- Department of Animal Ecology and Systematics, Justus Liebig University Giessen, Giessen, Germany
Microplastics are omnipresent in the oceans and threaten marine animals through physical contact or ingestion. Short-term studies have already shown that reef-building stony corals respond differently to microplastics than natural food. However, it remains unknown whether corals exhibit acclimation mechanisms to combat the effects of microplastic exposure. Specifically, the long-term effects of microplastics on the feeding and defense behavior of reef-building corals remain unexplored. Therefore, the goal of this study was to infer potential acclimation mechanisms in the behavior of the corals. For this, four reef-building species (Acropora muricata, Porites lutea, Pocillopora verrucosa, and Heliopora coerulea) were exposed in a long-term experiment to microplastics for 15 months. Subsequently, coral feeding rates on microplastics and natural food (Artemia sp. cysts), feeding discrimination, and reactions to both were assessed in a 24 h pulse exposure experiment. The results showed that corals’ feeding rates did not decrease after long-term exposure to microplastics. Similarly, the feeding discrimination (i.e., ratio of feeding on microplastics and natural food) did not differ after long-term exposure to microplastics. Moreover, corals showed no changes in defense behavior (i.e., mucus production or extrusion of mesenterial filaments) against microplastics. These findings suggest that symbiotic, reef-building corals do not develop mechanisms to adapt to long-term microplastic exposure. Thus, microplastic pollution might constitute a constant stressor for coral organisms, likely leading to sustained energy expenditures and impaired health.
Introduction
Microplastic pollution poses an increasing threat to marine ecosystems (Law and Thompson, 2014; Auta et al., 2017; Galloway et al., 2017), including coral reefs (Lamb et al., 2018; de Oliveira Soares et al., 2020; Huang et al., 2021). Microplastics are defined as polymer particles between 1 µm and 1 mm in size and can occur in different shapes, structures, and densities (Hartmann et al., 2019). They may cause harm to marine organisms as they often harbor toxins that can be transferred to the organisms (Saliu et al., 2019; Patterson et al., 2020). In addition, microplastics also develop a distinct biofilm on the surface, called the “plastisphere” (Zettler et al., 2013; Amaral-Zettler et al., 2020), which may contain potential coral pathogens (e.g., Vibrio spp. strains; Franco et al., 2020; Kesy et al., 2020) or foster confusion with prey (Hall et al., 2015). Ultimately, microplastics may also lead to compromised health through increased feeding efforts, reduced food uptake, and subsequent energy losses (Wright et al., 2013; Yin et al., 2018). In reef-building corals, exposure to microplastics is associated with reduced growth (Chapron et al., 2018; Hankins et al., 2021), lower feeding rates (Savinelli et al., 2020; Corinaldesi et al., 2021), changes in photosynthetic efficiency (Lanctôt et al., 2020), a compromised immune system (Tang et al., 2018), and an overall deterioration in health (Reichert et al., 2019). These adverse effects are often attributed to direct contact with microplastic particles, regularly followed by ingestions. Feeding on microplastics is suspected of causing increased energy demand through energetically costly microplastic handling (i.e., repeated particle captures, ingestion, and egestion) (Chapron et al., 2018; Mouchi et al., 2019; Reichert et al., 2019). Although microplastic particles are typically covered with a biofilm, ingestion does not likely result in a considerable nutritional return (Murphy and Quinn, 2018; Reichert et al., 2018; Reichert et al., 2019). Even though previous studies showed that corals typically egest indigestible material within 24 h (Mills and Sebens, 2004; Allen et al., 2017; Reichert et al., 2018), the gastric space is occupied by the indigestible and nutrient-deficient material, which prevents the digestion of food (Murphy and Quinn, 2018; Rotjan et al., 2019). Furthermore, recurrent ingestions and blockages of the digestive tract (Hankins et al., 2018) might cause incorporation into the coral skeleton (Hierl et al., 2021; Krishnakumar et al., 2021; Reichert et al., 2021a). Yet not all coral species ingest microplastics in the same amounts and might thus be unequally threatened. Some coral species, such as Dipsastraea pallida, ingest as much microplastics as natural food (Hall et al., 2015). Other species even appear to prefer plastic particles over their natural food, such as Astrangia poculata (Rotjan et al., 2019). Still other coral species appear to recognize plastic particles as indigestible or ingest them to a lesser extent compared to natural food, e.g., Danafungia scruposa (Corona et al., 2020), Goniastrea retiformis (Martin et al., 2019), and Astroides calycularis (Savinelli et al., 2020). However, methods vary between studies, limiting comparisons.
Corals can also actively repel unwanted, indigestible particles. General defense mechanisms include active cleaning, i.e., polyp and ciliary movements, mucus production, mesenterial filaments extrusion, and tissue contraction and expansion to remove sediments from their surface (Stafford-Smith and Ormond, 1992; Stafford-Smith, 1993; Erftemeijer et al., 2012). Several coral species showed similar defensive reactions (i.e., mucus production in Porites spp. or extrusion of mesenterial filaments in Pocillopora spp.) when they encounter microplastics (Reichert et al., 2018; Martin et al., 2019; Mouchi et al., 2019; Lanctôt et al., 2020).
The numerous adverse effects following contact with microplastics suggest that microplastics are a stressor for reef-building corals (Reichert et al., 2019; Hankins et al., 2021). Corals possess various mechanisms to cope with environmental stressors (Dallmeyer et al., 1982; Brown, 1997; Gates and Edmunds, 1999). For example, corals respond with altered feeding behavior to better cope with elevated temperatures, sedimentation, and ocean acidification (Anthony and Fabricius, 2000; Grottoli et al., 2006; Towle et al., 2015). This phenomenon, known as heterotrophic plasticity, helps corals improve their energy budget and maintain their physiological status under extended periods of stress (Fox et al., 2018; Sturaro et al., 2021). However, it is still unclear whether reef-building corals can also adapt their behavior to microplastics and thus avoid microplastic ingestion in the future.
Therefore, the general goal of our study was to assess changes in coral behavior after long-term exposure to microplastic particles. Specifically, we determined whether corals adapt to long-term microplastic exposure through (I) heterotrophic plasticity (i.e., no. of ingested microplastic particles), (II) improved discrimination (i.e., no. of ingested microplastics per natural food particle), or (III) increased defense mechanisms (i.e., the occurrence of mucus production or extrusion of mesenterial filaments). To this end, we conducted a controlled long-term microcosm experiment in which the feeding and defense behavior of four common reef-building coral species exposed to polyethylene (PE) microplastics over 15 months was compared to a microplastic-free control group. The findings may help to better understand the adaptive response of vital reef-building organisms to microplastic pollution.
Materials and Methods
Studied Coral Species
Four common Indo-Pacific coral species, Acropora muricata (Linnaeus, 1758), Pocillopora verrucosa (Ellis & Solander, 1786), Porites lutea Milne-Edwards & Haime, 1851, and Heliopora coerulea (Pallas, 1766), were used for the controlled microcosm experiment, representing similar polyp sizes (1–2 mm diameter) but different morphologies (i.e., branching, massive, and columnar). For A. muricata, P. verrucosa, and P. lutea, 18 fragments per species were included, derived from three origin colonies. For H. coerulea, six fragments were included, derived from one origin colony. Corals were sampled in the Red Sea and off Bali between 2013 and 2015 or derived from a zoological garden (for details on colony origin and CITES numbers see Supplementary Table S1), and kept under laboratory conditions in the Ocean2100 facility for at least six months before the start of the long-term experiment (temperature: 26 ± 0.5°C; light intensity: 200 µmol photons m−2 s−1 at a photoperiod of 10:14 dark:light).
Experimental Design
Coral fragments were exposed to polyethylene (PE) microplastics (~200 particles L-1) or microplastic-free control conditions for 15 months, and feeding and defense behavior was studied (Figure 1). Feeding rates and defensive reactions were quantified in individual incubations via the number of particles left after 24 h of pulse exposure. Coral feeding rates were assessed by exposure to control and microplastic particles: First, a control quantification of feeding activity was conducted with Artemia sp. cysts (Kellogg, 1906) to avoid potential effects of microplastic feeding on the control feed. Then the coral feeding rates on microplastics were assessed. Defense behavior was observed at the end of the 24 h exposure.
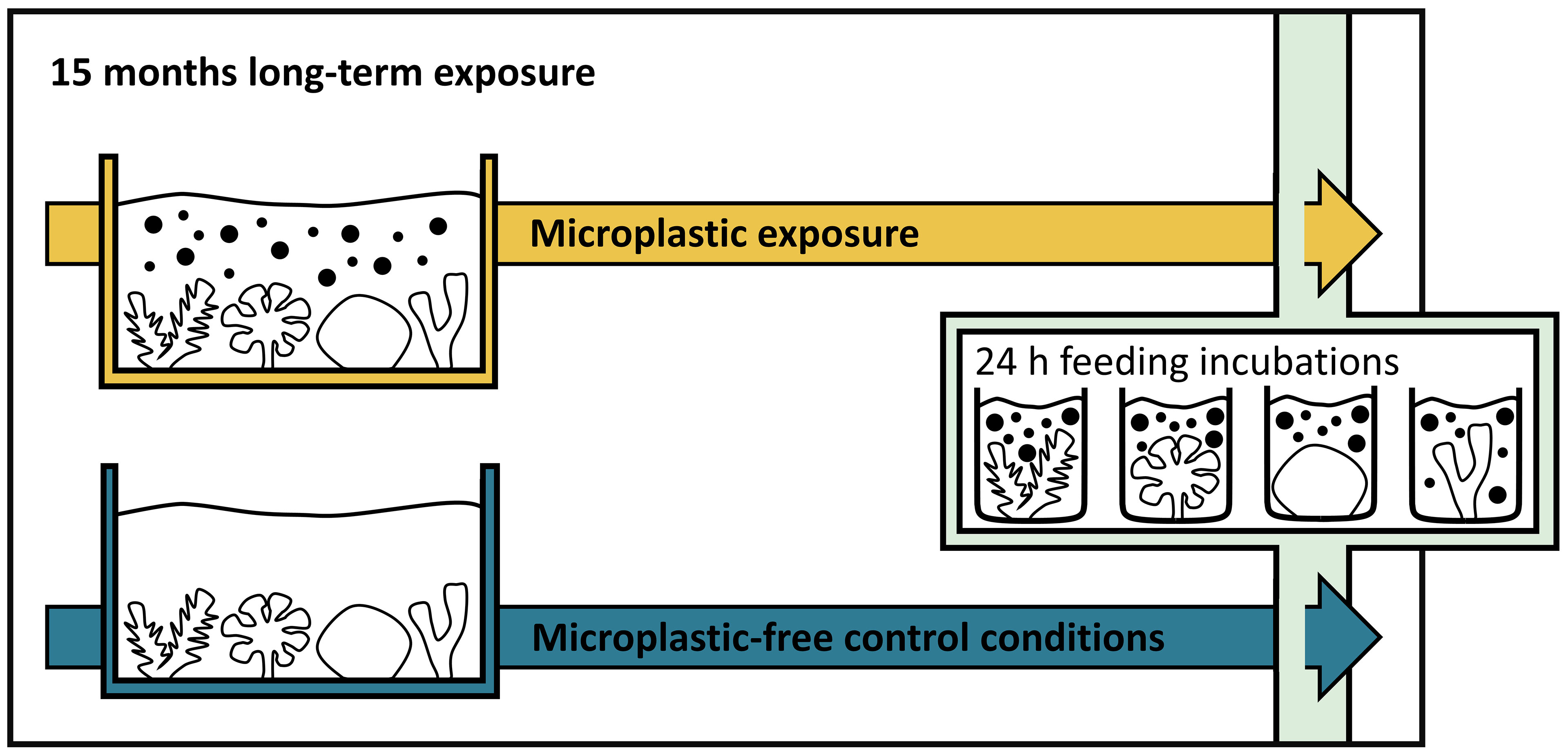
Figure 1 The timeline of the experiment displays coral fragments from 15-month long-term microplastic exposure (in yellow; top) and from long-term control conditions (in blue; bottom). Corals’ feeding and defense behavior on Artemia sp. cysts and microplastics were assessed in separate, consecutive 24 h pulse exposures at the end of the experiment.
Long-Term Microplastic Exposure
The long-term exposure was conducted in six 80 L tanks (n = 3 per treatment). The tanks were connected to a reef mesocosm system (~4,000 L artificial seawater) to create near-natural conditions with an exchange rate of 120 L per day (≙ 150% of the tank volume). Three weeks prior to the start, coral fragments were distributed to the tanks (one fragment per colony per species per tank) and randomly allocated within the tanks with a min. distance of 5 cm to avoid interferences. Tank outflows were equipped with filters (65 µm mesh size) to retain particles inside the experimental tanks allowing a biofilm to form on the submerged particles. Water parameters were maintained under controlled conditions during the long-term experiment (temperature: 26 ± 0.2°C; light intensity: 135 µmol photons m−2 s−1 at a photoperiod of 10:14 dark:light), and corals were fed indirectly through the connected mesocosm system, which was supplied with frozen food (i.e., copepods and Mysis spp.) daily. For additional information on the technical setup see Supplementary Methods S1.1.
Microplastic Characteristics and Treatment
Irregular black PE microplastic particles (density: 0.95 g cm-3; for FTIR chart see Figure S1; Novoplastik, Germany) were used, as it is one of the most common polymer types in reef waters (Saliu et al., 2018; Patterson et al., 2020). The size of the PE particles (diameter: 184 ± 95 µm (mean ± SD), for details, see Figure S2 and Supplementary Methods S1.2) corresponds to the size of the plankton diet of corals (Houlbrèque and Ferrier-Pagès, 2009). Pristine particles were sterilized in ethanol (70% abv; 24 h) and then rinsed with deionized (DI) water. 2.5 mg L-1 sterilized microplastics were added to the tanks resulting in a concentration of ~ 200 particles L-1 or 0.25 mg L-1 after 48 h in the long-term experimental tank setup. The biofilm growth on the microplastics reduced the buoyancy to about the same density as seawater, making them available to the corals. Microplastic concentration in the water surrounding the corals was monitored every two months, with higher number of measurements at the beginning of the experiment until concentrations stabilized. For this, 50 mL tank water was filtered onto a 65 µm gauze filter (3–5 replicate measurements per tank) and the number of particles were counted under a stereo microscope to extrapolate the concentration per L. Concentrations were maintained at ~200 particles L-1 (201 ± 67 particles L-1, n = 518 measurements at 125 timepoints) over the course of the experiment by the addition of fresh particles when necessary.
Pulse Exposure to Natural Feed and Microplastic
Artemia sp. Cysts Fed as Natural Feed Control
Decapsulated brine shrimp eggs (Artemia cf. franciscana (Kellogg, 1906); INVE Technologies, Belgium) were used as control feed in the 24 h pulse exposure because they are a standard feed for corals in aquaculture (Helmuth and Sebens, 1993; Sebens et al., 1998). The size of the Artemia sp. cysts (diameter: 175 ± 16 (mean ± SD) was comparable to the size of the microplastic particles used (Figure S2).
Biofouled Microplastics
The same PE particles were used in the long-term and the 24 h pulse exposure. To mimic near-natural conditions in the pulse exposure, biofouled particles were generated. For this, pristine microplastics were sterilized as described above. Then microplastics were incubated for 16 days in 50 L of 35 µm filtrated artificial seawater from the reef mesocosm system to allow for a biofilm to form [26°C, salinity: 34 ± 2 ‰, two 80 W fluorescent lamps (Aquablue Special and Blue Plus, ATI, Germany), two pumps (SW-4, Jebao, China)].
Incubations
Feeding and defense behavior were assessed in incubations where corals were provided with Artemia sp. cysts (control feed) or microplastics for 24 h of pulse exposure. The number of particles added to the incubation was chosen to match the number of polyps per coral fragment to ensure the availability of particles. For the Artemia sp. cyst feeding, 5 ± 0.008 mg cysts, equivalent to ~1,760 ± 66 particles L-1 (weighed with analytical balance (accuracy: 0.001 mg, CPA2P, Sartorius, Germany)) were suspended in 0.8 L of 0.22 µm filtrated seawater (Express Plus Membrane, Millipore, USA). For the microplastic feeding, 270 ml of a microplastic-seawater suspension (see section biofouled microplastics) was added to 530 ml of 0.22 µm filtrated seawater, equivalent to 1,850 ± 230 particles L-1. The coral fragments were hung upside down in the beakers and secured with fishing line (d = 0.12 mm, Spiderwire stealth code red braid, Pure Fishing, USA). This positioning was chosen to avoid particle accumulation at the base of the concrete socket. Particles were uniformly distributed within the water column by air supply (two 228 mm glass pipettes) and a stir bar (20 mm × 8 mm (l × d) rotating at ~500 rpm). Ten pulse exposure incubations were performed simultaneously in one run on a multipoint stirring incubator (Rades et al. 2022). For each run, coral fragments were randomly chosen from the microcosm setup (n = 7), and their feeding behavior was assessed simultaneously with coral-free control incubations (n = 3, equipped with pipettes, stir bars, and bare fishing lines only). A total of eight runs per treatment (each lasting for 24 h, for both microplastics and natural food) were conducted, with a mean time interval of 2–3 days between each run. Temperatures were kept at 26 ± 0.3°C through a water bath.
Assessment of Feeding Rates
Particle Recovery, Documentation, and Quantification
After the 24 h pulse exposure, particles were retrieved from each incubation and quantified. For this purpose, all equipment was removed from the incubation chambers. Fragments were gently shaken in the water to free adhering particles and placed back in the microcosm setup. All equipment and fragments were carefully rinsed with 0.22 µm filtered seawater between all steps to retain all adherent particles and avoid contamination. The seawater particle suspension was vacuum filtrated onto a cellulose nitrate filter (8 µm pore size, Sartorius, Germany). The filter was documented under a digital microscope (VHX-2000, Keyence, Japan) using the automatic 2D image stitching mode with the VH-Z20W lens at 50x magnification and a mounted polarizing filter. Pictures were saved as TIFF. Microplastic particle numbers were automatically determined using ImageJ (v1.51s; Rueden et al., 2017). For a detailed description of image processing and counting, see Table S2.
Determination of Coral Surface Area
Coral tissue surface area was used to standardize feeding rates per cm2. The surface area was quantified via 3D scanning, following established procedures (Reichert et al., 2016; Reichert et al., 2018; Reichert et al., 2019). Briefly, 3D models of coral fragments were created after the pulse exposure using a hand-held 3D scanner (Artec Spider with Artec Studio 10, Artec 3D, Luxembourg) and coral tissue surface area derived (for details, see Table S3 and Supplementary Methods S1.3).
Calculation of Feeding Rates
Feeding rates (in number of particles per cm2 per 24 h) were assessed as the difference between the residual particles of the incubations with corals and the control incubations, based on established procedures (Hii et al., 2009). For this, the count of remaining particles in the coral feeding chamber (number of particlescoral) was subtracted from the mean number of particles found in the control chambers (mean number of particlescontrol) of the respective run (Equation 1).
Calculated feeding rates were standardized to the surface area of the coral and expressed as the number of particles ingested per cm2 coral surface area per 24 h.
Quantifying the Ability of Corals to Discriminate Between Microplastics and Natural Food
The number of fed microplastic particles per fed Artemia sp. cyst was determined to assess the ability of corals to discriminate microplastics from natural food. This discrimination ability value was calculated per coral fragment. These values were then compared between long-term exposure conditions (microplastic vs. microplastic-free).
Reactions of Corals to Microplastic Pulse Exposure
Visible physiological reactions of corals to the microplastic pulse exposure (i.e., mucus production, extruded mesenterial filaments) were documented at the end of the 24 h incubation for each fragment. Because of a possible simultaneous occurrence of both reactions, observations were broadly classified as (1) reaction or (2) no reaction. Reactions during the control feeding with Artemia sp. cysts were assessed similarly to distinguish between reactions to the feeding procedure itself and the microplastic exposure.
Statistics
Statistical analyzes were conducted with “R” (v4.1.2, R Core Team, 2021) using the “RStudio” interface (v2021.9.0.351, RStudio Team, 2021). Unless otherwise stated, tests from the “rstatix” package (v0.7.0, Kassambara, 2021) were used. Data were tested for normality using the histogram, Shapiro-Wilk test, and Q-Q and P-P plots (Almeida et al., 2019) and found to be non-normally distributed. Therefore, non-parametric tests were used further on. Kruskal-Wallis with Dunn’s post hoc tests examined interspecific differences in microplastic and Artemia sp. cyst feeding rates. Wilcoxon tests were used to evaluate intraspecific differences in feeding rates between particle types (microplastics vs. Artemia sp. cysts). Wilcoxon tests were also used to compare the impacts of the two long-term exposure treatments on feeding rates. The effect of long-term exposure on the ability of corals to discriminate between microplastics and natural food (Artemia sp. cysts) was evaluated using Wilcoxon tests, with one extreme value (> Q3 + 3 × IQR) removed beforehand. Kruskal-Wallis with Dunn’s post hoc tests examined interspecific differences in the ability of discrimination. The impact of long-term exposure to microplastics on coral reactions was assessed using Fisher’s exact tests. Pearson’s χ2 tests were used to compare coral reactions between microplastics and Artemia sp. cysts. Effect sizes for Fisher’s exact test and the χ2 test were obtained using the “effectsize” package (v0.5, Ben-Shachar et al., 2020).
Results
Coral Feeding Rates on Microplastics After Long-Term Exposure
Long-term microplastic exposure did not affect coral feeding rates on microplastic particles in any species (Wilcoxon tests, p > 0.05; Figure 2A and Table S4). Similarly, the exposure did not affect feeding rates on the control feed (Artemia sp. cysts), (Wilcoxon tests, p > 0.05; Figure S4A and Table S5). In general, corals showed lower feeding rates on microplastics (1.06 ± 2.08; mean ± SD) than on Artemia sp. cysts (7.02 ± 8.18; mean ± SD). Specifically, A. muricata (Wilcoxon test, p < 0.001) and P. verrucosa (Wilcoxon test, p < 0.001) fed significantly fewer microplastics (Figure S5 and Table S6). However, there were no interspecific differences in feeding rates for either microplastics or Artemia sp. cysts (Kruskal-Wallis and Dunn’s tests, p > 0.05; Figure S6 and Table S7 and S8).
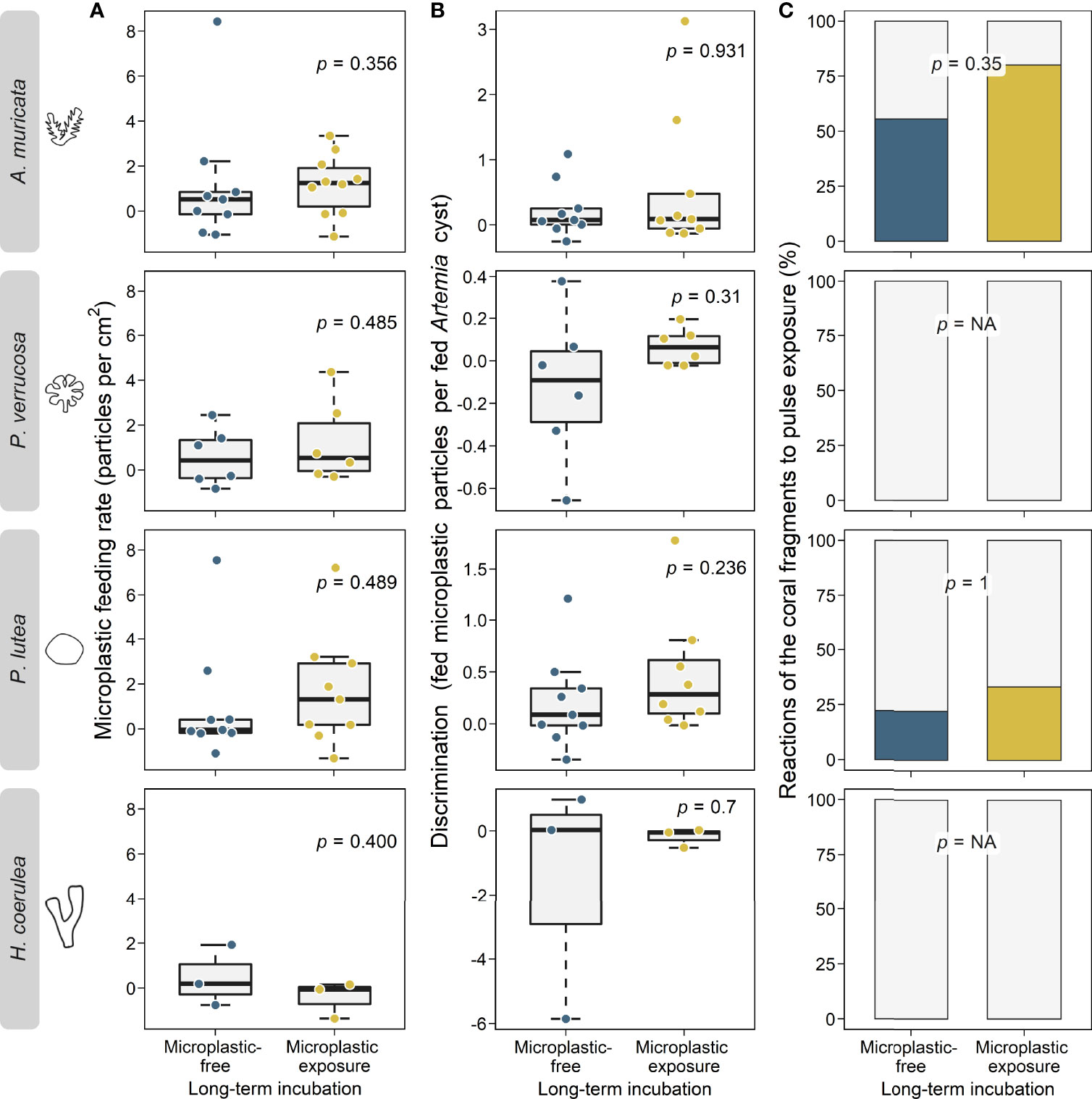
Figure 2 Impacts of long-term exposure to microplastics (in yellow) and microplastic-free control conditions (in blue) on coral microplastic feeding and defense behavior of the four coral species A muricata, P. verrucosa, P. lutea, and H coerulea. (A): Coral feeding rates on microplastics (particles per cm2 coral tissue per 24 h) after long-term exposure to microplastics and microplastic-free control conditions. Data is displayed as box-and-whisker plots with raw data points. P-values are derived from Wilcoxon tests. (B): Corals’ discrimination ability (no. of fed microplastic particles per fed particle of natural food (Artemia sp. cysts)) after long-term exposure to microplastics and microplastic-free control conditions. Data is displayed as box-and-whisker plots with raw data points. P-values are derived from Wilcoxon tests. (C): Corals’ defense reactions to microplastic (% of fragments that show reactions) after long-term exposure to microplastic and microplastic-free control conditions Data is displayed as percent stacked bar charts and p-values are derived from Fisher’s exact tests. NA, not available.
Coral Ability to Discriminate Between Microplastics and Natural Food After Long-Term Exposure
Discrimination ability (no. of microplastic particles ingested per Artemia sp. cyst ingested) did not differ between the two long-term conditions (microplastic exposure: 0.34 ± 0.76 microplastic particles per Artemia cyst; mean ± SD vs. microplastic free: -0.06 ± 1.24 microplastic particles per Artemia cyst; mean ± SD) in any species (Wilcoxon tests, p > 0.05, Figure 2B and Table S8). In addition, no interspecific differences were found (Kruskal-Wallis and Dunn’s tests, p > 0.05, Figure S7 and Table S10 and S11).
Coral Defense Reactions After Long-Term Exposure
A. muricata and P. lutea reacted with mucus release and extrusion of mesenterial filaments to the 24 h pulse exposures. P. verrucosa and H. coerulea did not show physiological reactions. Although the reactions occurred more frequently after long-term microplastic exposure, the effect was not statistically significant (Fisher’s exact tests, p > 0.05; Figure 2C and Table S12). Similarly, the long-term microplastic exposure did not affect the frequency of reactions when corals were fed Artemia sp. cysts (Fisher’s exact tests, p > 0.05; Figure S4B and Table S12). In general, reactions occurred more often when corals were fed with microplastics than with Artemia sp. cysts (significant for A. muricata; Chi-squared test, p = 0.013; Figure S8 and Table S13). Specifically, corals fed less when they showed defense reactions (significant for A. muricata; Wilcoxon test, p = 0.048; Figure S9 and Table S14).
Discussion
Corals Do Not Adapt Feeding Mechanisms to Decrease Microplastic Uptake
Our results indicate that corals do not exhibit heterotrophic plasticity in response to long-term microplastic exposure as feeding rates remained unaltered after long-term microplastic exposure (research question I). To avoid stress potentially caused by long-term microplastic exposure, corals would need to either reduce their feeding rates in general or increase their feeding selectivity. However, unchanged feeding rates suggest that the corals studied do not possess or do not activate mechanisms of heterotrophic plasticity to reduce microplastic uptake in response to long-term exposure to microplastics.
Heterotrophic feeding activity has been found to shift (Hughes and Grottoli, 2013; Fox et al., 2019) under certain environmental stresses (e.g., heat stress, turbidity, or ocean acidification; Anthony and Fabricius, 2000; Bessell-Browne et al., 2014; Towle et al., 2015; Pupier et al., 2021). However, microplastics apparently do not trigger mechanisms of heterotrophic plasticity to reduce microplastic uptake. This suggestion is in line with Axworthy and Padilla-Gamiño (2019), who showed that microplastic feeding rates did not change in response to temperature stress. Furthermore, the findings of the short-term study by Chapron et al. (2018), in which Artemia capture rates remained unchanged after microplastic exposure conditions, are confirmed by our findings. A lack of adaptation to changing environmental conditions suggests that as concentrations increase, microplastic particle uptake might also increase linearly, possibly as seen for suspended particulate matter (Anthony, 1999; Anthony and Fabricius, 2000) or suspended sediments (Anthony, 2000).
After offering both types of particles independently, we found that the corals ingested the natural food at a higher rate than microplastics. In general, these findings are consistent with previous studies (Axworthy and Padilla-Gamiño, 2019; Savinelli et al., 2020), although there is also a counterexample (Rotjan et al., 2019). Yet, particle numbers deviated (SD) in average by 38 microplastic particles and 141 Artemia sp. cysts in the pulse exposures. Thus, the sometimes even negative feeding rates are a result of the mathematical approach used to quantify the feeding rates, and indicate low or no the feeding during the pulse exposure.
Long-Term Exposure Does Not Lead to Better Discrimination Between Microplastics and Natural Food
Our results also suggest that corals do not better discriminate between microplastics and natural food (research question II). The number of microplastics fed per Artemia cyst fed remained unchanged for all species. This result indicates that corals were not more effective in avoiding microplastic ingestion after long-term exposure. Although corals generally fed fewer microplastics than natural food, discriminatory ability did not improve further. The basic discriminatory ability of corals leading to higher feeding rates on natural food has been previously shown for reef-building corals (Hall et al., 2015; Martin et al., 2019) but seems to be species-specific as indicated by other studies (Allen et al., 2017; Rotjan et al., 2019). Chemical stimuli might mediate the discrimination process (Houlbrèque and Ferrier-Pagès, 2009). Microplastic feeding may be triggered by both biofilm- or plastic-related stimulants (Allen et al., 2017; Diana et al., 2020). It can be assumed that the biofilm on the particle mainly drives microplastic uptake, as most studies indicate that particles covered with a biofilm are more likely to be ingested than pristine particles (Corona et al., 2020; Weideman et al., 2020). A comparison with sediments supports this concept: particles that are perceived as a source of nutrients due to microbiota colonization are more likely to be taken up by corals than sediments that are poor in nutrients (Mills et al., 2004).
Corals Do Not Increase Defense Mechanisms to Reduce Microplastic Uptake
Our results indicate that corals do not change the frequency of defense reactions to adapt to long-term microplastic exposure (research question 3) and do not appear to develop adaptive defense mechanisms to reduce the impacts of microplastic exposure. On the one hand, an increase in defense reactions could reduce the number of ingested particles. However, such defense behavior may also hinder feeding on natural food (Hughes, 1980; Anthony, 2000) and would thus be unfavorable in the long term. On the other hand, it could also have been assumed that defense reactions would be reduced in response to long-term microplastic exposure to avoid continuous energy expenditures. However, none were detected here, suggesting that microplastic exposure does not alter these reactions.
As an aside, our results confirm that defensive reactions like mucus production and extrusion of mesenterial filaments are typical reactions of A. muricata and P. lutea to microplastics (Reichert et al., 2018; Martin et al., 2019; Mouchi et al., 2019; Jiang et al., 2021). Reactions were observed when corals were exposed to microplastics and less frequently when corals were exposed to natural food. The observed reactions can be interpreted as either defense or feeding reactions, which explains their designation as so-called “multitools” (Brown and Howard, 1985; Brown and Bythell, 2005). However, the observed reactions seem to be defensive, as corals ingested fewer microplastics when reactions were shown.
Our results further indicate that corals apparently ingest microplastics even in the absence of natural food (sensu Corona et al., 2020). Yet, coral microplastic feeding rates might be even higher in the presence of natural food, which triggers feeding through inherent chemical stimulants (Helland et al., 2000; Houlbrèque and Ferrier-Pagès, 2009). Additionally, different polymer types might cause different reactions because of their physical and chemical properties (e.g., shape, size, additives). Further, the influence of microplastics may vary over time as the particles’ properties can be altered through environmental conditions and absorb locally present pollutants. Therefore, further studies should also consider using other microplastics and combinations (i.e., different shapes, polymer types, concentrations, and mix with natural feed) to better resemble in situ conditions.
Conclusions
In summary, our findings suggest that reef-building corals lack acclimation mechanisms (i.e., feeding, avoidance, and defense mechanisms) to reduce the effects of microplastic exposure over longer periods of time. A lack of acclimation implies that microplastics will constitute a permanent stressor in coral reefs. However, it is still unclear to what degree corals will be cumulatively affected by repeated microplastic contacts during their lifetime. Given the projected increase in microplastic concentrations (Everaert et al., 2018; Isobe et al., 2019) and the lack of adaptive mechanisms (i.e., foraging and defensive), microplastic pollution might be of increasing concern. In combination with other stressors (e.g., global warming and ocean acidification; Hoegh-Guldberg et al., 2007; Conti-Jerpe et al., 2020; Reichert et al., 2021b), microplastics are likely to contribute to shifts in coral reef communities worldwide. Therefore, immediate action is needed to curb microplastic and plastic pollution, in close coordination with protective measures against global warming and ocean acidification, to preserve the remaining coral reefs.
Data Availability Statement
The datasets presented in this study are publicly available at figshare: https://doi.org/10.6084/m9.figshare.19073312.
Author Contributions
MR: Methodology, Software, Formal Analysis, Investigation, Writing – Original Draft, Visualization. JR: Conceptualization, Methodology, Formal Analysis, Resources, Data Curation, Writing – Review and Editing, Project Administration, Supervision. PS: Resources, Writing – Review and Editing. TW: Conceptualization, Writing – Review and Editing.
Funding
This study was conducted as part of the ‘Ocean 2100’ global change simulation project of the Colombian-German Center of Excellence in Marine Sciences (CEMarin) funded by the German Academic Exchange Service (DAAD, project number 57480468).
Conflict of Interest
The authors declare that the research was conducted in the absence of any commercial or financial relationships that could be construed as a potential conflict of interest.
Publisher’s Note
All claims expressed in this article are solely those of the authors and do not necessarily represent those of their affiliated organizations, or those of the publisher, the editors and the reviewers. Any product that may be evaluated in this article, or claim that may be made by its manufacturer, is not guaranteed or endorsed by the publisher.
Acknowledgments
We are thankful for Angelina Arnold’s contribution to the 15-month long-term experiment and Maren Ziegler’s helpful comments on the manuscript.
Supplementary Material
The Supplementary Material for this article can be found online at: https://www.frontiersin.org/articles/10.3389/fmars.2022.863187/full#supplementary-material
References
Allen A. S., Seymour A. C., Rittschof D. (2017). Chemoreception Drives Plastic Consumption in a Hard Coral. Mar. pollut. Bull. 124, 198–205. doi: 10.1016/j.marpolbul.2017.07.030
Almeida A., Loy A., Hofmann H. (2019). Ggplot2 Compatible Quantile-Quantile Plots in R. R. J. 10, 248. doi: 10.32614/RJ-2018-051
Amaral-Zettler L. A., Zettler E. R., Mincer T. J. (2020). Ecology of the Plastisphere. Nat. Rev. Microbiol. 18, 139–151. doi: 10.1038/s41579-019-0308-0
Anthony K. R. N. (1999). Coral Suspension Feeding on Fine Particulate Matter. J. Exp. Mar. Bio. Ecol. 232, 85–106. doi: 10.1016/S0022-0981(98)00099-9
Anthony K. (2000). Enhanced Particle-Feeding Capacity of Corals on Turbid Reefs (Great Barrier Reef, Australia). Cor. Reef. 19, 59–67. doi: 10.1007/s003380050227
Anthony K. R. N., Fabricius K. E. (2000). Shifting Roles of Heterotrophy and Autotrophy in Coral Energetics Under Varying Turbidity. J. Exp. Mar. Bio. Ecol. 252, 221–253. doi: 10.1016/S0022-0981(00)00237-9
Auta H. S., Emenike C. U., Fauziah S. H. (2017). Distribution and Importance of Microplastics in the Marine Environment: A Review of the Sources, Fate, Effects, and Potential Solutions. Environ. Int. 102, 165–176. doi: 10.1016/j.envint.2017.02.013
Axworthy J. B., Padilla-Gamiño J. L. (2019). Microplastics Ingestion and Heterotrophy in Thermally Stressed Corals. Sci. Rep. 9, 18193. doi: 10.1038/s41598-019-54698-7
Ben-Shachar M., Lüdecke D., Makowski D. (2020). Effectsize: Estimation of Effect Size Indices and Standardized Parameters. J. Open Source Software 5, 2815. doi: 10.21105/joss.02815
Bessell-Browne P., Stat M., Thomson D., Clode P. L. (2014). Coscinaraea Marshae Corals That Have Survived Prolonged Bleaching Exhibit Signs of Increased Heterotrophic Feeding. Cor. Reef. 33, 795–804. doi: 10.1007/s00338-014-1156-z
Brown B. E. (1997). Adaptations of Reef Corals to Physical Environmental Stress. Adv. Mar. Biol. 31, 221–99. doi: 10.1016/S0065-2881(08)60224-2
Brown B. E., Bythell J. C. (2005). Perspectives on Mucus Secretion in Reef Corals. Mar. Ecol. Prog. Ser. 296, 291–309. doi: 10.3354/meps296291
Brown B. E., Howard L. S. (1985). Assessing the Effects of ‘Stress’ on Reef Corals. Adv. Mar. Biol., 1–63. doi: 10.1016/S0065-2881(08)60049-8
Chapron L., Peru E., Engler A., Ghiglione J. F., Meistertzheim A. L., Pruski A. M., et al. (2018). Macro- and Microplastics Affect Cold-Water Corals Growth, Feeding and Behaviour. Sci. Rep. 8, 15299. doi: 10.1038/s41598-018-33683-6
Conti-Jerpe I. E., Thompson P. D., Wong C. W. M., Oliveira N. L., Duprey N. N., Moynihan M. A., et al. (2020). Trophic Strategy and Bleaching Resistance in Reef-Building Corals. Sci. Adv. 6, eaaz5443. doi: 10.1126/sciadv.aaz5443
Corinaldesi C., Canensi S., Dell’Anno A., Tangherlini M., Di Capua I., Varrella S., et al. (2021). Multiple Impacts of Microplastics can Threaten Marine Habitat-Forming Species. Commun. Biol. 4, 431. doi: 10.1038/s42003-021-01961-1
Corona E., Martin C., Marasco R., Duarte C. M. (2020). Passive and Active Removal of Marine Microplastics by a Mushroom Coral (Danafungia Scruposa). Front. Mar. Sci. 7. doi: 10.3389/fmars.2020.00128
Dallmeyer D. G., Porter J. W., Smith G. J. (1982). Effects of Particulate Peat on the Behavior and Physiology of the Jamaican Reef-Building Coral Montastrea Annularis. Mar. Biol. 68, 229–233. doi: 10.1007/BF00409589
de Oliveira Soares M., Matos E., Lucas C., Rizzo L., Allcock L., Rossi S. (2020). Microplastics in Corals: An Emergent Threat. Mar. pollut. Bull. 161, 111810. doi: 10.1016/j.marpolbul.2020.111810
Diana Z., Sawickij N., Rivera N. A., Hsu-Kim H., Rittschof D. (2020). Plastic Pellets Trigger Feeding Responses in Sea Anemones. Aquat. Toxicol. 222, 105447. doi: 10.1016/j.aquatox.2020.105447
Erftemeijer P. L. A., Riegl B., Hoeksema B. W., Todd P. A. (2012). Environmental Impacts of Dredging and Other Sediment Disturbances on Corals: A Review. Mar. pollut. Bull. 64, 1737–1765. doi: 10.1016/j.marpolbul.2012.05.008
Everaert G., Van Cauwenberghe L., De Rijcke M., Koelmans A. A., Mees J., Vandegehuchte M., et al. (2018). Risk Assessment of Microplastics in the Ocean: Modelling Approach and First Conclusions. Environ. pollut. 242, 1930–1938. doi: 10.1016/j.envpol.2018.07.069
Fox M. D., Elliott Smith E. A., Smith J. E., Newsome S. D. (2019). Trophic Plasticity in a Common Reef-Building Coral: Insights From δ 13 C Analysis of Essential Amino Acids. Funct. Ecol. 33, 2203–2214. doi: 10.1111/1365-2435.13441
Fox M. D., Williams G. J., Johnson M. D., Radice V. Z., Zgliczynski B. J., Kelly E. L. A., et al. (2018). Gradients in Primary Production Predict Trophic Strategies of Mixotrophic Corals Across Spatial Scales. Curr. Biol. 28, 3355–3363.e4. doi: 10.1016/j.cub.2018.08.057
Franco A., Rückert C., Blom J., Busche T., Reichert J., Schubert P., et al. (2020). High Diversity of Vibrio Spp. Associated With Different Ecological Niches in a Marine Aquaria System and Description of Vibrio Aquimaris Sp. Nov. Syst. Appl. Microbiol. 43, 126123. doi: 10.1016/j.syapm.2020.126123
Galloway T. S., Cole M., Lewis C. (2017). Interactions of Microplastic Debris Throughout the Marine Ecosystem. Nat. Ecol. Evol. 1, 1–8. doi: 10.1038/s41559-017-0116
Gates R. D., Edmunds P. J. (1999). The Physiological Mechanisms of Acclimatization in Tropical Reef Corals. Am. Zool. 39, 30–43. doi: 10.1093/icb/39.1.30
Grottoli A. G., Rodrigues L. J., Palardy J. E. (2006). Heterotrophic Plasticity and Resilience in Bleached Corals. Nature 440, 1186–1189. doi: 10.1038/nature04565
Hall N. M., Berry K. L. E., Rintoul L., Hoogenboom M. O. (2015). Microplastic Ingestion by Scleractinian Corals. Mar. Biol. 162, 725–732. doi: 10.1007/s00227-015-2619-7
Hankins C., Duffy A., Drisco K. (2018). Scleractinian Coral Microplastic Ingestion: Potential Calcification Effects, Size Limits, and Retention. Mar. pollut. Bull. 135, 587–593. doi: 10.1016/j.marpolbul.2018.07.067
Hankins C., Moso E., Lasseigne D. (2021). Microplastics Impair Growth in Two Atlantic Scleractinian Coral Species, Pseudodiploria Clivosa and Acropora Cervicornis. Environ. pollut. 275, 116649. doi: 10.1016/j.envpol.2021.116649
Hartmann N. B., Hüffer T., Thompson R. C., Hassellöv M., Verschoor A., Daugaard A. E., et al. (2019). Are We Speaking the Same Language? Recommendations for a Definition and Categorization Framework for Plastic Debris. Environ. Sci. Technol. 53, 1039–1047. doi: 10.1021/acs.est.8b05297
Helland S., Triantaphyllidis G. V., Fyhn H. J., Evjen M. S., Lavens P., Sorgeloos P., et al. (2000). Modulation of the Free Amino Acid Pool and Protein Content in Populations of the Brine Shrimp Artemia spp. Mar. Biol., 137, 1005–1016. doi: 10.1007/s002270000409
Helmuth B., Sebens K. (1993). The Influence of Colony Morphology and Orientation to Flow on Particle Capture by the Scleractinian Coral Agaricia Agaricites (Linnaeus). J. Exp. Mar. Bio. Ecol. 165, 251–278. doi: 10.1016/0022-0981(93)90109-2
Hierl F., Wu H. C., Westphal H. (2021). Scleractinian Corals Incorporate Microplastic Particles: Identification From a Laboratory Study. Environ. Sci. pollut. Res. 28, 37882–37893. doi: 10.1007/s11356-021-13240-x
Hii Y. S., Soo C. L., Liew H. C. (2009). Feeding of Scleractinian Coral, Galaxea Fascicularis, on Artemia Salina Nauplii in Captivity. Aquac. Int. 17, 363–376. doi: 10.1007/s10499-008-9208-4
Hoegh-Guldberg O., Mumby P. J., Hooten A. J., Steneck R. S., Greenfield P., Gomez E., et al. (2007). Coral Reefs Under Rapid Climate Change and Ocean Acidification. Science 318, 1737–1742. doi: 10.1126/science.1152509
Houlbrèque F., Ferrier-Pagès C. (2009). Heterotrophy in Tropical Scleractinian Corals. Biol. Rev. 84, 1–17. doi: 10.1111/j.1469-185X.2008.00058.x
Huang W., Chen M., Song B., Deng J., Shen M., Chen Q., et al. (2021). Microplastics in the Coral Reefs and Their Potential Impacts on Corals: A Mini-Review. Sci. Tot. Environ. 762, 143112. doi: 10.1016/j.scitotenv.2020.143112
Hughes R. N. (1980). Optimal Foraging Theory in the Marine Context. Annu. Rev. Mar. Biol. Oceanogr. 18, 423–481. doi: 10.1007/BF02347099
Hughes A. D., Grottoli A. G. (2013). Heterotrophic Compensation: A Possible Mechanism for Resilience of Coral Reefs to Global Warming or a Sign of Prolonged Stress? PLoS One 8, e81172. doi: 10.1371/journal.pone.0081172
Isobe A., Iwasaki S., Uchida K., Tokai T. (2019). Abundance of non-Conservative Microplastics in the Upper Ocean From 1957 to 2066. Nat. Commun. 10, 417. doi: 10.1038/s41467-019-08316-9
Jiang S., Zhang Y., Feng L., He L., Zhou C., Hong P., et al. (2021). Comparison of Short- and Long-Term Toxicity of Microplastics With Different Chemical Constituents on Button Polyps. (Protopalythoa Sp.). ACS Earth Sp. Chem. 5, 12–22. doi: 10.1021/acsearthspacechem.0c00213
Kassambara A. (2021) Rstatix: Pipe-Friendly Framework for Basic Statistical Tests. Available at: https://cran.r-project.org/package=rstatix.
Kellogg V. L. (1906). A New Artemia and its Life Conditions. Science 24, 594–596. doi: 10.1126/science.24.619.594-b
Kesy K., Labrenz M., Scales B. S., Kreikemeyer B., Oberbeckmann S. (2020). Vibrio Colonization Is Highly Dynamic in Early Microplastic-Associated Biofilms as Well as on Field-Collected Microplastics. Microorganisms 9, 76. doi: 10.3390/microorganisms9010076
Krishnakumar S., Anbalagan S., Hussain S. M., Bharani R., Godson P. S., Srinivasalu S. (2021). Coral Annual Growth Band Impregnated Microplastics (Porites Sp.): A First Investigation Report. Wetl. Ecol. Manage. 9., 677–87 doi: 10.1007/s11273-021-09786-9
Lamb J. B., Willis B. L., Fiorenza E. A., Couch C. S., Howard R., Rader D. N., et al. (2018). Plastic Waste Associated With Disease on Coral Reefs. Sci. (80-. ). 359, 460–462. doi: 10.1126/science.aar3320
Lanctôt C. M., Bednarz V. N., Melvin S., Jacob H., Oberhaensli F., Swarzenski P. W., et al. (2020). Physiological Stress Response of the Scleractinian Coral Stylophora Pistillata Exposed to Polyethylene Microplastics. Environ. pollut. 263, 114559. doi: 10.1016/j.envpol.2020.114559
Law K. L., Thompson R. C. (2014). Microplastics in the Seas. Sci. (80-. ). 345, 144–145. doi: 10.1126/science.1254065
Martin C., Corona E., Mahadik G. A., Duarte C. M. (2019). Adhesion to Coral Surface as a Potential Sink for Marine Microplastics. Environ. pollut. 255, 113281. doi: 10.1016/j.envpol.2019.113281
Mills M. M., Lipschultz F., Sebens K. P. (2004). Particulate Matter Ingestion and Associated Nitrogen Uptake by Four Species of Scleractinian Corals. Cor. Reef. 23, 311–323. doi: 10.1007/s00338-004-0380-3
Mills M. M., Sebens K. P. (2004). Ingestion and Assimilation of Nitrogen From Benthic Sediments by Three Species of Coral. Mar. Biol. 145, 1097–1106. doi: 10.1007/s00227-004-1398-3
Mouchi V., Chapron L., Peru E., Pruski A. M., Meistertzheim A.-L., Vétion G., et al. (2019). Long-Term Aquaria Study Suggests Species-Specific Responses of Two Cold-Water Corals to Macro-and Microplastics Exposure. Environ. pollut. 253, 322–329. doi: 10.1016/j.envpol.2019.07.024
Murphy F., Quinn B. (2018). The Effects of Microplastic on Freshwater Hydra Attenuata Feeding, Morphology & Reproduction. Environ. pollut. 234, 487–494. doi: 10.1016/j.envpol.2017.11.029
Patterson J., Jeyasanta K. I., Sathish N., Edward J. K. P., Booth A. M. (2020). Microplastic and Heavy Metal Distributions in an Indian Coral Reef Ecosystem. Sci. Tot. Environ. 744, 140706. doi: 10.1016/j.scitotenv.2020.140706
Pupier C. A., Mies M., Fine M., Bastos Francini-Filho R., Pereira Brandini F., Zambotti-Villela L., et al. (2021). Lipid Biomarkers Reveal the Trophic Plasticity of Octocorals Along a Depth Gradient. Limnol. Oceanogr. 66, 2078–2087. doi: 10.1002/lno.11746
Rades M., Schubert P., Ziegler M., Kröckel M., Reichert J. (2022). Building plan for a temperature-controlled multi-point stirring incubator. protocols.io 10. doi: 10.17504/protocols.io.dm6gpb34dlzp/v1
R Core Team (2021) R: A Language and Environment for Statistical Computing. Available at: https://www.r-project.org/.
Reichert J., Arnold A. L., Hammer N., Miller I. B., Rades M., Schubert P., et al. (2021a). Reef-Building Corals Act as Long-Term Sink for Microplastic. Glob. Change Biol 28, 33–45. doi: 10.1111/gcb.15920
Reichert J., Arnold A. L., Hoogenboom M. O., Schubert P., Wilke T. (2019). Impacts of Microplastics on Growth and Health of Hermatypic Corals are Species-Specific. Environ. pollut. 254, 113074. doi: 10.1016/j.envpol.2019.113074
Reichert J., Schellenberg J., Schubert P., Wilke T. (2016). 3D Scanning as a Highly Precise, Reproducible, and Minimally Invasive Method for Surface Area and Volume Measurements of Scleractinian Corals. Limnol. Oceanogr. Methods 14, 518–526. doi: 10.1002/lom3.10109
Reichert J., Schellenberg J., Schubert P., Wilke T. (2018). Responses of Reef Building Corals to Microplastic Exposure. Environ. pollut. 237, 955–960. doi: 10.1016/j.envpol.2017.11.006
Reichert J., Tirpitz V., Anand R., Bach K., Knopp J., Schubert P., et al. (2021b). Interactive Effects of Microplastic Pollution and Heat Stress on Reef-Building Corals. Environ. pollut. 290, 118010. doi: 10.1016/j.envpol.2021.118010
Rotjan R. D., Sharp K. H., Gauthier A. E., Yelton R., Lopez E. M. B., Carilli J., et al. (2019). Patterns, Dynamics and Consequences of Microplastic Ingestion by the Temperate Coral, Astrangia Poculata. Proc. R. Soc B. Biol. Sci. 286, 20190726. doi: 10.1098/rspb.2019.0726
RStudio Team (2021) RStudio: Integrated Development Environment for R. Available at: http://www.rstudio.com/.
Rueden C. T., Schindelin J., Hiner M. C., DeZonia B. E., Walter A. E., Arena E. T., et al. (2017). ImageJ2: ImageJ for the Next Generation of Scientific Image Data. BMC Bioinf. 18, 1–26. doi: 10.1186/s12859-017-1934-z
Saliu F., Montano S., Garavaglia M. G., Lasagni M., Seveso D., Galli P. (2018). Microplastic and Charred Microplastic in the Faafu Atoll, Maldives. Mar. pollut. Bull. 136, 464–471. doi: 10.1016/j.marpolbul.2018.09.023
Saliu F., Montano S., Leoni B., Lasagni M., Galli P. (2019). Microplastics as a Threat to Coral Reef Environments: Detection of Phthalate Esters in Neuston and Scleractinian Corals From the Faafu Atoll, Maldives. Mar. pollut. Bull. 142, 234–241. doi: 10.1016/j.marpolbul.2019.03.043
Savinelli B., Vega Fernández T., Galasso N. M., D’Anna G., Pipitone C., Prada F., et al. (2020). Microplastics Impair the Feeding Performance of a Mediterranean Habitat-Forming Coral. Mar. Environ. Res. 155, 104887. doi: 10.1016/j.marenvres.2020.104887
Sebens K. P., Grace S. P., Helmuth B., Maney E. J., Miles J. S. (1998). Water Flow and Prey Capture by Three Scleractinian Corals, Madracis Mirabilis, Montastrea Cavernosa and Porites Porites in a Field Enclosure. Mar. Biol. 131, 347–360. doi: 10.1007/s002270050328
Stafford-Smith M. G. (1993). Sediment-Rejection Efficiency of 22 Species of Australian Scleractinian Corals. Mar. Biol. 115, 229–243. doi: 10.1007/BF00346340
Stafford-Smith M., Ormond R. (1992). Sediment-Rejection Mechanisms of 42 Species of Australian Scleractinian Corals. Mar. Freshw. Res. 43, 683. doi: 10.1071/MF9920683
Sturaro N., Hsieh Y. E., Chen Q., Wang P., Denis V. (2021). Trophic Plasticity of Mixotrophic Corals Under Contrasting Environments. Funct. Ecol. 35, 2841–2855. doi: 10.1111/1365-2435.13924
Tang J., Ni X., Zhou Z., Wang L., Lin S. (2018). Acute Microplastic Exposure Raises Stress Response and Suppresses Detoxification and Immune Capacities in the Scleractinian Coral Pocillopora Damicornis. Environ. pollut. 243, 66–74. doi: 10.1016/j.envpol.2018.08.045
Towle E. K., Enochs I. C., Langdon C. (2015). Threatened Caribbean Coral Is Able to Mitigate the Adverse Effects of Ocean Acidification on Calcification by Increasing Feeding Rate. PLoS One 10, e0123394. doi: 10.1371/journal.pone.0123394
Weideman E. A., Munro C., Perold V., Omardien A., Ryan P. G. (2020). Ingestion of Plastic Litter by the Sandy Anemone Bunodactis Reynaudi. Environ. pollut. 267, 115543. doi: 10.1016/j.envpol.2020.115543
Wright S. L., Rowe D., Thompson R. C., Galloway T. S. (2013). Microplastic Ingestion Decreases Energy Reserves in Marine Worms. Curr. Biol. 23, R1031–R1033. doi: 10.1016/j.cub.2013.10.068
Yin L., Chen B., Xia B., Shi X., Qu K. (2018). Polystyrene Microplastics Alter the Behavior, Energy Reserve and Nutritional Composition of Marine Jacopever (Sebastes Schlegelii). J. Haz. Mater. 360, 97–105. doi: 10.1016/j.jhazmat.2018.07.110
Keywords: coral reefs, feeding rate, ingestion, long-term exposure, acclimation
Citation: Rades M, Schubert P, Wilke T and Reichert J (2022) Reef-Building Corals Do Not Develop Adaptive Mechanisms to Better Cope With Microplastics. Front. Mar. Sci. 9:863187. doi: 10.3389/fmars.2022.863187
Received: 26 January 2022; Accepted: 25 May 2022;
Published: 29 June 2022.
Edited by:
Youji Wang, Shanghai Ocean University, ChinaReviewed by:
Daniel Rittschof, Duke University, United StatesAmilcar Leví Cupul-Magaña, University of Guadalajara, Mexico
Copyright © 2022 Rades, Schubert, Wilke and Reichert. This is an open-access article distributed under the terms of the Creative Commons Attribution License (CC BY). The use, distribution or reproduction in other forums is permitted, provided the original author(s) and the copyright owner(s) are credited and that the original publication in this journal is cited, in accordance with accepted academic practice. No use, distribution or reproduction is permitted which does not comply with these terms.
*Correspondence: Marvin Rades, c3Zlbi5tLnJhZGVzQGJpby51bmktZ2llc3Nlbi5kZQ==