- 1Integrated Molecular Ecology Department, Stazione Zoologica Anton Dohrn, Naples, Italy
- 2School of Geographical & Earth Sciences, University of Glasgow, Glasgow, United Kingdom
- 3Biology Department, University of Cadiz, Cadiz, Spain
- 4Marine Plant Ecology Research Group, Centre for Marine Sciences, University of Algarve, Faro, Portugal
- 5Physics and Chemistry Department, University of Cadiz, Cadiz, Spain
- 6Department of Ecology and Environmental Sciences, Umeå Marine Sciences Centre, Umeå University, Umeå, Sweden
- 7UMR ENTROPIE, LabEx CORAIL, University of Reunion, Reunion, France
Seagrasses are gaining attention thanks to their metabolism and potential major role as carbon sinks, with further implications as nature-based solutions against climate change. Despite their recognized importance and the growing number of studies published, there is still a striking paucity of information on seagrass metabolism and contribution to biogeochemical cycles for some seagrass species and ocean areas. In this study we assessed the metabolic balance and nutrient cycling contribution of seagrasses to the benthic compartment of a tropical reef lagoon in Reunion Island, providing original information on a barely studied seagrass species (Syringodium isoetifolium) and a poorly studied ocean region (West Indian Ocean). We measured the net productivity, respiration and the metabolic balance in different components of the lagoon benthic compartment (i.e. seagrass, sediment, and benthic community) and the water-sediment nutrient benthic fluxes at differently impacted sites within the lagoon. The biogeochemical environmental variability, including inorganic and organic indicators of anthropogenic contamination, was also assessed at each site.
Large spatial variability was detected in the metabolic balance of each benthic component assessed, also associated with the natural and/or anthropic-driven environmental variability found in the lagoon. The seagrass S. isoetifolium was net autotrophic across the lagoon and contributed to the lagoon benthic metabolism with net plant productivity exceeding by one order of magnitude the plant respiration. The lowest seagrass metabolism was detected at the impacted site. The metabolic balance of the sediment was heterotrophic but the high productivity of S. isoetifolium contributed to reducing the heterotrophy of the whole benthic community. The lagoon-wide benthic metabolic balance was slightly heterotrophic, but the associated uncertainty ranged from autotrophy to heterotrophy. Nutrient concentrations in the lagoon were low and the benthic community capacity for nutrient retention (uptake) and removal (denitrification and anammox) indicated potential for buffering moderate nutrient inputs into the lagoon. Organic contaminants of emerging concern (CECs) were low but detectable in the lagoon, especially in highly frequented beach areas, arising as an environmental quality indicator of interest.
1 Introduction
Within the coral reef systems, the reef lagoonal benthic communities (sensu lato) play a key role in the overall reef primary production (Gattuso et al., 1998; Heil et al., 2004), significantly contributing to inorganic carbon (CO2) fluxes and organic matter remineralization (Gattuso et al., 1998; Borges et al., 2006). The impacts of local anthropogenic pressures, such as eutrophication (Bell, 1992) or the increase of contaminants of emerging concern (CECs) (Reid et al., 2019), and climate change (Hughes et al., 2003) pose a serious threat to the biodiversity, resilience and functioning of these valuable ecosystems. As a consequence of natural or human-driven pressures, shifts in the composition of benthic primary producer and productivity are expected, implying changes in the benthic metabolism, the sediment biogeochemical composition, and the nutrient water-sediment fluxes (Eyre and Ferguson, 2002; Smetacek and Zingone, 2013). Nutrient fluxes in the benthic compartment are controlled by biogeochemical processes closely tied to the metabolic activity of the benthic community (Risgaard-Petersen and Ottosen, 2000; Barrón et al., 2006). Changes in the physico-chemical environmental conditions may impact the biological community (composition, functional role and productivity), which in turn may also modify the chemical and physical conditions and processes taking place in the sediments, ultimately affecting nutrient fluxes, cycling and storage capacity of the benthic compartment (Eyre and Ferguson, 2002). The study of the metabolic status of the benthic reef compartment, in terms of production and respiration and nutrient fluxes, is thus essential for the determination of the metabolic status (i.e. auto- or heterotrophic) of coral reef systems and for forecasting their potential to cope and respond to events of natural or anthropogenic-induced environmental forcing (Eyre and Ferguson, 2002; Taddei et al., 2007).
While microphytobentos represents the main primary producer in unvegetated soft-bottom lagoons (Taddei et al., 2007; Hochard et al., 2012), the presence of marine macrophytes, macroalgae and seagrasses, is also frequent in the reef lagoons and may constitute important contributors to primary production and the overall metabolic balance of reef system (Gattuso et al., 1998; Heil et al., 2004). Seagrasses are considered “foundation” species with the capacity to modify the physico-chemical environment around them creating suitable conditions for themselves and for the associated community (Dayton, 1972; Ricart et al., 2021). Moreover, seagrass meadows are gaining further momentum among the scientific and stake-holder communities because of the ecosystem services attributed to them and their potential as nature-based solutions for climate change mitigation (Seddon et al., 2020; Macreadie et al., 2021). The characteristic below-ground rhizomatic-root system of seagrasses physically connects the water mass and the sediment altering the diffusive water-sediment nutrient fluxes and further modifying the magnitude and velocity of the biogeochemical processes that occur both in the water and the sediment (Bouma et al., 2009). Among the valuable essential services attributed to seagrasses (Orth et al., 2006), those related to water oxygenation (Duarte et al., 2010), nutrient cycling (Eyre and Ferguson, 2002) and carbon sequestration (Fourqurean et al., 2012) are closely linked to seagrass productivity and metabolic balance. Indeed, seagrass meadows are considered among the most productive coastal systems on Earth (Duarte and Chiscano, 1999). However, despite the studies published, information on seagrass metabolism is yet inconclusive and large uncertainties remain on the quantification of seagrass net metabolic contribution to the system productivity and nutrient fluxes (Duarte et al., 2010; Van Dam Bryce et al., 2021; Ward et al., 2022). Large inter- and intraspecific variability has been described in seagrass productivity (Duarte et al., 2010). This plasticity has been associated to spatio-temporal variability (Enríquez et al., 2004; Olivé et al., 2013), environmental forcing derived from natural- or anthropogenic-driven perturbations, such as diseases, nutrient-derived eutrophication, or warming (Olivé et al., 2009; Graham et al., 2021), and “top-down” control of the associated community (Martínez-Crego et al., 2014).
Despite their recognized importance and the growing number of studies published in the last decades, seagrasses have been traditionally overlooked in coral reef systems (Duarte et al., 2008). At present, there is a particular striking paucity of information on seagrass metabolism and contribution to biogeochemical cycles in some tropical areas, such as the West Indian Ocean (Duarte et al., 2010; Ward et al., 2022). Moreover, information available on tropical seagrasses is biased and very limited information is available for some species, such is the case of Syringodium isoetifolium (Duarte et al., 2010; Ward et al., 2022). To help fulfilling this gap, this work provides novel and original information on the metabolism of a poorly studied seagrass species (i.e. S. isoetifolium) and a poorly studied ocean region (i.e. West Indian Ocean).
This work aims at evaluating the productivity and metabolic contribution of a tropical seagrass to the metabolism and nutrient cycling of the benthic compartment in a reef lagoon system, considering the environmental biogeochemical variability associated to the lagoon. This was addressed by evaluating the metabolic balance of different benthic components (i.e. seagrass, sediment, and whole benthic community) and the nutrient fluxes of the benthic community across a tropical reef lagoon. We used the reef lagoon in Reunion Island (West Indian Ocean) as a model system and the seagrass S. isoetifolium as a model species. The fast-growing species S. isoetifolium is the only seagrass present in Reunion (Cuvillier et al., 2017). It thrives in sandy bottoms along the inner reef lagoon and its coverture can reach up to 30% of the lagoon (Cuvillier et al., 2017). Despite its limited extension, the reef lagoon is exposed to different levels of natural and anthropogenic environmental pressure (Chazottes et al., 2008; Clavier et al., 2008). Indeed, time-series analysis have revealed large seascape variability of S. isoetifolium communities across the lagoon associated with natural (e.g. storms, grazing) and anthropogenic (e.g. warming, nutrient inputs) environmental forcing (Cuvillier et al., 2017). However, the productivity and metabolic assessment of the seagrass S. isoetifolium in the lagoon remains unexplored. Oxygen was chosen as the main descriptor to assess productivity and explore the metabolic balance in the different components of the benthic compartment of the lagoon together with its spatial variability. The oxygen metabolic balance has been commonly used as a physiological indicator of the metabolic status across organismal scales (Gillooly et al., 2001), benthic communities (Roth et al., 2019), and the benthic compartment (Revsbech et al., 1981; Glud, 2008). Oxygen fluxes are also closely associated with other nutrient fluxes (e.g. carbon (Duarte et al., 2010) and nitrogen (Risgaard-Petersen, 2003). Nitrogen fluxes in the benthic compartment were also assessed to evaluate the potential role of the benthic community as sink or source of nutrients in the lagoon as well as testing the potential of the benthic lagoon community to face increases in the nutrient load.
2 Material and methods
2.1 Study site
Reunion Island (Mascarene Archipelago, Western Indian Ocean) has been identified as a marine hotspot of biodiversity for coral reefs with highest ecological value (Roberts et al., 2002). The coral reef systems of Reunion Island (S 21°, E 55°) are restricted to the western and southern coasts of the island. This study was conducted in La Saline fringing reef (9 km long, up to 500 m wide, 1 to 1.5 m deep), which is the most extensive reef of Reunion Island (Tedetti et al., 2020). The lagoon is exposed to semidiurnal tides ranging from 0.1 m (neap) to 0.9 m (spring). The sediment in the reef lagoon is mainly composed of coarse sand, scattered with coral fragments, over a limestone substrate (Clavier et al., 2008). The lagoon is considered an oligotrophic system with low nutrient levels, though local areas potentially exposed to nutrient inputs, mainly through groundwater discharges and runoff from land, have been reported along the lagoon (Clavier et al., 2008; Tedetti et al., 2020).
Monospecific beds of the seagrass Syringodium isoetifolium were selected according to previous works and three different stations were set along the reef lagoon as a representative of different environmental pressures (i.e. nutrient enrichment associated to submarine groundwater discharge and human pressure) (Chazottes et al., 2008; Cuvillier et al., 2017). “Saint-Gilles” (Site A, STG, S 21.07020°; E 055.22031°) was selected as pristine meadow with low anthropogenic pressure and little or no nutrient enrichment; “Passe Ermitage” (Site B, PAS, S 21.08629°; E 055.22705°), located close to an intermittent stream receiving the effluent of a sewage plant, was selected as impacted site exposed to high submarine groundwater discharge, nutrient inputs, and hydrodynamics; and “Saline” (Site C, SAL, S 21.09597°; E 055.23338°), a frequented beach area, was selected as representative of intermediate impact with moderate human pressure and average nutrient availability (Figure 1).
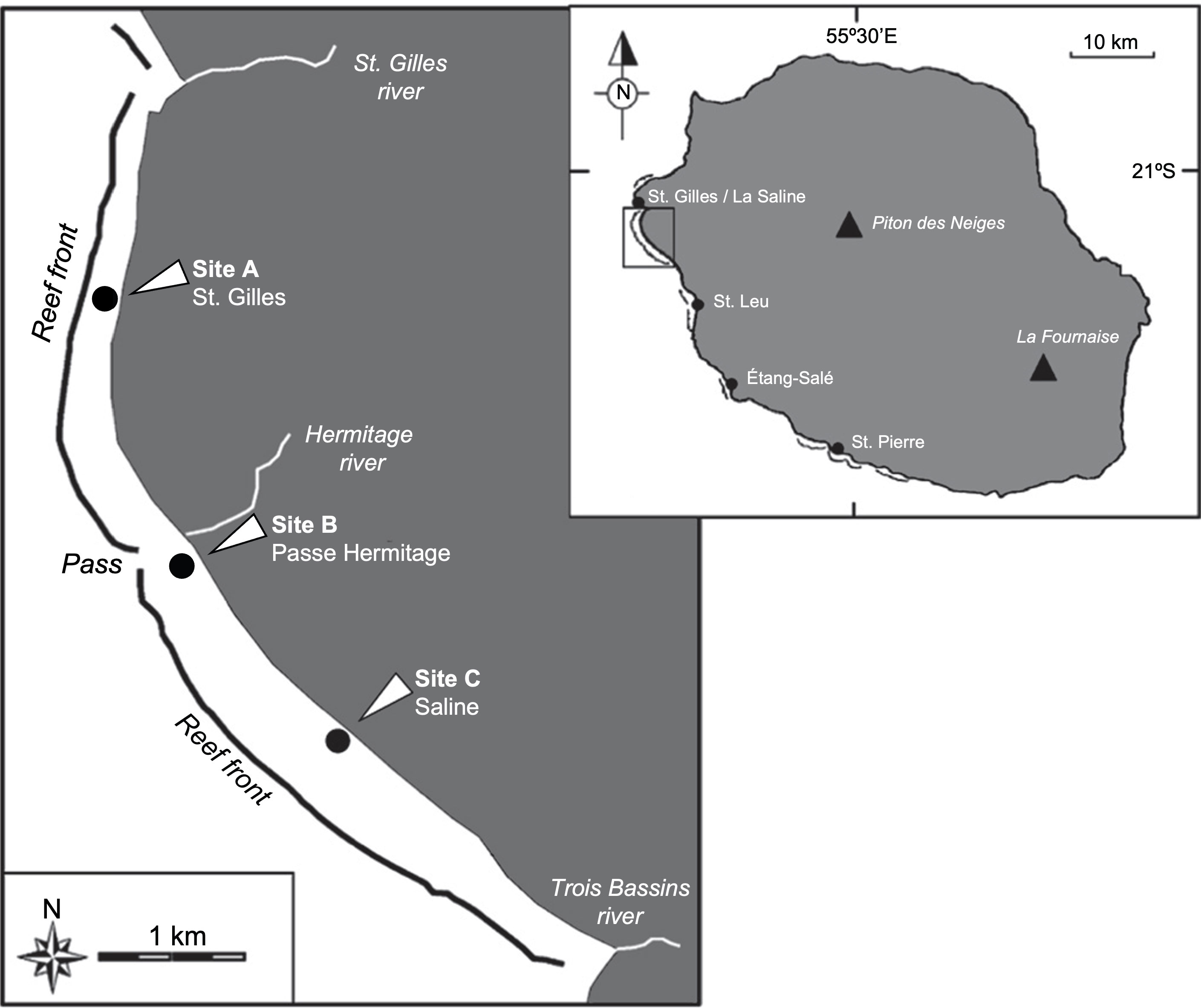
Figure 1 Sampling locations at Reunion Island. “Saint-Gilles” (STG, site A), “Passe Ermitage” (PAS, site B) and “Saline” (SAL, site C). Modified from Clavier et al. (2008).
At each station, we measured the main environmental biogeochemical descriptors (including physico-chemical parameters, inorganic nutrients, and organic contaminants of emerging concern, CECs) and studied three different components of benthic compartment: i) seagrasses (plant and meadow level), ii) sediment, and iii) the whole benthic community (which includes the sediment, seagrasses, and its associated benthic community, such as benthic infauna, epiphytes, etc.). Field work was conducted in June 2018.
2.2 Environmental biogeochemical descriptors
Descriptors of the water mass in the lagoon around the seagrass meadows were monitored using a combination of autonomous sensors and analytical techniques. Temperature, dissolved oxygen (PreSens®), and irradiance (Odyssey Integrating PAR sensor, Dataflow Systems) were measured in situ at each sampling location. Water samples for salinity, nutrients, pH and alkalinity analysis were also taken at the sampling locations for later analysis at University of La Reunion. Salinity was measured using a multiparameter probe (YSI 6920). Ammonium analyses were performed spectrophotometrically (Kontron Uvikon 922) according to the indo-phenol blue method following Aminot and Kerouel (2004). Nitrate, nitrite, and phosphate were measured using a Seal Autoanalyzer III and standard analysis methods. Potentiometric determination of pH (Radiometer TIM865 titrator with combined pH electrode pHC2401-8) was performed at 25°C using Tris/HCl and 2-aminopyridine/HCl buffers in synthetic seawater to calibrate the electrode (Dickson et al., 2007). Alkalinity was determined by potentiometric titration using 0.01 M HCl in NaCl to approximate the ionic strength of seawater using Certified Reference Material from A. Dickson laboratory (Scripps Institution of Oceanography) as standard.
Sediment samples around the seagrass meadows were collected using Plexiglas tubes (i.d. 5 cm, length 30 cm, n = 3). Organic matter content of the first centimetre was measured as loss on ignition (burned at 550 °C for 4 hours). Chlorophyll content was measured in the top one cm, extracting the photosynthetic pigments with 100% methanol for 24 hours at 4 °C (Thompson et al., 1999) and determining the pigment content spectrophotometrically as previously described (Ritchie, 2008). Selected emerging organic contaminants (CECs), among the most widely detected in the sediment, such as polycyclic fragrances, nitro musks, triclosan (antibacterial) and methyl triclosan (its metabolite), nonylphenol isomers (surfactant), DEET (insect repellent), UV filters and organophosphorus flame retardants (OPFRs) were analysed by in-cell clean-up pressurised liquid extraction (PLE) followed by gas chromatography-triple quadrupole mass spectrometry (GC-MS/MS; SCION 456-GC and SCION TQ, Bruker). Limits of detection (lod) of the method, calculated using a signal to noise ratio of 3:1, ranged between 0.003 and 2.4 ng g-1. Further details on the extraction and detection methodology can be found in Pintado-Herrera et al. (2016).
2.3 Seagrass metabolism
The net productivity and respiration of S. isoetifolium individuals were evaluated measuring oxygen evolution during in situ incubations (Olivé et al., 2017) with PreSens® optodes. At each location (site A, B and C), plants (circa 10-15 cm rhizome bearing 5-7 shoots) were randomly collected, gently cleaned, and incubated in gas-tight zip-bags (PPC+PET) with its own surrounding water. At each site, two rounds of incubations were performed around solar noon (noon ± 3 hour). On each round, incubations for net plant productivity (NPP) (transparent chambers, n = 5) and respiration (R) (darkened chambers, n = 5) were performed. Seagrass structural descriptors (biomass and morphometry) were also recorded after the incubations for rates normalisation. Productivity and respiration of seagrass meadow at each site was calculated considering the biomass of seagrass per area at each of the studied sites. Daily productivity, respiration, and metabolic balance budgets of S. isoetifolium plant and meadow were calculated assuming a 12 h daylight photoperiod as it was in Reunion Island during the sampling period. The metabolic balance was calculated as the output between daily productivity and respiration. Since incubations ran at noon (i.e. maximum irradiance), seagrass productivity measurements were most likely maximised (Olivé et al., 2016), so the daily budgets calculated, for both plant and meadow, can be considered a proxy of maximum productivity and metabolic balance.
2.4 Sediment metabolism
2.4.1 Sampling procedure
Three small cores (10 cm length, 4 cm i.d., n = 3 per site) enclosing sediment were randomly collected within the seagrass meadows at each sampling site (A, B and C) for microsensor measurements. Cores and in situ water (≈ 20 L) were transported immediately to the laboratory (within 30 minutes after collection) and maintained at 26 °C, similar to in situ water temperature.
2.4.2 Sediment microprofiles
Diffusive fluxes (O2) of the water-sediment were evaluated using oxygen microelectrodes in the laboratory on collected cores. Cores were maintained in a temperature-controlled aquarium at 26 °C with bubbling to ensure adequate oxygen saturation and water turbulence. Cores were exposed to light conditions (white LED, ≈ 50 μmol quanta m-2 s-1) for one hour before starting the measurements and, subsequently, to dark conditions for another hour. A minimum of 3 microprofiles were measured in each core in both light and dark conditions using Clark type oxygen microelectrodes (Unisense, Denmark). The microsensor tip was 50 µm in diameter and profiles were calibrated using O2 concentrations in the vigorously bubbled overlying water as 100% O2 saturation and the signal at anoxic depths as zero. Microsensors were connected to a picoammeter (Multimeter, Unisense, Denmark) and driven into the sediment using a motorised micromanipulator (MM33, Unisense, Denmark) in vertical steps of 100 µm. O2 fluxes across the sediment-water interface were calculated by Fick’s first law of diffusion, using the vertical gradient in the diffusive boundary layer following the equation:
Where, D0 is the diffusion coefficient of oxygen in water at the temperature and salinities of the sample and ∂[O2]/∂z is the oxygen concentration gradient measured in the diffusive boundary layer. Daily metabolic fluxes and budgets were calculated assuming a 12 h daylight photoperiod.
2.5 Benthic community metabolism and water-sediment fluxes
2.5.1 Sampling procedure
Six sediment cores (30 cm length, 5.6 cm i.d., n = 6 per site) enclosing the whole community, i.e. sediment + seagrass + the associated benthic community (e.g. benthic infauna, epiphytes, etc), were randomly collected within the seagrass meadows at each sampling site (A, B, C) for community incubations. Transportation and laboratory setting up was conducted as for sediment cores (section 2.4.1).
2.5.2 Community incubations
Total oxygen and nutrient fluxes between the benthic community and the overlying water were measured by sediment core incubations. Three cores were maintained at dark conditions, whereas the other three were maintained illuminated (white LED, ≈50 μmol quanta m-2 s-1). To ensure turbulence in the water column during the incubation, magnetic stirrers were mounted inside each core and driven by an external rotating magnet. Thirty minutes before the start of the incubations, , was added to each one of the cores to reach a final concentration of 5 µM (initial , concentration in the cores ranged 0.4-0.8 µM) to trace processes of the N cycle (i.e. denitrification and anammox). Stirring was kept on and cores left without the stopper to avoid changes in the oxygen concentration of the water. Initial water samples for nutrients (i.e. nitrate, nitrite and ammonium) were collected and initial oxygen concentration was measured using one oxygen microsensor (Unisense, Denmark). The sediment cores were then sealed by closing the tubes with rubber stoppers and kept closed for 3-3.8 hours of incubation. Water samples were withdrawn from the cores using a syringe before closing the cores with the stoppers and at the end of the incubation.
The change in concentration of gases and nutrients in the water column were used to calculate the benthic community-water fluxes as a mass balance following the equation:
Where: Cinitial and Cfinal were the concentrations measured before closing with the stopper and immediately after the incubation, respectively; t was the incubation time; V was the water volume and A was the area of the sediment. Samples for nutrients were filtered through a 0.2 µm nylon filter into a polyethylene vial and stored at -18 °C until later analysis. Nutrients concentration was analysed at Cadiz University as described in García-Robledo et al. (2014). Samples for the determination of 15N-N2 were collected and preserved with ZnCl2 for later analysis. The excess concentration of 29N2 and 30N2 was measured in a CF-IRMS at the Centre for Geomicrobiology, Aarhus University (Denmark). Rate calculations were done according to Thamdrup and Dalsgaard (2002). Daily fluxes and budgets were calculated assuming a 12 h daylight photoperiod.
2.6 Statistical Analysis
One-way ANOVAs were performed to determine differences among the three sites studied in the environmental parameters as well as in oxygen and nutrient fluxes measured on each component (i.e. seagrasses, sediment, benthic community) in both light and darkness. The significance level was set at α = 0.05. Data were transformed (square-root) when necessary to meet parametric conditions. If -parametric conditions were not meet after transformation, a non-parametric Kruskal-Wallis test was applied. To link the biogeochemical descriptors and the oxygen fluxes measured on each component of the lagoon, a Spearman’s Rank correlation analysis was conducted considering the normalized mean values at each station (i.e. n=3 ranks, station A, B and C) using the IBM SPSS software. Lineal regression model was run between nutrient fluxes and initial nutrients concentration. Unless otherwise indicated, statistical analysis was performed in R (version 4.1.1, R Core Team 2021) using RStudio (version 2021.09.0). Values along the text are mean ± standard deviation (SD) to give indication about the variability of the data. Bars in figures represent mean ± standard error of the mean (SEM) to give indication of the uncertainty in the estimate of the mean (Cumming et al., 2007). Standard deviation of daily budgets was calculated considering error propagation of the mean (Laffers, 2010).
3 Results
3.1 General biogeochemical description
Biogeochemical descriptors of both water and sediment measured at each site are shown in Table 1. Nutrient concentrations (, , , ,) were low (< 0.5 µM), with the pristine location (St A) showing the lowest N concentrations (Table 1, p < 0.001). The organic matter content in the sediment was less than 5% and chlorophyll concentration remained lower than 10 µg g-1, with the highest values measured at site C (Table 1).
The presence of organic contaminants of emerging concern (CECs) in the sediment was generally low across the lagoon (lower than 3.72 ng g-1 DW). The main organic contaminants found in the sediment were sunscreen UV filters, organophosphate flame retardants (OPFRs) and nonylphenols (NPs), which were detected at all three sites studied (Table 1). The higher concentrations of CECs were detected at site C (i.e. 3.7 ng g-1 DW of UV filter octocrylene, OC, and 0.9 ng g-1 DW of OPFR tri-n-butyl phosphate, TnBP, Table 1). (See Table S1 in Supplemental Material for a complete list of CECs analysed but not detected).
3.2 Seagrass metabolism
The plant metabolic balance, understood as the balance between productivity and respiration, was autotrophic in all sites studied and averaged plant net productivity of S. isoetifolium significantly exceeded respiration by more than 6-fold (85 ± 9 and 14 ± 3 µmol O2 g DW-1 h-1, respectively, Figure 2; Table S2). However, net productivity and respiration trends differed among locations (Figure 2, p < 0.001 and p < 0.01, respectively, Table S2). Plants growing in the pristine location of St. Gilles (STG, Site A) showed the highest productivity, while those growing at the impacted site Passe Hermitage (PAS, Site B) showed the highest respiration (106 ± 14 and 19 ± 7 µmol O2 g DW-1 h-1, respectively). The daily plant metabolic balance (PMB) was autotrophic in all sites studied but the PMB in the pristine location (Site A) was double of the impacted site (Site B) (Figure 2).
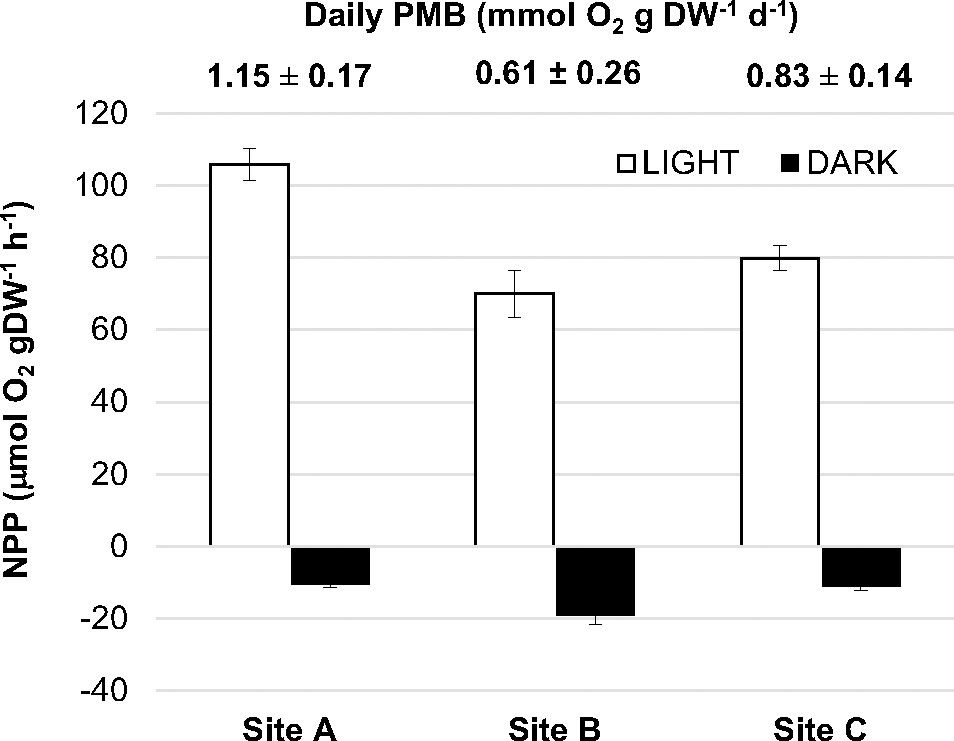
Figure 2 Net plant oxygen production of S. isoetifolium measured in light (net plant productivity, NPP, white columns) and in darkness (respiration, R, black columns) at “Saint-Gilles” (STG, site A), “Passe Ermitage” (PAS, site B) and “Saline” (SAL, site C). Bars are mean ± SEM. Numbers above indicate the daily plant metabolic balance (PMB) for each site (mean ± SD).
The plant and meadow structure also differed among the 3 locations sampled. Plant morphotype (leaf length and above/below biomass ratio) and shoot density were lower at Passe Hermitage (site B) (authors’ personal observation). Accordingly, seagrass biomass per area was significantly lower at Site B while similar biomass was recorded at sites A and C (276 ± 122; 53 ± 14; 320 ± 133 g DW m-2, for St A, B and C, respectively, p < 0.001). These differences resulted in significantly lower meadow net productivity and respiration at site B under light and dark conditions, respectively (Figure 3, p < 0.001, Table S2). Overall, the daily meadow metabolic balance (MMB) in all sites was autotrophic. However, the daily metabolic balance inferred for the meadow at Site B was around the 10% of that estimated at sites A and C (Figure 3). The mean daily metabolic balance estimated for S. isoetifolium meadows in the Reunion lagoon was 204.14 ± 21.97 mmol O2 m-2 d-1.
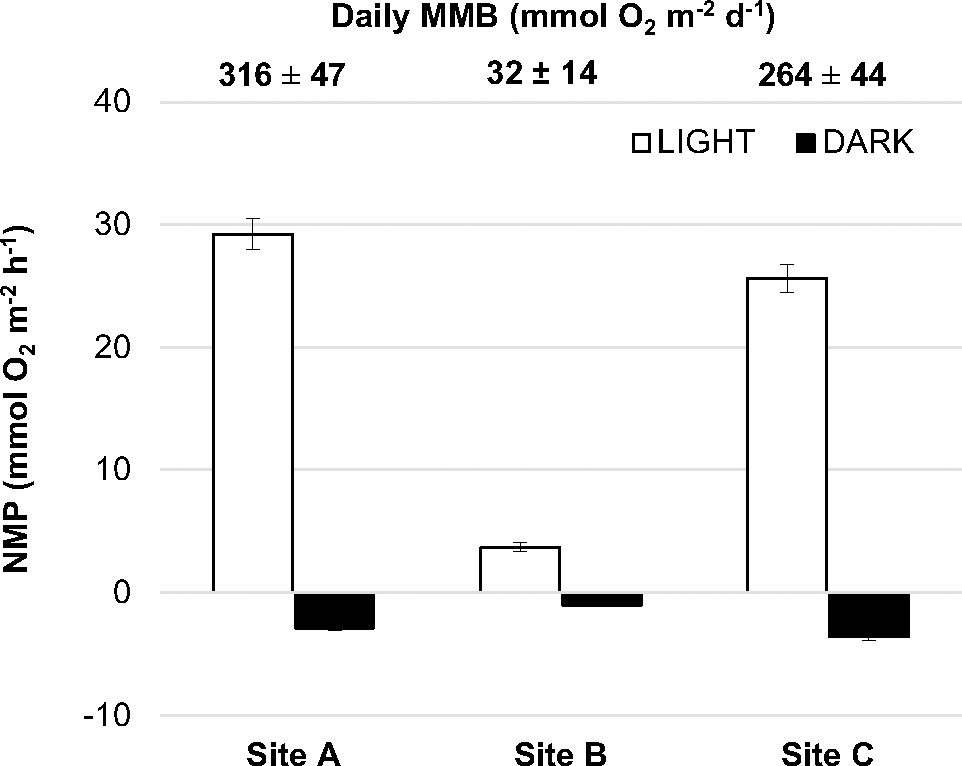
Figure 3 Seagrass net meadow productivity measured in light (white columns) and dark (black columns) conditions. Bars are mean ± SEM. Numbers above indicate the daily seagrass meadow metabolic balance (MMB) (mean ± SD) for each site.
3.3 Sediment metabolism
Oxygen microprofiles showed that oxygen was quickly consumed in the first 2-3 mm of the sediment at the three sampling sites. In the site A (STG), net production profiles showed a clear production peak below the sediment-water interface, extending the oxygen penetration depth to 3-4 mm. Site B (PAS) showed the lowest oxygen penetration depth, being as low as 1 mm in some dark profiles (Figure S1).
Despite occasional profiles showing net production under light conditions, sediment oxygen fluxes were negative (i.e. oxygen consumption) (Figure 4). Among locations studied, the pristine site (St A) showed the lowest oxygen consumption in the sediment in both light and dark conditions (Figure 4, significant in darkness p < 0.05, Table S2). The daily metabolic oxygen balance in the sediment component (SMB, only sediment) was net heterotrophic in all sites (Figure 4). The average daily metabolic balance in the lagoon for the sediment component was -16.31 ± 4.20 mmol O2 m-2 d-1.
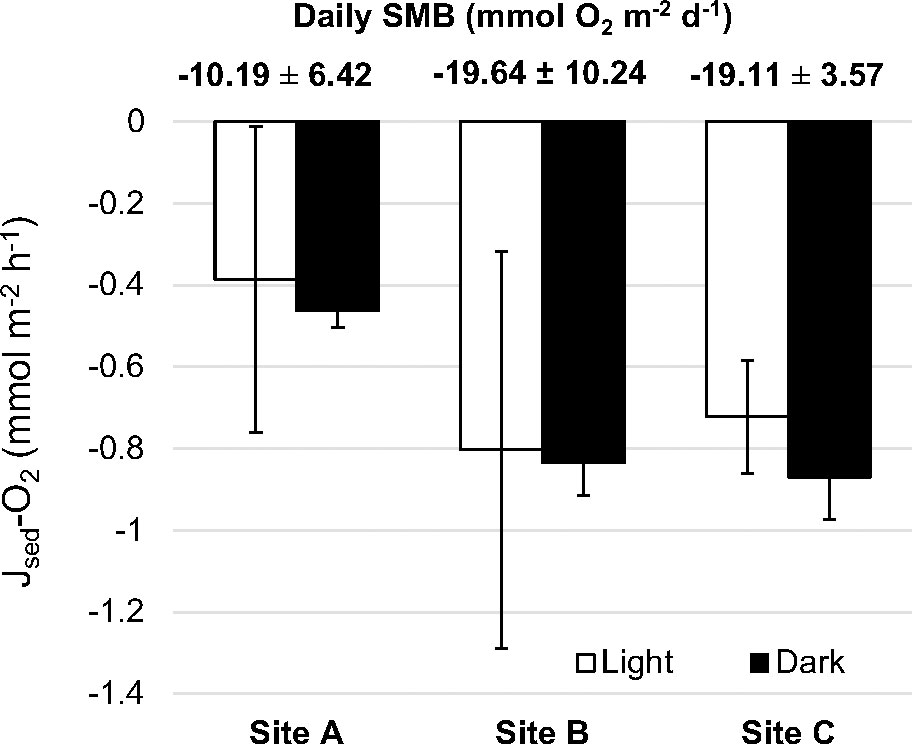
Figure 4 Sediment metabolic fluxes of oxygen measured in light (white columns) and dark (black columns) conditions. Bars are mean ± SEM. Numbers above indicate the daily sediment metabolic balance (SMB) (mean ± SD) for each site.
3.4 Benthic community metabolism and nutrient fluxes
The benthic community metabolism (i.e. sediment, seagrasses, and the associated benthic community) was net autotrophic in light conditions, releasing oxygen at a rate of 1.4 to 1.9 mmol m-2 h-1, being similar in the three sites studied (Figure 5; Table S2). In darkness, oxygen was consumed at rates of 1.2 to 4 mmol m-2 h-1, with the highest consumption rates measured at site C. The averaged daily metabolic balance in the lagoon for the benthic community (CMB) was -8.63 ± 24.86 mmol O2 m-2 d-1, that is, a net consumption of O2 although the high variability associated to this value covers the range from autotrophic to heterotopic net balance.
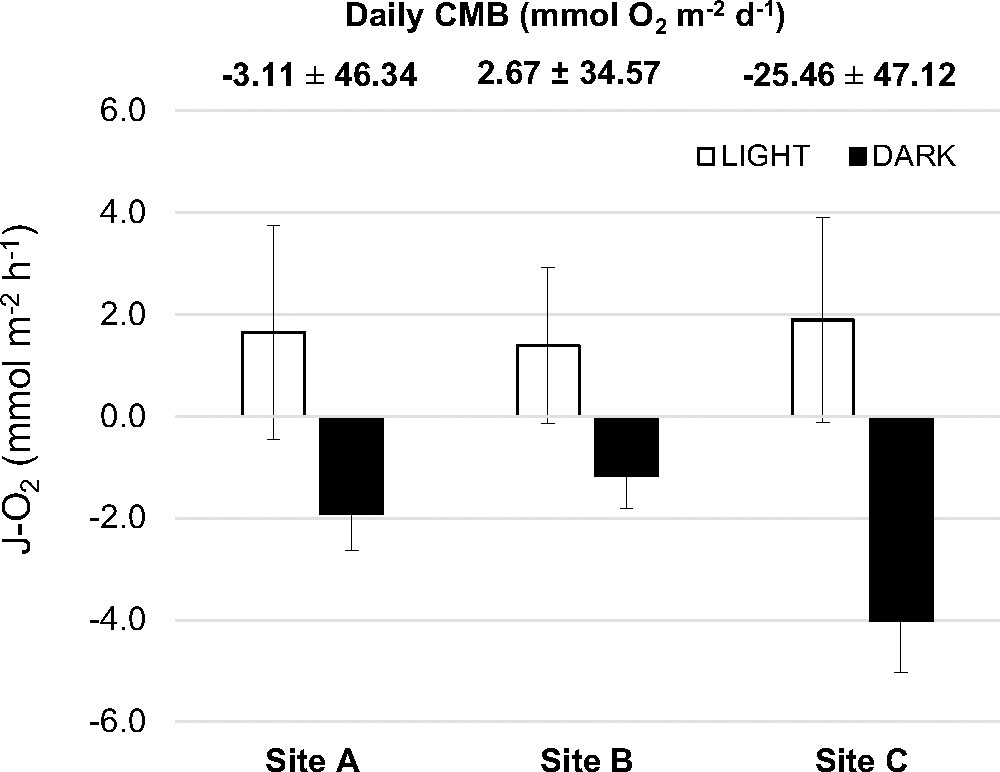
Figure 5 Community metabolic fluxes of oxygen measured in light (white columns) and dark (black columns) conditions. Positive rates mean net release from the community to the water column whereas negative values represent net consumption rates from the benthic community. Bars are mean ± SEM. Numbers above indicate the daily benthic community metabolic balance (CMB) (mean ± SD) for each site.
In general, the benthic community consumed both ammonium and nitrate under light and dark conditions, although the large heterogeneity in the rates measured resulted in large deviations from the mean value and therefore no significant differences were found between sites (Figures 6A, B Table S2). Nitrate consumption by denitrification and anammox was low (< 0.1 µmol m-2 h-1) and a minor fraction of the total nitrate consumption (0.1-1.1%) but it was measured at significant rates in the three sites (p < 0.05, t-test). No differences were detected among stations in nitrate consumption but for denitrification in darkness (Table S2). Daily denitrification rates ranged from 0.02 to 1.4 µmol m-2 d-1, with higher rates measured at station A while anammox rates varied between 0.5 and 2.2 µmol m-2 d-1 with no clear trend found among sites (Figure 6C).
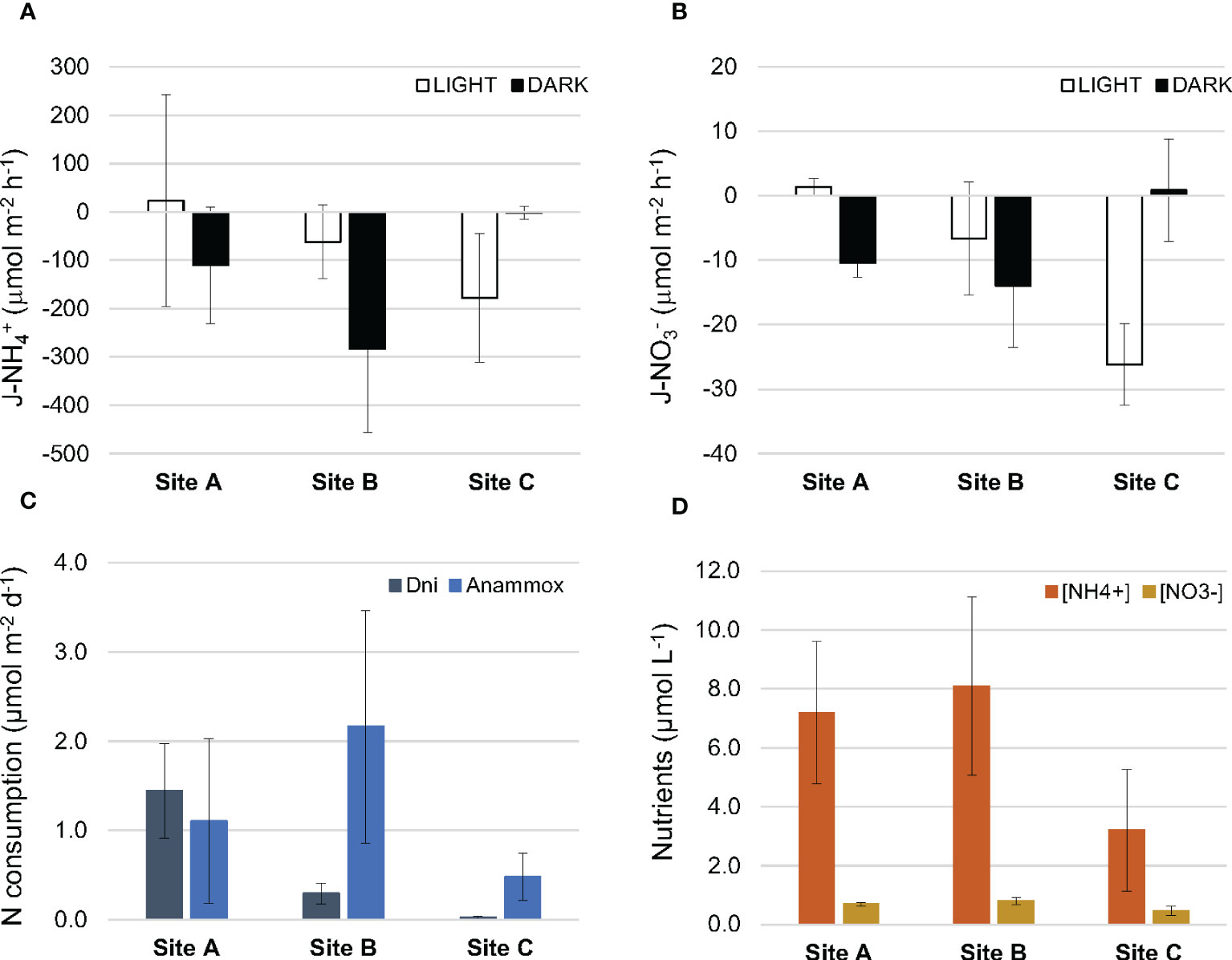
Figure 6 Net community fluxes of ammonium (A), and nitrate (B). Daily community nitrogen consumption rates (denitrification (Dni) and anammox) (C). Nutrient concentrations (ammonium and nitrate) recorded in the water at the beginning of the incubation (D). Bars are mean ± SEM.
Initial nutrient concentrations measured in the core incubations were higher than those measured in samples collected in situ, especially for ammonium (Figure 6D, Table 1, respectively). This likely resulted in net consumption fluxes higher than those expected at in situ concentrations. Similarly, the measured ammonium fluxes are much higher than nitrate fluxes despite the similar trace levels found in situ for both nutrients.
4 Discussion
This study provides the first metabolic dataset for the seagrass Syringodium isoetifolium in the West Indian Ocean. The seagrass community in the Reunion lagoon was net autotrophic and contributed to the lagoon benthic metabolism. Large variability among sites was detected in the metabolic balance of each one of the benthic components assessed (i.e. seagrass, sediment, community) also associated with the natural and/or anthropic-driven environmental variability found in the lagoon. The metabolic balance of the benthic compartment in Reunion was slightly heterotrophic but highly variable. The high productivity and autotrophic metabolic balance of S. isoetifolium helped decreasing the sediment net heterotrophy. No significant nutrient inputs were detected in this study and the benthic community showed potential capacity for maintaining the lagoon oligotrophic condition via nitrogen retention (uptake by microphytobenthos and seagrasses) and removal (denitrification and anammox) but other sources of contamination, such as organic CECs, may potentially affect the benthic community and metabolism in the lagoon and should be considered in the future studies.
4.1 Biogeochemistry of Reunion lagoon
Biogeochemical descriptors measured in the water column were in the range of those previously reported in the Reunion lagoon (Tedetti et al., 2020). Nutrients measured in the water mass, as well as organic matter and chlorophyll in the sediment, were low and within the range expected for a tropical oligotrophic sandy lagoon (van Tussenbroek, 2011; Bayraktarov and Wild, 2014). Environmental pressures in the lagoon have been historically attributed to nutrients loads from watershed associated to increasing urbanization and agriculture (Cuet, 1989). However, the low nutrient concentration measured in this study does not support the hypothesis that Reunion lagoon is experiencing significant nutrients load or facing eutrophication risk, even at the “impacted” site of Passe Hermitage.
Together with inorganic nutrients, submarine groundwater discharges and rivers may also carry organic matter and contaminants to the lagoon (Tedetti et al., 2020). Organic contaminants are a growing threat in marine systems and their toxicity has been largely demonstrated (Soares et al., 2008; Miller et al., 2021). In this study, we analysed the presence of organic contaminants of emerging concern (CECS) as potential sources of anthropogenic pressure, providing the first report on CECs in the sediment of Reunion lagoon. In general, the number and concentration of organic contaminants detected in the lagoon were low in comparison with other coral reef ecosystems and tropical areas (Kawahata et al., 2004; Mitchelmore et al., 2019). Even though, CECs were still detectable in the sediment, which is particularly relevant considering the sandy matrix of Reunion lagoon and the low affinity of organic contaminants for sandy substrates (Pignatello, 2012). The main groups of CECs found in the sediment were the UV filters, widely used as sunscreen, organophosphorus flame retardants (OPFRs), widely used as plasticizers, and detergent-like nonionic surfactant nonylphenols (NPs). UV filters (sunscreen) were the most detected CECs in the lagoon at concentrations similar than those reported in several coastal areas (Tsui et al., 2015; Apel et al., 2018) while NPs and OPFRs concentrations were lower compared to other coral reef and coastal areas (Kawahata et al., 2004; Chen et al., 2019). River flow, runoff, and groundwater discharges carrying industrial and urban wastewater are considered main entries of CECs into coral reef systems (Kroon et al., 2020). However, the higher concentrations of UV filters detected at Saline (Site C), a popular frequented beach area, are likely due to sunscreen being directly released into the environment during recreational activities. This site also showed the higher percentage of organic matter, which may further favours CECs accumulation in the sediment due to the known interaction and affinity of organic pollutants with sediment organic matter (Pignatello, 2012). Benthic organisms may further accumulate CECs, as it has been recently reported for UV filters in belowground tissues of seagrasses (Agawin et al., 2022). Once incorporated, CECs may disrupt metabolic pathways in benthic organisms altering the metabolic balances at different trophic levels, with possible wider consequences in the marine food web and biogeochemical fluxes (Kawahata et al., 2004; Tsui et al., 2015; Miller et al., 2021). A significant negative correlation (Spearman’s rank correlation, Table S3) was found between CECs (e.g. UV filters OC, 4-MBC, EHMC and BP-3, and OPFRs TnBP, TiBP, and TEHP) and the respiration rates in the 3 compartments studied (i.e. seagrass meadow, community and, to a less extend, sediment) as well as with the community metabolic balance (CMB). Although not conclusive, the reported negative correlations may indicate a potential negative effect of CECs on the mitochondrial functioning and metabolic routes as a result of oxidative damage, as it has been described in other species (Yeh et al., 2017; Leitão et al., 2021), which may impact in the respiration rates of seagrasses and the lagoon system. Although further work is needed, the presence of CECs in Reunion lagoon may constitute an environmental driver of pressure also contributing to the high metabolic variability detected in the benthic compartment (see discussion below). Our results highlight the need of considering new emerging contaminants together with classical biogeochemical descriptors to properly describe the environmental context and detect potential drivers of pressure for the benthic community in areas of high ecological value, such as tropical coral reefs.
4.2 Metabolic balance in the lagoon compartments
4.2.1 Seagrass metabolism
Plants of S. isoetifolium in Reunion showed a positive metabolic balance, understood as the balance between photosynthesis and respiration, confirming the autotrophic contribution of seagrasses to coral reef systems. The positive metabolic output resulted from plant photosynthesis largely exceeding respiration in all the sites assessed. The average daily metabolic balance calculated for seagrass meadows in Reunion (204.14 ± 21.97 mmol O2 m-2 d-1) falls within the range attributed to seagrass communities (Duarte et al., 2010) and within other tropical small-species (Anton et al., 2020). We also note our estimate should be considered as optimized since seagrass metabolism was assessed at noon when seagrass productivity is likely to be maximised (Olivé et al., 2016).
The net productivity, respiration, and metabolic balance of S. isoetifolium plants displayed large variability (up to two-fold) among the sites studied. Large intraspecific plasticity has been attributed to the metabolism of seagrasses, as well as other physiological and morphological traits, associated with environmental drivers (Olivé et al., 2013; Enríquez et al., 2019; Peralta et al., 2021). Differences in the metabolic balance of plants found at the different sites likely reflected the exposure to different environmental conditions. We hypothesised that plants in site B were potentially exposed to higher environmental pressure, such as nutrients or contamination from sewage loads. The lower metabolic balance of plants was recorded at the impacted site B (PASS) and was mainly due to a higher oxygen consumption (i.e. respiration) in darkness, though the net productivity recorded at this site was also the lowest. Increased respiration associated with nutrient enrichment has been already documented as a metabolic response of seagrasses (Burkholder et al., 2007). However, nutrients, organic matter, and organic contaminants levels remained low at the three sites studied. Hydrodynamics may also affect morphological and physiological traits in seagrasses, for instance favouring the development of the anchoring system, decreasing the above/belowground biomass ratio, and the proportion of autotrophic tissues (Peralta et al., 2006). Plants at site B (PAS) were indeed exposed to a higher hydrodynamic regime, due to the proximity to the reef pass (Tedetti et al., 2020), likely contributing to the lower metabolism recorded at this site.
After integrating S. isoetifolium metabolism per area projected, seagrass meadows at Reunion also resulted in net autotrophic systems but the daily productivity greatly varied, up to 10-fold, among locations. Together with metabolic traits, morphological descriptors of S. isoetifolium also differed among locations. Previous monitoring of seagrass meadows conducted in Reunion revealed large variations in seagrass seascape coverage along the recent decades in the reef lagoon (Cuvillier et al., 2017). In agreement with seascape monitoring, we detected more than 5-fold variation in the seagrass biomass per area among the sites studied with significant lower biomass at site B (PAS). Both natural, physical (e.g. hydrodynamics) and biotic disturbances (e.g. grazing), as well as anthropogenic pressures (e.g. grubbing, nutrient inputs) have been pointed as main drivers conditioning seagrass meadows stability in Reunion (Cuvillier et al., 2017). Reduced growth rates and shoot density has been further described as a response of seagrass to environmental forcing, such as eutrophication (Olivé et al., 2009) and hydrodynamic perturbation events (Peralta et al., 2006). Higher energetic demands at plant level together with environmental forcing likely affected growth rates and biomass partitioning of S. isoetifolium resulting in significantly lower biomass cover at impacted location (PAS). This, in turn, scaled-up into significantly lower net metabolism and lower autotrophic contribution of seagrass meadows in the impacted site.
4.2.2 Sediment metabolism
The average daily metabolic balance calculated for the sediment component (-16.31 ± 4.20 mmol O2 m-2 d-1) was in the range reported in in situ sediment incubations conducted in the same locations (Taddei et al., 2007), reflecting a net oxygen consumption by the sediment likely being a carbon dioxide source.
The sediment oxygen fluxes measured in the sediment-water interface with microsensors were negative, i.e. net heterotrophic, even under light conditions at the irradiance levels set in the laboratory. The presence of benthic microalgae, microphytobenthos, was confirmed by the detection of chlorophyll a and c in the sediments of the three locations. Chlorophyll a concentration in the first centimetre of the sediment ranged from 6 to 9 µg g-1, equivalent to 43 to 59 mg m-2. These values were in the range of concentrations measured in other coral reef sediments where sediments are net autotrophic and microphytobenthos has been shown to be a relevant primary producer in the benthic compartment (Heil et al., 2004; Hochard et al., 2012; Cox et al., 2020). Despite the similar biomass of microphytobenthos (based on the chlorophyll concentrations) suggesting certain relevance of the microphytobenthos biomass in the benthic compartment, our measurements showed that the sediment was net heterotrophic. The relatively low light intensity reached in the laboratory, compared to the in situ light levels recorded in the field in this study and reported in other studies (Taddei et al., 2007), may have limited photosynthesis of microphytobenthos during the microsensor measurements. Some oxygen microprofiles showed net oxygen production, reflecting the potential for microphytobenthonic productivity. The larger variability in the sediment fluxes reported under light conditions was caused by the occasional presence of net productive spots with high gross productivity, even at the low light intensity set in the lab conditions. In situ oxygen microprofiles could provide further relevant information about the contribution of benthic microalgae to the metabolism of the coral reef sediment (Glud et al., 2000). However, such measurements are technically complex and were not carried out during the present study. Future studies should therefore consider the in situ use of microsensors, to fully characterise the metabolic role of microphytobenthos in the coral reef system of Reunion island.
4.2.3 Community metabolism
Oxygen fluxes between the benthic community and the water column measured with core incubations integrated the metabolism of the whole benthic community, including the sediment, seagrasses, and the associated benthic community.
The large variability of the values measured in diffusive (sediment) and community fluxes at each site did not allow the detection of significant changes in the metabolic balance among the three sites studied, however noticeable differences could be observed between the contribution of the sediment alone and the whole community to the net oxygen consumption. As it could be expected, oxygen fluxes measured in dark conditions were 2-4 times higher than the values obtained for the sediment with microsensors, reflecting the increase in oxygen consumption by the other community components (seagrasses and associated benthic community). Despite including the seagrasses, the respiration rates of the community were in the same range of those reported for bare sediment measured using in situ benthic incubation chambers close to our study sites (Taddei et al., 2007; Taddei et al., 2008). The respiration of the sediment was about 21-25% of the community respiration in sites A and C, increasing up to 70% in site B. The high contribution of the community in the respiratory rates measured in sites A and C revealed that the benthic community has a relevant role in the net oxygen consumption in the sandy areas of the reef lagoon. Previous studies in the lagoon had however considered only the bare sediment (Taddei et al., 2007; Taddei et al., 2008), excluding the contribution of the community associated with the seagrass meadows. On the other hand, the lowest contribution of the benthic community to the oxygen consumption rates in darkness was detected in Passe Hermitage (St B), which also held the lowest seagrass coverage and biomass.
The major contribution of the seagrasses to the benthic community metabolism was detected during light conditions. Unlike the sediment component, the benthic community was net autotrophic at the three stations, shifting the role of the lagoon to a net oxygen producer. Similarly to the microphytobenthonic productivity measured in the sediment, the irradiance during the laboratory incubations was low compared to in situ values, likely limiting the net oxygen production by the seagrass community. In situ community incubations similar to those performed in the bare sediments by Taddei et al. (2007) could provide useful information about the metabolic activity of the seagrass meadows, further supporting the relevance of the benthic compartment in the net production of the reef lagoon as our results suggest.
4.2.4 Lagoon-wide metabolic balances
Lagoon-wide, the mean daily benthic community metabolic balance estimated for the lagoon was slightly heterotrophic (-8.63 ± 24.86 mmol O2 m-2 d-1). However, the high uncertainty associated to this value does not allow to assertively characterize the system as net heterotrophic but close to zero oscillating between autotrophy and heterotrophy. Mean O2 fluxes varied circa 10-fold among sites studied with large variability also associated within each site. The large metabolic variability found on the species S. isoetifolium across reduced spatial scales (hundreds of metres) has been also described in other seagrass species (Olivé et al., 2013). Moreover, the sediment and the community metabolism also varied significantly across the lagoon highlighting the need of considering spatial variability when calculating global metabolic budgets. As Clavier et al. (2008) noted for temporal scales, metabolic budgets measured in limited areas and then extrapolated to larger areas without considering the associated variability may entail large inaccuracies when used in ecosystem models.
Despite this variability, the global metabolic balance of the benthic community was less heterotrophic than the sediment compartment (-8.63 ± 24.86 and -16.31 ± 4.20 mmol O2 m-2 d-1, respectively). Although seagrass beds coverage in Reunion Island is relatively low and limited to one single species, this study reveals the major role that seagrasses may hold in contributing to the autotrophic metabolic balance of reef lagoon. In order to get a closer estimate of the contribution of seagrass meadows to the community metabolism, we performed a scaling-up exercise. The productivity of seagrass meadow (NMP), measured under natural light conditions, was recalculated for the irradiance used in the laboratory for the sediment and community incubations (i.e. laboratory LED lights) using the PI curves parameters for S. filiforme provided by Major and Dunton (2000). At the laboratory irradiance, the NMP of seagrass meadows was reduced by circa 85% (i.e. 4.38 ± 0.59, 0.56 ± 0.16, 3.84 ± 0.52 mmol O2 m-2 h-1, for St. A, B and C, respectively) falling within the same order of magnitude of metabolic rates measured in the sediment and the benthic community (Figures 4 and 5). When adding the seagrass contribution to the oxygen fluxes measured in the sediment (i.e. sediment + seagrass), the NPP values obtained (3.99, -0.25, 3.11 mmol O2 m-2 h-1, for St A, B, C, respectively) fit closer to the community fluxes measured in the laboratory (1.65, 1.39, 1.89 mmol O2 m-2 h-1, for St A, B and C, respectively, Figure 5). Indeed, estimated mean daylight fluxes for the lagoon calculated from sediment + seagrass data closely matched those obtained for the benthic community (2.29 and 1.65 mmol O2 m-2 h-1, respectively). These results highlight the major role that seagrass S. isoetifolium plays in turning the benthic metabolism of the Reunion lagoon from net heterotrophy into net autotrophy and further confirm seagrasses as a key component to consider when calculating benthic fluxes and global metabolism in the benthic compartment.
4.2.5 Nutrient fluxes
Nitrogen fluxes in the community incubations were also assessed to evaluate the potential role of the benthic community as sink or source of nutrients in the lagoon. In situ nitrogen and phosphorus nutrient concentrations were below 0.5 µM at the three stations, confirming the low nutrient availability in the water column and the oligotrophic conditions of the lagoon. However, the sampling of the cores for community incubations likely induced a perturbation in the low nutrient conditions of the water column. Ammonium concentrations measured in the water column inside the cores ranged from 1 to 5 µM, being 2-10 times higher than the concentrations measured in situ (about 0.5 µM). Ammonium accumulates in the porewater (Capone et al., 1992) and the increased concentrations measured in the cores suggest that sediment resuspension during the collection or later manipulation of the sediment cores and the release of porewater ammonium may have taken place. Nitrate concentrations in the water column during the incubations were similar to those measured in situ, suggesting that nitrate did not accumulate in the porewater as has also been measured in other coral reef sediments (Capone et al., 1992) and the sediment manipulation did not cause a relevant increase in the nitrate concentration inside the cores.
Ammonium and nitrate fluxes were significantly and negatively correlated with the concentration of each nutrient at the beginning of the incubation (linear regression, p < 0.01, Table S4), likely reflecting the nutrient limitation status of the community. At low nutrient concentrations, fluxes were close to zero or even positive whereas negative values (i.e. net consumption) were detected in samples with higher initial nitrogen concentrations. The increase in ammonium concentration caused by the slight sediment disturbance resulted in increased ammonium consumption. Similarly, the increase in nitrate concentrations caused by isotope addition proportionally increased nitrate consumption rates. In both cases, the increase in nutrient concentration resulted in a proportional increase in nutrient flux to the benthic compartment. These results indicate the capacity of the benthic ecosystem for nutrient uptake when nutrient concentrations increase in the water column. Nutrient fluxes in seagrass communities has been shown to be proportional to the nutrient concentrations in the water column at low concentrations, being consequence of nutrient uptake by the different components of the community (seagrasses and microphytobenthos) (Cornelisen and Thomas, 2006). Therefore, slight and sporadic increases in nutrient loading to the reef could be buffered by the nutrient retention capacity of the seagrass benthic community, which absorbs nutrients from the water column at a rate proportional to the concentration of nutrients in the water column. Nutrient discharges from groundwater systems have been described in the area (Tedetti et al., 2020) and the potential nutrient uptake from the seagrass community (Alexandre et al., 2011) might dampen the negative impacts of nutrient enrichment in oligotrophic ecosystem such as the Reunion Island lagoon.
The nitrate flux towards the benthic community also included denitrification and anammox processes. Nitrate transformations to nitrogen gas were detected in the community incubations at the three sites confirming the presence of this process in the sediments of the reef lagoon. Rates measured were low as could be expected by the low nitrate levels found in the lagoon, resulting in rates similar to those found in subtropical seagrass sediments (Salk et al., 2017). Dissimilatory reduction of nitrate to ammonium (DNRA) was not measured in this study and it might induce the underestimation of denitrification and anammox rates (Song et al., 2013; Salk et al., 2017). However, DNRA rates in intact sediment cores are commonly low and the impact on denitrification and anammox rates are negligible (Song et al., 2016). On average, only 0.4-1.2% of the nitrate flux to the sediment was converted to dinitrogen (N2) and removed from the system. While the nutrients incorporated by the photosynthetic community can return to the system through the degradation of their biomass, denitrification and anammox processes imply a loss of nitrogen from the system. These nitrogen consumption processes of the benthic compartment, although slow, remove a fraction of the nutrient load of the ecosystem, likely contributing to the maintenance of the oligotrophic conditions of the lagoon.
Data availability statement
The datasets presented in this study can be found in online repositories. The names of the repository/repositories and accession number(s) can be found below: ZENODO repository (https://doi.org/10.5281/zenodo.6822296).
Author contributions
IO, EG-R, JS, RS, NK, PC, and PF contributed to conception and design of the study; IO, EG-R, JS, PC, and PF conducted field work; IO, EG-R, MP-H, and PC conducted laboratory analysis; IO and EG-R organized and analysed the database; IO wrote the first draft of the manuscript; EG-R and MP-H wrote sections of the manuscript. All authors contributed to manuscript revision, read, and approved the submitted version.
Funding
This research was supported by funding from the European Union’s Horizon 2020 research and innovation programme under grant agreements No 654182 (ENVRIplus project, TNA-MACRORE) and No 752250 (Marie Skłodowska-Curie grant scheme MSCA-IF-EF-ST, SEAMET). This study received funding from the Integrative Marine Ecology Department from Stazione Zoologica Anton Dohrn; from the 2014-2020 European Regional Development Fund (ERDF) Operational Programme; the Department of Economy, Knowledge, Business and University of the Regional Government of Andalusia (Spain) (FEDER-UCA18-107225); from the Portuguese national funds from FCT - Foundation for Science and Technology through projects UIDB/04326/2020, UIDP/04326/2020 and LA/P/0101/2020.
Acknowledgments
Authors thank the ENVRIplus project for providing TransNational Access (TNA) to OSU-Réunion stations - MACRORE project. IO was supported by a postdoctoral grant (H2020-MSCA-IF-2016-752250). EG-R is supported by the Spanish Ramon y Cajal Program (RYC2019-027675-I). The authors thank Sophie Bureau, for her valuable help and her technical support in the field.
Conflict of interest
The authors declare that the research was conducted in the absence of any commercial or financial relationships that could be construed as a potential conflict of interest.
Publisher’s note
All claims expressed in this article are solely those of the authors and do not necessarily represent those of their affiliated organizations, or those of the publisher, the editors and the reviewers. Any product that may be evaluated in this article, or claim that may be made by its manufacturer, is not guaranteed or endorsed by the publisher.
Supplementary material
The Supplementary Material for this article can be found online at: https://www.frontiersin.org/articles/10.3389/fmars.2022.867986/full#supplementary-material
References
Agawin N. S. R., Sunyer-Caldú A., Díaz-Cruz M. S., Frank-Comas A., García-Márquez M. G., Tovar-Sánchez A. (2022). Mediterranean Seagrass posidonia oceanica accumulates sunscreen UV filters. Mar. Pollut. Bull. 176, 113417. doi: 10.1016/j.marpolbul.2022.113417
Alexandre A., Silva J., Bouma T. J., Santos R. (2011). Inorganic nitrogen uptake kinetics and whole-plant nitrogen budget in the seagrass zostera noltii. J. Exp. Mar. Biol. Ecol. 401, 7–12. doi: 10.1016/j.jembe.2011.03.008
Aminot A., Kerouel R. (2004). Hydrologie des écosystèmes marins: Paramètres et analyses (Paris: IFREMER).
Anton A., Baldry K., Coker D. J., Duarte C. M. (2020). Drivers of the low metabolic rates of seagrass meadows in the red Sea. Front. Mar. Sci. 7. doi: 10.3389/fmars.2020.00069
Apel C., Tang J., Ebinghaus R. (2018). Environmental occurrence and distribution of organic UV stabilizers and UV filters in the sediment of Chinese bohai and yellow seas. Environ. Pollut. 235, 85–94. doi: 10.1016/j.envpol.2017.12.051
Barrón C., Duarte C. M., Frankignoulle M., Borges A. V. (2006). Organic carbon metabolism and carbonate dynamics in a Mediterranean seagrass (Posidonia oceanica), meadow. Estuar. Coast. 29 (3), 417–426. doi: 10.1007/BF02784990
Bayraktarov E., Wild C. (2014). Spatiotemporal variability of sedimentary organic matter supply and recycling processes in coral reefs of tayrona national natural park, Colombian Caribbean. Biogeosciences 11 (11), 2977–2990. doi: 10.5194/bg-11-2977-2014
Bell P. R. F. (1992). Eutrophication and coral reefs–some examples in the great barrier reef lagoon. Water Res. 26 (5), 553–568. doi: 10.1016/0043-1354(92)90228-V
Borges A. V., Schiettecatte L. S., Abril G., Delille B., Gazeau F. (2006). Carbon dioxide in European coastal waters. Estuar. Coast. Shelf. Sci. 70, 375–387. doi: 10.1016/j.ecss.2006.05.046
Bouma T. J., Olenin S., Reise K., Ysebaert T. (2009). Ecosystem engineering and biodiversity in coastal sediments: posing hypotheses. Helgoland. Mar. Res. 63 (1), 95–106. doi: 10.1007/s10152-009-0146-y
Burkholder J. M., Tomasko D. A., Touchette B. W. (2007). Seagrasses and eutrophication. J. Exp. Mar. Biol. Ecol. 350 (1–2), 46–72. doi: doi: 10.1016/j.jembe.2007.06.024
Capone D. G., Dunham S. E., Horrigan S. G., Duguay L. (1992). Microbial nitrogen transformations in unconsolidated coral reef sediments. Mar. Ecol. Prog. Ser. 80, 75–88. doi: 10.1016/j.jembre.2007.06
Chazottes V., Reijmer J. J. G., Cordier E. (2008). Sediment characteristics in reef areas influenced by eutrophication-related alterations of benthic communities and bioerosion processes. Mar. Geol. 250 (1), 114–127. doi: 10.1016/j.margeo.2008.01.002
Chen M., Gan Z., Qu B., Chen S., Dai Y., Bao X. (2019). Temporal and seasonal variation and ecological risk evaluation of flame retardants in seawater and sediments from bohai bay near tianjin, China during 2014 to 2017. Mar. pollut. Bull. 146, 874–883. doi: 10.1016/j.marpolbul.2019.07.049
Clavier J., Chauvaud L., Cuet P., Esbelin C., Frouin P., Taddei D., et al. (2008). Diel variation of benthic respiration in a coral reef sediment (Reunion island, Indian ocean). Estuar. Coast. Shelf. Sci. 76 (2), 369–377. doi: 10.1016/j.ecss.2007.07.028
Cornelisen C. D., Thomas F. I. M. (2006). Water flow enhances ammonium and nitrate uptake in a seagrass community. Mar. Ecol. Prog. Ser. 312, 1–13. doi: 10.3354/MEPS312001
Cox T. E., Cebrian J., Tabor M., West L., Krause J. W. (2020). Do diatoms dominate benthic production in shallow systems? a case study from a mixed seagrass bed. Limnol. Oceanog. Lett. 5 (6), 425–434. doi: 10.1002/lol2.10167
Cuet P. (1989). Influence des résurgences d'eaux douces sur les caractéristiques physico-chimiques et métaboliques de l'écosystème récifal à la réunion (Océan indien). Thèse de doctorat Chimie de l'environnement. http://www.theses.fr/1989AIX30044, Université d‚Aix-Marseille III
Cumming G., Fidler F., Vaux D. L. (2007). Error bars in experimental biology. J. Cell Biol. 177 (1), 7–11. doi: 10.1083/jcb.200611141
Cuvillier A., Villeneuve N., Cordier E., Kolasinski J., Maurel L., Farnier N., et al. (2017). Causes of seasonal and decadal variability in a tropical seagrass seascape (Reunion island, south western Indian ocean). Estuar. Coast. Shelf. Sci. 184, 90–101. doi: 10.1016/j.ecss.2016.10.046
Dayton P. (1972). Toward an understanding of community resilience and the potential effects of enrichment to the benthos at McMurdo sound, Antarctica. Proc. Colloquium. Conserv. Prob. Antarctica. 81–96.
Dickson A. G., Sabine C. L., Christian J. R. (2007). Guide to best practices for ocean CO2 measurements. Canada: PICES special publication 3, 191 pp.
Duarte C. M., Chiscano C. L. (1999). Seagrass biomass and production: a reassessment. Aquat. Bot. 65 (1), 159–174. doi: 10.1016/S0304-3770(99)00038-8
Duarte C., Dennison W., Orth R., Carruthers T. (2008). The charisma of coastal ecosystems: addressing the imbalance. Estuar. Coast. 31 (2), 233–238. doi: 10.1007/s12237-008-9038-7
Duarte C. M., Marbà N., Gacia E., Fourqurean J. W., Beggins J., Barrón C., et al. (2010). Seagrass community metabolism: Assessing the carbon sink capacity of seagrass meadows. Global Biogeochem. Cyc. 24 (4), GB4032. doi: 10.1029/2010gb003793
Enríquez S., Marbà N., Cebrián J., Duarte C. M. (2004). Annual variation in leaf photosynthesis and leaf nutrient content of four Mediterranean seagrasses. Botanica Mar. 47, 295–306. doi: 10.1515/BOT.2004.035
Enríquez S., Olivé I., Cayabyab N., Hedley J. D. (2019). Structural complexity governs seagrass acclimatization to depth with relevant consequences for meadow production, macrophyte diversity and habitat carbon storage capacity. Sci. Rep. 9 (1), 14657. doi: 10.1038/s41598-019-51248-z
Eyre B. D., Ferguson A. J. P. (2002). Comparison of carbon production and decomposition, benthic nutrient fluxes and denitrification in seagrass, phytoplankton, benthic microalgae- and macroalgae-dominated warm-temperate Australian lagoons. Mar. Ecol. Prog. Ser. 229, 43–59. doi: 10.3354/meps229043
Fourqurean J. W., Duarte C. M., Kennedy H., Marba N., Holmer M., Mateo M. A., et al. (2012). Seagrass ecosystems as a globally significant carbon stock. Nat. Geosci. 5 (7), 505–509. doi: 10.1038/ngeo1477
García-Robledo E., Corzo A., Papaspyrou S. (2014). A fast and direct spectrophotometric method for the sequential determination of nitrate and nitrite at low concentrations in small volumes. Mar. Chem. 162, 30–36. doi: 10.1016/j.marchem.2014.03.002
Gattuso J. P., Frankignoulle M., Wollast R. (1998). Carbon and carbonate metabolism in coastal aquatic ecosystems. Annu. Rev. Ecol. Systemat. 29 (1), 405–434. doi: 10.1146/annurev.ecolsys.29.1.405
Gillooly J. F., Brown J. H., West G. B., Savage V. M., Charnov E. L. (2001). Effects of size and temperature on metabolic rate. Science 293 (5538), 2248–2251. doi: 10.1126/science.1061967
Glud R. N. (2008). Oxygen dynamics of marine sediments. Mar. Biol. Res. 4 (4), 243–289. doi: 10.1080/17451000801888726
Glud R. N., Gundersen J. K., Ramsing N. B. (2000). “"Electrochemical and optical oxygen microsensors for in situ measurements. in in situ monitoring of aquatic systems: Chemical analysis and speciation,",” in In situ monitoring of aquatic systems. Ed. Horvai G. (Chichester, New York: Wiley), 20–73.
Graham O. J., Aoki L. R., Stephens T., Stokes J., Dayal S., Rappazzo B., et al. (2021). Effects of seagrass wasting disease on eelgrass growth and belowground sugar in natural meadows. Front. Mar. Sci. 8. doi: 10.3389/fmars.2021.768668
Heil C. A., Chaston K., Jones A., Bird P., Longstaff B., Costanzo S., et al. (2004). Benthic microalgae in coral reef sediments of the southern great barrier reef, Australia. Coral. Reefs. 23 (3), 336–343. doi: 10.1007/s00338-004-0390-1
Hochard S., Pinazo C., Rochelle-Newall E., Pringault O. (2012). Benthic pelagic coupling in a shallow oligotrophic ecosystem: Importance of microphytobenthos and physical forcing. Ecol. Model. 247, 307–318. doi: 10.1016/j.ecolmodel.2012.07.038
Hughes T. P., Baird A. H., Bellwood D. R., Card M., Connolly S. R., Folke C., et al. (2003). Climate change, human impacts, and the resilience of coral reefs. Science 301 (5635), 929–933. doi: 10.1126/science.1085046
Kawahata H., Ohta H., Inoue M., Suzuki A. (2004). Endocrine disrupter nonylphenol and bisphenol a contamination in Okinawa and ishigaki islands, Japan–-within coral reefs and adjacent river mouths. Chemosphere 55 (11), 1519–1527. doi: 10.1016/j.chemosphere.2004.01.032
Kroon F. J., Berry K. L. E., Brinkman D. L., Kookana R., Leusch F. D. L., Melvin S. D., et al. (2020). Sources, presence and potential effects of contaminants of emerging concern in the marine environments of the great barrier reef and Torres strait, Australia. Sci. Tot. Environ. 719, 135140. doi: 10.1016/j.scitotenv.2019.135140
Laffers R. (2010). Error propagation calculator. Available at: https://www.eoas.ubc.ca/ciurses/eosc252/ error-propagation-calculator-fj.htm.
Leitão I., Leclercq C. C., Ribeiro D. M., Renaut J., Almeida A. M., Martins L. L., et al. (2021). Stress response of lettuce (Lactuca sativa) to environmental contamination with selected pharmaceuticals: A proteomic study. J. Proteomics 245, 104291. doi: 10.1016/j.jprot.2021.104291
Macreadie P. I., Costa M. D. P., Atwood T. B., Friess D. A., Kelleway J. J., Kennedy H., et al. (2021). Blue carbon as a natural climate solution. Nat. Rev. Earth Environ. 2 (12), 826–839. doi: 10.1038/s43017-021-00224-1
Major K. M., Dunton K. H. (2000). Photosynthetic performance in syringodium filiforme: seasonal variation in light-harvesting characteristics. Aquat. Bot. 68 (3), 249–264. doi: 10.1016/S0304-3770(00)00115-7
Martínez-Crego B., Olivé I., Santos R. (2014). CO2 and nutrient-driven changes across multiple levels of organization in Zostera noltii ecosystems. Biogeosciences 11 (24), 7237–7249. doi: 10.5194/bg-11-7237-2014
Miller I. B., Pawlowski S., Kellermann M. Y., Petersen-Thiery M., Moeller M., Nietzer S., et al. (2021). Toxic effects of UV filters from sunscreens on coral reefs revisited: regulatory aspects for “reef safe” products. Environ. Sci. Europe. 33 (1), 74. doi: 10.1186/s12302-021-00515-w
Mitchelmore C. L., He K., Gonsior M., Hain E., Heyes A., Clark C., et al. (2019). Occurrence and distribution of UV-filters and other anthropogenic contaminants in coastal surface water, sediment, and coral tissue from Hawaii. Sci. Tot. Environ. 670, 398–410. doi: 10.1016/j.scitotenv.2019.03.034
Olivé I., García-Sánchez M. P., Brun F. G., Vergara J. J., Pérez-Lloréns J. L. (2009). Interactions of light and organic matter under contrasting resource simulated environments: the importance of clonal traits in the seagrass Zostera noltii. Hydrobiologia 629, 199–208. doi: 10.1007/s10750-009-9773-1
Olivé I., Silva J., Costa M. M., Santos R. (2016). Estimating seagrass community metabolism using benthic chambers: The effect of incubation time. Estuar. Coast. 39 (1), 138–144. doi: 10.1007/s12237-015-9973-z
Olivé I., Silva J., Lauritano C., Costa M. M., Ruocco M., Procaccini G., et al. (2017). Linking gene expression to productivity to unravel long- and short-term responses of seagrasses exposed to CO2 in volcanic vents. Sci. Rep. 7, 42278. doi: 10.1038/srep42278
Olivé I., Vergara J. J., Pérez-Lloréns J. L. (2013). Photosynthetic and morphological photoacclimation of the seagrass Cymodocea nodosa to season, depth and leaf position. Mar. Biol. 160, 285–297. doi: 10.1007/s00227-012-2087-2
Orth R. J., Carruthers T. J. B., Dennison W. C., Duarte C. M., Fourqurean J. W., Heck K. L., et al. (2006). A global crisis for seagrass ecosystems. Bioscience 56, 987–996. doi: 10.1641/0006-3568(2006)56[987:AGCFSE]2.0.CO;2
Peralta G., Brun F. G., Pérez-Lloréns J. L., Bouma T. J. (2006). Direct effects of current velocity on the growth, morphometry and architecture of seagrasses: a case study on Zostera noltii. Mar. Ecol. Prog. Ser. 327, 135–142. doi: 10.3354/meps327135
Peralta G., Godoy O., Egea L. G., de los Santos C. B., Jiménez-Ramos R., Lara M., et al. (2021). The morphometric acclimation to depth explains the long-term resilience of the seagrass Cymodocea nodosa in a shallow tidal lagoon. J. Environ. Manage. 299, 113452. doi: 10.1016/j.jenvman.2021.113452
Pignatello J. J. (2012). Dynamic interactions of natural organic matter and organic compounds. J. Soils. Sed. 12 (8), 1241–1256. doi: 10.1007/s11368-012-0490-4
Pintado-Herrera M. G., González-Mazo E., Lara-Martín P. A. (2016). In-cell clean-up pressurized liquid extraction and gas chromatography–tandem mass spectrometry determination of hydrophobic persistent and emerging organic pollutants in coastal sediments. J. Chromatogr. A. 1429, 107–118. doi: 10.1016/j.chroma.2015.12.040
Reid A. J., Carlson A. K., Creed I. F., Eliason E. J., Gell P. A., Johnson P. T. J., et al. (2019). Emerging threats and persistent conservation challenges for freshwater biodiversity. Biol. Rev. 94 (3), 849–873. doi: 10.1111/brv.12480
Revsbech N. P., JØrgensen B. B., Brix O. (1981). Primary production of microalgae in sediments measured by oxygen microprofile, H14CO3 - fixation, and oxygen exchange methods1. Limnol. Oceanog. 26 (4), 717–730. doi: 10.4319/lo.1981.26.4.0717
Ricart A. M., Ward M., Hill T. M., Sanford E., Kroeker K. J., Takeshita Y., et al. (2021). Coast-wide evidence of low pH amelioration by seagrass ecosystems. Global Change Biol. 27, 2580–2591. doi: 10.1111/gcb.15594
Risgaard-Petersen N. (2003). Coupled nitrification-denitrification in autotrophic and heterotrophic estuarine sediments: On the influence of benthic microalgae. Limnol. Oceanog. 48 (1), 93–105. doi: 10.4319/lo.2003.48.1.0093
Risgaard-Petersen N., Ottosen L. D. M. (2000). Nitrogen cycling in two temperate zostera marina beds: seasonal variation. Mar. Ecol. Prog. Ser. 198, 93–107. doi: 10.3354/meps198093
Ritchie R. J. (2008). Universal chlorophyll equations for estimating chlorophylls a, b, c, and d and total chlorophylls in natural assemblages of photosynthetic organisms using acetone, methanol, or ethanol solvents. Photosynthetica 46 (1), 115–126. doi: 10.1007/s11099-008-0019-7
Roberts C. M., McClean C. J., Veron J. E. N., Hawkins J. P., Allen G. R., McAllister D. E., et al. (2002). Marine biodiversity hotspots and conservation priorities for tropical reefs. Science 295 (5558), 1280–1284. doi: 10.1126/science.1067728
Roth F., Wild C., Carvalho S., Rädecker N., Voolstra C. R., Kürten B., et al. (2019). An in situ approach for measuring biogeochemical fluxes in structurally complex benthic communities. Methods Ecol. Evol. 10 (5), 712–725. doi: 10.1111/2041-210X.13151
Salk K. R., Erler D. V., Eyre B. D., Carlson-Perret N., Ostrom N. E. (2017). Unexpectedly high degree of anammox and DNRA in seagrass sediments: Description and application of a revised isotope pairing technique. Geochim. Cosmochim. Acta 211, 64–78. doi: 10.1016/j.gca.2017.05.012
Seddon N., Chausson A., Berry P., Girardin C. A. J., Smith A., Turner B. (2020). Understanding the value and limits of nature-based solutions to climate change and other global challenges. Philos. Trans. R. Soc. B.: Biol. Sci. 375 (1794), 20190120. doi: 10.1098/rstb.2019.0120
Smetacek V., Zingone A. (2013). Green and golden seaweed tides on the rise. Nature 504 (7478), 84–88. doi: 10.1038/nature12860
Soares A., Guieysse B., Jefferson B., Cartmell E., Lester J. N. (2008). Nonylphenol in the environment: A critical review on occurrence, fate, toxicity and treatment in wastewaters. Environ. Int. 34 (7), 1033–1049. doi: 10.1016/j.envint.2008.01.004
Song G. D., Liu S. M., Kuypers M. M. M., Lavik G. (2016). Application of the isotope pairing technique in sediments where anammox, denitrification, and dissimilatory nitrate reduction to ammonium coexist. Limnol. Oceanog.: Methods 14 (12), 801–815. doi: 10.1002/lom3.10127
Song G. D., Liu S. M., Marchant H., Kuypers M. M. M., Lavik G. (2013). Anammox, denitrification and dissimilatory nitrate reduction to ammonium in the East China Sea sediment. Biogeosciences 10 (11), 6851–6864. doi: 10.5194/bg-10-6851-2013
Taddei D., Bucas G., Clavier J., Cuet P., Frouin P. (2007). Carbon fluxes at the water sediment interface in reunion island fringing reef. West. Indian Ocean. J. Mar. Sci. 6 (2), 137–146.
Taddei D., Cuet P., Frouin P., Esbelin C., Clavier J. (2008). Low community photosynthetic quotient in coral reef sediments. Comptes. Rendus. Biol. 331 (9), 668–677. doi: 10.1016/j.crvi.2008.06.006
Tedetti M., Bigot L., Turquet J., Guigue C., Ferretto N., Goutx M., et al. (2020). Influence of freshwater discharges on biogeochemistry and benthic communities of a coral reef ecosystem (La réunion island, Indian ocean). Front. Mar. Sci. 7. doi: 10.3389/fmars.2020.596165
Thamdrup B., Dalsgaard T. (2002). Production of N2 through anaerobic ammonium oxidation coupled to nitrate reduction in marine sediments. Appl. Environ. Microbiol. 68 (3), 1312–1318. doi: 10.1128/AEM.68.3.1312-1318.2002
Thompson R. C., Tobin M. L., Hawkins S. J., Norton T. A. (1999). Problems in extraction and spectrophotometric determination of chlorophyll from epilithic microbial biofilms: towards a standard method. J. Mar. Biol. Assoc. Unite. Kingdom. 79 (3), 551–558. doi: 10.1017/S0025315498000678
Tsui M. M. P., Leung H. W., Kwan B. K. Y., Ng K.-Y., Yamashita N., Taniyasu S., et al. (2015). Occurrence, distribution and ecological risk assessment of multiple classes of UV filters in marine sediments in Hong Kong and Japan. J. Haz. Mat. 292, 180–187. doi: 10.1016/j.jhazmat.2015.03.025
Van Dam Bryce R., Zeller Mary A., Lopes C., Smyth Ashley R., Böttcher Michael E., Osburn Christopher L., et al. (2021). Calcification-driven CO2 emissions exceed “Blue carbon” sequestration in a carbonate seagrass meadow. Sci. Adv. 7 (51), eabj1372. doi: 10.1126/sciadv.abj1372
van Tussenbroek B. I. (2011). Dynamics of seagrasses and associated algae in coral reef lagoons. Hidrobiológica 21 (3), 293–310.
Ward M., Kindinger T. L., Hirsh H. K., Hill T. M., Jellison B. M., Lummis S., et al. (2022). Reviews and syntheses: Spatial and temporal patterns in seagrass metabolic fluxes. Biogeosciences 19 (3), 689–699. doi: 10.5194/bg-19-689-2022
Keywords: benthic community, metabolic balance, nutrient fluxes, productivity, reef lagoon, respiration, seagrass
Citation: Olivé I, García-Robledo E, Silva J, Pintado-Herrera MG, Santos R, Kamenos NA, Cuet P and Frouin P (2022) Contribution of the seagrass Syringodium isoetifolium to the metabolic functioning of a tropical reef lagoon. Front. Mar. Sci. 9:867986. doi: 10.3389/fmars.2022.867986
Received: 01 February 2022; Accepted: 01 July 2022;
Published: 28 July 2022.
Edited by:
Fanny Noisette, Université du Québec à Rimouski, CanadaReviewed by:
Ludovic Pascal, Université du Québec à Rimouskii, CanadaDominique Davoult, Sorbonne Universités, France
Copyright © 2022 Olivé, García-Robledo, Silva, Pintado-Herrera, Santos, Kamenos, Cuet and Frouin. This is an open-access article distributed under the terms of the Creative Commons Attribution License (CC BY). The use, distribution or reproduction in other forums is permitted, provided the original author(s) and the copyright owner(s) are credited and that the original publication in this journal is cited, in accordance with accepted academic practice. No use, distribution or reproduction is permitted which does not comply with these terms.
*Correspondence: Irene Olivé, aXJlbmUub2xpdmVAc3puLml0