- 1Alfred Wegener Institute, Helmholtz Centre for Polar and Marine Research, Bremerhaven, Germany
- 2School of Biological and Marine Sciences, University of Plymouth, Plymouth, United Kingdom
- 3Department of Algal Development and Evolution, Max Planck Institute for Biology Tübingen, Tübingen, Germany
- 4Applied Ecology and Phycology, University of Rostock, Rostock, Germany
- 5Division of Biotechnology and Plant Health, Norwegian Institute of Bioeconomy Research (NIBIO), Ås, Norway
- 6Biomarine Resource Valorisation, Division of Food Production and Society, Norwegian Institute of Bioeconomy Research (NIBIO), Bodø, Norway
- 7The Marine Science Institute, College of Science, University of the Philippines, Quezon City, Philippines
Kelp forests in the North Atlantic are at risk of decline at their warm temperature distribution margins due to anthropogenic temperature rise and more frequent marine heat waves. To investigate the thermal adaptation of the cold-temperate kelp Laminaria digitata, we sampled six populations, from the Arctic to Brittany (Spitsbergen, Tromsø, Bodø [all Norway], Helgoland [Germany], Roscoff and Quiberon [both France]), across the species’ entire distribution range, spanning 31.5° latitude and 12-13°C difference in mean summer sea surface temperature. We used pooled vegetative gametophytes derived from several sporophytes to approximate the genetic diversity of each location. Gametophytes were exposed to (sub-) lethal high (20-25°C) and (sub-) optimal low (0-15°C) temperature gradients in two full-factorial, common-garden experiments, subjecting subsets of populations from different origins to the same conditions. We assessed survival of gametophytes, their ability to develop microscopic sporophytes, and subsequent growth. We hypothesized that the thermal performance of gametophytes and microscopic sporophytes corresponds to their local long-term thermal history. Integrated gametophyte survival revealed a uniform upper survival temperature (UST) of 24°C among five tested populations (Tromsø to Quiberon). In contrast, following two weeks of thermal priming of gametophytes at 20-22°C, sporophyte formation at 15°C was significantly higher in southern populations (Quiberon and Roscoff) compared to the high-latitude population of Tromsø. Between 0-15°C, survival of the Arctic population (Spitsbergen) was negatively correlated with increasing temperatures, while the southern-most population (Quiberon) showed the opposite. Thus, responses of survival at low, and sporophyte formation at high temperatures, support the concept of local adaption. On the other hand, sporophyte formation between 0-15°C peaked at 6-9°C in the Quiberon and at 9-12°C in the Spitsbergen population. Sporophyte growth rates (GR) both in length and width were similar for Spitsbergen, Tromsø and Quiberon; all had maximum GRs at 12-15°C and low GRs at 0-6°C. Therefore, responses of sporophyte formation and growth at low temperatures do not reflect ecotypic adaptation. We conclude that L. digitata populations display trait-dependent adaptation, partly corresponding to their local temperature histories and partly manifesting uniform or unpredictable responses. This suggests differential selection pressures on the ontogenetic development of kelps such as L. digitata.
1 Introduction
Kelp forests are complex, biodiverse, and highly productive ecosystems that occur globally between warm-temperate and polar regions (Teagle et al., 2017), and in deep tropical waters (Graham et al., 2007). Even though they provide important ecosystem services, their socio-economic value is only starting to be recognized (e.g., Vásquez et al., 2014; Blamey and Bolton, 2018; Eger et al., 2021). Kelp forests benefit humankind directly (e.g., kelp harvesting, fishing, tourism), indirectly (e.g., habitat provision, climate regulation, nutrient cycling, carbon capture), and through cultural and spiritual value itself (biodiversity, recreation, science; Filbee-Dexter and Wernberg, 2018). A key, habitat-forming kelp is the digitate brown alga Laminaria digitata, which occurs on the upper subtidal rocky shore in cold-temperate to Arctic regions of the North Atlantic (Lüning, 1990). Its distribution is limited between Spitsbergen (Norway) in the north, and southern Brittany (France) in the south, but it also inhabits the western North Atlantic between the northeast coast of the US and Greenland (Lüning, 1990; Assis et al., 2017a). It therefore occurs in a broad range of temperatures stretching from the 0°C February to the 18°C August sea-surface isotherm (Müller et al., 2009).
Various abiotic factors influence the life cycle and productivity of kelps; however, temperature is one of the major factors determining their biogeographical distribution (Lüning, 1984; Lüning, 1990; Adey and Steneck, 2001). Laminaria digitata displays a haplo-diplontic, heteromorphic life cycle with an alternation between diploid, macroscopic sporophytes and haploid, microscopic gametophytes, where the latter are dioecious and sexually dimorphic (van den Hoek et al., 1995). Physiological processes differ in their thermal characteristics between ontogenetic stages, but both macro- and microscopic stages are similar in that they reveal a wider temperature range for growth than for reproduction (e.g., tom Dieck (Bartsch), 1992; Bartsch et al., 2013). The sporophytic phase of L. digitata shows maximal growth at 10-15°C (tom Dieck (Bartsch), 1992) and reproduces best at 5-10°C (Bartsch et al., 2013), whereas gametophyte growth is greatest at 15-18°C (Lüning, 1980) and gametogenesis best at 5-15°C (Martins et al., 2017). Ocean warming could thus negatively impact growth and photosynthesis of kelps (Roleda, 2009; Roleda, 2016), may result in delayed sporophyte recruitment due to prolonged vegetative growth of gametophytes, and could even lead to inhibition of reproduction and reduction in geographic range of the species (Oppliger et al., 2012).
In low nutrient and irradiance (white or red light) laboratory settings, gametophytes have, in most cases, survived and grown vegetatively for several months to decades and reproduced sexually when they were returned to optimal conditions (tom Dieck (Bartsch), 1993; Carney and Edwards, 2010; Silva et al., 2022). The ability to maintain such microscopic seed banks (Edwards, 2000) underlines the principal role that gametophytes play in controlling the species’ distribution and survival (Edwards, 2022). This survival mechanism can be vital at warm distribution margins, after prolonged summer heat stress, or after extreme thermal fluctuations (Ladah and Zertuche-González, 2007; Barradas et al., 2011). The exact contribution of the gametophyte phase to the persistence of kelp forests, however, remains partially speculative due to the difficulty of studying gametophytes in situ (Santelices et al., 1995; Destombe and Oppliger, 2011; Edwards, 2022).
Polar and cold-temperate regions have been heavily affected by anthropogenic climate change (Jueterbock et al., 2013; Filbee-Dexter et al., 2019) with mean sea surface temperature (SST) increases of ~0.11°C per decade between 1971 to 2010 (IPCC, 2014). Accordingly, SST isotherms have shifted predominantly poleward by 30-100 km per decade (Hansen et al., 2006). Future projections indicate further isotherm shifts of up to 600 km northwards (Hansen et al., 2006) and rising annual average SSTs of up to 3-4°C by 2100 (IPCC, 2014). Many coastal organisms, including kelps, will struggle to shift their ranges fast enough to match the current rapid warming projections, given their limited dispersal ability (Merzouk and Johnson, 2011; Assis et al., 2017b). More importantly, the increasing occurrence and intensity of marine heat waves may prove to be even more problematic for kelps (Smale et al., 2017; Filbee-Dexter et al., 2020; Oliver et al., 2021). Kelps respond differently, depending on the duration and magnitude of the heat stress (Eggert, 2012), but are vulnerable to temperature fluctuations that surpass their upper survival threshold for more than a day, particularly toward low-latitude range edges (Dayton and Tegner, 1984; Smale and Wernberg, 2013). Several large-scale range shifts have already been documented in the Northeast Atlantic for various kelp species such as Saccharina latissima in Norway (Moy and Christie, 2012) and Laminaria ochroleuca in southern Britain (Smale et al., 2015). In Spain, Saccorhiza polyschides, L. ochroleuca and Laminaria hyperborea have experienced severe regional contractions (Fernández, 2011; Voerman et al., 2013). A progressive disappearance of L. digitata has also been described in France as early as 1999 (Cosson, 1999), and on Helgoland, considerable biomass loss of L. digitata in the shallow sub-tidal was observed between 1968 and 2005 (Pehlke and Bartsch, 2008). Ecological niche modelling (ENM) and recent analysis of spatio-temporal distribution have shown and predicted northward range shifts of kelps, including L. digitata populations (Müller et al., 2009; Raybaud et al., 2013; Assis et al., 2017a; Assis et al., 2017b; Krause-Jensen et al., 2020).
Even though projected shifts in distribution patterns frequently presume that individuals of a species respond uniformly to climate change, there is evidence for intraspecific variation among populations of kelps (King et al., 2018; King et al., 2019; Liesner et al., 2020a; Becheler et al., 2022). In S. latissima, local mortality thresholds of sporophytes differ by ~3°C between populations from the NE and NW Atlantic, so single temperature tolerances should not be assumed to apply over an entire species’ distribution (Filbee-Dexter et al., 2020). Populations at the warm range edge are expected to contain unique genetic diversity that was maintained across glacial cycles (Maggs et al., 2008; Assis et al., 2017a), which may provide them with high physiological versatility and resilience (Wernberg et al., 2018), but these are especially threatened in the face of climate change. Furthermore, species with broad spatial distributions, occupying a range of thermal niches, may also have evolved unique genetic and phenotypic characteristics (Martins et al., 2020; Becheler et al., 2022). One of the best methods to study adaptive changes along latitudinal gradients are common-garden experiments (King et al., 2018; Martins et al., 2020; Becheler et al., 2022), in which material from different populations is subjected to the same environment, regardless of the geographical and environmental origin of populations. However, rather than dealing with multiple parental strains to yield substantial genetic representation, past studies have primarily used single clonal strains, but this has changed lately (e.g., Liesner et al., 2020a; Liesner et al., 2020b; Becheler et al., 2022; Gauci et al., 2022).
In this study, we investigated whether L. digitata gametophyte progeny derived from multiple local sporophytes from across their entire latitudinal distribution range are locally adapted to their long-term temperature history. Bearing in mind that L. digitata populations are genetically structured into a northern and southern clade (Liesner et al., 2020a; Neiva et al., 2020), we hypothesized that gametophytes derived from southern populations, which are regularly subjected to high summer temperatures ≥18°C (Quiberon, Roscoff [both France], and Helgoland [Germany]), will perform better at high temperatures, while gametophytes from northern populations, occasionally exposed to summer temperatures >8-12°C (Bodø, Tromsø and Spitsbergen [all Norway]), will perform better at low temperatures. To this end, two full-factorial, common-garden laboratory experiments were conducted on microscopic stages across populations, each with a different subset of populations, to investigate the fitness-related parameters of 1) upper survival temperatures (UST) of gametophytes integrated over time and sporophyte formation following thermal priming at sub-lethal high temperatures, and 2) gametophyte survival, sporophyte formation and sporophyte growth at low to optimum temperatures.
2 Materials and Methods
2.1 Study Sites
Samples were collected from the following six locations along the European NE Atlantic coast during summer, from north, in the Arctic, to south, on the coast of Brittany: Spitsbergen (SPT), Tromsø (TRM), Bodø (BOD), Helgoland (HLG), Roscoff (ROS) and Quiberon (QUI; Figures 1, 2; for sampling details see Liesner et al., 2020a). Spitsbergen and Quiberon, respectively, correspond to the northern and southern distribution boundaries of L. digitata. Maps were generated using a European Environment Agency coastline shapefile (European Environment Agency, 2021) and QGIS 3.8.2-Zanzibar software (QGIS Development Team, 2019).
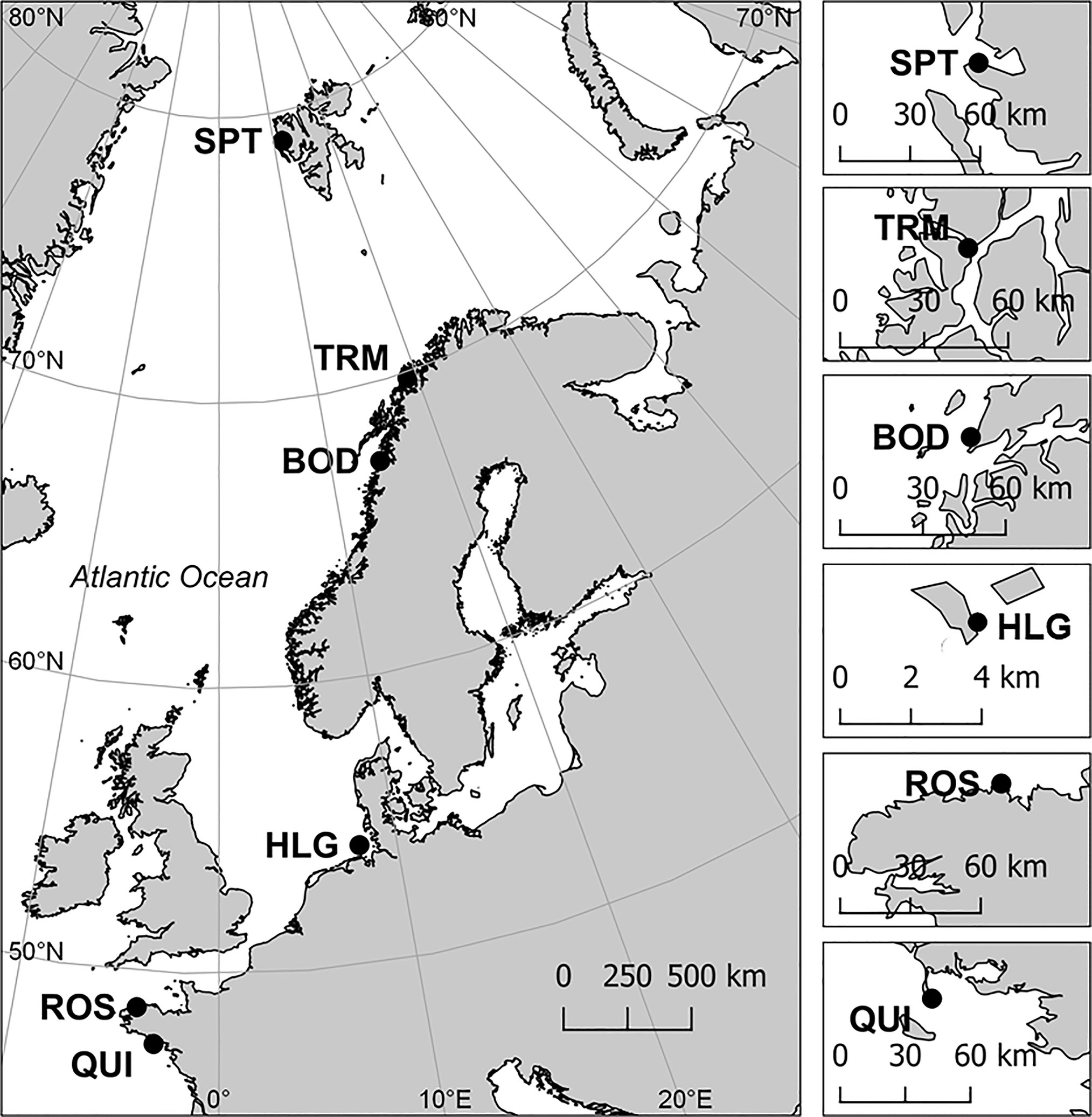
Figure 1 Sampling sites along the NE Atlantic coast. Inset maps show more precise locations within each area. Sampling sites were Spitsbergen, Norway (SPT), Tromsø, Norway (TRM), Bodø, Norway (BOD), Helgoland, Germany (HLG), Roscoff, France (ROS) and Quiberon, France (QUI).
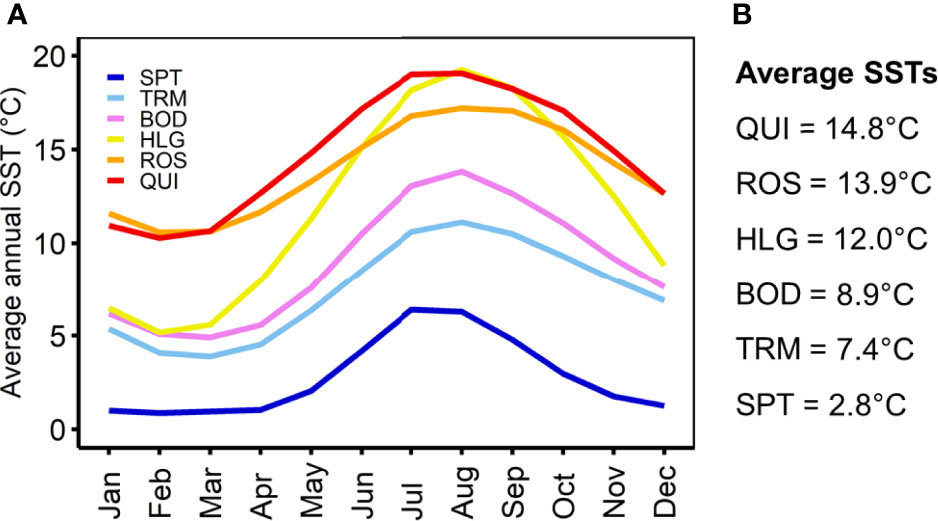
Figure 2 (A) Average annual sea surface temperatures (SSTs) for each month between 2010 and 2020 and (B) overall averages between 2010 and 2020. Satellite-derived data were obtained from the E.U. Copernicus Marine Service (2021). SPT, Spitsbergen; TRM, Tromsø; BOD, Bodø; HLG, Helgoland; ROS, Roscoff; QUI, Quiberon.
2.2 Sampling and Culture Conditions
Fertile L. digitata sporophytes (n=30-35, for Helgoland n=15), spaced ≥1 m apart, were collected at low tide in the infralittoral fringe at each location, and stored in ambient seawater flow-through tanks for immediate processing not lasting more than three days. Two soral discs per individual (Ø=20 mm) were cut out, briefly rinsed with fresh water, and placed in a 50 ml centrifuge tube containing two microscope slides and 45 ml sterile seawater. They were left for 1-3 days to allow released spores to settle. In samples from Bodø, sporulation was induced by leaving discs in a wet chamber overnight before adding sterile seawater. Saturated germanium dioxide solution (GeO2) was added (0.5 ml/l) to prevent diatom growth (Shea and Chopin, 2007). Tubes were transported in dark, cooled (<15°C) boxes from the respective locations to the Alfred Wegener Institute in Bremerhaven, Germany, within two days. Samples were stored at 5°C with a maximum irradiance of 5 µmol photons m-2 s-1 white light (LED Mitras Lightbar Daylight 150 controlled by a ProfiLux 3 computer, GHL Advanced Technology, Kaiserslautern, Germany), measured using a LI 190SB quantum sensor coupled to a LI-185B photometer (LI-COR-Biosciences, Lincoln, NE, USA) in a 16:8 h light:dark (LD) photoperiod. Microscope slides were then transferred to Petri dishes (Ø=9 cm) filled with iron-free, half strength Provasoli enriched seawater (PES) to inhibit gametogenesis (Provasoli, 1968; Lewis et al., 2013; iodide enrichment according to Tatewaki (1966), double concentration of Na2glycerophosphate) and GeO2, and stored at 15°C in 3-4 µmol photons m-2 s-1 red LED light to allow germination into vegetative gametophytes until start of experiment 1 (for details see Table S1). For experiment 2, pairs of male and female gametophytes, each originating from a single sporophyte, were isolated in 2016 (Spitsbergen) and in 2019 (Tromsø, Quiberon) into unialgal stock cultures and cultivated in 3 µmol photons m-2 s-1 red light at 15°C in ½ PES until start of the experiment in 2020 (for details see Table S2). This approach was needed, as long-term cultivation of dense, mixed gametophytes is often prone to hidden contamination that causes experimental bias, and because one sex may overgrow the other, resulting in an increasingly skewed sex-ratio (see Martins et al., 2017).
2.3 Experiment 1 – Upper Temperature Performance of Laminaria digitata Gametophytes
2.3.1 Stock Cultures
Five replicate gametophyte stock cultures were prepared for the Tromsø, Bodø, Roscoff and Quiberon populations (Table S1). Each stock contained gametophyte progeny derived from meiospores that originated from three distinct sporophytes and thus consisted of families of hundreds of sibling gametophytes. Replicates were therefore true biological replicates. Equal quantities of gametophyte material were gently fragmented with a sterile pestle and mortar, sieved to obtain a size fraction <100 µm and kept in suspension on a magnetic mixer at ~250 rpm. The density of each stock culture was estimated under an inverted microscope (Olympus CKX41, Olympus Co., Tokyo, Japan). Stock cultures were seeded into Petri dishes (Ø=6 cm) filled with 100 ml ½ PES to achieve a target density of 600 gametophytes cm-2. This density allows for good distinction between gametophytes and does not inhibit gametogenesis (Martins et al., 2017; Martins et al., 2020).
Samples from the Helgoland population had been collected a year before establishment of the standardized experimental protocol. Meiospores from Helgoland were released from sori after being stored for 1-2 days in <5 µmol photons m-2 s-1 white light in plastic bags filled with sterilized natural seawater, following the method of Bartsch (2018). Spore suspensions obtained from five individual sporophytes were used to inoculate four replicate dishes at a target density of 15,000 spores cm-2. Following meiospore germination in 15 µmol photons m-2 s-1 for four days, dishes were stored in darkness at 15°C for four weeks before initiation of the experiment. For each of the five lineages, four replicate dishes were placed into five temperature treatments (n=4 for five lineages; n=20 in total).
2.3.2 Experimental Set-up
Gametophytes from Tromsø, Bodø, Roscoff and Quiberon were acclimated for six days (three days at 15°C and three days at 20°C) in an irradiance of 2-3 µmol photons m-2 s-1 white LED light in a 16:8 h LD regime. Gametophytes were incubated in temperature-controlled water baths (Huber Variostat CC + Pilot ONE, Peter Huber Kältemaschinen GmbH, Offenburg, Germany). With the beginning of the priming period on day 0, the irradiance was raised to 15 µmol photons m-2 s-1 and gametophytes were exposed to high temperatures spanning 20°C, 21°C, 22°C, 23°C, 24°C and 25°C ± 0.1°C for 14 days. A 15°C treatment served as control to monitor gametogenesis in optimum conditions (Lüning, 1980). On day 14, gametophytes from all priming treatments were transferred to 15°C for 14 days under the same irradiance conditions, to observe potential recovery and sporophyte formation. Medium exchange took place on day 14 and 28. Spitsbergen material was excluded here, as the temperature tolerance of this population has been determined before (Franke et al., 2021).
Material from Helgoland was acclimated to low light (3-5 µmol photons m-2 s-1) for five days and brighter light (15 µmol photons m-2 s-1) for one day before start of the experiment. The experiment started immediately for the 15°C and 20°C treatments, while the remaining treatments underwent a temperature ramping period: 22°C (one day at 20°C), 24°C (one day at 20°C) and 25°C (one day at 20°C, two days at 24°C). There were no 21°C and 23°C treatments for the Helgoland population. Following the 14-day priming period, gametophytes were transferred to 20°C in 5 µmol photons m-2 s-1 for 14 days of post-culture.
In all populations, long-term post-cultivation in apparently lethal temperatures (24°C and 25°C) was checked against control (20°C) after approximately 3 to 15 weeks at 15°C in low red light and iron-free ½ PES.
2.3.3 Gametophyte Survival Under Heat Stress
Total gametophyte density was quantified on day 0, 4, 8, 11, 14 and after recovery on day 21 and 28 (day 0, 7, 14 and 28 for Helgoland; no day 28 for Bodø) under an inverted microscope. The mean number of randomly selected fields of view required to quantify ≥200 female gametophytes was estimated on day 0 and used as a standard for subsequent measurements. Cells were considered alive when they still showed pigmentation.
Due to differences in methodology between the direct seeding of spores from Helgoland and the use of multi-cellular, vegetative gametophyte progeny for the other populations, Helgoland had a significantly higher initial gametophyte density (single cells) on day 0 than Tromsø, Bodø, Roscoff and Quiberon (Kruskal-Wallis, X2 = 193.69, p<0.0001, df = 4). However, the absolute number of cells per dish was similar (Table S3). We therefore calculated integrated gametophyte survival over time as follows: for each priming treatment and population, we averaged gametophyte survival between day 4 and 28, based on six (BOD: five; HLG: three) points in time, to yield overall gametophyte survival over the entire experimental phase. Some replicates were excluded from statistical analysis when contaminations through diatoms or filamentous brown algae became visible.
2.3.4 Sporophyte Formation During Recovery From Thermal Priming
The formation of microscopic sporophytes (% of female gametophytes with sporophytes) at 15°C, following the 2-week-long thermal priming period, was quantified on day 28. Microscopic sporophytes were distinguished from released eggs when they were no longer spherical or showed first cell divisions.
2.4 Experiment 2 - Lower Temperature Performance of Laminaria digitata Gametophytes
2.4.1 Stock Cultures
Five male and five female stock cultures were prepared for the Spitsbergen, Tromsø and Quiberon populations, each containing equal quantities of pooled gametophyte material from five clonal isolates (always one pair of male and female gametophytes derived from one sporophyte) which had been obtained from different sporophytes (Table S2). A density of 300 male and 300 female gametophytes cm-2 was targeted. As these gametophytes were fragmented pieces of the five clonal cultures, the actual fraction of each clone per replicate was not controllable, however we assumed a random dispersal. Fragmentation, sieving, and density determination was conducted as described above. Due to logistical reasons, we only chose representatives of the coldest, warmest, and intermediate temperature environments of the species (Figure 2).
2.4.2 Experimental Set-up
For gametophytes from Spitsbergen, Tromsø and Quiberon, the temperature was ramped down over the course of six days, starting with a temperature of 15°C which was lowered daily by 3°C until the respective experimental temperature (0°C, 3°C, 6°C, 9°C, 12°C and 15°C ± 0.1°C) was reached one day before the start of the treatment period. These temperatures were chosen as they approximately correspond to the different local long-term SST averages (Figure 2). Ramping took place in 1-2 µmol photons m-2 s-1 white light in a 16:8 h LD cycle to suppress fertility. With the start of the 21-day long treatment period, the irradiance was increased to 15 ± 1 µmol photons m-2 s-1 white light with the same photoperiod, and the medium (½ PES) was exchanged once on day 14.
2.4.3 Gametophyte and Sporophyte Quantification
Counting of the gametophytes was conducted as described for experiment 1. The required number of fields of view to be counted was based on quantifying ≥400 total male and female gametophytes on day 0, as sexual dimorphism was not yet entirely evident. Due to significant differences in initial gametophyte densities (ANOVA, F(2,87)=24.95, p<0.0001; Table S3), percentage survival of gametophytes was quantified on day 0, 7, 14 and 21 relative to day 0. The formation of microscopic sporophytes (% of female gametophytes with sporophytes) and sporophyte density (sporophytes cm -2) were quantified on day 7, 14 and 21.
2.4.4 Sporophyte Size and Growth
The size of microscopic sporophytes was measured on day 7 and 21 via photographic images (camera: AxioCam ERc5s mounted on an inverted microscope). Per replicate, ten fields of view containing at least one sporophyte were randomly photographed. The length and width of the longest sporophyte in each photograph was measured using the imaging software ZEN 2 Blue Edition version 2.3. If only one sporophyte was present, this one was selected. As sporophyte formation is a continuous process stretching over several weeks, we assumed that the longest sporophytes in each photograph represented the oldest ones and thus provided the best proxy for maximal growth. Length to width ratio (L:W ratio) was derived for day 21. In addition, the linear length (GR length) and linear width growth rates (GR length and GR width), were calculated separately between day 7 and 21 as follows:
where x is the length or width (μm), and t is the time in weeks (wk) at time point 1 and 2.
2.5 Statistical Analysis
Statistics were performed using R version 3.6.3 (R Core Team, 2020). One-way ANOVA was performed to test initial gametophyte densities in experiment 2 and gametophyte survival at 24°C and 25°C on day 8 (HLG, day 7) in experiment 1. Initial densities in experiment 1 did not meet assumptions of homogeneity, so a non-parametric Kruskal-Wallis test was performed, with subsequent pairwise Wilcoxon rank sum testing and Bonferroni correction of p-values to reduce the probability of type I errors. Sporophyte GR length, GR width and L:W ratio were analyzed using ANCOVA, with sporophyte density (day 7) as a covariate, as density may influence growth rates. All other parameters were evaluated by two-way ANOVA. Statistically significant differences were compared with the post hoc Tukey honest significance difference (HSD) test using the “emmeans” package. Prior to analysis, data were tested for homogeneity of variance using the Levene’s test. Data with heterogeneous variances were either square-root, fourth-root or logarithmically transformed (Underwood, 1996). If assumptions of homogeneity were still not met, the significance level was lowered to p<0.01, as there is no non-parametric alternative for two-way ANOVA with post hoc tests. Reports of pairwise comparisons are described by the highest p-value.
3 Results
3.1 Experiment 1
3.1.1 Gametophyte Survival
Integrated survival of L. digitata gametophytes at (sub-) lethal high temperatures (20-25°C) over time (day 4-28) was significantly influenced by population and temperature, but there was no interaction (Table 1; Figure 3). Thus, all populations responded to temperature in the same way. Gametophytes from all populations suffered a loss of pigmentation at 24°C and 25°C, resulting in a significantly lower mean survival in the 24°C (19.9%-40.8%) and 25°C treatments (0.1%-24.3%) than in the 15-23°C treatments (70.4%-104.5%; [15°C = 20°C = 21°C = 22°C = 23°C] > [24°C = 25°C]; Tukey tests, p<0.05). Between populations, there were statistically significant differences in integrated gametophyte survival at 15°C ([ROS = BOD = TRM] > [QUI = HLG = BOD = TRM]; Tukey tests, p<0.05), at 20°C ([QUI = ROS = BOD =TRM] > [QUI = HLG]; Tukey tests, p<0.01) and at 22°C ([ROS = BOD = TRM] > [QUI = ROS = HLG]; Tukey tests, p<0.05). In gametophytes from all populations except for Helgoland, the survival rate intermittently rose above 100%, which probably indicates fragmentation of vegetative gametophytes into smaller pieces by die-off of intercalary gametophyte cells. However, this process was not quantified.
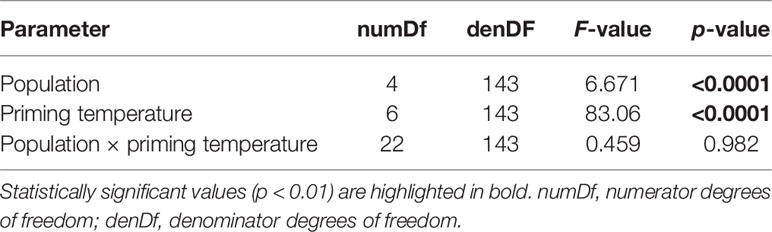
Table 1 Two-way ANOVA to assess the effects of priming temperature and population on integrated survival of Laminaria digitata gametophytes over time in experiment 1.
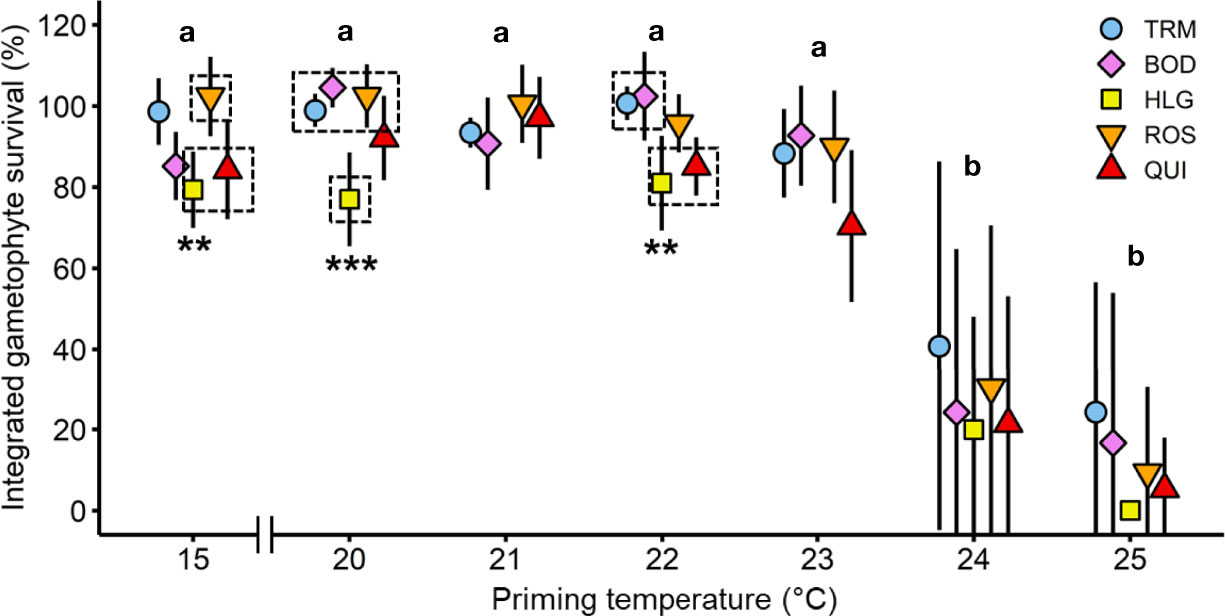
Figure 3 Integrated gametophyte survival over time of Laminaria digitata along a temperature gradient in experiment 1. Mean relative gametophyte survival between day 4 and 28 for priming treatments 15-25°C for Tromsø (TRM; n = 5; day 28, n = 4), Bodø (BOD; n = 5), Helgoland (HLG; n = 4), Roscoff (ROS; n = 5; day 21-28, n = 3) and Quiberon (QUI; n = 5; day 21-28, n = 4) averaged over six (BOD: five; HLG: three) points in time. Letters indicate statistical differences in survival between treatments within each population (ANOVA; Tukey test, p < 0.01). Asterisks and dashed boxes denote the degree of significant differences between populations within treatments (ANOVA, Tukey test, p < 0.05). Values are expressed as the mean of means ± SD.
With respect to percentage survival, gametophytes from all populations survived between 57%-100% in the 15-23°C treatments for 28 days (Figure S1). At higher temperatures, gametophytes from all populations suffered a loss of pigmentation with low survival at 24°C (<7.6% on day 14 and <2.2% on day 28) and no survival at 25°C (Figure S1). A noteworthy disparity was visible on day 8 (HLG, day 7) at 24°C and 25°C. In the 24°C treatment, gametophytes from Tromsø maintained a significantly higher survival than gametophytes from Bodø, Helgoland and Quiberon, while survival of gametophytes from Roscoff was intermediate (ANOVA, F(4,35)=6.42, p<0.001). After 8 days at 25°C, survival declined to <3.7% in gametophytes from all populations except for Tromsø, which displayed a significantly higher gametophyte survival of 51.7% at this point (F(4,35)=59.34, p<0.0001).
The long-term post-cultivation of the 24°C treatment (data not shown) revealed that single gametophyte cells from all populations survived and proliferated vegetatively again after a few weeks at 15°C (QUI, 3 weeks: 0.01%-0.14% males, 0% females; ROS, 5 weeks: 0%-0.1% males, 0%-0.03% females; HLG, 15 weeks: 0%-2.3% total gametophytes; BOD, 4 weeks: 0.01%-0.21% males, 0%-0.08% females; TRM: no data). However, at 25°C no gametophyte cells had survived at all.
3.1.2 Sporophyte Formation
During the 14-day thermal priming period, female gametophytes in the 20-25°C treatments from all populations remained in a vegetative state or died, while in the 15°C treatment eggs and sporophytes developed as expected. In all populations, only females that were primed at 20-23°C developed eggs and sporophytes after transfer to 15°C (Figure 4).
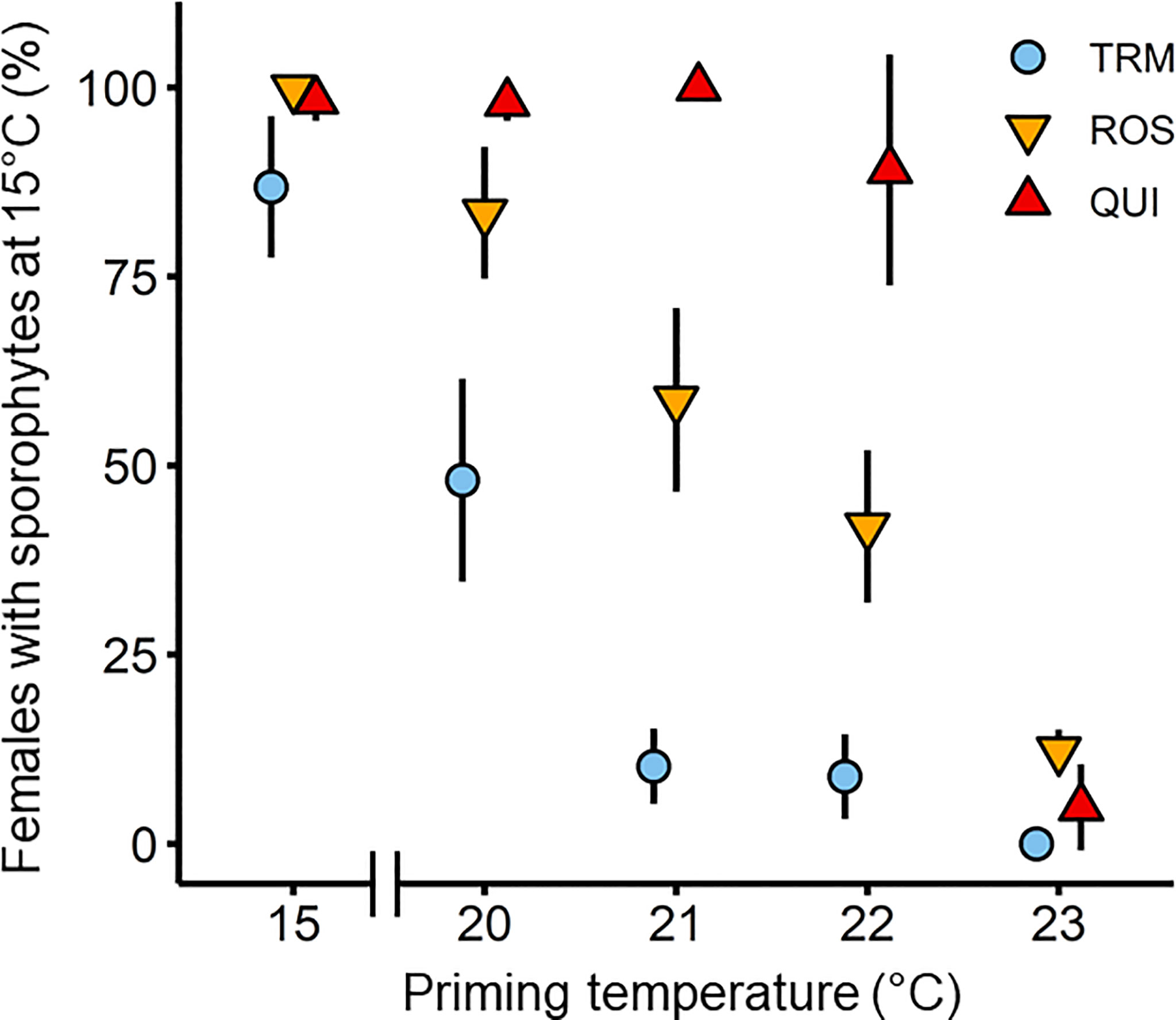
Figure 4 Formation of microscopic sporophytes of Laminaria digitata on day 28, after 14 days of thermal priming at (sub)-lethal non-fertilizing temperatures (20°C, 21°C, 22°C and 23°C) followed by 14 days of recovery at 15°C in the three populations Tromsø (TRM; n = 4), Roscoff (ROS; n = 3) and Quiberon (QUI; n = 4) in experiment 1. 15°C served as control temperature over 28 days. The 24°C treatment was excluded, as only 0%-3% of gametophytes survived and they did not become fertile during recovery at 15°C. There were no surviving gametophytes at 25°C. Values are expressed as the mean ± SD.
Sporophyte formation on day 28, 14 days after transfer to 15°C, was significantly influenced by priming temperature, population, and their interaction (Table 2), and decreased with increasing priming temperature in samples from all populations (Figure 4). Significant differences between populations were evident at priming temperatures of 20°C, 21°C and 22°C while at 23°C, female gametophytes from all populations showed no or very low mean sporophyte development (0%-12.3% females with sporophytes). At 24°C, gametophyte survival was only 0%-3% and gametophytes did not become fertile during recovery at 15°C. In the 20°C priming treatment, females from the southern populations Quiberon (98.0 ± 2.4%) and Roscoff (83.4 ± 8.7%) developed significantly more microscopic sporophytes per female gametophyte than the northern population Tromsø (48.1 ± 13.4%; Tukey test, p<0.0001). In the 21°C and 22°C priming treatments, respective sporophyte formation differed significantly among all populations (QUI [21°C: 100 ± 0%; 22°C: 89.1 ± 15.3%] > ROS [21°C: 58.7 ± 12.1%; 22°C: 42.0 ± 10.1%] >TRM [21°C: 10.2 ± 5.0%; 22°C: 8.8 ± 5.6%]; Tukey test, p<0.0001).
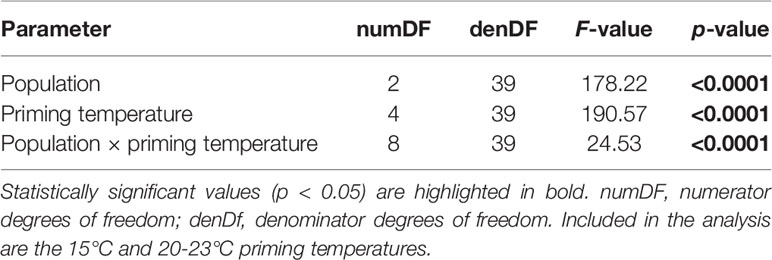
Table 2 Two-way ANOVA to examine the effects of priming temperature and population on the sporophyte formation of Laminaria digitata gametophytes from Tromsø, Roscoff and Quiberon on day 28 during experiment 1.
Within populations, female gametophytes from Quiberon showed the broadest temperature window for sporophyte formation (89.1%-100%) between 15°C and the 22°C priming treatment ([15°C = 20°C = 21°C = 22°C] > 23°C; Tukey test, p<0.0001). Sporophyte formation in females from Roscoff was highest at 15°C and in the 20°C priming treatment (99.6%-83.4%) and decreased linearly with warmer priming temperatures ([15°C = 20°C] > [21°C = 22°C] > 23°C; Tukey test, p<0.05). Reproductive success in the Tromsø population was highest at 15°C (86.9% ± 9.4%), intermediate at the 20°C priming temperature (48.1 ± 13.4%) and decreasing sharply to 8.8%-10.2% at the 21-23°C priming temperatures (15°C > 20°C > [21°C = 22°C = 23°C]; Tukey test, p<0.0001).
3.2 Experiment 2
3.2.1 Gametophyte Survival
Survival of L. digitata gametophytes from Spitsbergen, Tromsø and Quiberon at lower temperatures (0-15°C) is shown in Figure 5A. There was a significant population × temperature interaction for the survival of gametophytes after 21 days (Table 3). The significant population differences corresponded to the latitudinal origin of gametophytes. Gametophytes from the northern-most population of Spitsbergen survived better at lower temperatures, with significantly higher survival at 0°C (57.3% ± 8.4%) and 3°C (58.0% ± 8.6%) than at 12°C (35.3% ± 2.4%) and 15°C (36.9% ± 9.7%; [0°C = 3°C = 6°C = 9°C] > [6°C = 9°C = 15°C] > [6°C = 12°C = 15°C]; Tukey test, p<0.05). The southern population of Quiberon on the other hand showed significantly higher survival at 9-15°C (87.1%-88.4%) than at 0°C (66.1% ± 6.5%; [0°C = 3°C = 6°C] < [3°C = 6°C = 9°C = 12°C = 15°C]; Tukey test, p<0.05). Gametophytes from Tromsø showed significantly lower survival at 3°C (56.1% ± 7.3%) and 12°C (56.5% ± 5.6%) than at 15°C (78.0% ± 10.2%; [0°C = 3°C = 6°C = 9°C = 12°C] < [0°C = 6°C = 9°C = 15°C]; Tukey test, p<0.05).
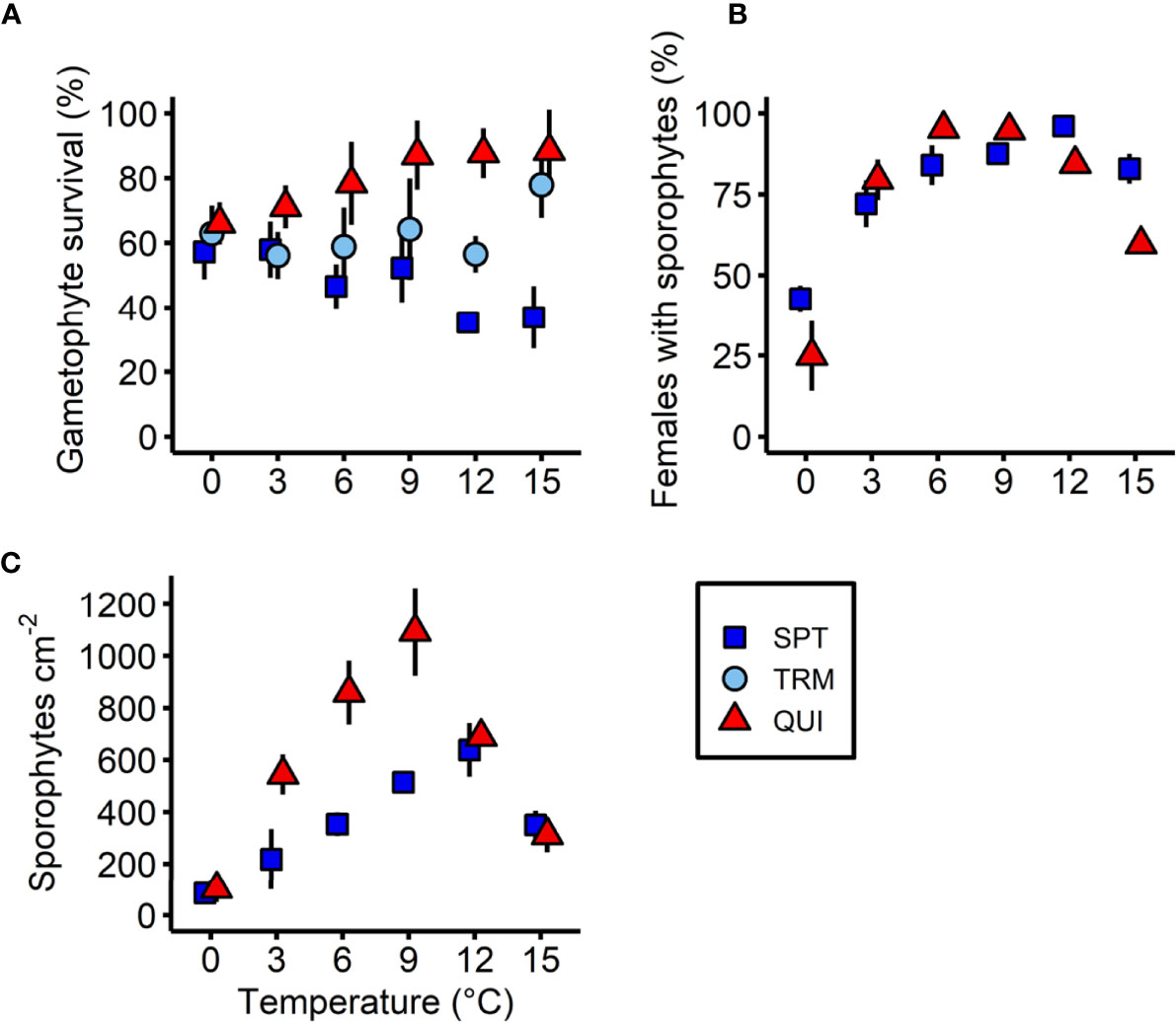
Figure 5 Effect of temperature on (A) the gametophyte survival (%), (B) females with microscopic sporophytes (%) and (C) absolute sporophyte density of Laminaria digitata on day 21 in three populations (Spitsbergen, SPT; Tromsø, TRM; Quiberon, QUI) along a temperature gradient between 0°C and 15°C during experiment 2. The Tromsø population was excluded in (B) and (C), due to high egg mortality after 14 days. Values are expressed as the mean ± SD (n = 5).
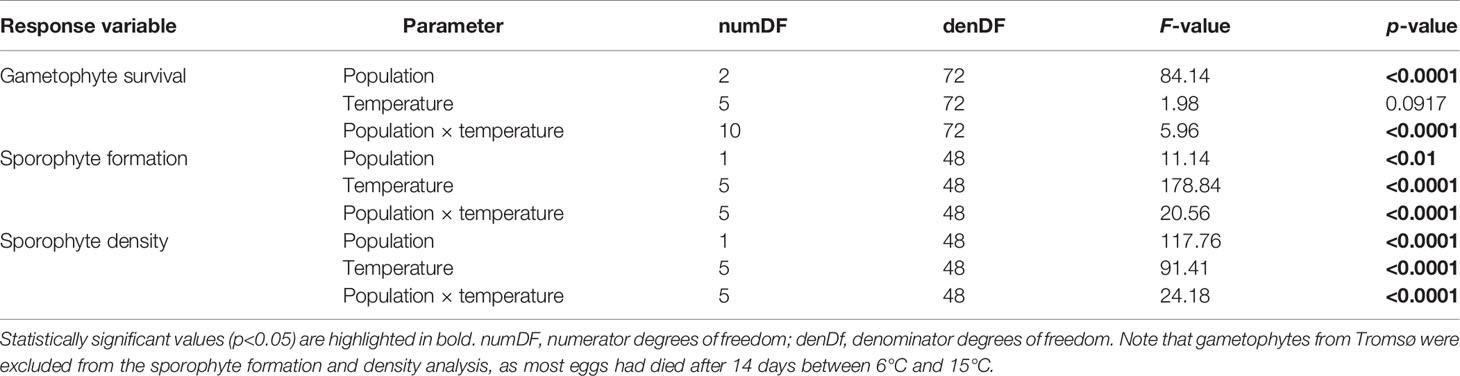
Table 3 Results of two-way ANOVAs to examine the effects of temperature and population on the response variables gametophyte survival, sporophyte formation and sporophyte density of Laminaria digitata from Spitsbergen, Tromsø and Quiberon on day 21 along the lower temperature gradient (0-15°C) in experiment 2.
3.2.2 Sporophyte Formation
The formation of microscopic sporophytes on day 21 was significantly affected by an interaction of population and temperature (Table 3; Figure 5B). Due to unknown reasons, many eggs in the Tromsø population disintegrated after 14 days between 6°C and 15°C, resulting in low numbers of offspring, so this population was excluded from the statistical analysis. In samples from Spitsbergen, the percentage of female gametophytes with sporophytes at 0°C (42.8% ± 4.0%) and 3°C (72.1% ± 7.3%) was significantly lower than between 6°C and 15°C (84.0%-96.0%; 0°C < 3°C < [6°C = 9°C = 15°C] < [9°C = 12°C]; Tukey test, p<0.05). In the Quiberon population, the highest proportion of females with sporophytes was observed in the mid temperatures between 3°C and 12°C (79.5%-95.2%), with maxima at 6°C and 9°C, which were significantly higher than at 0°C (24.9% ± 10.9%) and 15°C (59.7% ± 3.1%; 0°C < 15°C < [3°C = 12°C] < [9°C = 12°C] < [6°C = 9°C]; Tukey test, p<0.05). It is also noteworthy that relative sporophyte formation per female gametophyte in the northern-most population of Spitsbergen was significantly higher than in the southern population of Quiberon at 0°C, 12°C and 15°C, while there were no significant differences between the two populations at 3°C, 6°C and 9°C (Tukey test, p<0.05).
3.2.3 Sporophyte Density
Sporophyte density on day 21 was significantly affected by a population × temperature interaction for the Quiberon and Spitsbergen population, and the overall sporophyte density in the Spitsbergen population was much lower than for the Quiberon population (Table 3; Figure 5C). Sporophyte density in the Quiberon population was at its peak at 9°C (1094 sporophytes cm-2), which was significantly higher than in all other temperature treatments (0°C < 15°C < [3°C =12°C] < [6°C = 12°C] < 9°C; Tukey test, p<0.05). In the Spitsbergen population, sporophyte density was significantly higher at 9°C and 12°C (515 and 639 sporophytes cm-2) than at the other temperatures ([0°C = 3°C] < [3°C = 6°C = 15°C] < [9°C = 12°C]; Tukey test, p<0.05). At 3°C, 6°C and 9°C, sporophyte density was significantly higher in the Quiberon, compared to Spitsbergen population (Tukey test, p<0.0001), while there were no significant differences at 0°C, 12°C and 15°C.
3.2.4 Sporophyte Length and Width Growth
Linear GR length and GR width of sporophytes integrated between day 7 and day 21 revealed a significant population × temperature interaction, taking into account the significantly different sporophyte densities in all populations on day 7 (Table 4; Figures 6A, B). The overall sporophyte GR length (Figure 6A) was generally very low in cold temperatures and increased significantly towards higher temperatures, with the highest GRs length between 9°C and 15°C, irrespective of location (SPT: 0°C < 3°C < 6°C < 9°C < [12°C = 15°C]; Tukey test, p<0.05; TRM: 0°C < 3°C < 6°C < [9°C =15°C] < 12°C; Tukey test, p<0.01; QUI: 0°C < 3°C < 6°C < [9°C = 15°C] < [12°C = 15°C]; Tukey test, p<0.001). However, the GR length at 9°C, 12°C and 15°C was significantly higher in sporophytes from Tromsø (423 to 562 µm length week-1) and Quiberon (328 to 469 µm length week-1) than from Spitsbergen (210 to 317 µm length week-1; Tukey test, p<0.01). In cold temperatures, at 0°C and 3°C, GR length did not differ significantly between populations (26 to 52 µm length week-1). At 6°C, there was a significantly higher sporophyte GR length in the Tromsø population (174 µm length week-1) than in the Quiberon and Spitsbergen populations (112 and 104 µm length week-1; Tukey test, p<0.01).

Table 4 Two-way ANCOVA to examine the effects of temperature and population on sporophyte growth parameters of Laminaria digitata on day 21 along a lower temperature gradient (0-15°C) in experiment 2, with sporophyte density on day 7 as a covariate.
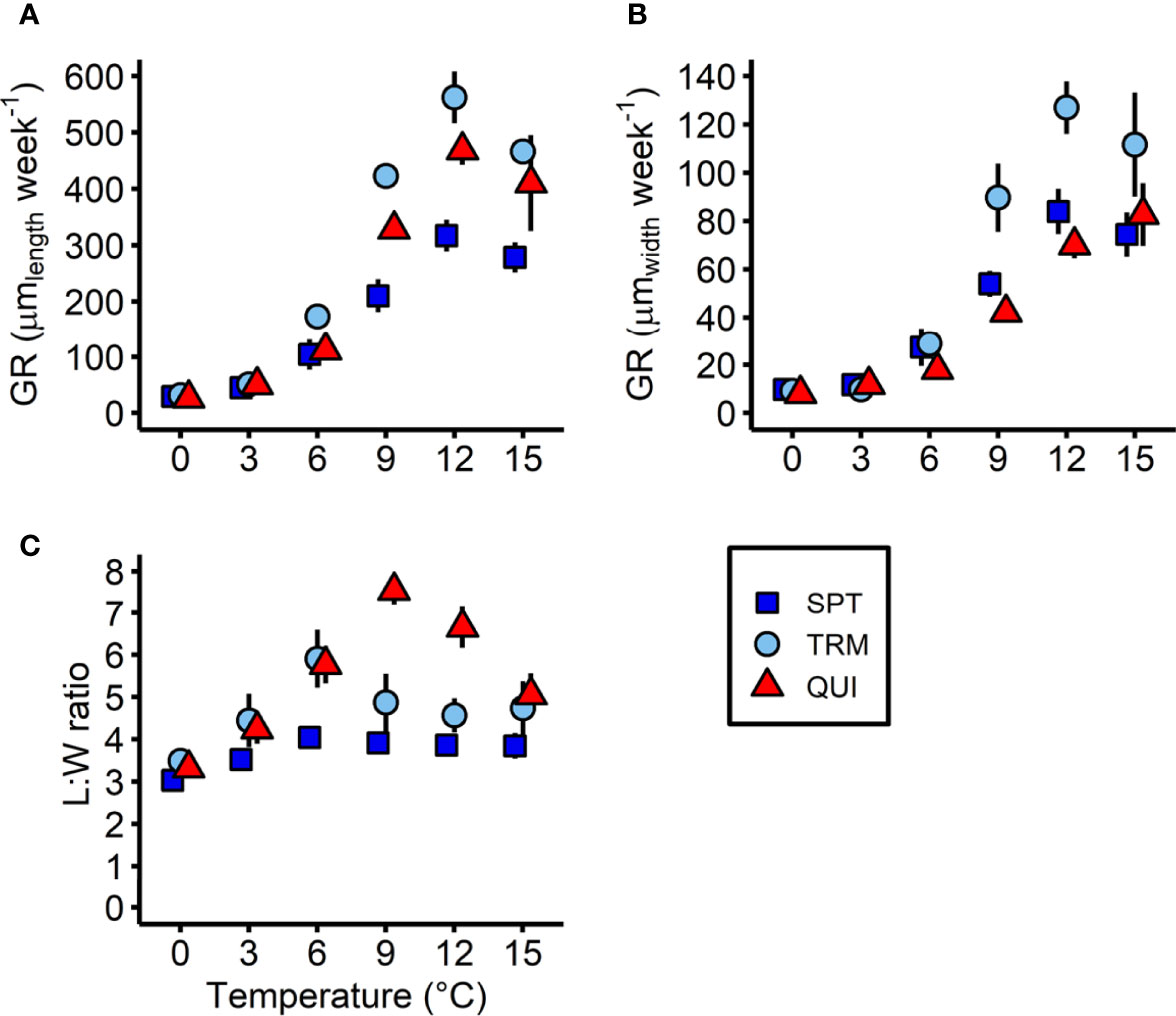
Figure 6 Sporophyte growth parameters of Laminaria digitata in three populations (Spitsbergen, SPT; Tromsø, TRM; Quiberon, QUI) on day 21 between 0°C and 15°C in experiment 2. (A) Linear sporophyte growth rate (GR) in length, (B) in width and (C) sporophyte length:width (L:W) ratio. Values are expressed as the mean of means ± SD (n = 5).
A similar pattern was observed in sporophyte GR width across all populations (Figure 6B). The GRs width were low at low temperatures and increased towards higher temperatures, but with differences between temperatures per population (SPT and TRM: [0°C = 3°C] < 6°C < 9°C < [12°C = 15°C]; Tukey test, p<0.05; QUI: 0°C < 3°C < 6°C < 9°C < [12°C = 15°C]; Tukey test, p<0.05). In the 9°C, 12°C and 15°C treatments, Tromsø sporophytes showed significantly higher GRs width (90 to 127 µm width week-1) than Spitsbergen (54 to 84 µm width week-1) and Quiberon sporophytes (42 to 83 µm width week-1; Tukey test, p<0.05). At 0°C and 3°C however, GRs width were very low and were same between populations (8 to 12 µm width week-1). At 6°C, there was a significantly higher sporophyte GR width in the Tromsø and Spitsbergen population (29 and 27 µm width week-1) than in the Quiberon population (18 µm width week-1; Tukey test, p<0.05).
A significant temperature × population interaction also became apparent for the L:W ratio on day 21 (Figure 6C; Table 4). Spitsbergen sporophytes showed a relatively constant L:W ratio of 3.0-4.1, but with a significantly lower L:W ratio at 0°C compared to the other populations (0°C < [3°C = 9°C = 12°C = 15°C] < [6°C = 9°C = 12°C = 15°C]; Tukey test, p<0.05). In sporophytes from Tromsø, the L:W ratio spiked at 6°C (5.9 ± 0.7) and was lowest at 0°C, 3°C and 12°C (3.5-4.6; [0°C = 3°C = 12°C] < [3°C = 9°C = 12°C = 15°C] < [6°C = 9°C]; Tukey test, p<0.05). Quiberon showed the lowest L:W ratio at 0°C (3.3 ± 0.2) and the highest at 9°C (7.6 ± 0.3; 0°C < 3°C < [6°C = 15°C] < 12°C < 9°C; Tukey test, p<0.05).
4 Discussion
This study highlights the importance of holistic approaches for the identification of intraspecific variation and local adaptation. Particularly sessile species with extensive latitudinal ranges may exhibit ecotypic adaptation as a means of survival, and so cannot be assumed to respond uniformly throughout their range and across different fitness-related parameters (Atkins and Travis, 2010; Mohring et al., 2014; Filbee-Dexter et al., 2020). The use of full-factorial, common-garden experiments, along with the usage of gametophyte progeny from several sporophytes, presents a sound method for studying adaptive changes in kelp populations from varying in situ environmental conditions, while also ensuring wider genetic diversity of populations, than studies based on single clonal cultures (King et al., 2018; Martins et al., 2020). Here, we provide evidence for ecotypic differentiation of microscopic stages of the key habitat-forming kelp Laminaria digitata along its NE Atlantic distribution range. By investigating microscopic stages and several associated life cycle processes, we highlight that the response pattern across populations towards (sub)-lethal high and (sub)-optimum low temperatures is partly uniform across populations and partly reflects the long-term local temperature history of the respective population and thus their biogeographic distribution patterns, indicating local adaptation. However, some responses varied in an unpredictable manner, deviating from their local long-term temperature history, which warrants further investigation.
4.1 Uniform Upper Survival Temperature of Gametophytes
Gametophytes from the five tested populations (Quiberon in the south, to Tromsø in the north), spanning a wide distribution range in the NE Atlantic, exhibited a similar response in the upper temperature gradient with a uniform UST of 24°C after a two-week exposure, with no short-term recovery and limited long-term recovery. Gametophytes from Spitsbergen have been investigated before and exhibited the same upper lethal temperature limit (Franke et al., 2021). This is despite local mean summer SSTs differing by ~12-13°C between the southern (Oppliger et al., 2014) and northern distribution margins (Fischer et al., 2019; Figure 2). Although none of the populations are likely to experience such high SSTs over 14 days in their natural environment, it should be noted that our experimental priming temperature of 24°C only exceeds the mean summer SST at the southern-most location in Quiberon by ~6°C, but by 18-19°C at the northern-most limit of the species in Spitsbergen (Oppliger et al., 2014; Fischer et al., 2019). Few studies have examined the intra-specific thermal response of microscopic gametophyte and sporophyte stages throughout their distribution gradient (Martinez, 1999; Oppliger et al., 2012; Mohring et al., 2014; Becheler et al., 2022). However, several previous studies have shown that the upper lethal limit with respect to survival and growth of gametophytes of Atlantic Laminaria spp. and Saccharina spp. was also similar across sites with different thermal environments (Bolton and Lüning, 1982; tom Dieck (Bartsch), 1992; Martins et al., 2020); but these authors only worked with single clonal isolates. With recent insights into the genetic heterogeneity of L. digitata, its reduced gene flow in marginal populations (e.g., Billot et al., 2003; King et al., 2020; Liesner et al., 2020a) and latitudinal adaptation of sporophytes towards heat stress (e.g., King et al., 2019; Liesner et al., 2020a), it became questionable whether this single-clonal approach was sufficient to understand the full tolerance width within a single kelp species. By applying a population approach and assuming that we incorporated several genetic lineages per location, our study now confirms that the upper thermal limit of L. digitata gametophytes is conserved along its whole distribution gradient in the NE, irrespective of the local long-term thermal environment. This supports early assumptions of Wiencke et al. (1994) that the upper survival limit of seaweed species is relatively fixed and illustrates that neutral genetic diversity is not always a good indicator for intra-specific performance plasticity (King et al., 2020).
Polar and temperate kelp species exhibit a complex alternation of generations between microscopic gametophytes and macroscopic sporophytes, where each life cycle stage exploits different ecological niches according to its physiological needs. This strategy is thought to provide an adaptive advantage, especially in variable environments (Ladah and Zertuche-González, 2007; Barradas et al., 2011). Microscopic gametophytes of kelps have been shown to be the most heat tolerant stage, regardless of population or species (tom Dieck (Bartsch), 1993; Ladah and Zertuche-González, 2007), highlighting their widespread ability to persist as hardy, long-lived seed banks, that can exist for up to decades without losing their fertility (tom Dieck (Bartsch), 1993; Edwards, 2000; Carney and Edwards, 2006; Edwards, 2022; Martins et al., 2020). The fact that such a high UST has been preserved in L. digitata gametophytes underlines the importance of this parameter as a survival mechanism in which persisting gametophytes can replenish sporophyte populations following die-off events (e.g., Bartsch et al., 2013). This may be especially important for southern populations that have in fact faced large-scale extirpation in the past (Lima et al., 2007; Fernández, 2011; Moy and Christie, 2012; Voerman et al., 2013; Smale et al., 2015).
After several weeks of post-cultivation at 15°C, gametophytes previously exposed to 24°C were observed to grow again. These may have arisen from single living cells in damaged multicellular gametophyte filaments or from unaccounted surviving gametophytes that we missed in our monitoring protocol, where we only sampled random areas of the petri dishes. Therefore, we highlight the importance of allowing long-term cultivation under growth-supporting conditions after exposure to environmental stressors, as we might be underestimating the survival potential of microscopic stages of kelps at near lethal conditions.
Interestingly, the few gametophytes that survived the 24°C priming treatment were primarily male. These results suggest differential survival capacities between sexes, with female gametophytes being more sensitive to heat than male gametophytes. In several species of the genus Laminaria, male gametophytes were reported to survive better in high temperatures than female gametophytes, (tom Dieck (Bartsch), 1993). Likewise, Arctic isolates of the morphologically similar species L. digitata and Hedophyllum nigripes, revealed higher heat tolerance in male than female gametophytes (Franke et al., 2021). Bolton and Lüning (1982) also found a 1°C higher UST in male L. digitata gametophytes compared to females, but not for the other N-Atlantic kelp species L. hyperborea, S. latissima and Arctic Laminaria solidungula. One explanation for the differential survival capacity of sexes is the ability of male gametophytes to reproduce vegetatively through fragmentation (Destombe and Oppliger, 2011), giving an illusion of higher survival. This is thought to act as a dispersal mechanism by reducing male to female distances (to <1 mm) to facilitate pheromone signaling and thereby enhancing reproductive success (Reed et al., 1997; Destombe and Oppliger, 2011). In the wild, more male survivors may increase the chance of females being fertilized, as an oogonium and antheridium only produce one egg and sperm, respectively (Destombe and Oppliger, 2011). Why sex-ratio is especially male-skewed at high temperatures (see also Lee and Brinkhuis, 1988; Izquierdo et al., 2002), however, is not clear, but may be linked to better stress resistance of males. In fact, gene expression profiles in vegetative S. latissima gametophytes have been shown to be different between temperatures and sexes (Monteiro et al., 2019). Only a small proportion (7-12%) of differentially expressed genes were consistent across temperatures, indicating that temperature strongly influences development. In general, functional categories linked to responses to stress and external and abiotic stimuli were over-represented in females. Females also responded more strongly at higher temperatures than males, suggesting a lower heat tolerance of females (Monteiro et al., 2019). These hypotheses may explain the significantly slower gametophyte mortality of the Tromsø population at 24°C and 25°C within the priming period compared to all other populations, as only this population was male-dominated (1.74 × more males than females on day 0 - an artificial effect of inconsistent seeding), which could have provided a survival advantage.
4.2 Ecotypic Survival of Gametophytes at Low to Optimum Temperatures
In contrast to the uniform lethal limit at high temperatures across populations, we discovered that gametophyte survival from three populations in the lower temperature gradient between 0-15°C was clearly reflective of the thermal environment from which they originated. The southern-most population of Quiberon showed a positive relationship of survival with increasing temperature, whereas the northern-most population Spitsbergen showed a negative relationship with increasing temperature. Gametophytes from both populations survived best near their in situ annual average SSTs at 15°C (Quiberon) and 0-3°C (Spitsbergen). Gametophytes from Tromsø, located in between, where the mean annual SSTs are ~7°C (Chepurin and Carton, 2012; Figure 2), revealed rather constant survival over temperatures, with significantly lower survival only at 3°C and 12°C compared to 15°C. This suggests that L. digitata populations have adapted in response to local long-term environmental conditions and that selection pressure towards local mean temperatures was higher than for USTs. A study by Mohring et al. (2014), that examined the thermal performance of microscopic stages of the warm-temperate kelp Ecklonia radiata from three regions across its latitudinal distribution in southern Australia, came to similar conclusions. Gametophyte survival (density) and growth (area of gametophytes) was optimal in conditions reflective of the environment in which they persist. These findings support the hypothesis that variability in the thermal performance of kelps with broad latitudinal distributions is influenced by biogeography and ecotypic adaptation to local temperature regimes, but that this adaptation is only reflected in naturally-occurring temperature ranges, shaping optimal responses towards local conditions rather than altering physiological thresholds.
North Atlantic kelps are generally quite young. The Arctic endemic kelp L. solidungula likely originated from the first major migration event of Pacific Laminaria through the Bering Strait around 5.3 Mya (Rothman et al., 2017). The cold-temperate species, such as L. digitata, followed later from a second migration event, which also gave rise to the warm-temperate species (Rothman et al., 2017). So, despite their ancestors crossing the Arctic from the Pacific to the Atlantic, North Atlantic kelps are mostly not Arctic in their temperature profile, except for the more distantly related L. solidungula (tom Dieck (Bartsch), 1992; tom Dieck (Bartsch), 1993; Roleda, 2016). The uniform UST of L. digitata gametophytes shown here, in combination with the ecotypic adaptation at lower temperatures, indicate that populations of this species are likely in an early stage of adaptation to cold environments, due to their relatively short cold-water history since around 3 My (sensu Wiencke et al., 1994).
Phylogeographic studies of NE Atlantic L. digitata have identified genetically distinct northern and southern clusters (King et al., 2020; Liesner et al., 2020a; Neiva et al., 2020). This genetic structure corresponds to the differentiation of thermal traits in L. digitata sporophytes (King et al., 2019; Liesner et al., 2020a) and might also explain the location-specific gametophyte survival at lower temperatures (this study). This divergence can be traced back to different post-glacial redistribution histories after the Last Glacial Maximum (20,000 YBP; Assis et al., 2017a; Neiva et al., 2020). Potential glacial refugia have been suggested near the unglaciated coastlines of the Armorican and Celtic Seas (Brittany and SW Britain; Maggs et al., 2008), and close to Ireland (Neiva et al., 2020; Schoenrock et al., 2020), which was at the border of former, heavily glaciated regions. Repeated range contractions into and expansions from these refugia may have maintained unique gene pools and facilitated genetic diversification across glacial cycles (Assis et al., 2017a; Neiva et al., 2020).
4.3 Location-Specific Temperature Effects on Sporophyte Development
Similarly, as for survival at the lower temperature end, gametophytes from Tromsø, Roscoff and Quiberon showed ecotypic responses in relative microscopic sporophyte formation per female gametophytes at the upper temperature end. This was apparent after thermal priming in high (sub)-lethal temperatures with subsequent transfer to fertility-inducing 15°C. While all three populations showed 87% to 99.8% reproductive success at continuous 15°C, indicating an optimum response, the limiting priming temperature for reproduction was 23°C in all populations. Gametophytes from the southern populations Quiberon and Roscoff exposed to priming temperatures of 20-22°C exhibited significantly higher reproductive success during recovery in optimal conditions compared to the northern population of Tromsø. Furthermore, female gametophytes from the southern-most population Quiberon showed no decline in sporophyte formation in these priming temperatures, whereas Roscoff revealed a clear negative relationship with increasing priming temperature. This variation towards thermal priming at high temperatures points towards thermal adaptation to local conditions. The impact of thermal priming on subsequent trait performance seems to be vital in explaining some of the observed trait plasticity in kelps. Recent discoveries revealed that L. digitata gametophytes from the North Sea and the Arctic (same locations as in this study) also behaved differently after recovery from short heatwave priming treatments (20-25°C), indicating ecotypic responses (Martins et al., 2020).
Within the same set of results, we additionally noted that reproductive success in gametophytes exposed to the 21°C and 22°C priming treatments was significantly different among all three populations in latitudinal sequence. Given the close geographic proximity (~1° latitude apart) and similarity in thermal environments (2°C difference in maximum monthly mean SSTs) of the two French sites, it is surprising that such a considerable difference was observed between the two. For comparison, Tromsø is situated roughly 22° latitude further north and the local annual average SST is approximately 7-8°C lower than in northern France (Chepurin and Carton, 2012; Oppliger et al., 2014). Therefore, it is likely that intraspecific variation not only plays a role between populations from the two opposite distribution edges, but also among neighboring populations themselves (Billot et al., 2003). Gene flow is low among L. digitata populations from Roscoff and Quiberon (Billot et al., 2003; Robuchon et al., 2014; Liesner et al., 2020a), which potentially facilitated differentiation between populations. The southern-most European L. digitata population of Quiberon might be under especially high selection pressure.
In the lower temperature gradient, relative sporophyte formation per female gametophyte also varied between populations, but not in a pattern that indicated adaptation to local temperatures. In fact, female gametophytes from the high-latitude population of Spitsbergen had a high relative sporophyte formation between 6-15°C, with optima at 9-12°C, compared to the low-latitude population of Quiberon which produced most sporophytes between 3-12°C, with optima at 6-9°C. The fact that reproduction in L. digitata gametophytes appears to be adapted to such a wide temperature window (3-15°C; tom Dieck (Bartsch), 1992; Müller et al., 2008; Martins et al., 2017), but not to low temperatures of 0°C, further supports the idea that the migration of L. digitata to the Arctic may have been a rather recent event (sensu Wiencke et al., 1994). On the other hand, absolute sporophyte development showed a different pattern. The Quiberon population produced more sporophytes per area at 3-9°C than the Spitsbergen population. This was likely influenced by the initial gametophyte density which was >100 gametophytes cm-2 higher in the former compared to the latter population (Table S3), providing Quiberon with more female cells that could potentially develop sporophytes. Aside from this difference between the two populations, and contrary to their relative ability to form sporophytes, absolute sporophyte development clearly peaked in a narrow range of intermediate temperatures (Quiberon at 9°C and Spitsbergen at 9-12°C). A similar mismatch between speed of gametogenesis and recruitment optima has been described before (Martins et al., 2017), pointing towards differential selection pressure on proximate ontogenetic processes, distinctively controlled by temperature.
4.4 Microscopic Sporophyte Growth Reveals No Local Adaptation
Linear GR length and GR width of microscopic sporophytes from different populations of L. digitata at 0-15°C were not obviously related to the thermal environment in which the populations occur. Although we found differences in GR length and GR width among populations (Spitsbergen, Tromsø and Quiberon), the general growth patterns were similar, with >4-fold higher GRs length and GRs width at 9-15°C than at 0-3°C. Thus, sporophyte growth at cool conditions is clearly not favored, irrespective of location. The higher GR length of the Tromsø population compared to Spitsbergen and Quiberon was likely attributable to the low reproductive success and consequently low sporophyte density of the Tromsø population, providing more space and nutrients for growth. Our results are in line with former studies that consistently showed maximum growth of juvenile L. digitata sporophytes between 10°C and 15°C (Bolton and Lüning, 1982; tom Dieck (Bartsch), 1992) and therefore do not suggest prominent ecotypic responses for this trait. The only other study investigating juvenile kelp sporophyte growth along latitudes in a common-garden approach dealt with Lessonia nigrescens. The reported thermal ecotypes in growth of microscopic sporophytes from three Chilean locations spanning 21° latitude (Martinez, 1999), were probably due to the presence of two cryptic species (Oppliger et al., 2012) and thus also do not suggest intraspecific, ecotypic adaptation of this trait.
Results of our study reveal that low-latitude populations of L. digitata, might rely on faster reproduction and sporophyte growth to minimize exposure of gametophytes to unpredictable thermal fluctuations in summer, whereas colder, high-latitude populations, that generally experience more stable thermal conditions, may benefit from delayed maturation and increased vegetative growth of gametophytes, resulting in multicellular gametophytes that can bear more offspring (Destombe and Oppliger, 2011; Oppliger et al., 2012). If slow recruitment is better in the cold, and fast recruitment better in warm temperatures, maybe it is this plasticity that is selected for. As temperatures of >9°C have not yet been encountered in the Arctic, the high temperature recruitment optimum of the Spitsbergen population may suggest that this trait has not been under selection pressure yet.
4.5 Sporophyte Morphology May Suggest Local Adaptation
In contrast to GRs, sporophyte morphology, expressed as L:W ratio, might suggest local adaptation. The southern range edge population of Quiberon showed the widest range in L:W ratio, with a maximum at 9°C. Sporophytes from Tromsø were less variable over the thermal treatments but peaked at 6°C. Spitsbergen sporophytes on the other hand, displayed a constantly low L:W ratio across all temperatures. Morphologically, this may imply that adult sporophytes from Quiberon generally grow long and thin at intermediate temperatures, whereas sporophytes from Spitsbergen grow wider rather than longer blades. It is, however, unclear whether these differences reflect local adaptation, or why a broad range in sporophyte morphology may be advantageous in warm environments as opposed to consistent morphology in cold environments. Nonetheless, whether this feature is plastic or genetically determined may have significant implications in selecting seedstocks for farming and domestication.
4.6 Implications of Thermal Responses of Gametophytes for Kelp Ecology
Intraspecific variation in thermal responses is prevalent among widely-distributed kelp species (Mohring et al., 2014; Saada et al., 2016; King et al., 2018; King et al., 2019; Liesner et al., 2020a; Martins et al., 2020). Yet, the specific mechanisms acting on different life history stages are not well-understood. We have shown that temperature-dependent responses of microscopic stages of L. digitata vary among life history stages and populations. The clear pattern of local adaptation we have identified in some traits, but not in others, may shed light on the crucial developmental processes under selection pressure and the fundamental niche of the species.
Gametophyte survival in lower (sub-) optimal temperatures and sporophyte formation after thermal priming at upper temperatures between 15°C and 23°C showed ecotypic responses across populations (Table 5). Phenotypic divergence in these traits may highlight their importance for survival in the natural environment. On the other hand, the upper survival limit of gametophytes was uniform across populations. Adapting the UST does not seem to have been under selection pressure in the past, and current evidence suggests that ocean warming is outpacing the adaptive capacity of kelp forests (Vranken et al., 2021). Sporophyte formation and growth at low temperatures showed a random response, potentially concurring with the overall low levels of local adaptation found in adult sporophytes (Liesner et al., 2020a). Consequently, variation manifests itself in different traits both in haploid and diploid stages. Examining only single parameters or life cycle stages might therefore lead to incomplete or even false interpretations, and so whole-life-cycle approaches, using a range of different fitness-related traits, are vital to reveal the complex survival strategies of kelps.
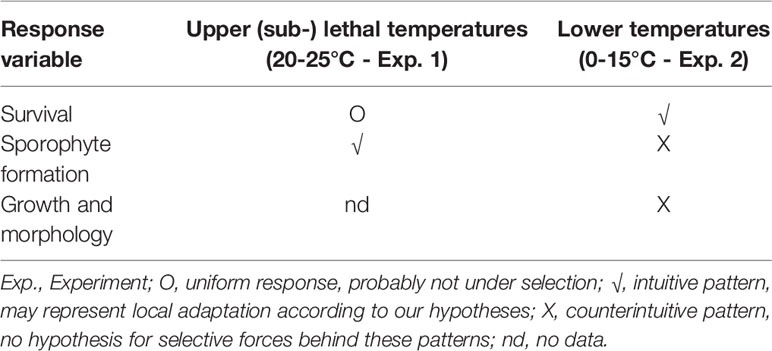
Table 5 Indication of local adaptation in Laminaria digitata gametophyte populations from across the species entire NE Atlantic distribution range with respect to different experimental temperatures and life history stages.
In conclusion, our study demonstrates trait-dependent ecotypic adaptation in the thermal performance of different microscopic life history stages of L. digitata across its entire NE Atlantic distribution. In the future, kelp forests will struggle to thermally acclimate, adapt, or shift their distribution ranges fast enough to keep up with the rising temperatures and marine heatwaves (Merzouk and Johnson, 2011). While local adaptation may delay the regional contractions of marginal populations, it is unlikely that they will cope with global warming in the long term (Liesner et al., 2020a; Neiva et al., 2020; Vranken et al., 2021). The potential for fast adaptation of kelps via thermal priming or trans-generational and epigenetic inheritance, is only starting to be understood (Liesner et al., 2020b; Gauci et al., 2022; Scheschonk et al., 2022). Therefore, the development of strategies to future-proof and restore kelp forests is of utmost importance (Wood et al., 2019; Coleman et al., 2020; Eger et al., 2020; Jueterbock et al., 2021).
Data Availability Statement
Data for this study were published open access (Schimpf et al., 2022) and can be found in the PANGAEA database: https://doi.org/10.1594/PANGAEA.943526.
Ethics Statement
Nagoya Protocol statement: Sampling in France and handling of data was conducted in accordance with the French legislation on Access and Benefit-Sharing of genetic resources (ABSCH-IRCC-FR-252418-1). With respect to Norway, we complied with our due diligence by sending an inquiry to the national authorities. In 2020, they replied that no permits were required and in late 2021 this statement was officially confirmed to the Alfred Wegener Institute by the Norwegian National Focal Point. The Norwegian regulation on the Nagoya Protocol has not yet entered into force.
Author Contributions
NS and IB designed the study, with contributions from DL and KF. DL, IB, and MR carried out field sampling. NS, KF, and DL conducted the experiments. NS analyzed the data. NS, IB, DL, KF, and MR contributed to the data interpretation and discussion. NS wrote the first draft of the manuscript. All authors contributed to manuscript revision and read and approved the submitted version.
Funding
This work was supported by the 2015-2016 BiodivERsA COFUND call for research proposals (program MARFOR), with the national funder German Research Foundation (DFG; grant no. VA 105/25-1). We acknowledge support by the Open Access Publication Funds of the Alfred Wegener Institute, Helmholtz Centre for Polar and Marine Research.
Conflict of Interest
The authors declare that the research was conducted in the absence of any commercial or financial relationships that could be construed as a potential conflict of interest.
Publisher’s Note
All claims expressed in this article are solely those of the authors and do not necessarily represent those of their affiliated organizations, or those of the publisher, the editors and the reviewers. Any product that may be evaluated in this article, or claim that may be made by its manufacturer, is not guaranteed or endorsed by the publisher.
Acknowledgments
We would like to thank A. Wagner for the sampling and clonal isolation of kelp material and technical assistance in the laboratory, C. Daniel for support with the image analysis, L. Foqueau for the SST data, C. Gauci for statistical advice and S. DeAmicis for supervision in this BSc Thesis.
Supplementary Material
The Supplementary Material for this article can be found online at: https://www.frontiersin.org/articles/10.3389/fmars.2022.870792/full#supplementary-material
References
Adey W. H., Steneck R. S. (2001). Thermogeography Over Time Creates Biogeographic Regions: A Temperature/Space/Time-Integrated Model and an Abundance-Weighted Test for Benthic Marine Algae. J. Phycol. 37, 677–698. doi: 10.1046/j.1529-8817.2001.00176.x
Assis J., Araújo M. B., Serrão E. A. (2017a). Projected Climate Changes Threaten Ancient Refugia of Kelp Forests in the North Atlantic. Glob. Change Biol. 24, e55–e66. doi: 10.1111/gcb.13818
Assis J., Berecibar E., Claro B., Alberto F., Reed D., Raimondi P., et al. (2017b). Major Shifts at the Range Edge of Marine Forests: The Combined Effects of Climate Changes and Limited Dispersal. Sci. Rep. 7, 44348. doi: 10.1038/srep44348
Atkins K. E., Travis J. M. J. (2010). Local Adaptation and the Evolution of Species’ Ranges Under Climate Change. J. Theor. Biol. 266, 449–457. doi: 10.1016/j.jtbi.2010.07.014
Barradas A., Alberto F., Engelen A. H., Serrão E. A. (2011). Fast Sporophyte Replacement After Removal Suggests Banks of Latent Microscopic Stages of Laminaria Ochroleuca (Phaeophyceae) in Tide Pools in Northern Portugal. Cah. Biol. Mar. 52, 435–439.
Bartsch I. (2018). “Derivation of Clonal Stock Cultures and Hybridization of Kelps,” in Protocols for Macroalgae Research. Eds. Charrier B., Wichard T., Reddy C. R. K. (Boca Raton, FL: CRC Press), 61–78. doi: 10.1201/b21460-3
Bartsch I., Vogt J., Pehlke C., Hanelt D. (2013). Prevailing Sea Surface Temperatures Inhibit Summer Reproduction of the Kelp Laminaria Digitata at Helgoland (North Sea). J. Phycol. 49, 1061–1073. doi: 10.1111/jpy.12125
Becheler R., Haverbeck D., Clerc C., Montecinos G., Valero M., Mansilla A., et al. (2022). Variation in Thermal Tolerance of the Giant Kelp’s Gametophytes: Suitability of Habitat, Population Quality or Local Adaptation? Front. Mar. Sci. In Press.
Billot C., Engel C. R., Rousvoal S., Kloareg B., Valero M. (2003). Current Patterns, Habitat Discontinuities and Population Genetic Structure: The Case of the Kelp Laminaria Digitata in the English Channel. Mar. Ecol. Prog. Ser. 253, 111–121. doi: 10.3354/meps253111
Blamey L. K., Bolton J. J. (2018). The Economic Value of South African Kelp Forests and Temperate Reefs: Past, Present and Future. J. Mar. Syst. 188, 172–181. doi: 10.1016/j.jmarsys.2017.06.003
Bolton J. J., Lüning K. (1982). Optimal Growth and Maximal Survival Temperatures of Atlantic Laminaria Species (Phaeophyta) in Culture. Mar. Biol. 66, 89–94. doi: 10.1007/BF00397259
Carney L. T., Edwards M. S. (2006). Cryptic Processes in the Sea: A Review of Delayed Development in the Microscopic Life Stages of Marine Macroalgae. Algae 21, 161–168. doi: 10.4490/ALGAE.2006.21.2.161
Carney L. T., Edwards M. S. (2010). Role of Nutrient Fluctuations and Delayed Development in Gametophyte Reproduction by Macrocystis Pyrifera (Phaeophyceae) in Southern California. J. Phycol. 46, 987–996. doi: 10.1111/j.1529-8817.2010.00882.x
Chepurin G. A., Carton J. A. (2012). Subarctic and Arctic Sea Surface Temperature and its Relation to Ocean Heat Content 1982–2010. J. Geophys. Res. 117, C06019. doi: 10.1029/2011JC007770
Coleman M. A., Wood G., Filbee-Dexter K., Minne A. J. P., Goold H. D., Vergés A., et al. (2020). Restore or Redefine: Future Trajectories for Restoration. Front. Mar. Sci. 7. doi: 10.3389/fmars.2020.00237
Cosson J. (1999). Sur La Disparition Progressive De Laminaria Digitata Sur Les Côtes Du Calvados (France). Cryptogam. Algol. 20, 35–42. doi: 10.1016/S0181-1568(99)80005-1
Dayton P. K., Tegner M. J. (1984). Catastrophic Storms, El Niño, and Patch Stability in a Southern California Kelp Community. Science 224, 283–285. doi: 10.1126/science.224.4646.283
Destombe C., Oppliger L. V. (2011). Male Gametophyte Fragmentation in Laminaria Digitata: A Life History Strategy to Enhance Reproductive Success. Cah. Biol. Mar. 52, 385–394.
Edwards M. S. (2000). The Role of Alternate Life-History Stages of a Marine Macroalga: A Seed Bank Analogue? Ecology 81, 2404–2415. doi: 10.2307/177463
Edwards M. S. (2022). It’s the Little Things: The Role of Microscopic Life Stages in Maintaining Kelp Populations. Front. Mar. Sci. 9. doi: 10.3389/fmars.2022.871204
Eger A. M., Marzinelli E., Baes R., Blain C., Blamey L., Carnell P. E., et al. (2021). The Economic Value of Fisheries, Blue Carbon, and Nutrient Cycling in Global Marine Forests. EcoEvoRxiv [Preprint]. doi: 10.32942/osf.io/n7kjs
Eger A. M., Marzinelli E., Gribben P., Johnson C. R., Layton C., Steinberg P. D., et al. (2020). Playing to the Positives: Using Synergies to Enhance Kelp Forest Restoration. Front. Mar. Sci. 7. doi: 10.3389/fmars.2020.00544
Eggert A. (2012). “Seaweed Responses to Temperature,” in Seaweed Biology: Novel Insights Into Ecophysiology, Ecology and Utilization, Ecological Studies. Eds. Wiencke C., Bischof K. (Berlin, Heidelberg: Springer), 47–66. doi: 10.1007/978-3-642-28451-9_3
E.U. Copernicus Marine Service (2021) Global Ocean OSTIA Sea Surface Temperature and Sea Ice Analysis. Available at: https://resources.marine.copernicus.eu/products.
European Environment Agency (2021) EEA Coastline – Polyline. Available at: https://www.eea.europa.eu/data-and-maps/data/eea-coastline-for-analysis-2/gis-data/eea-coastline-polyline.
Fernández C. (2011). The Retreat of Large Brown Seaweeds on the North Coast of Spain: The Case of Saccorhiza Polyschides. Eur. J. Phycol. 46, 352–360. doi: 10.1080/09670262.2011.617840
Filbee-Dexter K., Wernberg T. (2018). Rise of Turfs: A New Battlefront for Globally Declining Kelp Forests. BioScience 68, 64–76. doi: 10.1093/biosci/bix147
Filbee-Dexter K., Wernberg T., Fredriksen S., Norderhaug K. M., Pedersen M. F. (2019). Arctic Kelp Forests: Diversity, Resilience and Future. Global Planet. Change 172, 1–14. doi: 10.1016/j.gloplacha.2018.09.005
Filbee-Dexter K., Wernberg T., Grace S. P., Thormar J., Fredriksen S., Narvaez C. N., et al. (2020). Marine Heatwaves and the Collapse of Marginal North Atlantic Kelp Forests. Sci. Rep. 10, 13388. doi: 10.1038/s41598-020-70273-x
Fischer P., Schwanitz M., Brand M., Posner U., Gattuso J.-P., Alliouane S., et al. (2019). Hydrographical Time Series Data of the Littoral Zone of Kongsfjorden, Svalbard 2018 (Alfred Wegener Institute - Biological Institute Helgoland, PANGAEA). doi: 10.1594/PANGAEA.897349
Franke K., Liesner D., Heesch S., Bartsch I. (2021). Looks can be Deceiving: Contrasting Temperature Characteristics of Two Morphologically Similar Kelp Species Co-Occurring in the Arctic. Bot. Mar. 64, 163–175. doi: 10.1515/bot-2021-0014
Gauci C., Bartsch I., Martins N., Liesner D. (2022). Cold Thermal Priming of Laminaria Digitata (Laminariales, Phaeophyceae) Gametophytes Enhances Gametogenesis and Thermal Performance of Sporophytes. Front. Mar. Sci. 9. doi: 10.3389/fmars.2022.862923
Graham M. H., Kinlan B. P., Druehl L. D., Garske L. E., Banks S. (2007). Deep-Water Kelp Refugia as Potential Hotspots of Tropical Marine Diversity and Productivity. Proc. Natl. Acad. Sci. U S A 104, 16576–16580. doi: 10.1073/pnas.0704778104
Hansen M. M., Nielsen E. E., Mensberg K.-L. D. (2006). Underwater But Not Out of Sight: Genetic Monitoring of Effective Population Size in the Endangered North Sea Houting (Coregonus Oxyrhynchus). Can. J. Fish. Aquat. Sci. 63, 780–787. doi: 10.1139/F05-260
IPCC (2014). Climate Change 2014: Synthesis Report. Contribution of Working Groups I, II and III to the Fifth Assessment Report of the Intergovernmental Panel on Climate Change. Eds. Core Writing Team, Pachauri R. K., Meyer L. A. (Geneva, Switzerland: IPCC), 151.
Izquierdo J., Pérez-Ruzafa I. M., Gallardo T. (2002). Effect of Temperature and Photon Fluence Rate on Gametophytes and Young Sporophytes of Laminaria Ochroleuca Pylaie. Helgol. Mar. Res. 55, 285–292. doi: 10.1007/s10152-001-0087-6
Jueterbock A., Minne A. J. P., Cock J. M., Coleman M. A., Wernberg T., Scheschonk L., et al. (2021). Priming of Marine Macrophytes for Enhanced Restoration Success and Food Security in Future Oceans. Front. Mar. Sci. 8. doi: 10.3389/fmars.2021.658485
Jueterbock A., Tyberghein L., Verbruggen H., Coyer J. A., Olsen J. L., Hoarau G. (2013). Climate Change Impact on Seaweed Meadow Distribution in the North Atlantic Rocky Intertidal. Ecol. Evol. 3, 1356–1373. doi: 10.1002/ece3.541
King N. G., McKeown N. J., Smale D. A., Bradbury S., Stamp T., Jueterbock A., et al. (2020). Hierarchical Genetic Structuring in the Cool Boreal Kelp, Laminaria Digitata: Implications for Conservation and Management. ICES J. Mar. Sci. 77, 1906–1913. doi: 10.1093/icesjms/fsaa055
King N. G., McKeown N. J., Smale D. A., Moore P. J. (2018). The Importance of Phenotypic Plasticity and Local Adaptation in Driving Intraspecific Variability in Thermal Niches of Marine Macrophytes. Ecography 41, 1469–1484. doi: 10.1111/ecog.03186
King N. G., McKeown N. J., Smale D. A., Wilcockson D. C., Hoelters L., Groves E. A., et al. (2019). Evidence for Different Thermal Ecotypes in Range Centre and Trailing Edge Kelp Populations. J. Exp. Mar. Biol. Ecol. 514–515, 10–17. doi: 10.1016/j.jembe.2019.03.004
Krause-Jensen D., Archambault P., Assis J., Bartsch I., Bischof K., Filbee-Dexter K., et al. (2020). Imprint of Climate Change on Pan-Arctic Marine Vegetation. Front. Mar. Sci. 7. doi: 10.3389/fmars.2020.617324
Ladah L. B., Zertuche-González J. A. (2007). Survival of Microscopic Stages of a Perennial Kelp (Macrocystis Pyrifera) From the Center and the Southern Extreme of its Range in the Northern Hemisphere After Exposure to Simulated El Niño Stress. Mar. Biol. 152, 677–686. doi: 10.1007/s00227-007-0723-z
Lee J. A., Brinkhuis B. H. (1988). Seasonal Light and Temperature Interaction Effects on Development of Laminaria Saccharina (Phaeophyta) Gametophytes and Juvenile Sporophytes. J. Phycol. 24, 181–191. doi: 10.1111/j.1529-8817.1988.tb00076.x
Lewis R. J., Green M. K., Afzal M. E. (2013). Effects of Chelated Iron on Oogenesis and Vegetative Growth of Kelp Gametophytes (Phaeophyceae). Phycol. Res. 61, 46–51. doi: 10.1111/j.1440-1835.2012.00667.x
Liesner D., Fouqueau L., Valero M., Roleda M. Y., Pearson G. A., Bischof K., et al. (2020a). Heat Stress Responses and Population Genetics of the Kelp Laminaria Digitata (Phaeophyceae) Across Latitudes Reveal Differentiation Among North Atlantic Populations. Ecol. Evol. 10, 9144–9177. doi: 10.1002/ece3.6569
Liesner D., Shama L. N. S., Diehl N., Valentin K., Bartsch I. (2020b). Thermal Plasticity of the Kelp Laminaria Digitata (Phaeophyceae) Across Life Cycle Stages Reveals the Importance of Cold Seasons for Marine Forests. Front. Mar. Sci. 7. doi: 10.3389/fmars.2020.00456
Lima F. P., Ribeiro P. A., Queiroz N., Hawkins S. J., Santos A. M. (2007). Do Distributional Shifts of Northern and Southern Species of Algae Match the Warming Pattern? Glob. Change Biol. 13, 2592–2604. doi: 10.1111/j.1365-2486.2007.01451.x
Lüning K. (1980). Critical Levels of Light and Temperature Regulating the Gametogenesis of Three Laminaria Species (Phaeophyceae). J. Phycol. 16, 1–15. doi: 10.1111/j.1529-8817.1980.tb02992.x
Lüning K. (1984). Temperature Tolerance and Biogeography of Seaweeds: The Marine Algal Flora of Helgoland (North Sea) as an Example. Helgol. Meeresunters. 38, 305–317. doi: 10.1007/BF01997486
Lüning K. (1990). Seaweeds: Their Environment, Biogeography and Ecophysiology (New York: John Wiley & Sons).
Maggs C. A., Castilho R., Foltz D., Henzler C., Jolly M. T., Kelly J., et al. (2008). Evaluating Signatures of Glacial Refugia for North Atlantic Benthic Marine Taxa. Ecology 89, S108–S122. doi: 10.1890/08-0257.1
Martinez E. A. (1999). Latitudinal Differences in Thermal Tolerance Among Microscopic Sporophytes of the Kelp Lessonia Nigrescens (Phaeophyta: Laminariales). Pac. Sci. 53, 74–81.
Martins N., Pearson G. A., Bernard J., Serrão E. A., Bartsch I. (2020). Thermal Traits for Reproduction and Recruitment Differ Between Arctic and Atlantic Kelp Laminaria Digitata. PLos One 15, e0235388. doi: 10.1371/journal.pone.0235388
Martins N., Tanttu H., Pearson G. A., Serrão E. A., Bartsch I. (2017). Interactions of Daylength, Temperature and Nutrients Affect Thresholds for Life Stage Transitions in the Kelp Laminaria Digitata (Phaeophyceae). Bot. Mar. 60, 109–121. doi: 10.1515/bot-2016-0094
Merzouk A., Johnson L. E. (2011). Kelp Distribution in the Northwest Atlantic Ocean Under a Changing Climate. J. Exp. Mar. Biol. Ecol. 400, 90–98. doi: 10.1016/j.jembe.2011.02.020
Mohring M. B., Wernberg T., Wright J. T., Connell S. D., Russell B. D. (2014). Biogeographic Variation in Temperature Drives Performance of Kelp Gametophytes During Warming. Mar. Ecol. Prog. Ser. 513, 85–96. doi: 10.3354/meps10916
Monteiro C., Heinrich S., Bartsch I., Valentin K., Corre E., Collén J., et al. (2019). Temperature Modulates Sex-Biased Gene Expression in the Gametophytes of the Kelp Saccharina Latissima. Front. Mar. Sci. 6. doi: 10.3389/fmars.2019.00769
Moy F. E., Christie H. (2012). Large-Scale Shift From Sugar Kelp (Saccharina Latissima) to Ephemeral Algae Along the South and West Coast of Norway. Mar. Biol. Res. 8, 309–321. doi: 10.1080/17451000.2011.637561
Müller R., Laepple T., Bartsch I., Wiencke C. (2009). Impact of Oceanic Warming on the Distribution of Seaweeds in Polar and Cold-Temperate Waters. Bot. Mar. 52, 617–638. doi: 10.1515/BOT.2009.080
Müller R., Wiencke C., Bischof K. (2008). Interactive Effects of UV Radiation and Temperature on Microstages of Laminariales (Phaeophyceae) From the Arctic and North Sea. Clim. Res. 37, 203–213. doi: 10.3354/cr00762
Neiva J., Serrão E. A., Paulino C., Gouveia L., Want A., Tamigneaux É., et al. (2020). Genetic Structure of Amphi-Atlantic Laminaria Digitata (Laminariales, Phaeophyceae) Reveals a Unique Range-Edge Gene Pool and Suggests Post-Glacial Colonization of the NW Atlantic. Eur. J. Phycol. 55, 517–528. doi: 10.1080/09670262.2020.1750058
Oliver E. C. J., Benthuysen J. A., Darmaraki S., Donat M. G., Hobday A. J., Holbrook N. J., et al. (2021). Marine Heatwaves. Annu. Rev. Mar. Sci. 13, 313–342. doi: 10.1146/annurev-marine-032720-095144
Oppliger L. V., Correa J. A., Engelen A. H., Tellier F., Vieira V., Faugeron S., et al. (2012). Temperature Effects on Gametophyte Life-History Traits and Geographic Distribution of Two Cryptic Kelp Species. PLos One 7, e39289. doi: 10.1371/journal.pone.0039289
Oppliger L. V., von Dassow P., Bouchemousse S., Robuchon M., Valero M., Correa J. A., et al. (2014). Alteration of Sexual Reproduction and Genetic Diversity in the Kelp Species Laminaria Digitata at the Southern Limit of its Range. PLos One 9, e102518. doi: 10.1371/journal.pone.0102518
Pehlke C., Bartsch I. (2008). Changes in Depth Distribution and Biomass of Sublittoral Seaweeds at Helgoland (North Sea) Between 1970 and 2005. Clim. Res. 37, 135–147. doi: 10.3354/CR00767
Provasoli L. (1968). “Media and Prospects for the Cultivation of Marine Algae. In Cultures and Collections of Algae,” in Proceedings of the US-Japan Conference, Hakone, September 1966. Eds. Watanabe A., Hattori A. (Tokyo: Japan Society of Plant Physiology), 63–75.
QGIS Development Team (2019) QGIS Geographic Information System. Open Source Geospatial Foundation. Available at: https://qgis.org.
Raybaud V., Beaugrand G., Goberville E., Delebecq G., Destombe C., Valero M., et al. (2013). Decline in Kelp in West Europe and Climate. PLos One 8, e66044. doi: 10.1371/journal.pone.0066044
R Core Team (2020). R: A Language and Environment for Statistical Computing. R Foundation for Statistical Computing (Vienna, Austria). Available at: https://www.R-project.org/.
Reed D. C., Anderson T. W., Ebeling A. W., Anghera M. (1997). The Role of Reproductive Synchrony in the Colonization Potential of Kelp. Ecology 78, 2443–2457. doi: 10.2307/2265905
Robuchon M., Le Gall L., Mauger S., Valero M. (2014). Contrasting Genetic Diversity Patterns in Two Sister Kelp Species Co-Distributed Along the Coast of Brittany, France. Mol. Ecol. 23, 2669–2685. doi: 10.1111/mec.12774
Roleda M. Y. (2009). Photosynthetic Response of Arctic Kelp Zoospores Exposed to Radiation and Thermal Stress. Photochem. Photobiol. Sci. 8, 1302–1312. doi: 10.1039/b901098j
Roleda M. Y. (2016). Stress Physiology and Reproductive Phenology of Arctic Endemic Kelp Laminaria Solidungula J. Agardh. Polar. Biol. 39, 1967–1977. doi: 10.1007/s00300-015-1813-x
Rothman M. D., Mattio L., Anderson R. J., Bolton J. J., Verbruggen H. (2017). A Phylogeographic Investigation of the Kelp Genus Laminaria (Laminariales, Phaeophyceae), With Emphasis on the South Atlantic Ocean. J. Phycol. 53, 778–789. doi: 10.1111/jpy.12544
Saada G., Nicastro K. R., Jacinto R., McQuaid C. D., Serrão E. A., Pearson G. A., et al. (2016). Taking the Heat: Distinct Vulnerability to Thermal Stress of Central and Threatened Peripheral Lineages of a Marine Macroalga. Divers. Distrib. 22, 1060–1068. doi: 10.1111/ddi.12474
Santelices B., Hoffmann A. J., Aedo D., Bobadilla M., Otaíza R. (1995). A Bank of Microscopic Forms on Disturbed Boulders and Stones in Tide Pools. Mar. Ecol. Prog. Ser. 129, 215–228. doi: 10.3354/meps129215
Scheschonk L., Bischof K., Kopp M. E. L., Jueterbock A. (2022). Differences by Origin in Methylome Suggest Eco-Phenotypes in the Kelp Saccharina Latissima. Evol. Appl. Advance online publication. doi: 10.1111/eva.13382
Schimpf N. M., Liesner D., Franke K., Roleda M. Y., Bartsch I. (2022). Local Adaptation of Microscopic Stages of North Atlantic Laminaria digitata to High and Low Temperatures Along Latitudes: Survival, Reproduction and Growth. PANGAEA. doi: 10.1594/PANGAEA.943526
Schoenrock K. M., O’Connor A. M., Mauger S., Valero M., Nieva J., Serrão E. A., et al. (2020). Genetic Diversity of a Marine Foundation Species, Laminaria Hyperborea (Gunnerus) Foslie, Along the West Coast of Ireland. Eur. J. Phycol. 55, 310–326. doi: 10.1080/09670262.2020.1724338
Shea R., Chopin T. (2007). Effects of Germanium Dioxide, an Inhibitor of Diatom Growth, on the Microscopic Laboratory Cultivation Stage of the Kelp, Laminaria Saccharina. J. Appl. Phycol. 19, 27–32. doi: 10.1007/s10811-006-9107-x
Silva C. F., Pearson G. A., Serrão E. A., Bartsch I., Martins N. (2022). Microscopic Life Stages of Arctic Kelp Differ in Their Resilience and Reproductive Output in Response to Arctic Seasonality. Eur. J. Phycol. Advance online publication. doi: 10.1080/09670262.2021.2014983
Smale D. A., Wernberg T. (2013). Extreme Climatic Event Drives Range Contraction of a Habitat-Forming Species. Proc. R. Soc. B. Biol. Sci. 280, 1–9. doi: 10.1098/rspb.2012.2829
Smale D. A., Wernberg T., Vanderklift M. A. (2017). Regional-Scale Variability in the Response of Benthic Macroinvertebrate Assemblages to a Marine Heatwave. Mar. Ecol. Prog. Ser. 568, 17–30. doi: 10.3354/meps12080
Smale D. A., Wernberg T., Yunnie A. L. E., Vance T. (2015). The Rise of Laminaria Ochroleuca in the Western English Channel (UK) and Comparisons With its Competitor and Assemblage Dominant Laminaria Hyperborea. Mar. Ecol. 36, 1033–1044. doi: 10.1111/maec.12199
Tatewaki M. (1966). Formation of a Crustaceous Sporophyte With Unilocular Sporangia in Scytosiphon Lomentaria. Phycologia 6, 62–66. doi: 10.2216/i0031-8884-6-1-62.1
Teagle H., Hawkins S. J., Moore P. J., Smale D. A. (2017). The Role of Kelp Species as Biogenic Habitat Formers in Coastal Marine Ecosystems. J. Exp. Mar. Biol. Ecol. 492, 81–98. doi: 10.1016/j.jembe.2017.01.017
tom Dieck (Bartsch) I. (1992). North Pacific and North Atlantic Digitate Laminaria Species (Phaeophyta): Hybridization Experiments and Temperature Responses. Phycologia 31, 147–163. doi: 10.2216/i0031-8884-31-2-147.1
tom Dieck (Bartsch) I. (1993). Temperature Tolerance and Survival in Darkness of Kelp Gametophytes (Laminariales, Phaeophyta): Ecological and Biogeographical Implications. Mar. Ecol. Prog. Ser. 100, 253–264. doi: 10.3354/meps100253
Underwood A. J. (1996). “Analysis of Variance”, in Experiments in Ecology: Their Logical Design and Interpretation Using Analysis of Variance (Cambridge: Cambridge University Press), 140–197. doi: 10.1017/CBO9780511806407.007
van den Hoek C., Mann D. G., Jahns H. M. (1995). Algae: An Introduction to Phycology (Cambridge: Cambridge University Press).
Vásquez J. A., Zuñiga S., Tala F., Piaget N., Rodríguez D. C., Vega J. M. A. (2014). Economic Valuation of Kelp Forests in Northern Chile: Values of Goods and Services of the Ecosystem. J. Appl. Phycol. 26, 1081–1088. doi: 10.1007/s10811-013-0173-6
Voerman S. E., Llera E., Rico J. M. (2013). Climate Driven Changes in Subtidal Kelp Forest Communities in NW Spain. Mar. Environ. Res. 90, 119–127. doi: 10.1016/j.marenvres.2013.06.006
Vranken S., Wernberg T., Scheben A., Severn-Ellis A. A., Batley J., Bayer P. E., et al. (2021). Genotype–Environment Mismatch of Kelp Forests Under Climate Change. Mol. Ecol. 30, 3730–3746. doi: 10.1111/mec.15993
Wernberg T., Coleman M. A., Bennett S., Thomsen M. S., Tuya F., Kelaher B. P. (2018). Genetic Diversity and Kelp Forest Vulnerability to Climatic Stress. Sci. Rep. 8, 1851. doi: 10.1038/s41598-018-20009-9
Wiencke C., Bartsch I., Bischoff B., Peters A. F., Breemann A. M. (1994). Temperature Requirements and Biogeography of Antarctic, Arctic and Amphiequatorial Seaweeds. Bot. Mar. 37, 247–259. doi: 10.1515/botm.1994.37.3.247
Keywords: kelp, gametophytes, temperature, local adaptation, North Atlantic, latitudinal gradient, populations, thermal priming
Citation: Schimpf NM, Liesner D, Franke K, Roleda MY and Bartsch I (2022) Microscopic Stages of North Atlantic Laminaria digitata (Phaeophyceae) Exhibit Trait-Dependent Thermal Adaptation Along Latitudes. Front. Mar. Sci. 9:870792. doi: 10.3389/fmars.2022.870792
Received: 07 February 2022; Accepted: 09 May 2022;
Published: 17 June 2022.
Edited by:
Bernardo Antonio Perez Da Gama, Fluminense Federal University, BrazilReviewed by:
Trine Bekkby, Norwegian Institute for Water Research (NIVA), NorwayLydia Ladah, Center for Scientific Research and Higher Education in Ensenada (CICESE), Mexico
Copyright © 2022 Schimpf, Liesner, Franke, Roleda and Bartsch. This is an open-access article distributed under the terms of the Creative Commons Attribution License (CC BY). The use, distribution or reproduction in other forums is permitted, provided the original author(s) and the copyright owner(s) are credited and that the original publication in this journal is cited, in accordance with accepted academic practice. No use, distribution or reproduction is permitted which does not comply with these terms.
*Correspondence: Nele M. Schimpf, bmVsZS5zY2hpbXBmQGdteC5uZXQ=