily represent those of their affiliated organizations, or those of the publisher, the editors and the reviewers. Any product that may be evaluated in this article or claim that may be made by its manufacturer is not guaranteed or endorsed by the publisher.
Research integrity at Frontiers
Learn more about the work of our research integrity team to safeguard the quality of each article we publish.