- 1Key Laboratory for Sustainable Development of Marine Fisheries, Ministry of Agriculture/Yellow Sea Fisheries Research Institute, Chinese Academy of Fishery Sciences, Qingdao, China
- 2Function Laboratory for Marine Fisheries Science and Food Production Processes, Qingdao National Laboratory for Marine Science and Technology, Qingdao, China
- 3Key Laboratory of Biofuels, Shandong Provincial Key Laboratory of Energy Genetics, Qingdao Institute of Bioenergy and Bioprocess Technology, Chinese Academy of Sciences, Qingdao, China
Shellfish and macro-algae integrated multi-trophic aquaculture (IMTA) contribute greatly to the sustainability of aquaculture. However, the effects of large-scale shellfish and macro-algae aquaculture on the functions of the ocean carbon sink are not clear. To clarify these effects, we studied the spatial and temporal changes of inorganic and organic carbon systems in seawater under different aquaculture modes (monoculture or polyculture of shellfish and macro-algae) in Sanggou Bay, together with the variation of other environmental factors. The results show that the summertime dissolved oxygen (DO) concentration in the shellfish culture zone was significantly lower than other zones (p < 0.05), with a minimum value of 7.07 ± 0.25 mg/L. The variation of pH and total alkalinity (TA) were large across different culture modes, and the seawater in the shellfish culture zone had the lowest pH and TA than the other zones. Seasonal environment and aquaculture modes significantly affected the variation of dissolved inorganic carbon (DIC), CO2 partial pressure (pCO2), dissolved organic carbon (DOC), and particulate organic carbon (POC) concentrations. The highest values of DIC, pCO2, and POC appeared in summer, and the lowest appeared in winter. For DOC concentration, the lowest value appeared in autumn. Spatially, DIC and pCO2 were highest in the shellfish culture zone and lowest in the macro-algae culture zone, DOC was highest in the macro-algae culture zone and lowest in the shellfish culture zone, and POC was lower in the shellfish culture zone and macro-algae culture zone and higher in the remaining zones. The results of sea–air CO2 fluxes showed that except for the shellfish culture zone during summertime, which released CO2 to the atmosphere, all culture zones were the sinks of atmospheric CO2 during the culture period, with the whole bay being a strong CO2 sink during autumn and winter. In summary, large-scale shellfish–macro-algae IMTA plays an important role in the local carbon cycle and contributes to mitigating ocean acidification and hypoxia.
Introduction
Anthropogenic CO2 emissions have negative impacts on the environment, and the climate change caused by these emissions may be the most important global environmental problem currently facing mankind (Jiang et al., 2015). If there is no change in the status quo, longer heatwaves and more frequent extreme precipitation events are expected to occur (Petrović and Lobanov, 2020). The ocean is the largest sink of anthropogenic CO2 (Sabine et al., 2004), as it absorbs approximately 30% of anthropogenically released CO2 (Gruber et al., 2019). The total amount of CO2 emitted by human activities in 2018 was approximately 11.5 ± 0.9 GtC, and approximately 2.6 ± 0.6 GtC was absorbed by the ocean (Friedlingstein et al., 2019). Consequently, the carbon sink function of the marine ecosystem has received increased attention (Jiang et al., 2015).
The absorption of CO2 in the atmosphere by the ocean results in an increase in CO2 on the surface of seawater, which disrupts the balance of the original carbon system and results in ocean acidification (Gattuso et al., 2018), and this affects the survival of calcified organisms and the sensory abilities of fish (Bignami et al., 2013). The large-scale aquaculture, especially seaweed farming, can alter the ability of the marine ecosystem to absorb CO2 and alleviate ocean acidification at the local scale (Gattuso et al., 2018). Macro-algae can absorb excess CO2 through photosynthesis and increase the pH of nearby water through physiological processes (Krause-Jensen et al., 2016; Koweek et al., 2017). During their growth stage, they also release a large amount of dissolved organic carbon (DOC), and this DOC can enter the food web or form refractory dissolved organic carbon (RDOC), thus remaining in the seawater for a long time and eventually resulting in carbon sequestration (Zhang et al., 2017; Li et al., 2018). Large-scale seaweed farming can strengthen the ocean carbon sink function and prevent ocean acidification (Duarte et al., 2017; Xiao et al., 2021). However, a large-scale macro-algae culture can also result in the excessive consumption of dissolved inorganic carbon (DIC), nitrogen, phosphorus, and other nutrients (Zou et al., 2004).
Integrated multi-trophic aquaculture (IMTA) is an effective approach for overcoming the problems associated with the excessive consumption of nutrients in macro-algae culture and increasing the sustainability of macro-algae aquaculture (Barrington et al., 2010). The shellfish–macro-algae IMTA model has been highly successful and is the most economically feasible solution for recovering nutrients in the open water (Fang et al., 2020). The nutrients released by shellfish can be fully absorbed and utilized by macro-algae. O2 and debris produced by macro-algae and the increased water pH promote the survival and growth of shellfish. Shellfish–macro-algae aquaculture is thus an economically efficient and environmentally friendly form of aquaculture (Tang et al., 2011). There is growing evidence that the CaCO3 shells generated during the calcification process of shellfish growth can remove carbon from the coastal ecosystem, thus improving the carbon absorption capacity of the shelf-edge sea, and significantly affect the carbon cycle of coastal ecosystems (Tang et al., 2011; Han et al., 2020). However, the impact of the CO2 released during shellfish respiration and calcification on the carbon sink function of the marine ecosystem remains controversial. There have been reports that pure shellfish culture may be an important additional source of CO2 in seawater and disrupt the balance of the local carbonate system, which affects the carbon sink capacity of marine ecosystems (Han et al., 2017).
The ocean is the largest carbon pool in the world; it thus plays an important role in regulating global climate change. However, large-scale aquaculture methods can vary in their effects on the carbon sink function of marine ecosystems (Jiang et al., 2013; Han et al., 2020). The exchange of CO2 at the sea–air interface is a key ocean carbon cycle process, and its exchange flux is essential for understanding the transfer of CO2 in seawater, as well as the source and sink effects of the marine ecosystem on atmospheric CO2 (Li et al., 2005). Most current studies on CO2 flux have focused on the ocean or macro-algae culture (Jiang et al., 2013; Xiao et al., 2021); research on the effects of the shellfish–macro-algae aquaculture mode on CO2 exchange flux and the carbon cycle needs further study (Li et al., 2021). Additionally, because the concentration of DIC in seawater is higher than that of particulate organic carbon (POC) and DOC (Eglinton and Repeta, 2003), most studies of the role of shellfish–macro-algae aquaculture in the carbon cycle have focused on the inorganic carbon cycle; by contrast, few studies have examined the effect of shellfish–macro-algae aquaculture on the organic carbon cycle (Jiang et al., 2013; Jiang et al., 2015; Fang and Jiang, 2021; Li et al., 2021). The inorganic carbon pump only mediates the migration of CO2 from the atmospheric carbon pool to the ocean carbon pool, and the CO2 that enters the ocean must be stored by the biological pump to achieve long-term storage (Honda, 2003). Therefore, the organic carbon cycle also plays an important role in the marine biological carbon sink, so attention should also be paid to the cycling changes of organic carbon in the breeding process (Liu et al., 2021). Nevertheless, there are few reports on overall effects of aquaculture on inorganic and organic carbon cycles.
In this study, seasonal changes in the inorganic and organic carbon cycles in the kelp aquaculture zone, shellfish culture zone, and shellfish–macro-algae polyculture zone; the CO2 flux of different aquaculture modes in different seasons; and the effects of large-scale aquaculture on the carbon sink and water environment of the marine ecosystem were examined in Sanggou Bay, which is a typical shellfish–macro-algae IMTA area in northern China. The results of this study provide new insights that aid our understanding of the role of the shellfish–macro-algae aquaculture in the marine carbon cycle and could be used to optimize the marine carbon sink function and the sustainability of aquaculture.
Materials and methods
Study Area and Sampling Stations
Located on the east side of Shandong Peninsula in eastern China (37°01′~37°09′ N, 122°24′~122°35′ E), Sanggou Bay covers an area of approximately 144 km2 and has an average water depth of 7.5 m (Zhang et al., 2009). Kelp (Saccharina japonica), Pacific oysters (Crassostrea gigas), and scallops (Chlamys farreri) are the main marine species produced in this region (Li et al., 2021). Currently, Sanggou Bay consists of a shellfish culture zone (inside the bay), macro-algae culture zone (outside the bay), and shellfish–macro-algae polyculture zone (in the middle of the bay) (Figure 1). The culture cycle of kelp is from autumn to the following summer (harvest usually begins in May and is completed by early July), and the culture cycle of shellfish is divided into one or two years depending on the actual situation, with seedlings sown in spring and harvested in autumn each year.
Four on-site surveys were conducted in Sanggou Bay in April (spring), July (summer), October (autumn), and January (winter) of 2019. Each survey was conducted on a survey ship, and two sections were surveyed at the same time in the direction of the coastal current at peak tide. The survey stations are shown in Figure 1; each aquaculture zone contained four stations, and the control area outside the bay also included four stations.
Analysis Method
A water quality analyzer (EXO2, YSI, Yellow Spring, OH, USA) was used on-site to measure the dissolved oxygen (DO), water temperature, pH, salinity, and other indicators. CO2 partial pressure (pCO2) on the surface water was measured with a CO2 partial pressure meter (OceanPack; Csubctech, Kiel, Germany). Water samples were collected with a water sample collector (KC, Silkeborg, Denmark). The Whatman GF/F membrane and cellulose acetate filter membrane were used for suction filtration on-site, and 200 and 1,000 ml surface seawater samples were filtered. After the Whatman GF/F membrane was acidified, the POC in the water was measured with an elemental analyzer (EL; Elementar, Langenselbold, Germany). The filtrate was transferred into a 30 ml jar that had been burned at 450°C for 4 h in advance, and an appropriate amount of saturated HgCl2 solution was added with a syringe to inhibit microbial activity. The jar was then tightly capped and sealed with a sealing film, and the DIC and DOC were determined by a total organic carbon analyzer (Multi N/C; Jena, Jena, Germany). The cellulose acetate filters and water samples after suction filtration were stored in a refrigerator at 4°C for the analysis of chlorophyll (Chl-a) and total alkalinity (TA). The fluorescence method was used to determine the Chl-a concentration. TA was measured using an automatic potentiometric titration method (848 Titrino Plus; Metrohm, Herisau, Switzerland) with a measurement accuracy of ± 5 mmol/L. All samples collected were processed the same day and stored in a low-temperature freezer. The samples that required instrumental analyses were analyzed within a week of collection.
The sea–air interface CO2 exchange flux was estimated using the following formula: F=k×αs×ΔpCO2, where F (mmol/m2·d) is the CO2 exchange flux at the sea–air interface, which represents the CO2 exchange flux (the intensity of the atmospheric CO2 source and sink). The positive and negative signs indicate the direction of the source and sink. When seawater absorbs CO2 from the atmosphere and acts as a sink, F is a negative value. When seawater releases CO2 into the atmosphere and acts as a source, F is a positive value. k (cm/h) is the gas transmission speed at the sea–air interface; αs (mol/kg·atm) is the solubility coefficient of CO2 in seawater, which is a function related to temperature and salinity and is calculated using the Weiss (1974) formula; and ΔpCO2 is the difference between seawater and pCO2 in the atmosphere. In this study, the value of pCO2 in the atmosphere was 410 µatm (https://keelingcurve.ucsd.edu/), and the gas transmission speed (k) at the sea–air interface was the average value (10.3) of the continental shelf sea area (Jiang et al., 2013).
Data Analysis
Statistical analysis was performed using SPSS 17.0. Analysis of variance (ANOVA) was used to analyze the effects of season and zone on environmental factors, carbon components, pCO2, and sea–air CO2 flux. According to the results of the homogeneity test, Tukey’s honestly significant difference or Tamhane’s T2 test was used to evaluate the significance of differences between groups (p <0.05) after ANOVA.
Results
Spatiotemporal Variability in Environmental Parameters
Table 1 shows the averages for the environmental factors in the study area. The water temperature decreased from the inside of the bay to the outside of the bay in spring and summer, while the opposite was true in autumn and winter. The salinity in the summer was low because of the higher rainfall and land runoff, and salinity in the entire bay in the other seasons did not significantly vary (p>0.05). The season (F=5.10, p<0.05) and aquaculture zone (F=4.59, p<0.05) significantly affected the DO concentration of surface seawater, and the interaction between the two on the DO concentration was significant (seasonal × zone: F=8.32, p<0.05). The Chl-a concentration showed pronounced seasonal variation (F=14.59, p<0.05). Although the Chl-a concentration of the shellfish culture zone appeared to be lower than the other aquaculture zones, there was no significant effect of the aquaculture zone on the Chl-a concentration (F=2.16, p=0.136). The aquaculture mode significantly (F=11.79, p<0.05) affected the pH. The pH was lowest in the shellfish culture zone and highest in the macro-algae culture zone. The aquaculture mode (F=6.32, p<0.05) had a significant impact on TA, and the TA was significantly lower in the shellfish culture zone than in the other regions. Additionally, the season (F=257.33, p<0.05) had a significant effect on changes in TA. TA decreases with temperature increases.
Spatiotemporal Variability in Dissolved Inorganic Carbon and pCO2
The season and aquaculture mode significantly affected the spatiotemporal changes in DIC (season: F=325.75, p<0.05; zone: F=7.18, p<0.05; Figure 2) and pCO2 (season: F=38.97, p< 0.05; zone: F=11.79, p<0.05; Figure 3) in the surface seawater of Sanggou Bay, and the interaction between the season and aquaculture mode also had a significant effect on DIC and pCO2 (DIC: season × zone: F= 9.75, p<0.05; pCO2: season × zone: F=4.39, p<0. 05). DIC in the surface seawater of Sanggou Bay was highest in summer, followed by autumn, spring, and winter; pCO2 was highest in summer, followed by spring, autumn, and winter. The surface seawater DIC concentration and pCO2 were highest in the shellfish culture zone and lowest in the macro-algae culture zone. The pCO2 concentration in the control area did not significantly differ from that in the shellfish–macro-algae polyculture zone (p>0.05). In sum, the pCO2 level in the whole bay increased with temperature.
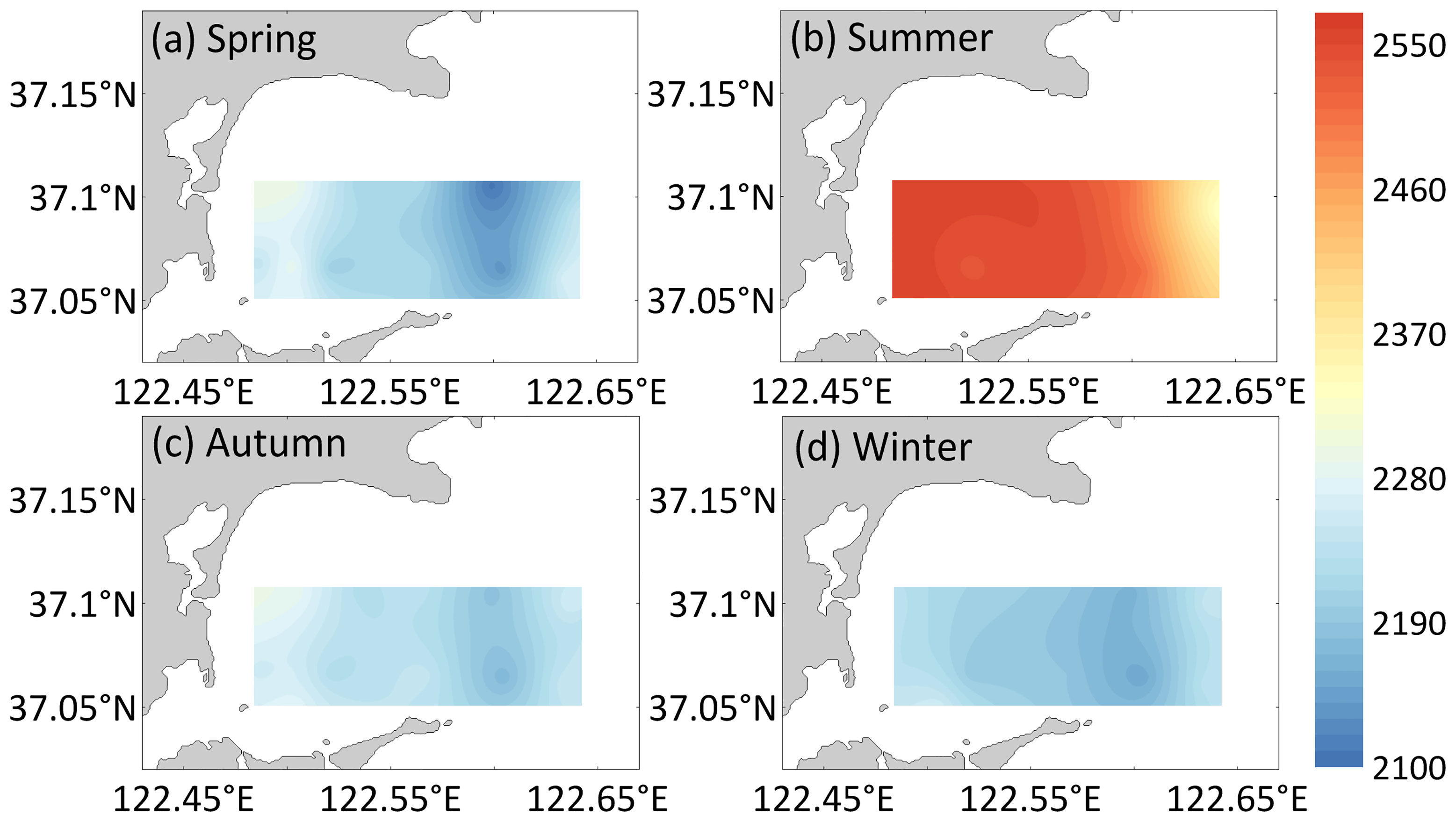
Figure 2 Horizontal distribution of DIC (μmol/L) for the (A) spring, (B) summer, (C) autumn, and (D) winter surface water in Sanggou Bay.
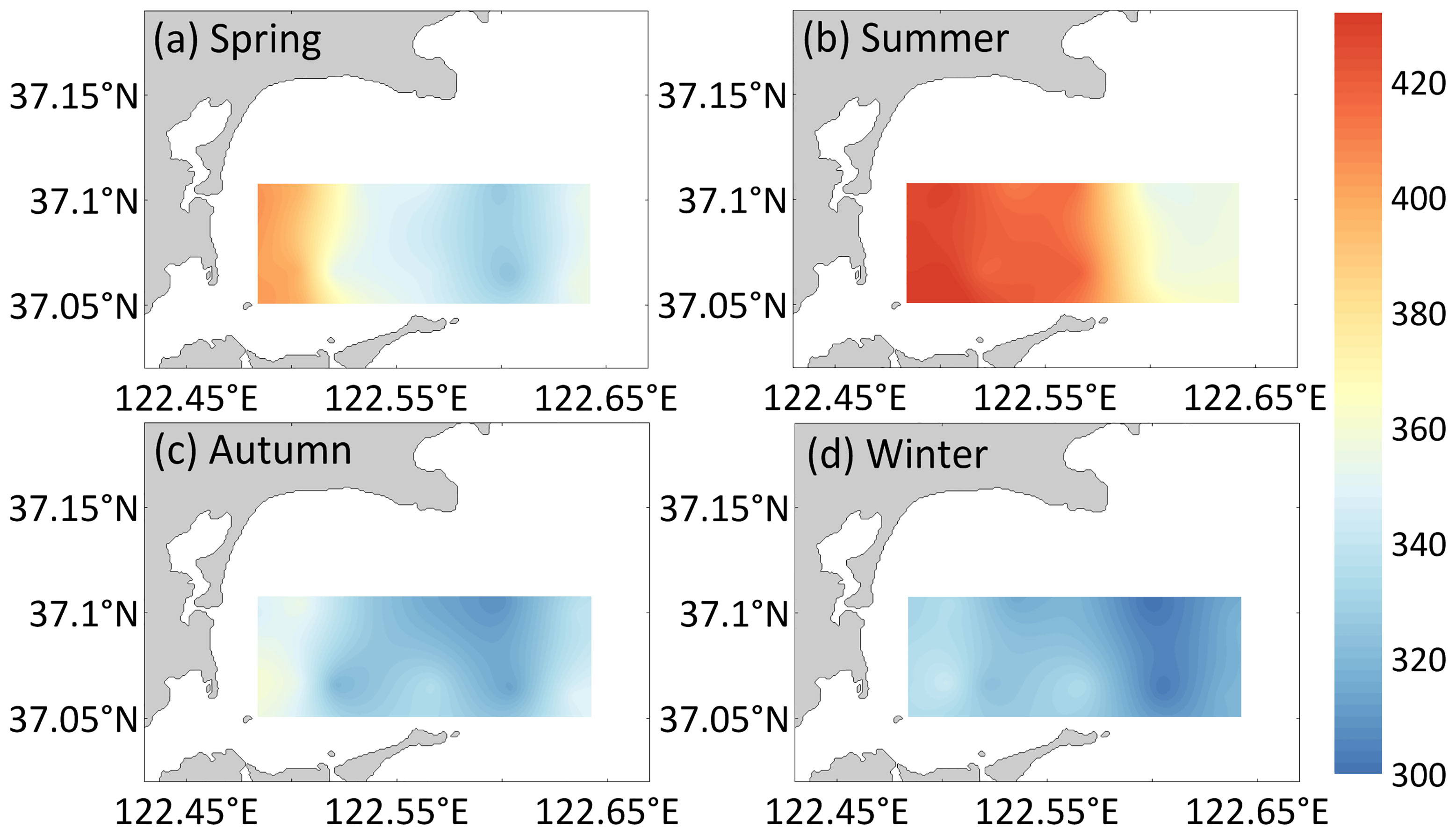
Figure 3 Horizontal distribution of pCO2 (μatm) for the (A) spring, (B) summer, (C) autumn, and (D) winter surface water in Sanggou Bay.
Spatiotemporal Variability of Dissolved Organic Carbon and Particulate Organic Carbon
Similar to the change law of DIC and pCO2, different seasons and aquaculture modes also significantly affect the distributions of DOC (season: F=35.88, p< 0. 05, zone: F=4.32, p<0. 05, Figure 4) and POC (season: F=11.23, p< 0. 05, zone: F=4.59, p<0. 05, Figure 5) in the surface seawater of Sanggou Bay. The season and aquaculture mode have a significant interaction effect on DOC (season × zone: F=8.07, p<0. 05) and POC (season × zone: F=8.07, p<0. 05). Different from DIC and pCO2, the DOC concentration in winter and summer is relatively high, the DOC concentration in spring and autumn is relatively low, and the autumn DOC concentration is the lowest. From a spatial point of view, the overall performance of different aquaculture zones is that the DOC concentration in the macro-algae culture zone is the highest, and the DOC concentration in the shellfish culture zone is the lowest. Nevertheless, the DOC concentration in the shellfish culture zone in summer was significantly higher than those in other areas (p<0.05). The POC concentration in the surface seawater of Sanggou Bay follows the sequence of summer > spring > autumn > winter. From the perspective of spatial changes, the POC concentration in the shellfish culture zone and macro-algae culture zone surface seawater is lower, and the POC concentration in the shellfish–macro-algae polyculture zone and control area is higher.
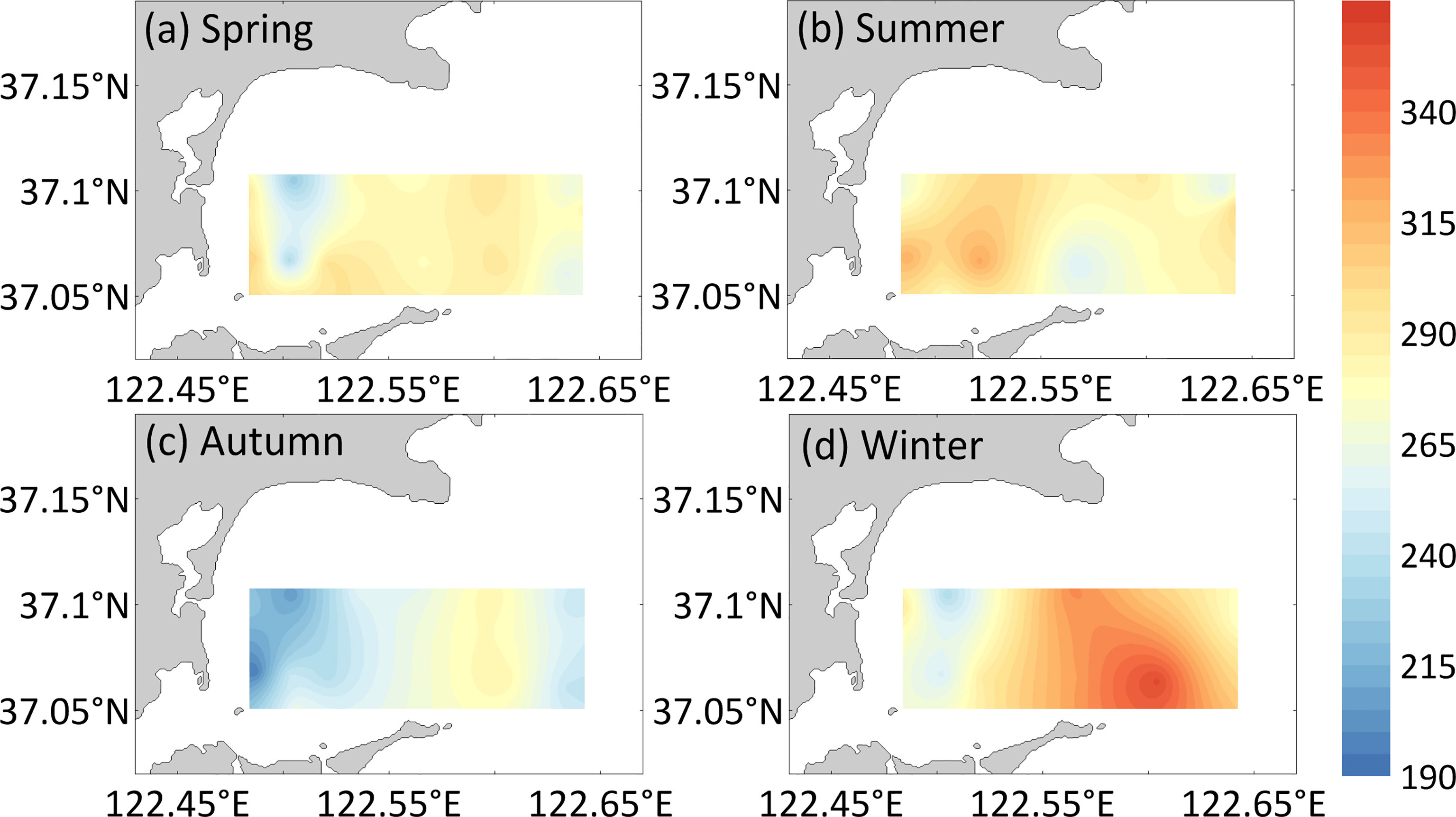
Figure 4 Horizontal distribution of DOC (μmol/L) for the (A) spring, (B) summer, (C) autumn, and (D) winter surface water in Sanggou Bay.
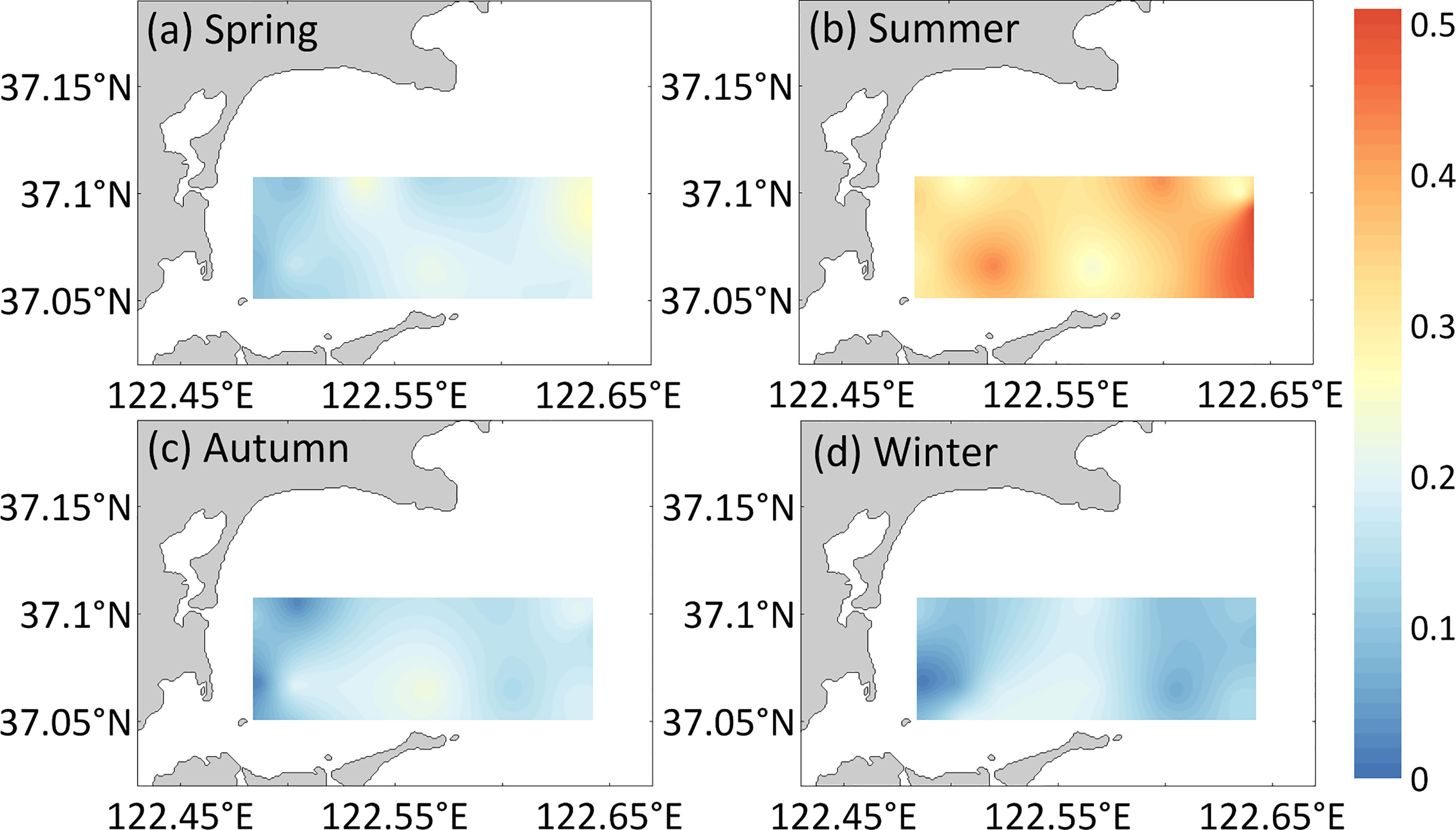
Figure 5 Horizontal distribution of POC (mg/L) for the (A) spring, (B) summer, (C) autumn, (D) winter surface water in Sanggou Bay.
Spatiotemporal Variability in Sea–Air CO2 Flux
Figure 6 shows the annual sea–air CO2 flux in Sanggou Bay. In spring, the shellfish culture zone was a weak sink of atmospheric CO2. In summer, the shellfish culture zone and shellfish–macro-algae polyculture zone were the sources of atmospheric CO2. In autumn and winter, the entire bay was a strong sink of atmospheric CO2, and the macro-algae culture zone played the largest role in gathering atmospheric CO2. The season (F=210.43, p<0.05) and aquaculture mode (F=44.14, p<0.05) had significant effects on the CO2 flux, and the interaction between the two was significant (season × zone: F=7.396, p<0.05). The sea–air CO2 flux in Sanggou Bay varies differently between seasons, where a significant difference was concluded between spring and winter and between summer and autumn, and a highly significant difference was found between summer and winter. (Table 2). Pearson correlation analysis revealed that changes in the CO2 exchange flux at the sea–air interface in different seasons were affected by different environmental factors (Table 3). DIC and Chl-a were the most important factors in spring. In autumn and winter, pH and DIC were the most important factors. In summer, the sea–air CO2 flux was most significantly affected by Chl-a, TA, and DO. Throughout the year, water temperature and DIC were the key factors affecting sea–air CO2 exchange flux.
Discussion
Effects of Shellfish–Macro-Algae Aquaculture on Environmental Factors
Macro-algae can absorb DIC in seawater through photosynthesis, reduce pCO2 levels, and increase the DO and pH of seawater. By contrast, the respiration and calcification of shellfish result in the release of CO2 into seawater, increase DIC and pCO2, and reduce the pH (Delille et al., 2009). A macro-algae culture can effectively alleviate ocean acidification (Xiao et al., 2021). Calcified organisms (e.g., bivalves, corals) that are particularly susceptible to ocean acidification can benefit from these effects (Kroeker et al., 2013). Thus, the co-cultivation of bivalves and seaweeds in the IMTA system can protect bivalves from the effects of ocean acidification and provide them with sufficient oxygen. The IMTA system can also alleviate the pressure associated with the release of nutrients by bivalves into the environment, as the macro-algae in the system absorb nutrients (Han et al., 2020). It is important to note that the shellfish–macro-algae IMTA model in the survey area has been based on the expansion of kelp culture. Because of the poor high-temperature resistance of kelp, large-scale shellfish farming is carried out for up to 4 months in the study area after kelp is harvested in summer. According to previous studies, the metabolism of shellfish is highest in July and August, which corresponds to the period when shellfish grow most vigorously. In addition, bacteria are most active under high-temperature conditions in summer, and the excrement of cultured shellfish is quickly decomposed, which increases the vulnerability of the shellfish culture zone to hypoxia and eutrophication (Li et al., 2018). Therefore, the large-scale shellfish culture in summer may impose substantial ecological pressures (Liu et al., 2021), and this was confirmed in this study. The pH and DO of the shellfish culture zone and shellfish–macro-algae polyculture zone (actually, only shellfish is cultured in the polyculture zone) decreased to varying degrees in summer; the average pH of the shellfish culture zone was only 7.73, and the DO was 7.07 mg/L. The calcification rate of shellfish decreased by 13.5% when the pH decreased by 0.1 units, and shellfish are not able to synthesize shells when the pH is 7.3 (Zhang et al., 2011; Wahl et al., 2018). The growth of fish is also inhibited when the DO decreases to 5 mg/L, and the large-scale death of crustaceans may occur when DO decreases to 4 mg/L (Sugden, 2017). Therefore, changes in environmental factors associated with the aquaculture mode of Sanggou Bay in summer pose risks to cultured organisms such as shellfish and fish. The pH and DO of the shellfish culture zone in summer were 0.12-0.39 units and 0.94-1.06 mg/L lower compared with values from surrounding areas, respectively. The pH and DO in the macro-algae culture zone were 0.10-0.34 units and 0.03-0.53 mg/L higher compared with values in adjacent seas, respectively. Therefore, macro-algae culture can theoretically offset the impact of CO2 produced by shellfish respiration and calcification on the pH and DO of the sea. Because of the high temperature of water in summer, kelp cannot grow. Thus, large macro-algae that can withstand high temperatures (e.g., Gracilaria lemaneiformis) needs to be bred to reduce the environmental impact of summer shellfish farming (Liu et al., 2021).
In other seasons, the environmental parameters tend to better because of the cultivation of macro-algae in the study area. Macro-algae culture can not only improve the environmental parameters but can also absorb the nutrients in water, which alleviates eutrophication (Mao et al., 2009). Previous studies have confirmed that the buffering effect of macro-algae culture is sufficient to partially offset the decrease in pH caused by ocean acidification in recent decades. Compared with natural seaweed systems, macro-algae culture has a greater potential to adapt to future fluctuations in the ocean pH (Krause-Jensen et al., 2016). However, the water exchange capacity in Sanggou Bay was weak because of the blocking effect of breeding facilities and cultured organisms (Liu et al., 2017); there is thus a need to ensure that the scale of macro-algae culture is not excessive to prevent the excessive consumption of nutrients. In autumn, some of the shellfish are harvested, which causes some nutrients to be removed, and kelp absorbs large amounts of nutrients in the early stages of growth. Therefore, the Chl-a concentration was lowest in autumn, indicating that even if shellfish ingestion is reduced at this time, macro-algae cultivation in the sea prevents the growth of phytoplankton. Therefore, the excessive expansion of macro-algae culture will restrict the growth of macro-algae; an appropriate amount of macro-algae culture is required for aquaculture to be environmentally friendly (Fang et al., 2020).
Effects of Shellfish–Macro-Algae Aquaculture on the Inorganic Carbon Cycle in Seawater
The inorganic carbon system in the aquaculture zone undergoes various changes due to the physiological activities of shellfish and macro-algae. Large-scale macro-algae and bivalve farming has affected the sea–air CO2 exchange flux in Sanggou Bay. The shellfish and macro-algae biomass was highest in the winter, and this is when the sea–air CO2 exchange flux was the highest in the study area. The CO2 exchange flux between the ocean and the atmosphere is the most important process determining the marine inorganic carbon budget (Jiang et al., 2015). Therefore, DIC is an important factor affecting the annual sea–air CO2 exchange flux in Sanggou Bay.
The physiological activities of some calcified organisms in their natural habitats can significantly affect the inorganic carbon cycle of the surrounding water (Page et al., 2019). Changes in the inorganic carbon cycle induced by shellfish culture are usually more pronounced than those in natural habitats because the density of cultured shellfish is much higher than that of naturally occurring species (Li et al., 2021). Longer seawater residence times can also substantially affect changes in the marine inorganic carbon system (Page et al., 2019). The weak water exchange capacity of Sanggou Bay induces major changes in the inorganic carbon cycle in the shellfish culture zone (Li et al., 2021). In all seasons, the lowest pH and TA and highest DIC and pCO2 were observed in the shellfish culture zone. Although the CO2 emitted by shellfish respiration does not affect the TA in seawater (Zhang et al., 2011), the calcification process of shellfish leads to a decrease in seawater TA, which limits the ability of seawater to absorb CO2 and affects the carbonate balance (Han et al., 2020). Bivalves are the main cause of the decline in TA in Sanggou Bay, which may lead to an increase in the pCO2 in the shellfish culture zone. Although bivalves absorb a certain amount of DIC during the calcification of synthetic shells, the DIC released during respiration is significantly higher (Jiang et al., 2015). Therefore, the respiration of a large number of bivalves in the bay may increase the accumulation and emission of inorganic carbon in the water environment (Li et al., 2021). The sedimentation of organisms aggravates the bioburden of sediments, and the remineralization of sediments is an important source of DIC (Jiang et al., 2015). When the bivalves experience high summer temperatures, the DIC concentration in the shellfish culture zone is the highest due to physiological processes such as respiration and excretion. However, some studies have shown that the calcification process of bivalves can regulate the inorganic carbon cycle more than respiration under certain conditions. For example, oysters can remove inorganic carbon from seawater through calcification (Ren, 2014). The respiration of bivalves is weakened by decreases in temperature (e.g., winter), which explains the lack of differences in the concentration of DIC in the shellfish culture zone compared with that of other regions. This might also be one of the reasons why the sources and sinks of the sea–air CO2 flux in the shellfish culture zone change seasonally in this study. The effects of water temperature on the bay sea–air CO2 flux may not be mediated through physical effects, such as solubility, but instead through indirect effects, such as the physiological activities of cultured organisms.
The results regarding the sea–air CO2 flux in the shellfish culture zone in this study differed from those of previous studies. This might be caused by the different methods used for calculating the sea–air CO2 flux, including the gas transmission speed, the pCO2 in the atmosphere, and the pCO2 in the surface water. The acquisition of pCO2 in the surface water is usually automatically obtained by software by monitoring parameters such as pH, temperature, TA, and salinity. Therefore, a small error in a certain parameter may have a substantial impact on the results. Studies of the same aquaculture zone in the same season have found that differences in pCO2 in the surface water were greater than 50 μatm (Li et al., 2021; Xiao et al., 2021). Therefore, pCO2 in the water was directly measured by a CO2 partial pressure meter, albeit some error in the absolute value is possible. The general patterns of pCO2 in this study were relatively clear. Hence, we mainly focused on the different patterns of CO2 flux and pCO2 and did not discuss absolute values.
Macro-algae uses bicarbonate ions as an external carbon source for photosynthesis, ingests a large amount of DIC during growth, and affects the pCO2 and TA in surface seawater (Axelsson et al., 2000). The net primary production of macro-algae aquaculture can promote the absorption of atmospheric CO2, and the difference in the macro-algae growth rate in different seasons significantly affects the level of the sea–air CO2 exchange flux (Jiang et al., 2013; Xiao et al., 2021), which was verified in this study. Compared with the natural seaweed system, a macro-algae aquaculture has more flexibility and scalability in terms of space planning (Xiao et al., 2021). Macro-algae culture also responded positively to increases in CO2, which can increase the productivity of macro-algae culture, result in the absorption of additional CO2 (Krause-Jensen et al., 2016), promote a continuous increase in pH, and provide marine life with a refuge for ocean acidification (Hofmann et al., 2011). Previous studies have shown that the effects of the proliferation of coastal macro-algae on surface seawater pCO2 can last up to 3 months following the end of a water bloom (Gazeau et al., 2005). However, this was not a pattern that was observed in our study. The pCO2 in the surface seawater of the study area increased significantly after macro-algae was harvested. This might be explained by the fact that the continuous impact of macro-algae on natural water cannot offset the effects of shellfish and other cultured organisms.
Additionally, after the macro-algae culture was initiated, the sea–air CO2 flux in Sanggou Bay was significantly improved. Therefore, whether shellfish culture acts as carbon sink or source is greatly dependent on the participation of the macro-algae aquaculture, which may be another reason why the sources and sinks of the sea–air CO2 flux in the shellfish culture zone change seasonally in this study. Furthermore, we speculate that in bays or waters with weak hydrodynamics, the effect of macro-algae culture could potentially increase the water residence time locally, which may affect the biological process regarding the inorganic carbon cycle. The studies of the effect of macro-algae on the inorganic carbon cycle at the mesocosm scale in the laboratory have been carried out, and the effects of macro-algae on the inorganic carbon cycle in Sanggou Bay are weaker than the effects observed in the laboratory (Jiang et al., 2015; Han et al., 2017; Fang et al., 2020; Han et al., 2020; Liu et al., 2021). Characterizing changes in the inorganic carbon system might be difficult if the time that seawater remains in the macro-algae culture zone is relatively short (Li et al., 2021). Therefore, changes in environmental factors such as ocean currents, as well as multiple effects such as the carbonate balance and biological metabolic processes affect changes in the inorganic carbon cycle of the marine ecosystem (Koweek et al., 2017; Wahl et al., 2018).
Effects of Shellfish–Macro-Algae Aquaculture on the Organic Carbon Cycle in Seawater
The concentrations of DOC and POC significantly varied among seasons and regions in the surface seawater of Sanggou Bay. In winter, the biomass of macro-algae farmed in Sanggou Bay was the largest, and the macro-algae released DOC into the water during their growth (Li et al., 2018). Hence, a higher DOC concentration was observed in the macro-algae culture zone in winter. Jiao et al. (2018) suggested that microbial carbon fixation is a major part of the missing carbon sink, as it involves the conversion of labile DOC into RDOC and its storage in seawater for a long time. Zhang et al. (2017) found that the RDOC generated by macro-algae culture is almost equal to the amount of carbon that it uses during its growth. Therefore, macro-algae culture can not only effectively improve the inorganic carbon cycle but can also effectively improve the organic carbon cycle, thereby increasing the strength of the marine carbon sink. The biomass of macro-algae was lowest in Sanggou Bay in summer. However, the DOC concentration in Sanggou Bay was higher in summer than in spring and autumn. This can be explained by the growth of phytoplankton in summer, which results in the release of a large amount of DOC (Mou et al., 2017). The manure produced by shellfish culture in summer also increases the organic carbon pool and DOC (Ning et al., 2016). A large amount of organic debris is produced when kelp is harvested in summer. The decaying organic debris and shellfish feces can release a large amount of DOC into the seawater via decomposition by microorganisms (Mahmood et al., 2017). The DOC concentration was lowest in autumn because the macro-algae were recently cultivated, and the growth of phytoplankton was inhibited by macro-algae. Additionally, some of the shellfish are harvested, and a large amount of carbon is removed from the system in autumn (Li et al., 2018). Therefore, the DOC concentration was lowest in autumn. The DOC concentration in the shellfish culture zone was low in the rest of the seasons except for summer. This suggests that the DOC released by shellfish culture can be quickly reused and thus may not be easily stored in seawater (Zhang et al., 2017; Li et al., 2018).
POC is the main form of carbon solidification and migration output in seawater (Hung et al., 2000). Although it only accounts for approximately 10% of marine organic carbon, the ratio of POC to DOC in marine primary production products is 5:1 (Jiao and Wang, 1994). POC is closely related to life processes and the primary productivity of organisms; it is thus an important material in the food chain of marine organisms. The source of POC in seawater in Sanggou Bay is mainly live phytoplankton, which is the main food source for shellfish (Xia et al., 2013). Therefore, the POC concentration in the shellfish culture zone was low. The shellfish culture can effectively remove POC through filter-feeding activity and generate biological deposits such as feces, which has a substantial impact on carbon biogeochemical processes (Jiang et al., 2015). The POC concentration in the macro-algae culture zone was similarly low because of competition with phytoplankton for nutrients. The POC concentration was higher in the shellfish–macro-algae polyculture zone than in the macro-algae culture zone and shellfish culture zone. On the one hand, this indicates that shellfish–macro-algae IMTA has little effect on the nutrient structure of the sea area and does not inhibit the reproduction of phytoplankton. On the other hand, this suggests that shellfish–macro-algae IMTA can better promote the migration and transformation of particulate carbon.
Overall, DOC and POC play an important role in the marine carbon cycle, and the shellfish and macro-algae in the shellfish–macro-algae aquaculture can affect the organic carbon cycle through their physiological activities. Therefore, the cycle of organic carbon requires consideration in future studies of the carbon sink function of marine ecosystems.
Conclusions
Large-scale aquaculture alters the carbon sink function and carbon cycle of marine ecosystems, and aquaculture modes vary in their effects. The macro-algae culture and shellfish culture have substantial effects on the sea–air CO2 flux in Sanggou Bay and inorganic and organic carbon cycles in water. The macro-algae culture can effectively improve various environmental characteristics, promote the absorption of atmospheric CO2, and provide calcified organisms with refuges from ocean acidification. Although shellfish culture reduces the absorption capacity of seawater for CO2, its efficient filtration capacity can also effectively influence the organic carbon cycle. We believe that the carbon sink function of shellfish culture must be restricted to certain conditions to be realized (e.g., temperature, co-culture of macro-algae). Large-scale shellfish–macro-algae IMTA plays an important role in the promotion of carbon cycling.
Data Availability Statement
The original contributions presented in the study are included in the article/supplementary material. Further inquiries can be directed to the corresponding author.
Author Contributions
Conceptualization, YL and JZ. Methodology, YL. Experience operimental, WW. Sample analysis, WW and JY. Data curation, XW and YZhong. Writing—original draft preparation, YL. Writing—review and editing, YL. Revision process, HL and YZhang. All authors have read and agreed to the published version of the manuscript.
Funding
This research was funded by The National Key R&D Program of China (2020YFA0607603, 2020YFA0607602), Joint Fund of National Natural Science Foundation of China (U1906216), Strategic Priority Research Program of the Chinese Academy of Sciences (XDA23050402), Ministry of agriculture national outstanding agricultural talents and innovative team “shallow aquaculture capacity and healthy aquaculture” and Central Public-interest Scientific Institution Basal Research Fund, YSFRI, CAFS (NO. 20603022022012).
Conflict of Interest
The authors declare that the research was conducted in the absence of any commercial or financial relationships that could be construed as a potential conflict of interest.
Publisher’s Note
All claims expressed in this article are solely those of the authors and do not necessarily represent those of their affiliated organizations, or those of the publisher, the editors and the reviewers. Any product that may be evaluated in this article, or claim that may be made by its manufacturer, is not guaranteed or endorsed by the publisher.
References
Axelsson L., Jesús M., Félix F. (2000). Utilization of HCO3– at High Ph by the Brown Macroalga Laminaria Saccharina. Eur. J. Phycol. 35 (1), 53–59. doi: 10.1080/09670260010001735621
Barrington K., Neil R., Thierry C., Shawn R., Bryn R. (2010). Social Aspects of the Sustainability of Integrated Multi-Trophic Aquaculture. Aquacult. Int. 18 (2), 201–211. doi: 10.1007/s10499-008-9236-0
Bignami S., Enochs I. C., Manzello D. P., Sponaugle S., Cowen R. K. (2013). Ocean Acidification Alters the Otoliths of a Pantropical Fish Species With Implications for Sensory Function. P. Natl. A. Sci. 110 (18), 7366–7370. doi: 10.1073/pnas.1301365110
Delille B., Borges A. V., Delille D. (2009). Influence of Giant Kelp Beds (Macrocystis Pyrifera) on Diel Cycles of Pco2 and DIC in the Sub-Antarctic Coastal Area. Estuar. Coast. Shelf. S 81 (1), 114–122. doi: 10.1016/j.ecss.2008.10.004
Duarte C. M., Wu J., Xiao X., Bruhn A., Krause-Jensen D. (2017). Can Seaweed Farming Play a Role in Climate Change Mitigation and Adaptation? Front. Mar. Sci. 4. doi: 10.3389/fmars.2017.00100
Eglinton T. I., Repeta D. J. (2003). Organic Matter in the Contemporary Ocean. Treat. Geochem. 6, 145–180. doi: 10.1016/B0-08-043751-6/06155-7
Fang J., Fang J., Chen Q., Mao Y., Jiang Z., Du M., et al. (2020). Assessing the Effects of Oyster/Kelp Weight Ratio on Water Column Properties: An Experimental IMTA Study at Sanggou Bay, China. J. Oceanol. Limnol. 38 (6), 1914–1924. doi: 10.1007/s00343-019-9109-6
Friedlingstein P., Jones M. W., O’Sullivan M., Andrew R. M., Hauck J., Peters G. P., et al. (2019). Global Carbon Budget 2019. Earth Syst. Sci. Data. 11 (4), 1783–1838. doi: 10.5194/essd-11-1783-2019
Gattuso J., Magnan A. K., Bopp L., Cheung W., Duarte C. M., Hinkel J., et al. (2018). Ocean Solutions to Address Climate Change and its Effects on Marine Ecosystems. Front. Mar. Sci. 5. doi: 10.3389/fmars.2018.00337
Gazeau F., Duarte C. M., Gattuso J. P., Barrón C., Navarro N., Ruiz S., et al. (2005). Whole-System Metabolism and CO2 Fluxes in a Mediterranean Bay Dominated by Seagrass Beds (Palma Bay, NW Mediterranean). Biogeosciences 2 (1), 43–60. doi: 10.5194/bg-2-43-2005
Gruber N., Clement D., Carter B. R., Feely R. A., Heuven S., Hoppema M., et al. (2019). The Oceanic Sink for Anthropogenic CO2 From 1994 to 2007. Science 363 (6432), 1193–1199. doi: 10.1126/science.aau5153
Han T., Shi R., Qi Z., Huang H., Liang Q., Liu H. (2017). Interactive Effects of Oyster and Seaweed on Seawater Dissolved Inorganic Carbon Systems: Implications for Integrated Multi-Trophic Aquaculture. Aquacult. Env. Interac. 9, 469–478. doi: 10.3354/aei00246
Han T., Shi R., Qi Z., Huang H., Wu F., Gong X. (2020). Biogenic Acidification of Portuguese Oyster Magallana Angulata Mariculture can be Mediated Through Introducing Brown Seaweed Sargassum Hemiphyllum. Aquaculture 520, 734972. doi: 10.1016/j.aquaculture.2020.734972
Hofmann G. E., Smith J. E., Johnson K. S., Send U., Levin L., Micheli F., et al. (2011). High-Frequency Dynamics of Ocean PH: A Multi-Ecosystem Comparison. PloS One 6 (12), e28983. doi: 10.1371/journal.pone.0028983
Honda M. C. (2003). Biological Pumping in Northwestern North Pacific. J. Oceanogr. 59 (5), 671–684. doi: 10.1023/B:JOCE.0000009596.57705.0c
Hung J., Lin P., Liu K. (2000). Dissolved and Particulate Organic Carbon in the Southern East China Sea. Cont. Shelf. Res. 20 (4-5), 545–569. doi: 10.1016/S0278-4343(99)00085-0
Jiang W., Fang J. (2021). Effects of Mussel-Kelp Ratios in Integrated Mariculture on the Carbon Dioxide System in Sanggou Bay. J. Sea. Res. 167, 101983. doi: 10.1016/j.seares.2020.101983
Jiang Z., Fang J., Mao Y., Han T., Wang G. (2013). Influence of Seaweed Aquaculture on Marine Inorganic Carbon Dynamics and Sea–Air CO2 Flux: SEAWEED AQUACULTURE and INORGANIC CARBON DYNAMICS. J. World. Aquacult. Soc 44 (1), 133–140. doi: 10.1111/jwas.12000
Jiang Z., Li J., Qiao X., Wang G., Bian D., Jiang X., et al. (2015). The Budget of Dissolved Inorganic Carbon in the Shellfish and Seaweed Integrated Mariculture Area of Sanggou Bay, Shandong, China. Aquaculture 446, 167–174. doi: 10.1016/j.aquaculture.2014.12.043
Jiao N., Cai R., Zheng Q., Tang K., Liu J., Jiao F., et al. (2018). Unveiling the Enigma of Refractory Carbon in the Ocean. Natl. Sci. Rev. 5 (4), 459–463. doi: 10.1093/nsr/nwy020
Jiao N., Wang R. (1994). Marine Primary Production Photodynamics and Product Structure. Acta Oceanol. Sin. 16 (5), 85–91. (In Chinese with English abstract).
Koweek D. A., Nickols K. J., Leary P. R., Litvin S. Y., Bell T., Luthin T., et al. (2017). A Year in the Life of a Central California Kelp Forest: Physical and Biological Insights Into Biogeochemical Variability. Biogeosciences 14 (1), 31–44. doi: 10.5194/bg-14-31-2017
Krause-Jensen D., Marbà N., Sanz-Martin M., Hendriks I. E., Thyrring J., Carstensen J., et al. (2016). Long Photoperiods Sustain High Ph in Arctic Kelp Forests. Sci. Adv. 2 (12), e1501938. doi: 10.1126/sciadv.1501938
Kroeker K. J., Kordas R. L., Crim R., Hendriks I. E., Ramajo L., Singh G. S., et al. (2013). Impacts of Ocean Acidification on Marine Organisms: Quantifying Sensitivities and Interaction With Warming. Global. Change. Biol. 19 (6), 1884–1896. doi: 10.1111/gcb.12179
Li N., Li X., Song J. (2005). Key Biogeochemistry Processes of Marine Carbon Cycle. Chinese J. Mar. Environ. Sci. 24 (2), 75–80. doi: 10.3969/j.issn.1007-6336.2005.02.021
Liu Y., Wang X., Wu W., Yang J., Wu N., Zhang J. (2021). Experimental Study of the Environmental Effects of Summertime Cocultures of Seaweed Gracilaria Lemaneiformis (Rhodophyta) and Japanese Scallop Patinopecten Yessoensis in Sanggou Bay, China. Fishes 6 (4), 53. doi: 10.3390/fishes6040053
Liu Y., Zhang J., Fang J., Lin F., Wu W. (2017). Analysis of the Air-Sea Surface Carbon Dioxide Flux and Its Interaction With Aquaculture Activities in Sanggou Bay. Prog. Fish. Sci. 38 (6), 1–8. doi: 10.11758/yykxjz.20160331001
Li J., Zhang W., Ding J., Xue S., Huo E., Ma Z., et al. (2021). Effect of Large-Scale Kelp and Bivalve Farming on Seawater Carbonate System Variations in the Semi-Enclosed Sanggou Bay. Sci. Total. Environ. 753, 142065. doi: 10.1016/j.scitotenv.2020.142065
Li H., Zhang Y., Liang Y., Chen J., Zhu Y., Zhao Y., et al. (2018). Impacts of Maricultural Activities on Characteristics of Dissolved Organic Carbon and Nutrients in a Typical Raft-Culture Area of the Yellow Sea, North China. Mar. Pollut. Bull. 137, 456–464. doi: 10.1016/j.marpolbul.2018.10.048
Mahmood T., Fang J., Jiang Z., Ying W., Zhang J. (2017). Seasonal Distribution, Sources and Sink of Dissolved Organic Carbon in Integrated Aquaculture System in Coastal Waters. Aquacult. Int. 25 (1), 71–85. doi: 10.1007/s10499-016-0014-0
Mao Y., Yang H., Zhou Y., Ye N., Fang J. (2009). Potential of the Seaweed Gracilaria Lemaneiformis for Integrated Multi-Trophic Aquaculture With Scallop Chlamys Farreri in North China. J. Appl. Phycol. 21 (6), 649–656. doi: 10.1007/s10811-008-9398-1
Mou S., Zhang Y., Li G., Li H., Liang Y., Tang L., et al. (2017). Effects of Elevated CO2 and Nitrogen Supply on the Growth and Photosynthetic Physiology of a Marine Cyanobacterium, Synechococcus Sp. PCC7002. J. Appl. Phycol. 29 (4), 1755–1763. doi: 10.1007/s10811-017-1089-3
Ning Z., Liu S., Zhang G., Ning X., Li R., Jiang Z., et al. (2016). Impacts of an Integrated Multi-Trophic Aquaculture System on Benthic Nutrient Fluxes: A Case Study in Sanggou Bay, China. Aquacult. Env. Interac. 8, 221–232. doi: 10.3354/aei00144
Page H. N., Courtney T. A., Carlo E., Howins N. M., Koester I., Andersson A. (2019). Spatiotemporal Variability in Seawater Carbon Chemistry for a Coral Reef Flat in Kāne’ohe Bay, Hawai’i. Limnol. Oceanog. 64 (3), 913–934. doi: 10.1002/lno.11084
Petrović P., Lobanov M. M. (2020). The Impact of R&D Expenditures on CO2 Emissions: Evidence From Sixteen OECD Countries. J. Clean. Prod. 248, 119187. doi: 10.1016/j.jclepro.2019.119187
Ren L. H. (2014). Research on Carbon Sequestration of Cultured Oyster (Crassostrea Gigas) and Its Fouling Organisms in Sungo Bay (Beijing, China: University of Chinese Academy of Sciences, Doctor Dissertation).
Sabine C. L., Feely R. A., Gruber N., Key R. M., Lee K., Bullister J. L., et al. (2004). The Oceanic Sink for Anthropogenic CO2. Science 305 (5682), 367–371. doi: 10.1126/science.1097403
Sugden A. M. (2017). Threats of Coastal Hypoxia. Science 356 (6333), 38.1–3838. doi: 10.1126/science.356.6333.38-a
Tang Q., Zhang J., Fang J. (2011). Shellfish and Seaweed Mariculture Increase Atmospheric CO2 Absorption by Coastal Ecosystems. Mar. Ecol. Prog. Ser. 424, 97–104. doi: 10.3354/meps08979
Wahl M., Covachã S., Saderne V., Hiebenthal C., Müller J. D., Pansch C., et al. (2018). Macromacro-Algae May Mitigate Ocean Acidification Effects on Mussel Calcification by Increasing Ph and Its Fluctuations: Biogenic Fluctuations Mitigate OA Effects. Limnol. Oceanog. 63 (1), 3–21. doi: 10.1002/lno.10608
Weiss R. F. (1974). Carbon Dioxide in Water and Seawater: The Solubility of a Non-Ideal Gas. Mar. Chem. 2 (3), 203–215. doi: 10.1016/0304-4203(74)90015-2
Xia B., Chen B., Cui Y., Cui Z., Zhang X., Liu H., et al. (2013). Distribution and Source of Organic Carbon in Sanggou Bay in Summer. Prog. Fish. Sci. 34 (1), 44–49. doi: 10.3969/j.issn.1000-7075.2013.01.007
Xiao X., Agustí S., Yu Y., Huang Y., Chen W., Hu J., et al. (2021). Seaweed Farms Provide Refugia From Ocean Acidification. Sci. Total. Environ. 776, 145192. doi: 10.1016/j.scitotenv.2021.145192
Zhang M., Fang J., Zhang J., Li B., Ren S., Mao Y., et al. (2011). Effect of Marine Acidification on Calcification and Respiration of Chlamys Farreri. J. Shellfish Res. 30 (2), 267–271. doi: 10.2983/035.030.0211
Zhang J., Hansen P., Fang J., Wang W., Jiang Z. (2009). Assessment of the Local Environmental Impact of Intensive Marine Shellfish and Seaweed Farming—Application of the MOM System in the Sungo Bay, China. Aquaculture 287 (3-4), 304–310. doi: 10.1016/j.aquaculture.2008.10.008
Zhang Y., Zhang J., Liang Y., Li H., Li G., Chen X., et al. (2017). Carbon Sequestration Processes and Mechanisms in Coastal Mariculture Environments in China. Sci. China Earth Sci. 60 (12), 2097–2107. doi: 10.1007/s11430-017-9148-7
Keywords: shellfish, macro-algae, aquaculture, carbon sink, Sanggou Bay
Citation: Liu Y, Zhang J, Wu W, Zhong Y, Li H, Wang X, Yang J and Zhang Y (2022) Effects of Shellfish and Macro-Algae IMTA in North China on the Environment, Inorganic Carbon System, Organic Carbon System, and Sea–Air CO2 Fluxes. Front. Mar. Sci. 9:864306. doi: 10.3389/fmars.2022.864306
Received: 28 January 2022; Accepted: 31 March 2022;
Published: 29 April 2022.
Edited by:
Dapeng Liu, Georgia Institute of Technology, United StatesReviewed by:
Junbo Zhang, Shanghai Ocean University, ChinaZhe Pan, Hebei Agricultural University, China
Guang-Tao Zhang, Institute of Oceanology (CAS), China
Copyright © 2022 Liu, Zhang, Wu, Zhong, Li, Wang, Yang and Zhang. This is an open-access article distributed under the terms of the Creative Commons Attribution License (CC BY). The use, distribution or reproduction in other forums is permitted, provided the original author(s) and the copyright owner(s) are credited and that the original publication in this journal is cited, in accordance with accepted academic practice. No use, distribution or reproduction is permitted which does not comply with these terms.
*Correspondence: Jihong Zhang, emhhbmdqaEB5c2ZyaS5hYy5jbg==