- 1Faculty of Fisheries and Protection of Waters, South Bohemian Research Center of Aquaculture and Biodiversity of Hydrocenoses, University of South Bohemia in České Budějovice, Vodňany, Czechia
- 2Instituto de Ciencias Marinas de Andaluciía (ICMAN), Consejo Superior de Investigaciones Científicas (CSIC), Puerto Real, Spain
- 3Climate and Environment Department, Norwegian Research Centre AS (NORCE), Bergen, Norway
- 4Department of Biology and Geology, Faculty of Experimental Sciences, Campus de Excelencia Internacional del Mar (CEI·MAR), Universidad de Almería, Almería, Spain
The present study investigates the effects of different feeding regimes with rotifers (Brachionus plicatilis) and Artemia salina on the gene expression and digestive enzymes in pikeperch (Sander lucioperca) larvae at 17 days post-hatch (DPH) over a period of 13 days. Five experimental feeding protocols were performed in four replicates. At 4 DPH, the larvae (total length= 5.62 ± 0.03 mm, body weight = 0.66 ± 0.16 mg) were divided into five experimental groups (2-L tanks) at initial density of 100 larvae per liter. Light intensity on the water surface was 90-100 lux and photoperiod was set at 13L: 11D (07:00 to 20:00 h). Water temperature, pH, and dissolved oxygen (DO) were measured before each feeding and the values were 17.8 ± 0.17°C, 7.3 ± 0.04 and 88.5 ± 2.53%. The fish larvae at 5 days post-hatch (DPH), were initially fed with rotifers (Brachionus plicatilis) for 3 days and from 8 to 17 DPH were fed with rotifers/Artemia for different time periods as follows: (A) only rotifers; (B) 8–13 DPH rotifers/14–17 DPH Artemia; (C) 8–10 DPH rotifers/11–17 DPH Artemia; (D) only Artemia; (E) a combination of rotifers and Artemia. Frozen paste of algae was added to the larval tanks twice a day (2 x 300,000 cells/mL). Rotifers and Artemia were provided as live feed to larvae three times a day with residual counts prior to each feeding. Feeding densities were steadily increased based on residual counts, performed prior to each feeding. The expression of genes related to intestinal development and maturation (aminopeptidase N, anpep; leucine aminopeptidase 3, lap3; intestinal-type alkaline phosphatase, alpi), together with key pancreatic digestive proenzymes (trypsinogen 1, try1; chymotrypsinogen b, ctrb; carboxyl ester lipase precursor, cel; phospholipase a2, pla2g1b; pancreatic alpha amylase, amy2a), were assessed. Additionally, the activity of six enzymes (trypsin, lipase, alkaline phosphatase, amino peptidase, amylase, and chymotrypsin) were determined. The highest expression of two genes related to intestine (lap3; anpep) were observed in the fish fed a combination of rotifers and Artemia from 8 DPH (Group E). The expression of amy2a, ctrb, pla2g1b, try1 was significantly lower in larvae fed rotifers until 14 DPH and replaced by Artemia afterwards (Group B). The specific activity of brush border membrane enzymes (alkaline phosphatase and aminopeptidase N) increased with combination of rotifers and Artemia in larval diet (Group E), indicating a more efficient functionality of digestive structures. The groups fed only with rotifers till 17 DPH (Group A) (38 ± 4.07%) and larvae fed with rotifers till 14 DPH followed by feeding with Artemia till 17 DPH (Group B) (36 ± 5.25%) showed significantly (P<0.05) lower survival rates than the other groups (54-67%). The group fed only with rotifers (Group A) showed significantly lower specific growth rate (SGR) than the other groups, and the highest SGR was found in the group fed with combination of rotifers and Artemia after 3 day rotifer feeding (Group E). The highest standard length (8.32 ± 0.48 mm) was obtained by combined feeding of rotifers and Artemia after 3 day of initial rotifer feeding. Combination of rotifers and Artemia from 8 DPH (Group E) could be considered a more appropriate diet for first feeding pikeperch larvae compared with later introduction of Artemia, as indicated by the higher expression of genes and activities of digestive enzymes. Our findings provide new insight into the effect of temporal sequence of rotifers and Artemia on the expression of genes and activities of digestive enzymes in pikeperch larvae.
Introduction
Among the species belonging to the percid family, the pikeperch (Sander lucioperca L.) has been recognized as a potential candidate of European inland aquaculture mainly because of the delicious flesh and high growth rate (Schulz et al., 2007; Kristan et al., 2016). Although a vast research data has been reported on pikeperch culture under controlled conditions (Policar et al., 2013; Policar et al., 2016; Khendek et al., 2018; Baekelandt et al., 2019; Penka et al., 2021), successful rearing of larvae is a major problem in intensive culture (Policar et al., 2019). The main challenges of the larval culture are high mortality and quality of cultured larvae (Policar et al., 2019). The survival rates of pikeperch during the larval stage are below 20%. In order to reduce the mortality rates of pikeperch larvae reared under controlled conditions, feeding protocols must be improved. Live prey is required during two first weeks after mouth opening of pikeperch. Afterwards, pikeperch can we successfully weaned. Earlier weaning of pikeperch, beginning from 12th DPH results in higher mortality and deformity of fish (Kestemont et al., 2007). Although Artemia is a common diet in fish larviculture, the nutritional profile, size and inconsistent in biochemical composition, makes it less suitable candidate for first feeding. In this respect, recent studies showed the importance of larval first feeding with rotifers (Brachionus plicatilis) with a supplementation of microalgae to provide a better growth and higher survival (Yanes-Roca et al., 2018; Imentai et al., 2020; Yanes-Roca et al., 2020a; Yanes-Roca et al., 2020b). It was reported that higher growth and survival was reached when larvae fed with B. plicatilis at the beginning of exogenous feeding and afterwards replaced with Artemia or a combination of two during first feeding (Imentai et al., 2020). Although successful protocol for first feeding is described based on the survival and growth parameters, the molecular basis of pikeperch larval nutrition during this crucial stage is largely unknown.
Expression of genes related to the digestive function is regulated at multiple levels and is species (Yúfera et al., 2018) and age-specific (Wang et al., 2006). The synthesis of digestive enzymes can be modulated by genes, hormones and nutrition (Peres et al., 1998). It has been reported that pancreatic trypsin, chymotrypsin, elastase, carboxypeptidase A, B and lipase of Japanese flounder (Paralichthys olivaceus) are expressed at first feeding (Srivastava et al., 2002). As well for the larvae of eel (Anguilla japonica) pancreas start to synthesize all digestive enzymes by the onset of exogenous feeding (Kurokawa et al., 2002).
Digestion is a critical for the growth and survival of fish larvae (Govoni et al., 1986). During this complex process, the ingested food is hydrolyzed by secretion of specific digestive enzymes into the gastrointestinal tract (Gilannejad et al., 2021). At mouth opening, pikeperch larval stomach is not yet developed, for which extra-cellular degradation of ingested prey in the intestine is mainly done by pancreas-derived enzymes (Hamza et al., 2015). In many fish species, larvae pancreatic (trypsin, amylase) and intestinal (leucine-alanine peptidase, Alkaline phosphatase, aminopeptidase N) enzyme activities usually increase at mouth opening and might vary in response to the nature and composition of the diet (Infante and Cahu, 1994; Hamza et al., 2007; Hamza et al., 2015).
Trypsin, chymotrypsin and amylase are among the key pancreatic enzymes that have been found in the larvae of Teleostei (Hoehne-Reitan and Kjorsvik, 2004). Trypsin is an important indicator of nutritional condition that is directly connected to protein metabolism. Its activity has been detected during the larval development of most studied marine fish (Cahu and Infante, 1995; Nolting et al., 1999; Infante and Cahu, 2001; Garcia-Gasca et al., 2006) and in some freshwater species (Chakrabarti et al., 2006; Silveira et al., 2013; Lahnsteiner, 2017; Palinska-Zarska et al., 2020). Activity of trypsin along with other pancreatic enzymes such as lipase and amylase are generally used as indexes of digestive function and maturation (Infante and Cahu, 1994; Ribeiro et al., 1999; Zambonino Infante and Cahu, 1999; Infante and Cahu, 2001).
Imentai et al. (2020) evaluated the growth, survival rate, and development of digestive organs using histological methods in S. lucioperca larvae under different feeding regimes with different times of shift in live preys rotifer to Artemia, at 17 DPH, age at which stomach has not been developed yet. The objectives of the present study were to determine the gene expression and activity levels of major digestive enzymes in S. lucioperca larvae in the same experimental design. Comparison between gene expression and actual activity of the different enzymes could provide a better understanding of the regulation mechanisms. To achieve this aim, expression of key pancreatic digestive proenzymes (trypsinogen 1, trypsin 1 precursor, try1; chymotrypsinogen b, chymotrypsin b precursor, ctrb; carboxyl ester lipase precursor; bile-salt activated lipase, cel; phospholipase a2, pla2g1b; pancreatic alpha amylase, amy2a), together with genes related to intestinal development and maturation (intestinal-type alkaline phosphatase, alpi; leucine aminopeptidase 3, lap3; aminopeptidase N, anpep) were assessed. Likewise, the activity of key enzymes: trypsin, lipase, alkaline phosphatase, amino peptidase, amylase and chymotrypsin were determined at 17 DPH.
Materials and Methods
Live Feed Culture
The rotifers Brachionus plicatilis used in this study were cultured in 50-L flat-bottomed tanks (n=3) at ambient temperature and salinity 30 ± 5 g L-1 using a batch culture protocol and fed Nannochloropsis sp. paste (Nanno 3600, ReedMariculture Inc., USA) at a rate of 1 mL L-1 of culture twice a day. The Artemia nauplii (Micro artemia cysts, Ocean Nutrition™, Belgium) were hatched (20-24 h) onsite following provided manual from the producer and were harvested every morning. After harvesting, the densities of both rotifers and Artemia (individuals mL-1) were estimated using a Sedgewick-Rafter counting chamber under a light microscope.
Fish and Experimental Design
Pikeperch larvae were reared at the Experimental Fish Facility of the Faculty of Fisheries and Protection of Waters, University of South Bohemia, Czech Republic. Newly hatched pikeperch larvae originating from nest spawning of pond-cultured broodstock (Malinovskyi et al., 2018) were acclimated to experimental RAS with water temperature of 15 ± 0.5°C at 3 DPH. At 4 DPH, the larvae (total length= 5.62 ± 0.03 mm, body weight = 0.66 ± 0.16 mg) were divided into five experimental groups with four replicates (2-L tanks) at initial density of 100 larvae per liter. All larvae at 5 DPH were initially fed with rotifers for 3 days and thereafter from 8 to 17 DPH were divided to 5 (A-E) different feeding regimes (Figure 1) and fed with rotifers and Artemia as follows: (A) larvae fed only with rotifers till 17 DPH; (B) larvae fed with rotifers till 14 DPH followed by feeding with Artemia till 17 DPH; (C) larvae fed with rotifers till 11 DPH followed by feeding with Artemia till 17 DPH; (D) larvae fed only with Artemia till 17 DPH; (E) larvae fed a combination of rotifers and Artemia till 17 DPH.
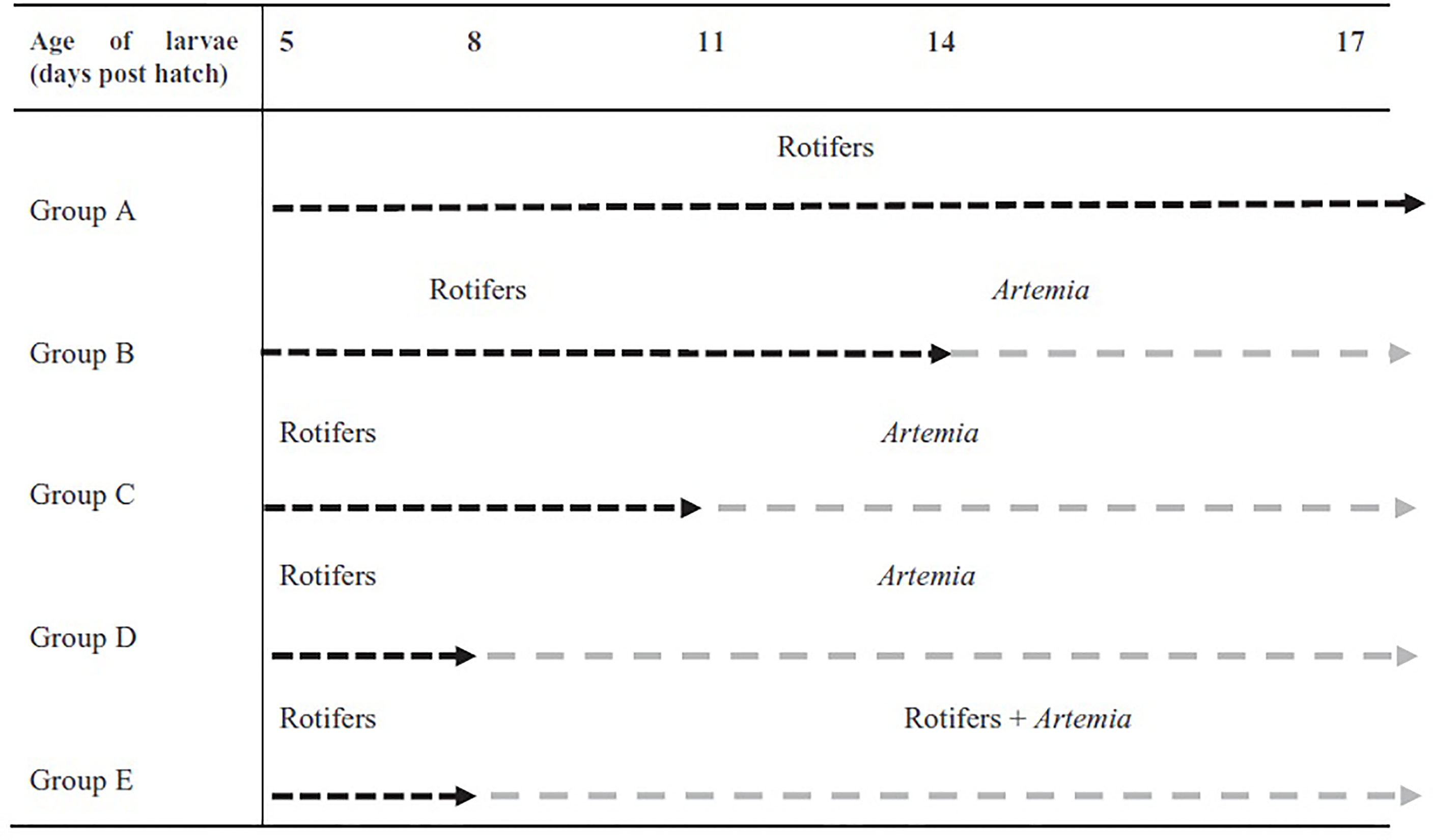
Figure 1 The applied feeding regimes for pikeperch larvae throughout the experiment (Imentai et al., 2020).
Frozen paste of algae was added to the larval tanks twice a day (2 x 300,000 cells/mL), beginning at 5 DPH and continuing throughout the experiment according to the mentioned protocol. Rotifers and Artemia were provided as live feed to larvae three times a day with residual counts prior to each feeding. Feeding densities were steadily increased based on residual counts, performed prior to each feeding (Supplementary Table 1). In order to improve larval feeding efficiency, prior to each feeding water flow was stopped and restarted in each tank after 2 h. Flow rates started at 100 mL/min and increased with time (Supplementary Table 1).
Water temperature, pH, and dissolved oxygen (DO) were measured before each feeding with a pH/temperature tester (HI98129, Hanna Combo) and an oxymeter (OxyGuard International A/S, Farum, Denmark) and the values were 17.8 ± 0.17°C, 7.3 ± 0.04 and 88.5 ± 2.53%, respectively. Total ammonia and nitrite concentrations were determined twice a week and maintained below 0.5 and 0.1 mg L-1, respectively. Light intensity on the water surface was 90-100 lux and photoperiod was set at 13L: 11D (07:00 to 20:00 h). Salinity was 2 ± 0.2 g L-1 which was kept constant by adding salt (Instant Ocean® Sea Salt) to the water of the whole culture system. Tanks were cleaned twice per day by siphoning out the faeces and dead larvae.
Sampling Procedure
At the end of the trial (17 DPH) all larvae were accounted for, and samples were collected to assess the larvae growth. Growth and survival rates were calculated using the following formula:
Specific growth rate (SGR, % day-1) = 100 (ln TLf- ln TLi)/t where TLi and TLf are initial and final total lengths, respectively, and t is the period in days.
Survival rate (%) = Final fish number/Initial fish number × 100
Sixty individuals per treatment were randomly collected and immediately frozen in liquid nitrogen and then stored at -80°C for enzyme activity and gene expression assays. For gene expression analyses, frozen larvae were transferred into an appropriate volume (1/10 w/v) of RNAlater®-ICE (Ambion Life Technologies), according to the manufacturer’s instructions, and were kept at -80 °C until RNA extraction.
Gene Expression of Digestive Proenzymes
The ZFIN Zebrafish Nomenclature Guidelines for gene and protein names and symbols (available here) were followed in this manuscript. Primers were designed using the Primer3 software v.0.4.0 (available here) and were synthesized by IDT® (Belgium).
Total RNA was extracted from individual whole larvae, homogenized by a Polytron® PT 1200 E with a dispersing tool PT-DA 03/2EC- E050 (Kinematica AG), and the NucleoSpin® XS kit (Macherey-Nagel). An on-column RNase-free DNase step was included to eliminate or reduce the genomic DNA. RNA quality and concentration were measured using the Bioanalyzer 2100 with the RNA 6000 Nano kit (Agilent Technologies, LifeSciences), and the Qubit® 2.0 Fluorometer with a Qubit® RNA BR Assay Kit (Invitrogen, Life Technologies), respectively. Samples with RNA integrity number higher than 8.0, were reverse transcribed using the qScript™ cDNA synthesis kit (Quanta BioSciences), where 250 ng of each total RNA sample was used as input in a total reaction volume of 20 µL. Afterwards, each sample was diluted with 10 mM Tris-HCl, 0.1 mM EDTA (pH 8) to a final concentration of 1.25 ng μL−1. QPCR reactions were run in Hard-Shell® Low-Profile Thin-Wall 96-Well Skirted PCR plates, with Microseal® B Adhesive Seals (BioRad), with 5 ng of cDNA (assumed from RNA input), 200 nM of each one of the forward and reverse primers (Supplementary Table 2), and IQ™ SYBR® Green Supermix (BioRad), in a final volume of 10 µL. Cycling program of 95°C, 10 min; [95°C, 15 s; 60°C, 30 s] × 40 cycles; melting curve 60-95°C, 0.5°C/5 s, was performed in a CFX Connect™ and a CFX 96 Real-Time Detection System with BioRad CFX Maestro Software v2.0 (BIORAD Laboratories).
Relative gene expression was calculated by the ΔΔCT method (Livak and Schmittgen, 2001) corrected for efficiencies (Pfaffl, 2001), where actin beta (actb) and eukaryotic elongation factor 1-alpha (eef1a) were used as internal reference genes to normalize the results, and a pool of cDNA samples belonging to the control group (C) were used as the calibrator to correct for inter-assay variations on all the plates. Adequacy of the actb and eef1a as internal control genes was confirmed by the target stability value (M = 0.61) and the coefficient of variance (CV = 0.21), established by the BioRad CFX Maestro Software. To verify the amplification efficiency, before analysis of the samples, 1:10 serial dilution of the calibrator (5 ng to 50 fg) was used to construct the calibration curves, with several pairs of primers. No template control (NTC) and no reverse transcriptase control (NTR) were included in the curves to discard the presence of artifacts and genomic DNA contaminations. Primers with a curve interpolating at least three points over six, efficiency (E) within the range of 90-110%, and a coefficient of determination (R2) higher than 0.98 were selected for the QPCR analyses. Details regarding the sequences of the primers, amplicon size, amplification range, and accession number of their sources are detailed in Supplementary Table 2.
Enzyme Activity Analyses
The activity of different digestive enzymes was determined using both fluorometric and colorimetric methods on samples formed by 3 pools of 15 larvae for each treatment. The fluorometric methods were applied to the determination of trypsin, chymotrypsin and lipase activities while colorimetric methods were used to determine amylase, alkaline phosphatase and aminopeptidase. Trypsin was assayed using Boc-Gln-Ala-Arg-7-amido-4-methylcoumarin hydrochloride and chymotrypsin was determined using ala-ala-phe-7-amido-4-methylcoumarin according to Rotllant et al. (2008). In both cases, a 10 μL volume of the diluted homogenate was mixed with 5 μL of the substrate and 195 μL of 50 mM Tris–HCl, 10 mM CaCl2 buffer (pH 8.0) in microplate wells. Fluorescence was measured at Λex/Λem of 380/440 for 5 min. Lipase activity was quantified using 4-methylumbelliferyl butyrate following the method described by Vaneechoutte et al. (1988). A stock solution was made by dissolving 100 mg of MUB in 10 mL DMSO, to which 100 μL Triton X-100 was added; the mixture was stored at –80°C. This stock solution was diluted in phosphate buffer pH 7.0 reaching a final concentration of 0.4 mM MUB. A 10 μL volume of the diluted homogenate (1:10) was added to the microplate well and mixed with 250 μL of MUB 0.4 mM. Fluorescence was measured at Λex/Λem of 355/460 nm. In these cases one unit of activity corresponded to 1 µmol of either methylcoumarin or methylumbelliferone released per min. Amylase activity was measured using soluble starch as substrate and 3,5-dinitrosalicylic acid (DNS) as specific reagent to determine reducing sugars, according to Miller (1959). 250 μL of starch solution and 250 μL of diluted sample with citrate buffer 50 mM at pH 6.5 were added to the test tubes which were incubated at 25°C for 15 min. Thereafter, 500 μL of DNS was added to the mixture and the tubes were placed in a bath at 100°C for 5 minutes; after cooling to room temperature, 5 ml of distilled water was added and the absorbance was read at 540 nm. The calibration curves were made with concentrations ranging from 0 to 2 gL−1 of glucose in citrate buffer. One unit of enzyme activity was defined as the amount of enzyme needed to release 1 μmol of glucose per minute under standard assay conditions. Leucine aminopeptidase was determined according to Maraux et al., (1973) using sodium phosphate buffer 50 mM at pH 7.2, and the substrate leucine p-nitroanilide (0.1 mM dissolved in DMSO), being readings carried out at 410 nm. Enzymatic activity was defined as 1 µmol of nitroanilide released per minute. Alkaline phosphatase activity was determined following the method described by Bergmeyer (1974) using a solution of 2% 4-nitrophenyl phosphate in NaOH-glycine 100 mmol l-1 buffer at pH 10.1. The reaction was stopped by adding a solution of NaOH (0.05 N) and the absorbance was measured at 405 nm. One unit of activity was defined as the liberation of 1 µmol of nitrophenyl per minute.
Statistical Analyses
All data are presented as mean ± the standard deviation (SD). Data were analyzed with the program RStudio (R Core Team, 2014). Data were analyzed for possible outliers using the Grubbs’ test (ESD method). Normal distribution of data and homogeneity of variance were confirmed by Shapiro-Wilk and Levene’s tests, respectively. Comparisons for gene expression were made using one-way ANOVA followed by Tukey post-hoc test when significant differences were found at p<0.05. In addition, PCA were carried out in order to summarize data structure and to integrate the information obtained through the measurement of multiple gene expression and enzyme activities. These analyses were carried out using the software Statgraphics Centurion (Statgraphics Technologies, The Plains, VI.EE. UU.).
Results
The survival rate was significantly higher in larvae from experimental groups C (54%), D (55%) and E (67%) (p<0.05) (Table 1). The group fed only with rotifers (Group A) showed significantly lower specific growth rate (SGR) than the other groups, and the highest SGR was found in the group fed with combination of rotifers and Artemia after 3 day rotifer feeding (Group E). The highest value of standard length (8.32 ± 0.48 mm) was found in the group fed with combination of rotifers and Artemia after 8 DPH (group E) (Table 1).

Table 1 Survival, SGR and standard length of S. lucioperca larvae at 17 (n= 60) days post hatch (DPH).
Gene Expression of Digestive Proenzymes
Gene expressions data are presented in Figure 2. Among the eight studied genes, seven showed significant differences in expression between dietary treatments and one (alpi) presented no significant interaction in expression among the experimental groups (p<0.05). The expression of anpep, lap3 and try1 was significantly upregulated in larvae fed rotifers for initial 3 days followed by feeding a combination of rotifers and Artemia (Group E) compared with other treatments (p<0.05). Although no significant, a similar trend was observed for alpi expression where larvae with feeding regime E showed also a higher value. The expression of amy2a, ctrb, pla2g1b, and try1 was significantly lower in larvae fed rotifers until 14 DPH and replaced by Artemia afterwards (Group B).
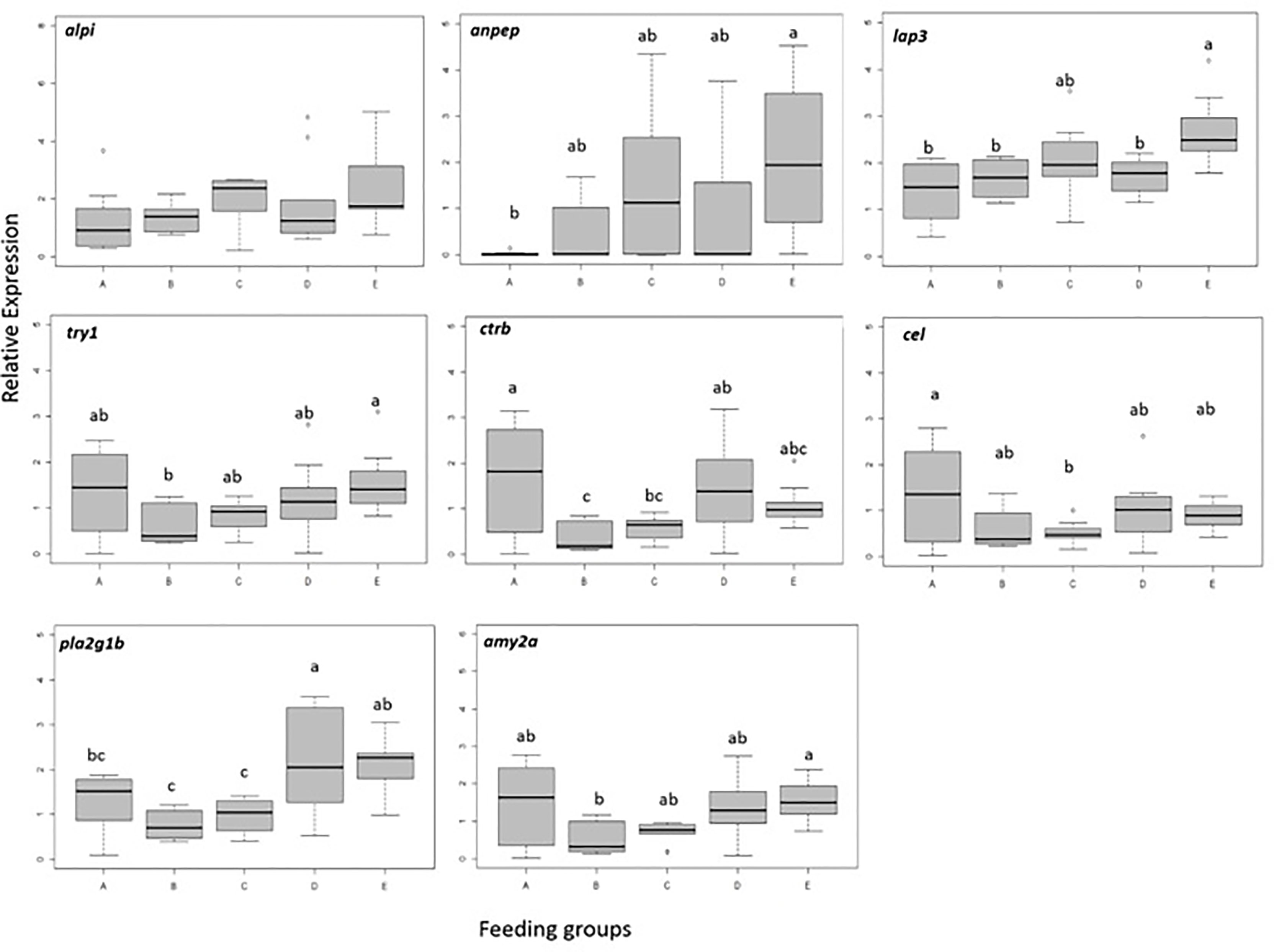
Figure 2 Relative expression of genes in pikeperch larvae at the end of experimental period (17 DPH) at different feeding regimes (n=10 larvae per group). Different letters denote significant (p<0.05) differences among feeding groups. Box limits correspond to upper and lower quartiles, whiskers to minimum and maximum values, horizontal bar to the median, and points show outliers outside the 1.5 times interquartile range.
Results of the PCA carried out on gene expression data are summarized in Figure 3. The combination of several variables into two principal components explained 80.8% of total variability observed among data. The PC1 in vertical axis was defined by expression of genes encoding digestive enzymes (amy, cel, ctr, try and to a lesser extent pla) which showed a clear correlation among them. The PC2 in the horizontal axis was defined by the expression of indicators of intestinal maturation; anpep and to a lesser extent alpi and lap3. The pattern of distribution of the samples belonging to each experimental group along the two axes evidenced some clear trends. A large proportion of A-labeled individuals were grouped in the area corresponding to negative values defined by the vectors in PC1, this meaning they present low values of expression of the digestive proenzymes. In contrast, B-labeled individuals were clustered in the opposite area, indicating higher values of expression for most digestive proenzymes. The remaining groups of individuals labelled C, D and E showed intermediate values of expression of such genes. In addition, the expression of genes related to maturation of the brush border membranes appeared to be low in groups A and B, high in the E-labeled group, and those in groups C and D showed a great variability and not a clear pattern.
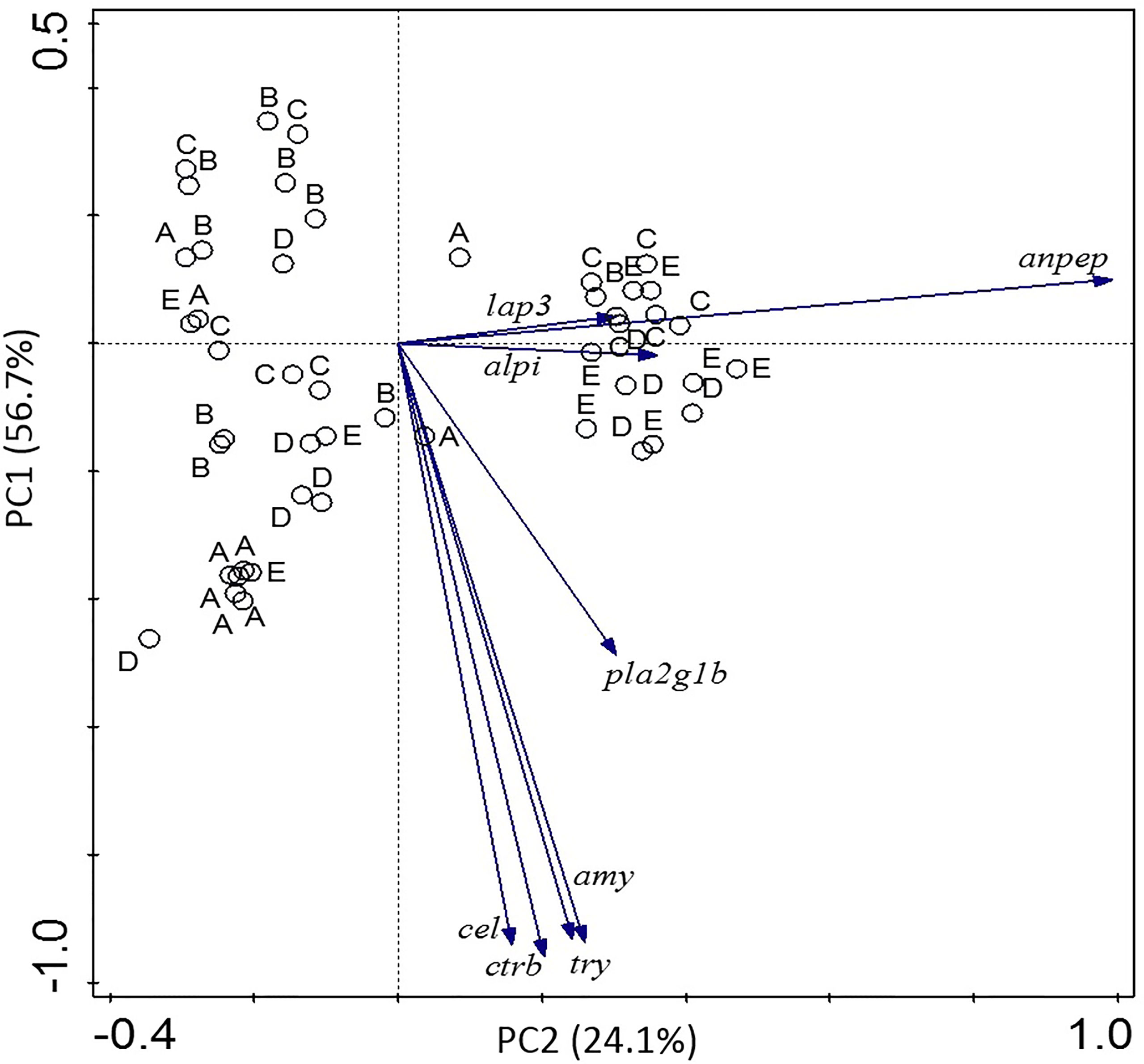
Figure 3 Principal component analysis (PCA) correlation biplot based on the first two principal components (PC1 and PC2) generated from gene expression of different digestive enzymes and indicators of intestinal maturation in the different experimental groups.
Enzyme Activity
The effect of different feeding regimes on the activities of digestive enzymes in pikeperch larvae is detailed in Figure 4. At 17 DPH, from the six tested digestive enzymes, two (amylase and chymotrypsin) did not show any activity while the rest displayed a significant difference among groups. The highest trypsin activity was observed in larvae from experimental groups B and E (p<0.05). There was no significant difference in trypsin activity among the other groups (p<0.05). Lipase activity was significantly lower in larvae from treatment A compared with the other groups (p<0.05). Data also revealed a significantly lower activity of alkaline phosphatase (AP) in larvae from group A (p<0.05). Whereas AP activity was highest in larvae from groups D and E (p<0.05). Similar to AP, Aminopeptidase N (AN) highest activity levels were found in larvae from treatments D and E (p<0.05).
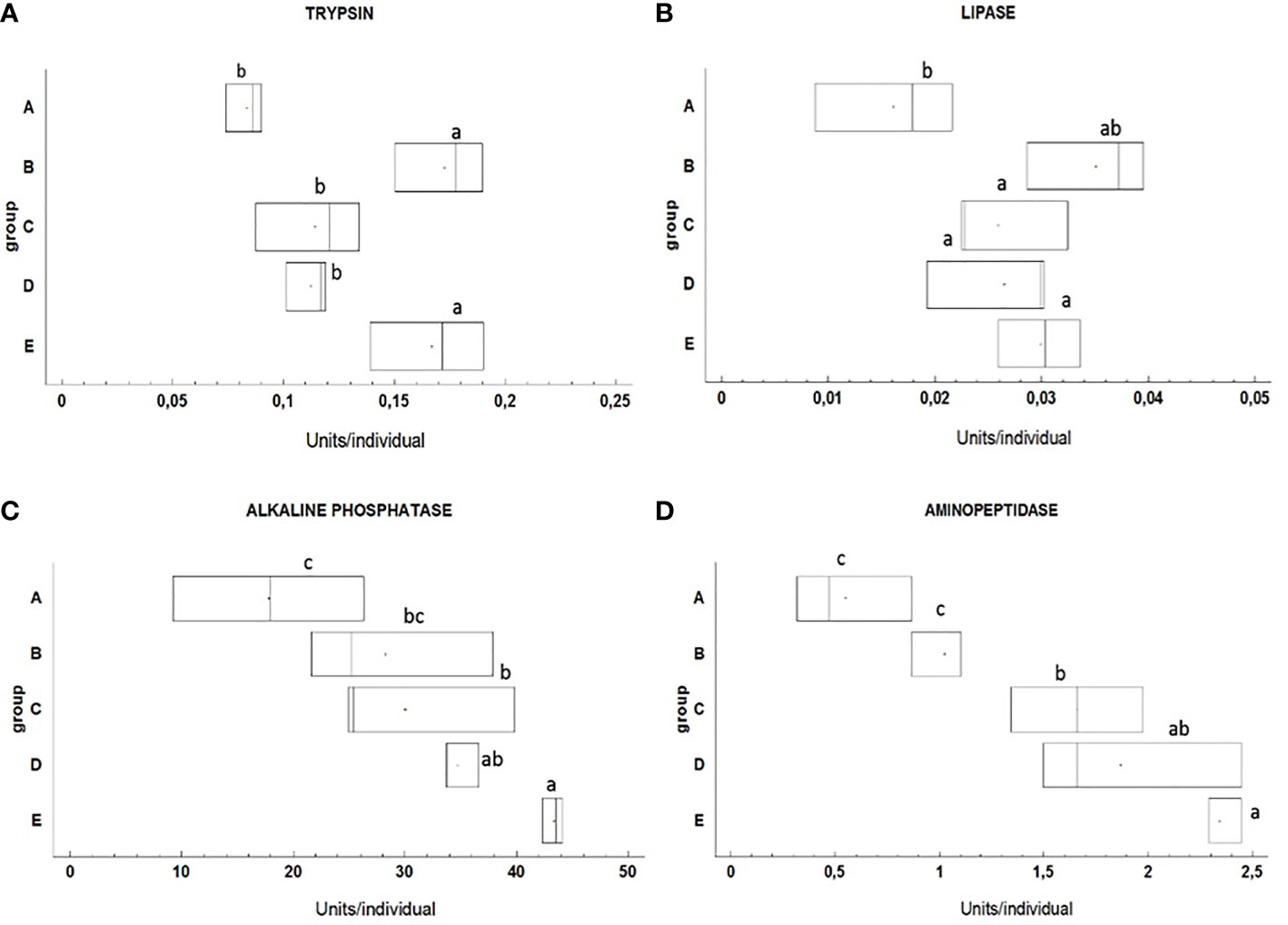
Figure 4 The activities of trypsin (A), lipase (B), alkaline phosphatase (C) and aminopeptidase (D) in pikeperch larvae at the end of experimental period (17 DPH) at different feeding regimes (n=3 pools of 15 larvae per group). Different letters indicate significant differences among groups (p<0.05). Box limits correspond to upper and lower quartiles, horizontal bar to the median, and points show mean values.
The PCA analysis performed with data from enzyme activities is detailed in Figure 5. In this case, the combination of different values of enzyme activity into two principal components accounted for 90.2% of total variability observed among data. The PC1 in the horizontal axis discriminated between individuals with generally high or low enzyme activities. The pattern of distribution of the data clearly segregated A-labeled samples, which presented the lower levels of activities and also the B-labeled samples, which presented high activities of trypsin and lipase but very low of AP and AN. Samples in groups C, D and E presented equilibrated profiles of both luminal and intestinal enzymes.
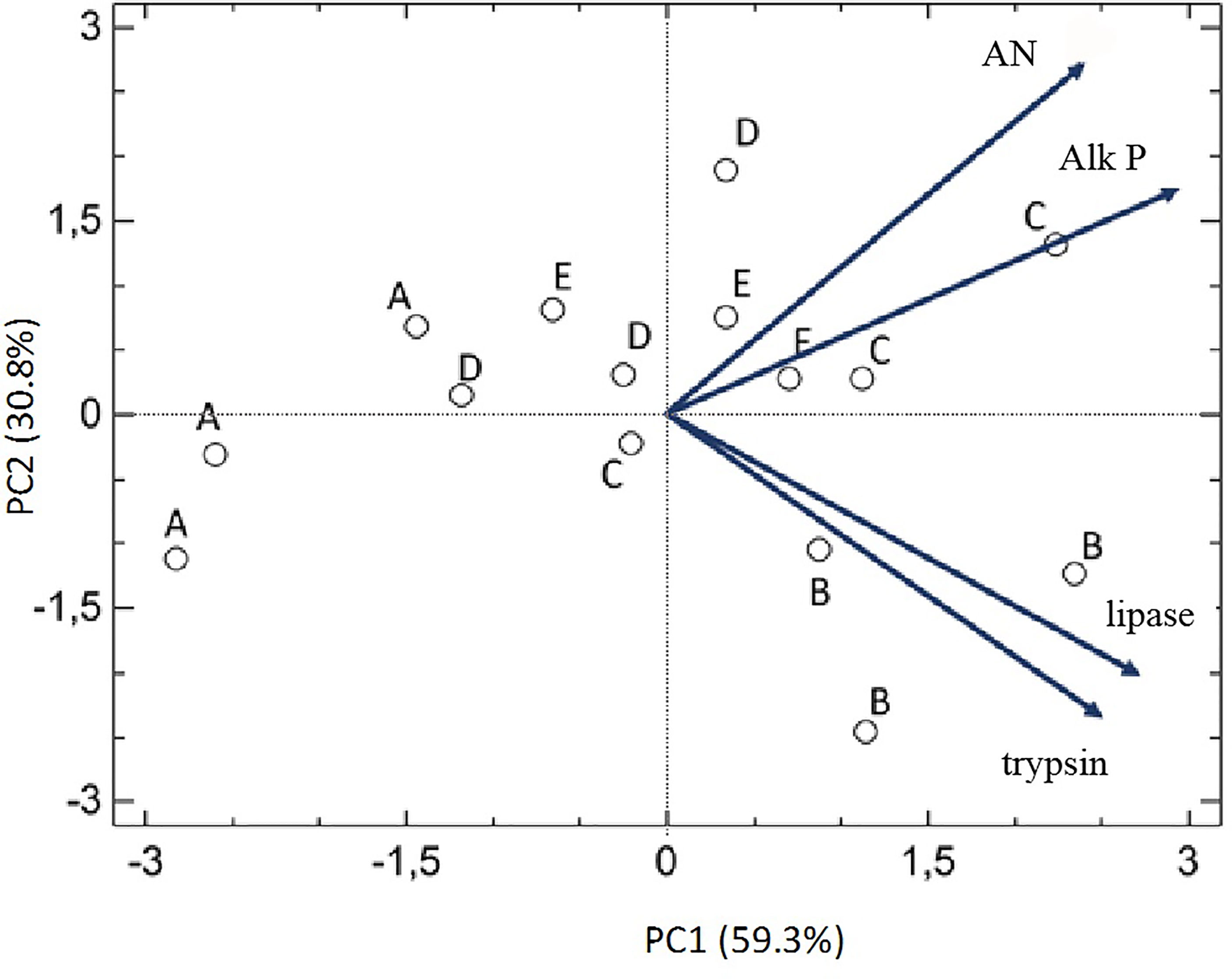
Figure 5 Principal component analysis (PCA) correlation biplot based on the first two principal components (PC1 and PC2) generated from the activity of different digestive enzymes in the different experimental groups.
Discussion
At present, molecular studies in pikeperch larvae are limited. In this study, the analysis of expression of key genes of digestive proenzymes and activities of selected digestive enzymes was conducted to evaluate the mechanisms involved in digestion under different feeding regime from 4 till 17 DPH of pikeperch larvae.
Several studies have reported the ontogenetic development of the digestive system of pikeperch (Kowalska et al., 2006; Hamza et al., 2007). In pikeperch larvae both pancreatic and intestinal enzyme activities already appear at hatching (Hamza et al., 2007). Therefore, it is most likely that these activities are genetically programed (Hamza et al., 2015). Leucine aminopeptidase (Lap) is located on the brush border membrane and its activity indicates the intestine develop into the adult mode of digestion, improving luminal digestive capacity (Infante and Cahu, 2001). In the present study, lap3 showed the highest expression in larvae fed rotifers and Artemia from 8 DPH until 17 DPH (Group E). Similarly, expression levels of anpep were high in Group E. The intestine lap3 and anpep genes expression results obtained for pikeperch larvae in this study was agreement with the survival rate and histological analysis of intestine in study published by Imentai et al. (2020). In contrast, an upregulation of anpep expression was observed in larvae of Atlantic bluefin tuna fed rotifers compared with copepods, which could be due to the enrichment of rotifers (Betancor et al., 2017). In our study, rotifers that were fed on Nannochloropsis sp. and Artemia were immediately used after hatching. Moreover, in this study a diluted paste of Nannochloropsis sp. was added to all larval tanks. Previous studies have reported that addition of algae during rearing larvae increases growth, survival and has a beneficial effect on the development of digestive tract (Naas and Harboe, 1992; Navarro and Sarasquete, 1998; Skjermo and Vadstein, 2020). Similarly, in this study the highest aminopeptidase (AN) activity was also detected in groups D and E. This together with the significant upregulation of anpep in Group E indicates a better efficiency of the digestive processes and the intestinal absorptive capacity in these treatments (Infante and Cahu, 2001). AN is an important intestinal brush border enzyme (Eichholz, 1968) involved in the fermentation process, and its main role is to facilitate the absorption and transport of nutrients through the enterocytes (Cahu and Infante, 2001). Conversely, alpi expression was insignificant to different prey shift time in S. lucioperca larvae. Alkaline phosphatase (AP) is an esterase, and its activity is produced by phosphorylated substrates such as phospholipids and phosphoproteins. AP has often been considered as a sensitive indicator of the nutritional status of larvae (Cahu and Infante, 1994; Ribeiro et al., 2002; Kamaszewski et al., 2014). In the present study, its specific activity was significantly higher in larvae fed Artemia alone or in combination with rotifer from 8 DPH (Groups D and E). This together with the trend of higher alpi expression level in Group E may suggest a better development and intestinal maturation process in these feeding regimes (Infante and Cahu, 2001). Ribeiro et al. (2002) reported higher AP activity in Solea senegalensis larvae fed Artemia compared with artificial compound diet.
Previous studies have shown that pancreatic enzymes such as trypsin, lipase, phospholipase, or amylase are regulated at a transcriptional level during larval development (Cahu and Infante, 2001; Kurokawa et al., 2002; Cahu et al., 2003). In this study the lipase activity was significantly higher in fish fed Artemia compared with larvae fed exclusively on rotifers. While amylase specific activity are usually reported in pikeperch larvae from hatching (Hamza et al., 2015) and chymotrypsin is detected during first feeding (Ljubobratovic et al., 2020), our attempts to detect those enzymes by using a highly sensitive fluorometric substrate and colorimetric method has failed. Similar results were found in Chelon labrosus larvae in which, in spite of detection of ctr transcripts, activity levels were inexistent (Gilannejad et al., 2020). According to the authors, higher levels of chymotrypsin activity might be necessary in larger animals.
The expression of pla2g1b, one of the genes involved in the digestion of lipids, was shown in this study to be influenced by prey shift time of pikeperch larvae. In our study, pla2g1b was upregulated in larvae fed rotifers and Artemia from 8 DPH until 17 DPH continuously (Group E). On the contrary, down regulation of pla2g1b expression in our study, showing that introducing Artemia later than 11 DPH (Groups A, B and C) might inhibit the hydrolysis of the sn-2 position of glycerophospholipids (Okamura et al., 2021). In our study, try1 expression increased with earlier introduction of Artemia into diet and was down regulated in larvae fed Artemia from 14 DPH (Group B). This decrease in the trypsinogen expression could be attributed to the increased contribution of the acid digestion (Srichanun et al., 2013).
Trypsin is an important indicator of nutritional condition that is directly connected to protein metabolism. It is mainly influenced by protein content and amino acid profile of the diet (Grendell and Rothman, 1981; Tseng et al., 1982). In the present study, try1 showed the highest expression in larvae fed rotifers and Artemia from 8 DPH until 17 DPH (Group E) and corresponds to higher specific activity in this group. While for Group B, larvae fed Artemia from 14 DPH try1 expression and specific activity were opposite. This could be attributed to differences in post transcriptional/translational regulatory mechanism leading to higher activity in this group. Hamza et al. (2007) reported the trypsin activity in pikeperch from hatching and observed its significantly higher activity in larvae fed Artemia compared with the weaned larvae.
Principal component analysis (PCA) is a technique for reducing the dimensionality of large datasets that are often difficult to interpret. It has been applied in a wide number of studies to synthetize information obtained from the analysis of multiple digestive enzymes. As an example, PCA was used to evaluate information obtained from the whole spectrum of activity of digestive enzymes in different species of Annelidae in order to identify their food preferences (Cichocka et al., 2021). Also, the digestive enzyme profile was integrated with other parameters (growth related genes, immune and stress indicators) to identify biological responses to the inclusion of camelina meal in diets of red seabream Pagrus major (Mzengereza et al., 2021). In addition, PCA served to discriminate functionality of different regions in the digestive system of different species like the limpet Megathura crenulata (Martin et al., 2011) or the common octopus (Bastos et al., 2020). In the present study, PCA was used to integrate the above-described information obtained from the expression of selected genes and activities of digestive enzyme in groups of pikeperch larvae receiving different feeding patterns. Results of PCA performed both on gene expression and enzyme activities were greatly coincident and clearly identified a less favorable status in larvae fed only with rotifers (A) which showed low enzyme activity and poor intestinal maturation. Feeding larvae with rotifers till 14 DPH followed by Artemia till 17 DPH (group B) seems to have a positive impact on the expression and activity of digestive enzymes, but not on the intestinal maturation, while in contrast, the combination of rotifers and Artemia till 17 DPH positively influenced markers of intestinal maturation and activity of brush border enzymes, although not so clearly the gene expression and activity of luminal digestive enzymes.
It is well documented that activities of digestive enzymes can vary depending on species, age, and diet, among others (Buddington and Doroshov, 1986; Infante and Cahu, 2001). At the early stages when the stomach has not been developed yet, the intestine and pancreas play a key role in food storage, protein hydrolyses, emulsification and feed transit modulation, all these processes being regulated by complex neuroendocrine mechanisms (Holmgren and Olsson, 2009). Pikeperch larvae, from the beginning of exogenous feeding, have a poor or moderately developed digestive system and mostly during the feeding period its enzymes activities are increasing (Lahnsteiner, 2017). The results based on activities of digestive enzymes showed that earlier shift time to Artemia and combination of rotifers and Artemia during first feeding larvae leaded to a higher activity of analyzed enzymes. This could be mainly attributed to a different nutrient composition of diets. Several studies have previously reported the difference at the protein level and amino acid profile between live preys, e.g. rotifers and Artemia (Lubzens et al., 1989; Chakraborty et al., 2007; Yanes-Roca et al., 2018). Artemia is a protein source with high digestibility in fish larvae (Rønnestad and Conceição, 2005), however without enrichment it does not fully cover the nutritional needs of larvae at the beginning of first feeding. While enriched Artemia is difficult to ingest particularly for a small mouth size larvae (Majoris et al., 2018). Although, the same prey can become suitable diet once gape size increases (Yufera and Darias, 2007). Yanes-Roca et al. (2018) suggested that rotifers are suitable for pikeperch larvae from the beginning of exogenous feeding (4-5 DPH) until 12 DPH due to their size and nutritional value. However, exclusive rotifer feeding from 12 DPH is a limit for pikeperch larvae which should be fed by combination of rotifers and Artemia from this age and later by Artemia forward to weaning.
In conclusion, the findings in this study showed that the activity of digestive enzymes and the gene expression was affected by feeding regime. The activities were increased in larvae that were co-fed or replaced on Artemia from 8 DPH until 17 DPH. This might indicate that combination of two diets promotes appetite or that the larvae were at the sub-optimal feeding status. The present findings corroborate previous study (Imentai et al., 2020), that feeding pikeperch larvae with B. plicatilis from 5 to 8 DPH and afterwards exclusively with Artemia or combination of rotifers and Artemia till 17 DPH can ensure high survival, growth rates, better development of digestive organs, and increased activity of digestive enzymes.
Data Availability Statement
The original contributions presented in the study are included in the article/Supplementary Material. Further inquiries can be directed to the corresponding author.
Ethics Statement
The animal study was reviewed and approved by EU-harmonized Animal Welfare Act of the Czech Republic.
Author Contributions
AI designed the study, performed the experiment, analyzed data, and wrote the manuscript. NG and GM-R analyzed the gene expression data and contributed to the revision of the manuscript. FML and FM analyzed the digestive enzymes data and FML contributed to the revision of the manuscript. TPě contributed to the statistical analysis. VD and HD gave valuable advice. TPo supervision and funding acquisition. All authors have read and approved the final version of this article.
Funding
The study was financially supported by the Ministry of Agriculture of the Czech Republic, project NAZV QK22020144.
Conflict of Interest
The authors declare that the research was conducted in the absence of any commercial or financial relationships that could be construed as a potential conflict of interest.
Publisher’s Note
All claims expressed in this article are solely those of the authors and do not necessarily represent those of their affiliated organizations, or those of the publisher, the editors and the reviewers. Any product that may be evaluated in this article, or claim that may be made by its manufacturer, is not guaranteed or endorsed by the publisher.
Supplementary Material
The Supplementary Material for this article can be found online at: https://www.frontiersin.org/articles/10.3389/fmars.2022.864536/full#supplementary-material
Abbreviations
Actb, actin beta; alpi, intestinal-type alkaline phosphatase; amy2a, pancreatic alpha amylase; anpep, aminopeptidase N; cel, carboxyl ester lipase precursor; bile-salt activated lipase; ctrb, chymotrypsinogen b; chymotrypsin b precursor; eef1a, eukaryotic elongation factor 1-alpha; lap3, leucine aminopeptidase 3; pla2g1b, phospholipase a2; try1, trypsinogen 1; trypsin 1 precursor; AP, Alkaline phosphatase; AN, Aminopeptidase N.
References
Baekelandt S., Mandiki S. N. M., Schmitz M., Kestemont P. (2019). Influence of the Light Spectrum on the Daily Rhythms of Stress and Humoral Innate Immune Markers in Pikeperch Sander Lucioperca. Aquaculture 499, 358–363. doi: 10.1016/j.aquaculture.2018.09.046
Bastos P., Fracalossi D. M., Chimal M. E., Sanchez A., Rosas C. (2020). Digestive Enzymes and Timing of Digestion in Octopus Vulgaris Type II. Aquaculture Rep. 16. doi: 10.1016/j.aqrep.2019.100262
Bergmeyer H. V. (1974). Phosphatases Methods of Enzymatic Analysis Vol. 2 (New York: Academic Press).
Betancor M. B., Ortega A., de la Gandara F., Tocher D. R., Mourente G. (2017). Molecular Aspects of Lipid Metabolism, Digestibility and Antioxidant Status of Atlantic Bluefin Tuna (T. Thynnus L.) Larvae During First Feeding. Aquaculture 479, 357–369. doi: 10.1016/j.aquaculture.2017.06.011
Buddington R. K., Doroshov S. I. (1986). Digestive Enzyme Complement of White Sturgeon (Acipenser Transmontanus). Comp. Biochem. Physiol. a-Physiol 83, 561–567. doi: 10.1016/0300-9629(86)90146-5
Cahu C. L., Infante J. L. Z. (1994). Early Weaning of Sea Bass (Dicentrarchus-Labrax) Larvae With a Compound Diet - Effect on Digestive Enzymes. Comp. Biochem. Physiol. a-Molecular Integr. Physiol. 109, 213–222. doi: 10.1016/0300-9629(94)90123-6
Cahu C. L., Infante J. L. Z. (1995). Maturation of the Pancreatic and Intestinal Digestive Functions in Sea Bass (Dicentrarchus Labrax): Effect of Weaning With Different Protein Sources. Fish Physiol. Biochem. 14, 431–437. doi: 10.1007/Bf00004343
Cahu C., Infante J. Z. (2001). Substitution of Live Food by Formulated Diets in Marine Fish Larvae. Aquaculture 200, 161–180. doi: 10.1016/S0044-8486(01)00699-8
Cahu C. L., Infante J. L. Z., Barbosa V. (2003). Effect of Dietary Phospholipid Level and Phospholipid : Neutral Lipid Value on the Development of Sea Bass (Dicentrarchus Labrax) Larvae Fed a Compound Diet. Br. J. Nutr. 90, 21–28. doi: 10.1079/Bjn2003880
Chakrabarti R., Rathore R. M., Kumar S. (2006). Study of Digestive Enzyme Activities and Partial Characterization of Digestive Proteases in a Freshwater Teleost, Labeo Rohita, During Early Ontogeny. Aquaculture Nutr. 12, 35–43. doi: 10.1111/j.1365-2095.2006.00379.x
Chakraborty R. D., Chakraborty K., Radhakrishnan E. V. (2007). Variation in Fatty Acid Composition of Artemia Salina Nauplii Enriched With Microalgae and Baker's Yeast for Use in Larviculture. J. Agric. Food Chem. 55, 4043–4051. doi: 10.1021/jf063654l
Cichocka J. M., Bielecki A., Swiatek P., Jablonska-Barna I., Kobak J., Hildebrand J., et al. (2021). The Activity of Hydrolytic Enzymes in the Digestive System of Acanthobdellida, Branchiobdellida and Hirudinida (Annelida, Clitellata) - Considerations on Similarity and Phylogeny. Eur. Zoological J. 9, 26–43. doi: 10.1080/24750263.2020.1851402
Eichholz A. (1968). Studies on the Organization of the Brush Border in Intestinal Epithelial Cells: V. Subfractionation of Enzymatic Activities of the Microvillus Membrane. Biochim. Biophys. Acta (BBA)-Biomembranes 163, 101–107. doi: 10.1016/0005-2736(68)90037-0
Garcia-Gasca A., Galaviz M. A., Gutierrez J. N., Garcia-Ortega A. (2006). Development of the Digestive Tract, Trypsin Activity and Gene Expression in Eggs and Larvae of the Bullseye Puffer Fish Sphoeroides Annulatus. Aquaculture 251, 366–376. doi: 10.1016/j.aquaculture.2005.05.029
Gilannejad N., Moyano F. J., Martinez-Rodriguez G., Yufera M. (2021). The Digestive Function of Gilthead Seabream Juveniles in Relation to Feeding Frequency. Aquaculture 531. doi: 10.1016/j.aquaculture.2020.735867
Gilannejad N., de Las Heras V., Martos-Sitcha J. A., Moyano F. J., Yúfera M., Martínez-Rodríguez G. (2020). Ontogeny of Expression and Activity of Digestive Enzymes and Establishment of gh/igf1 Axis in the Omnivorous Fish Chelon labrosus. Animals 10 (5), 874.
Govoni J. J., Boehlert G. W., Watanabe Y. (1986). The Physiology of Digestion in Fish Larvae. Environ. Biol. Fishes 16, 59–77. doi: 10.1007/Bf00005160
Grendell J. H., Rothman S. (1981). Digestive End Products Mobilize Secretory Proteins From Subcellular Stores in the Pancreas. Am. J. Physiology-Gastrointestinal Liver Physiol. 241, G67–G73. doi: 10.1152/ajpgi.1981.241.1.G67
Hamza N., Mhetli M., Kestemont P. (2007). Effects of Weaning Age and Diets on Ontogeny of Digestive Activities and Structures of Pikeperch (Sander Lucioperca) Larvae. Fish Physiol. Biochem. 33, 121–133. doi: 10.1007/s10695-006-9123-4
Hamza N., Ostaszewska T., Kestemont P. (2015). “Development and Functionality of the Digestive System in Percid Fishes Early Life Stages”, In Biology and Culture of Percid Fishes (Springer: Dordrecht), 239–264.
Hoehne-Reitan K., Kjorsvik E. (2004). Functional Development of the Liver and Exocrine Pancreas in Teleost Fish. Dev. Form Funct. Fishes Question Larval Adaptation 40, 9–36.
Holmgren S., Olsson C. (2009). The Neuronal and Endocrine Regulation of Gut Function. Fish Physiol. 28, 467–512. doi: 10.1016/S1546-5098(09)28010-1
Imentai A., Raskovic B., Steinbach C., Rahimnejad S., Yanes-Roca C., Policar T. (2020). Effects of First Feeding Regime on Growth Performance, Survival Rate and Development of Digestive System in Pikeperch (Sander Lucioperca) Larvae. Aquaculture 529. doi: 10.1016/j.aquaculture.2020.735636
Infante J. L. Z., Cahu C. (1994). Development and Response to a Diet Change of Some Digestive Enzymes in Sea Bass (Dicentrarchus-Labrax) Larvae. Fish Physiol. Biochem. 12, 399–408. doi: 10.1007/Bf00004304
Infante J. L. Z., Cahu C. L. (2001). Ontogeny of the Gastrointestinal Tract of Marine Fish Larvae. Comp. Biochem. Physiol. C-Toxicol Pharmacol. 130, 477–487. doi: 10.1016/S1532-0456(01)00274-5
Kamaszewski M., Ostaszewska T., Prusinska M., Kolman R., Chojnacki M., Zabytyvskij J., et al. (2014). Effects of Artemia Sp Enrichment With Essential Fatty Acids on Functional and Morphological Aspects of the Digestive System in Acipenser Gueldenstaedtii Larvae. Turkish J. Fisheries Aquat. Sci. 14, 929–938. doi: 10.4194/1303-2712-v14_4_12
Kestemont P., Xu X. L., Hamza N., Maboudou J., Toko I. I. (2007). Effect of Weaning Age and Diet on Pikeperch Larviculture. Aquaculture 264, 197–204. doi: 10.1016/j.aquaculture.2006.12.034
Khendek A., Chakraborty A., Roche J., Ledore Y., Personne A., Policar T., et al. (2018). Rearing Conditions and Life History Influence the Progress of Gametogenesis and Reproduction Performances in Pikeperch Males and Females. Animal 12, 2335–2346. doi: 10.1017/S1751731118000010
Kowalska A., Zakęś Z., Demska-Zakęś K. (2006). The Impact of Feeding on the Results of Rearing Larval Pikeperch, Sander Lucioperca (L.), With Regard to the Development of the Digestive Tract. Electronic J. Polish Agric. Universities Fisheries 9. Available at: http://www.ejpau.media.pl/volume9/issue2/art-05.html.
Kristan J., Blecha M., Policar T. (2016). Alcalase Treatment for Elimination of Stickiness in Pikeperch (Sander Lucioperca L.) Eggs Under Controlled Conditions. Aquaculture Res. 47, 3998–4003. doi: 10.1111/are.12850
Kurokawa T., Suzuki T., Ohta H., Kagawa H., Tanaka H., Unuma T. (2002). Expression of Pancreatic Enzyme Genes During the Early Larval Stage of Japanese Eel Anguilla Japonica. Fisheries Sci. 68, 736–744. doi: 10.1046/j.1444-2906.2002.00487.x
Lahnsteiner F. (2017). Digestive Enzyme System of Larvae of Different Freshwater Teleosts and Its Differentiation During the Initial Phase of Exogenous Feeding. Czech J. Anim. Sci. 62, 403–416. doi: 10.17221/25/2016-Cjas
Livak K. J., Schmittgen T. D. (2001). Analysis of Relative Gene Expression Data Using Real-time Quantitative PCR and the 2– ΔΔCT Method. Methods 25 (4), 402–408.
Ljubobratovic U., Kosanovic D., Demeny F. Z., Krajcsovics A., Vukotic G., Stanisavljevic N., et al. (2020). The Effect of Live and Inert Feed Treatment With Lactobacilli on Weaning Success in Intensively Reared Pike-Perch Larvae. Aquaculture 516. doi: 10.1016/j.aquaculture.2019.734608
Lubzens E., Tandler A., Minkoff G. (1989). Rotifers as Food in Aquaculture. Hydrobiologia 186, 387–400. doi: 10.1007/Bf00048937
Majoris J. E., Francisco F. A., Atema J., Buston P. M. (2018). Reproduction, Early Development, and Larval Rearing Strategies for Two Sponge-Dwelling Neon Gobies, Elacatinus Lori and E-Colini. Aquaculture 483, 286–295. doi: 10.1016/j.aquaculture.2017.10.024
Malinovskyi O., Vesely L., Blecha M., Kristan J., Policar T. (2018). The Substrate Selection and Spawning Behaviour of Pikeperch Sander Lucioperca L. Broodstock Under Pond Conditions. Aquaculture Res. 49, 3541–3547. doi: 10.1111/are.13819
Maraux S., Louvard D., Barath J. (1973). The Aminopeptidase From Hog Intestinal Brush Border. Biochim. Biophys. Acta (BBA)-Enzymology 321 (1), 282–295. doi: 10.1016/0005-2744(73)90083-1
Martin G. G., Martin A., Tsai W., Hafner J. C. (2011). Production of Digestive Enzymes Along the Gut of the Giant Keyhole Limpet Megathura Crenulata (Mollusca: Vetigastropoda). Comp. Biochem. Physiol. a-Molecular Integr. Physiol. 160, 365–373. doi: 10.1016/j.cbpa.2011.07.003
Miller G. L. (1959). Use of Dinitrosalicylic Acid Reagent for Determination of Reducing Sugar. Anal. Chem. 31 (3), 426–428.
Mzengereza K., Ishikawa M., Koshio S., Yokoyama S., Yukun Z., Shadrack R. S., et al. (2021). Growth Performance, Growth-Related Genes, Digestibility, Digestive Enzyme Activity, Immune and Stress Responses of De Novo Camelina Meal in Diets of Red Seabream (Pagrus Major). Animals 11. doi: 10.3390/ani11113118
Naas K., Harboe T. (1992). Enhanced First Feeding of Halibut Larvae (Hippoglossus Hippoglossus L.) in Green Water. Aquaculture 105, 143–156. doi: 10.1016/0044-8486(92)90126-6
Navarro N., Sarasquete C. (1998). Use of Freeze-Dried Microalgae for Rearing Gilthead Seabream, Sparus Aurata, Larvae: I. Growth, Histology and Water Quality. Aquaculture 167, 179–193. doi: 10.1016/S0044-8486(98)00311-1
Nolting M., Ueberschar B., Rosenthal H. (1999). Trypsin Activity and Physiological Aspects in Larval Rearing of European Sea Bass (Dicentrarchus Labrax) Using Live Prey and Compound Diets. J. Appl. Ichthyology-Zeitschrift Fur Angewandte Ichthyologie 15, 138–142. doi: 10.1046/j.1439-0426.1999.00138.x
Okamura Y., Miyanishi H., Kono T., Sakai M., Hikima J.-I. (2021). Identification and Expression of Phospholipase A2 Genes Related to Transcriptional Control in the Interleukin-17A/F1 Pathway in the Intestines of Japanese Medaka Oryzias Latipes. Fish Shellfish Immunol. Rep. 2, 100028. doi: 10.1016/j.fsirep.2021.100028
Palinska-Zarska K., Wozny M., Kamaszewski M., Szudrowicz H., Brzuzan P., Zarski D. (2020). Domestication Process Modifies Digestion Ability in Larvae of Eurasian Perch (Perca Fluviatilis), A Freshwater Teleostei (Vol 10, 2211, 2020). Sci. Rep. 10. doi: 10.1038/s41598-020-62689-2
Penka T., Malinovskyi O., Kristan J., Imentai A., Policar T. (2021). Effect of Density and Mixed Culture of Largemouth Bass (Micropterus Salmoides) and Pikeperch (Sander Lucioperca) on Growth, Survival and Feed Conversion Rate in Intensive Culture. Czech J. Anim. Sci. 66, 428–440. doi: 10.17221/59/2021-Cjas
Peres A., Infante J. L. Z., Cahu C. (1998). Dietary Regulation of Activities and mRNA Levels of Trypsin and Amylase in Sea Bass (Dicentrarchus Labrax) Larvae. Fish Physiol. Biochem. 19, 145–152. doi: 10.1023/A:1007775501340
Pfaffl M. W. (2001). A New Mathematical Model for Relative Quantification in Real-time RT–PCR. Nucleic Acids Res. 29 (9), e45.
Policar T., Blecha M., Krist'an J., Mraz J., Velisek J., Stara A., et al. (2016). Comparison of Production Efficiency and Quality of Differently Cultured Pikeperch (Sander Lucioperca L.) Juveniles as a Valuable Product for Ongrowing Culture. Aquaculture Int. 24, 1607–1626. doi: 10.1007/s10499-016-0050-9
Policar T., Schaefer F. J., Panana E., Meyer S., Teerlinck S., Toner D., et al. (2019). Recent Progress in European Percid Fish Culture Production Technology—Tackling Bottlenecks. Aquaculture Int. 27, 1151–1174. doi: 10.1007/s10499-019-00433-y
Policar T., Stejskal V., Kristan J., Podhorec P., Svinger V., Blaha M. (2013). The Effect of Fish Size and Stocking Density on the Weaning Success of Pond-Cultured Pikeperch Sander Lucioperca L. Juveniles. Aquaculture Int. 21, 869–882. doi: 10.1007/s10499-012-9563-z
R Core Team (2014). A Language and Environment for Statistical Computing (Vienna: Austria: Retrieved from R Foundation for Statistical Computing). Available at: http://www.Rproject.org.
Rønnestad I., Conceição L. E. (2005). Aspects of Protein and Amino Acids Digestion and Utilization by Marine Fish Larvae. Physiol. Ecol. Adaptations to Feeding Vertebrates (Science Publishers: Enfield), 389–416.
Ribeiro L., Zambonino-Infante J. L., Cahu C., Dinis M. T. (1999). Development of Digestive Enzymes in Larvae of Solea Senegalensis, Kaup 1858. Aquaculture 179, 465–473. doi: 10.1016/S0044-8486(99)00180-5
Ribeiro L., Zambonino-Infante J. L., Cahu C., Dinis M. T. (2002). Digestive Enzymes Profile of Solea Senegalensis Post Larvae Fed Artemia and a Compound Diet. Fish Physiol. Biochem. 27, 61–69. doi: 10.1023/B:Fish.0000021817.98363.47
Rotllant G., Moyano F. J., Andrés M., Díaz M., Estévez A., Gisbert E. (2008). Evaluation of Fluorogenic Substrates in the Assessment of Digestive Enzymes in a Decapod Crustacean Maja Brachydactyla Larvae. Aquaculture 282 (1-4), 90–96.
Schulz C., Bohm M., Wirth M., Rennert B. (2007). Effect of Dietary Protein on Growth, Feed Conversion, Body Composition and Survival of Pike Perch Fingerlings (Sander Lucioperca). Aquaculture Nutr. 13, 373–380. doi: 10.1111/j.1365-2095.2007.00487.x
Silveira J., Silva C. P., Cargnin-Ferreira E., Alexandre D., Elias M. A., Fracalossi D. M. (2013). Freshwater Catfish Jundia (Rhamdia Quelen) Larvae are Prepared to Digest Inert Feed at the Exogenous Feeding Onset: Physiological and Histological Assessments. Fish Physiol. Biochem. 39, 1581–1590. doi: 10.1007/s10695-013-9810-x
Skjermo J., Vadstein O. (2020). “The Effect of Microalgae on Skin and Gut Bacterial Flora of Halibut Larvae”, In Fish Farming Technology (CRC Press: London), 61–67.
Srichanun M., Tantikitti C., Utarabhand P., Kortner T. M. (2013). Gene Expression and Activity of Digestive Enzymes During the Larval Development of Asian Seabass (Lates Calcarifer). Comp. Biochem. Physiol. B-Biochemistry Mol. Biol. 165, 1–9. doi: 10.1016/j.cbpb.2013.02.005
Srivastava A. S., Kurokawa T., Suzuki T. (2002). mRNA Expression of Pancreatic Enzyme Precursors and Estimation of Protein Digestibility in First Feeding Larvae of the Japanese Flounder, Paralichthys Olivaceus. Comp. Biochem. Physiol. a-Molecular Integr. Physiol. 132, 629–635. doi: 10.1016/S1095-6433(02)00107-1
Tseng H., Grendell J., Rothman S. (1982). Food, Duodenal Extracts, and Enzyme Secretion by the Pancreas. Am. J. Physiology-Gastrointestinal Liver Physiol. 243, G304–G312. doi: 10.1152/ajpgi.1982.243.4.G304
Vaneechoutte M., Verschraegen G., Claeys G., Flamen P. (1988). Rapid Identification of Branhamella-Catarrhalis with 4-Methylumbelliferyl Butyrate. J. Clin. Microbiol. 26, 1227–1228. doi: 10.1128/Jcm.26.6.1227-1228.1988
Wang C. F., Xie S. Q., Zhu X. M., Wu L., Yang Y. X., Liu H. K. (2006). Effects of Age and Dietary Protein Level on Digestive Enzyme Activity and Gene Expression of Pelteobagrus Fulvidraco Larvae. Aquaculture 254, 554–562. doi: 10.1016/j.aquaculture.2005.11.036
Yanes-Roca C., Holzer A., Mraz J., Veselý L., Malinovskyi O., Policar T. (2020a). Improvements on Live Feed Enrichments for Pikeperch (Sander Lucioperca) Larval Culture. Animals 10, 401. doi: 10.3390/ani10030401
Yanes-Roca C., Leclercq E., Vesely L., Malinovskyi O., Policar T. (2020b). Use of Lactic Acid Bacteria During Pikeperch (Sander Lucioperca) Larval Rearing. Microorganisms 8, 238. doi: 10.3390/microorganisms8020238
Yanes-Roca C., Mraz J., Born-Torrijos A., Holzer A. S., Imentai A., Policar T. (2018). Introduction of Rotifers (Brachionus Plicatilis) During Pikeperch First Feeding. Aquaculture 497, 260–268. doi: 10.1016/j.aquaculture.2018.08.004
Yufera M., Darias M. J. (2007). The Onset of Exogenous Feeding in Marine Fish Larvae. Aquaculture 268, 53–63. doi: 10.1016/j.aquaculture.2007.04.050
Yúfera M., Moyano F. J., Martínez-Rodríguez G. (2018). “The Digestive Function in Developing Fish Larvae and Fry. From Molecular Gene Expression to Enzymatic Activity”, In Emerging Issues in Fish Larvae Research (Springer: Cham), 51–86.
Keywords: Artemia, digestion, feeding protocol, euryhaline rotifer, pikeperch larvae
Citation: Imentai A, Gilannejad N, Martínez-Rodríguez G, López FJM, Martínez FP, Pěnka T, Dzyuba V, Dadras H and Policar T (2022) Effects of First Feeding Regime on Gene Expression and Enzyme Activity in Pikeperch (Sander lucioperca) Larvae. Front. Mar. Sci. 9:864536. doi: 10.3389/fmars.2022.864536
Received: 28 January 2022; Accepted: 11 March 2022;
Published: 01 April 2022.
Edited by:
Benjamin Costas, University of Porto, PortugalReviewed by:
Mansour Torfi Mozanzadeh, South Iran Aquaculture Research Center, IranRafael Martinez-garcia, Universidad Juárez Autónoma de Tabasco, Mexico
Copyright © 2022 Imentai, Gilannejad, Martínez-Rodríguez, López, Martínez, Pěnka, Dzyuba, Dadras and Policar. This is an open-access article distributed under the terms of the Creative Commons Attribution License (CC BY). The use, distribution or reproduction in other forums is permitted, provided the original author(s) and the copyright owner(s) are credited and that the original publication in this journal is cited, in accordance with accepted academic practice. No use, distribution or reproduction is permitted which does not comply with these terms.
*Correspondence: Aiman Imentai, YWltZW50YWlAZnJvdi5qY3UuY3o=