- 1Key Laboratory of Sustainable Development of Marine Fisheries, Ministry of Agriculture and Rural Affairs, Yellow Sea Fisheries Research Institute, Chinese Academy of Fishery Sciences, Qingdao, China
- 2Laboratory for Marine Fisheries Science and Food Production Processes, Qingdao National Laboratory for Marine Science and Technology, Qingdao, China
- 3Benthic Resources, Institute of Marine Research, Bergen, Norway
Ocean acidification (OA) caused by elevated atmospheric CO2 concentration is predicted to have negative impacts on marine bivalves in aquaculture. However, to date, most of our knowledge is derived from short-term laboratory-based experiments, which are difficult to scale to real-world production. Therefore, field experiments, such as this study, are critical for improving ecological relevance. Due to the ability of seaweed to absorb dissolved carbon dioxide from the surrounding seawater through photosynthesis, seaweed has gained theoretical attention as a potential partner of bivalves in integrated aquaculture to help mitigate the adverse effects of OA. Consequently, this study investigates the impact of elevated pCO2 on the physiological responses of the Pacific oyster Crassostrea gigas in the presence and absence of kelp (Saccharina japonica) using in situ mesocosms. For 30 days, mesocosms were exposed to six treatments, consisting of two pCO2 treatments (500 and 900 μatm) combined with three biotic treatments (oyster alone, kelp alone, and integrated kelp and oyster aquaculture). Results showed that the clearance rate (CR) and scope for growth (SfG) of C. gigas were significantly reduced by elevated pCO2, whereas respiration rates (MO2) and ammonium excretion rates (ER) were significantly increased. However, food absorption efficiency (AE) was not significantly affected by elevated pCO2. The presence of S. japonica changed the daytime pHNBS of experimental units by ~0.16 units in the elevated pCO2 treatment. As a consequence, CR and SfG significantly increased and MO2 and ER decreased compared to C. gigas exposed to elevated pCO2 without S. japonica. These findings indicate that the presence of S. japonica in integrated aquaculture may help shield C. gigas from the negative effects of elevated seawater pCO2.
Introduction
Ocean acidification (OA) caused by elevated concentrations of atmospheric CO2 is one of the most serious environmental issues facing the world in the 21st century. Multiple studies have indicated that this more acidic oceanic environment will have negative consequences for marine life, such as decreasing calcification rates and impairing feeding, respiration, and physiological energetics (Gazeau et al., 2007; Rastrick et al., 2018a; Jiang et al., 2021). Damage to marine organisms by OA is likely to alter coastal biodiversity, ecosystem functioning and services, and global marine harvests (Cooley et al., 2009; Barry et al., 2011). Calcifying marine organisms such as molluscs, crustaceans, corals, and planktonic calcifies are predicted to be particularly sensitive due to the additional costs associated with calcification and maintenance of calcified structures (Fabry et al., 2008; Hofmann et al., 2010; Findlay et al., 2011; Rastrick et al., 2014). Marine bivalves are calcifying ecosystem engineers, which are important in ecosystem functioning and aquaculture (Cranford et al., 2012). In accordance with their ecological and commercial relevance, the interest in OA research on bivalves is growing steadily and has increased particularly in recent years (e.g., Berge et al., 2006; Cummings et al., 2011; Li et al., 2017; Zhao et al., 2017; Rastrick et al., 2018a; Tan and Zheng, 2019). Evidence from several previous studies suggests that elevated seawater pCO2 affects calcification (e.g., Ries et al., 2009; Melzner et al., 2011), growth (e.g., Thomsen et al., 2010; Kroeker et al., 2013), burrowing behavior (e.g., Clements et al., 2015; Peng et al., 2017), energetics (e.g., Wang et al., 2015; Zhao et al., 2017; Rastrick et al., 2018a) and immune response (e.g., Bibby et al., 2008; Zha et al., 2017) in bivalves such as oysters, mussels, and clams. However, much of the published information on bivalves comes from short-term laboratory-based manipulative experiments, which reduces the generalizability of results to real-world situations. With the increasing demand for improving the ecological validity of OA studies, it is necessary to scale up the methodology from laboratory- to field-level (Riebesell et al., 2008; Rastrick et al., 2018b).
The Pacific oyster, Crassostrea gigas, is one of the most important ecologically and economically cultured bivalves worldwide, with a global mariculture production of 643 thousand tons in 2018 (FAO, 2020). The effects of OA on the Pacific oyster have been well documented. Previous studies show that early developmental stages of oysters are more susceptible to increased seawater pCO2 (Kurihara et al., 2007; Gazeau et al., 2011). (Barton et al. 2012; 2015) reported that larval production and mid-stage growth (~120 to ~150 mm) of C. gigas showed a significantly negative correlation to naturally elevated carbon dioxide levels and clearly linked increased CO2 to the cause of severe loss of production at the Whiskey Creek Shellfish Hatchery on the Oregon coast. The calcification rates of C. gigas have been predicted to decline by 10% following the IPCC IS92a scenario (~740 ppmv in 2,100) (Gazeau et al., 2007). Negative impacts of elevated pCO2 to C. gigas acid–base status and immune response have also been reported by Lannig et al. (2010) and Wang et al. (2016). Despite increased understanding of the potential impacts of OA on Pacific oysters, studies regarding the physiological effects of OA on C. gigas under natural systems are scarce. Furthermore, there is still a lack of effective ways for the oyster aquaculture industry to help mitigate the potential negative impacts of OA.
Previous studies have postulated that Integrated Multi-Trophic Aquaculture (IMTA) may be a possible and sustainable solution to help mitigate some of the effects of climate change on production (Clements and Chopin, 2016; Tan and Zheng, 2020). The term “IMTA” refers to the combined aquaculture of various organisms at different trophic levels with complementary ecosystem functions in which the wastes and by-products from one species serve as the food source for one or a number of other species (Chopin, 2013). The seaweed aquaculture sector may be one of the key components of IMTA systems (Chopin, 2014). Seaweed within IMTA is traditionally regarded as a source of nutrient removal from the seawater of the nearby bivalve farms and plays a role in the bioremediation and ecological regulation of the aquaculture environment. Seaweed also has the ability to add oxygen and absorb dissolved carbon dioxide from the surrounding seawater through photosynthesis, producing biomass and contributing to carbon sequestration. It has been reported that ~0.34 million t carbon is removed from the coastal ecosystems each year by seaweed harvesting (Tang et al., 2011). The sea–air CO2 flux is enhanced by seaweed aquaculture (Jiang et al., 2013). Bay scale dissolved inorganic carbon (DIC) budget research showed that the annual DIC uptake by seaweed Saccharina japonica and Gracilaria lemaneiformis was about 1.0 × 105 t in Sanggou Bay, which drove the bay as a net DIC sink (Jiang et al., 2015). By sequestering carbon dioxide dissolved in seawater, seaweed plays a potential role in reducing OA and its biological effects on a local scale. An interesting study on the effect of dissolved CO2 levels (0, 100, 200, 250, and 300 ppm) on the dissolution rate of the outer shells of dead mollusks and spines of sea urchins in the presence and absence of seaweed, Chaetomorpha antennina, showed that the weight loss of the samples was curbed when seaweed was introduced (Kaladharan et al., 2019). In the coastal regions of southern Korea, an innovative research approach called the Coastal CO2 Removal Belt (CCRB) has established a pilot farm of perennial brown alga, Eklonia calva, which sequesters ~10 t of CO2 per ha per year (Chung et al., 2013). Jiang et al. (2014) carried out a mesocosm experiment to monitor the function of seaweed G. lemaneiformis in eliminating dissolved inorganic carbon released from the calcification and respiration processes of the scallop Chlamys farreri. The results showed that seaweed and bivalve integrated aquaculture practice can not only effectively balance the seawater carbonate system but also buffer acidification. However, although the ecological and economic benefits of IMTA have been highlighted, the role of seaweed within IMTA in mitigating the impacts of OA on bivalves (e.g., physiological activities, energy allocation and growth, etc.) needs further investigation, especially within natural systems.
The goal of this research was to examine: (1) whether the physiological processes and net energy balance of C. gigas were affected after medium-term exposure (30 days) to elevated pCO2 and (2) whether the presence of S. japonica could help mitigate these biological effects of elevated pCO2 on C. gigas within a natural system.
Materials and Methods
Animal Collection and Acclimatization
Experimental oysters (56.37 ± 8.20 mm shell length, 29.67 ± 3.91 g wet weight) and kelps (70–80 cm length) were collected on 7 May 2017 from a large-scale commercial aquaculture area (37°03′52.17″N, 122°33′11.54″E) in Sanggou Bay, Yellow Sea, China. All specimens were transported on ice to a small semi-enclosed harbor (37°02′14.71″N, 122°33′02.09″E; area 7900 m2; mean depth 1.5 m). The oysters were cleaned and maintained in flowing seawater in in situ mesocosms at ambient conditions (temperature ~19.0°C, salinity ~32, and seawater pHNBS ~8.10) for one week before the start of the experiment. One hundred and thirty healthy oysters were selected and individually labeled with a plastic-laminated number on the upper valve.
Experimental Setup and Procedure
Experiments were conducted using in situ mesocosms with six treatments: three biotic treatments (namely “oyster alone”, “kelp alone,” and “integrated aquaculture”), crossed with two pCO2 treatments (ambient, ~500 μatm and elevated, ~900 μatm). Each of the six treatments was replicated three times, for a total of 18 mesocosms. The mesocosms were suspended from a floating platform. Each sealed mesocosm was made of translucent polyethylene and was cylinder-shaped (0.5 m × 1.2 m, diameter × height) (Figure 1). Inlet and outlet valves allow for the control of seawater flow through each mesocosm. The top of each mesocosm was immersed to a depth of ~30 cm with the inlet and outlet pipes (Φ ~3 cm) extending ~50 cm above the water surface. The inlet pipe extended to the bottom of each mesocosm, allowing water mixing and preventing gradients from forming within the mesocosm.
Elevated pCO2 treatment was controlled by enriching natural seawater with CO2 (from a CO2 gas cylinder) in a submerged 1,000 L reservoir tank suspended from the same floating platform as the mesocosms (modified from Rastrick et al., 2014; Jiang et al., 2019; Jiang et al., 2021). The flow of pure CO2 to the reservoir tank was controlled via a pH controller (Aqua Medic GmbH, Germany) by switching on or off a valve when pH readings in the tank deviated from the predetermined set-points by ±0.05 pH units. The acidified seawater was then pumped to each elevated pCO2 mesocosm via flow rate controllers (60 L h−1). As the CO2-adjusted seawater was pumped out of the reservoir tank, it was replaced with natural ambient seawater using an automatic pump triggered by changes in volume within the tank. This gave a seawater residence time in the reservoir tank of just under an hour. Pumps in the reservoir tank prevented the formation of CO2 or temperature gradients, and the ambient temperature was maintained due to the submersion of the reservoir tank and the mesocosms.
Each “oyster alone” treatment contained 8 oysters hugged along a rope tied at the top of the mesocosm to simulate farm conditions. The kelp alone treatment contained 1 blade with holdfasts (70–80 cm length) that were tied at the top of the mesocosm to keep the orientation vertical as in the farm environment. An integrated aquaculture treatment contained both oysters (n = 8) and kelp (n = 1) hung in the same way. The oyster number and the ratio of oyster and kelp were determined based on our modeling research which was published in Lin et al. (2020). The experiment started on the 14 May 2017 (experiment day 1) and continued until 12 June 2017.
Monitoring of the Key Parameters of Seawater
During the 30-day experiment, seawater temperature, salinity, and dissolved oxygen concentration (DO) in each mesocosm were measured three times a day. The pH in the reservoir tank was also measured three times a day, and the pH in the inflow and outflow of each mesocosm was measured only at 12:00 daily. Seawater temperature, salinity, and dissolved oxygen concentration (DO) were measured using a Vernier’s LabQuest® 2 (Vernier Software & Technology, USA) equipped with the corresponding probes. The pH level was measured using a commercial combination electrode (Ross type, Orion) calibrated daily using a 3-point calibration procedure on the U.S. National Bureau of Standards (NBS) scale with a precision of ± 0.001 pH units. The concentration of chlorophyll-a in the ambient seawater was continuously monitored using the ACLW-RS chlorophyll turbidity temperature sensor (ALEC Electronics Co., Ltd., Japan) with a precision of ±0.1 μg L−1. Total alkalinity (AT) was analysed weekly via a Metrohm 848 Titrino plus automatic titrator (Metrohm, USA) on 100 ml of GF/F filtered seawater with an accuracy of ±5 mmol L−1. Carbonate system parameters, such as total dissolved inorganic carbon, aqueous partial pressure of CO2 (pCO2), the CaCO3 saturation state for calcite (Ωcal), and aragonite (Ωarag), were calculated from pHNBS and AT by the CO2SYS Package (Pierrot et al., 2006) using the dissociation constants of Mehrbach et al. (1973) and refit by Dickson and Millero (1987). No mortality of oysters was observed in both ambient and elevated pCO2 treatments throughout the experiment.
Physiological Measurements
The physiological measurements of oysters under different treatments were performed on 12 June 2017 (experiment day 30) by flow-through system after Jiang et al. (2017). Feeding experiments were performed by transferring 9 labeled individual oysters (3 randomly selected from each of the 3 replicate mesocosms per treatment) to individual flow-through chambers which were continuously supplied with the same water as supplied to the respective treatment mesocosms. The flow rates of each chamber were adjusted to 180–200 ml min−1 and determined by simultaneously measuring the volume of water collected from each outflow. Three identical chambers without oysters served as the control. The oysters were left undisturbed in chambers for 1 h to resume feeding before sampling water from the outlet of the chambers. The particle number in each chamber was measured by PAMAS S4031GO particle counter equipped with a tube of 50 μm aperture and set to count all particles >2 μm diameter in a 0.5 ml sub-sample. Three replicate counts are made on each sample and the mean calculated. This measurement was repeated at 60 min intervals over a period of 3 h. Clearance rate (CR) is then calculated as follows:
where C1 is the particulate concentration in control chamber (particle number ml−1), and C0 is the particulate concentration from each experimental chamber (particle number ml−1).
The absorption efficiency (AE) was determined using the following equation (Conover, 1966):
Where F represents the ash-free dry weight: dry weight ratio of food, and E represents the ash-free dry weight: dry weight ratio of the feces. Approximately 4 L of food samples were taken from the seawater being supplied to each treatment, and 4 sub-samples were filtered with pre-ashed and weighted glass fiber filters of 1.2 μm (Whatman GF/C 47mm). These filters were rinsed with isotonic ammonium formate (3%) to remove salts and dried to a constant weight (~48 h) at 65°C and weighed (W60), then ashed at 450°C for 6 h to get the ash-free dry weight W450. The particulate organic matter concentration (POM, mg L−1) is calculated by POM = (W60 − W450)/v. Each oyster was placed in the individual feces collector at the respective treatment conditions for ~24 h after the CR measurements. The feces collector (Φ ~10 cm) was made of the casing pipe equipped with an 80 μm mesh screen (after, Rastrick et al., 2018a). Feces were collected by a pipette and filtered with the organic content of the feces measured as described above.
The respiration rate (MO2) of individual oysters was measured in ~200 ml stop-flow glass respirometers held in a water bath at the same ambient temperature as the mesocosms and supplied with the same amount of water. Twelve respirometers were run simultaneously, with three chambers without oysters being used as controls. The inflow of each respirometer came from corresponding treatments, and constant flow rates were maintained by a multichannel peristaltic pump. Thirty minutes were allowed for the oysters to recover from handling stress, open and resume pumping, then the flow of water was stopped and MO2 was measured over the next 90 min. The oxygen concentration in each respirometer was not allowed to drop below 70% saturation throughout the experiment. The rate of decline in oxygen saturation in each respirometer was measured by a calibrated optical oxygen probe connected to a Vernier’s LabQuest® 2 (Vernier Software & Technology, USA). The initial and final oxygen concentrations in each respirometer were measured. The respiration rate (MO2) was then calculated using the following equation:
Where t0 and t1 represent the initial and finish times (min) of the measurement period, Ct represents the concentration of oxygen in the water (μmol O2 L−1) at time t; Vr represents the volume of the respirometer minus the animal.
After MO2 measurement, the ammonia excretion rate (ER) of the same group of oysters was measured. Water samples were taken from each respirometer, filtered by 0.45 μm cellulose acetate membrane filters, and then analysed for ammonia using the phenol-hypochlorite method. ER was calculated using the following equation:
Where Ctest and Ccontrol represent the ammonia concentration in the sample and control, respectively, Vr represents the volume of the respirometer minus the animal; t is the exposure time.
After all the physiological measurements, the oyster tissues were dissected from the shell and dried to a constant weight at 65°C. The CR, MO2, and ER were then corrected to a ‘standard body size’ of 1 g of dry weight mass-specific rates using the following equation: Ys = (Ws/We)b × Ye, where Ys is the physiological rate for an animal of standard weight, Ws is the standard weight (1 g), We is the observed weight of the animal (g), Ye is the uncorrected (measured) physiological rate, and b is the weight exponent for the physiological rate function (b = 0.67). Each physiological rate was then converted into energy equivalents (J h−1) and used to calculate the energy available for scope for growth (SfG). Values for MO2 were transformed into J h−1 using a conversion factor of 0.456 J μmol−1 O2 (Gnaiger, 1983), ER was converted to J h−1 using the conversion factor: 1 μmol NH4-N h−1 = 0.349 J h−1. SfG (J h−1 g−1) was calculated by SfG = Ab − R − U, where Ab is the total absorbed energy (J h−1 g−1), R is the energy lost in respiration (J h−1 g−1), and U is the energy lost in ammonia excretion (J h−1 g−1). Ab = CR (L g−1 h−1) × (mg POM L−1) × AE (%). The POM concentration was converted to joules using a conversion factor of 23 J mg−1 ash-free dry weight (Widdows and Johnson, 1988).
Specific Growth Rate
The wet weight-specific growth rate (SGR, % d−1) of each oyster was measured as follows: SGR = (ln(Wt/W0)/t) × 100%, where Wt and W0 are the wet weight (g) of the oyster at time t and at time 0, respectively, and t is the sampling time (d).
Data Analysis
Statistical analyses were conducted using the software SPSS 22.0 for Windows. The general linear mixed model (GLMM) was used to test the effects of culture treatments (oyster alone and integrated aquaculture) or pCO2 treatments (ambient, ~500 μatm and elevated, ~900 μatm) on physiological parameters with a mesocosm as a random variable nested within fixed factors (culture or pCO2 treatments). This design considers that 3 replicate mesocosms in each treatment were supplied with the same seawater, and therefore, mesocosms may not be considered true replicates. Differences between culture or pCO2 treatments were further tested by F-tests with a significance threshold of α = 0.05. All data are represented as means ± SD.
Results
Seawater Carbonate Chemistry
The daily variations in temperature, salinity, and pH during the experiment are shown in Figure 2. The ambient temperature and salinity were 19.08 ± 1.27°C and 32.86 ± 0.23, respectively. The controlled injection of CO2 separates the treatments successfully (pHNBS ~7.83 in elevated pCO2 treatments and 8.07 ± 0.03 in the ambient treatment) and keeps them relatively stable during the 30 days of exposure. Seawater chemistry variables over the 30-day experimental period for each pCO2 treatment are summarized in Table 1. There was no significant difference in temperature, salinity, chlorophyll a, DIC, Ωcal, Ωarag, and between treatments (P >0.05). The saturation percentage of dissolved oxygen in the exposure containers consistently exceeded 100% and was similar across treatments during the experiment. There was a significant difference between pHNBS and pCO2 between treatments (P <0.05).
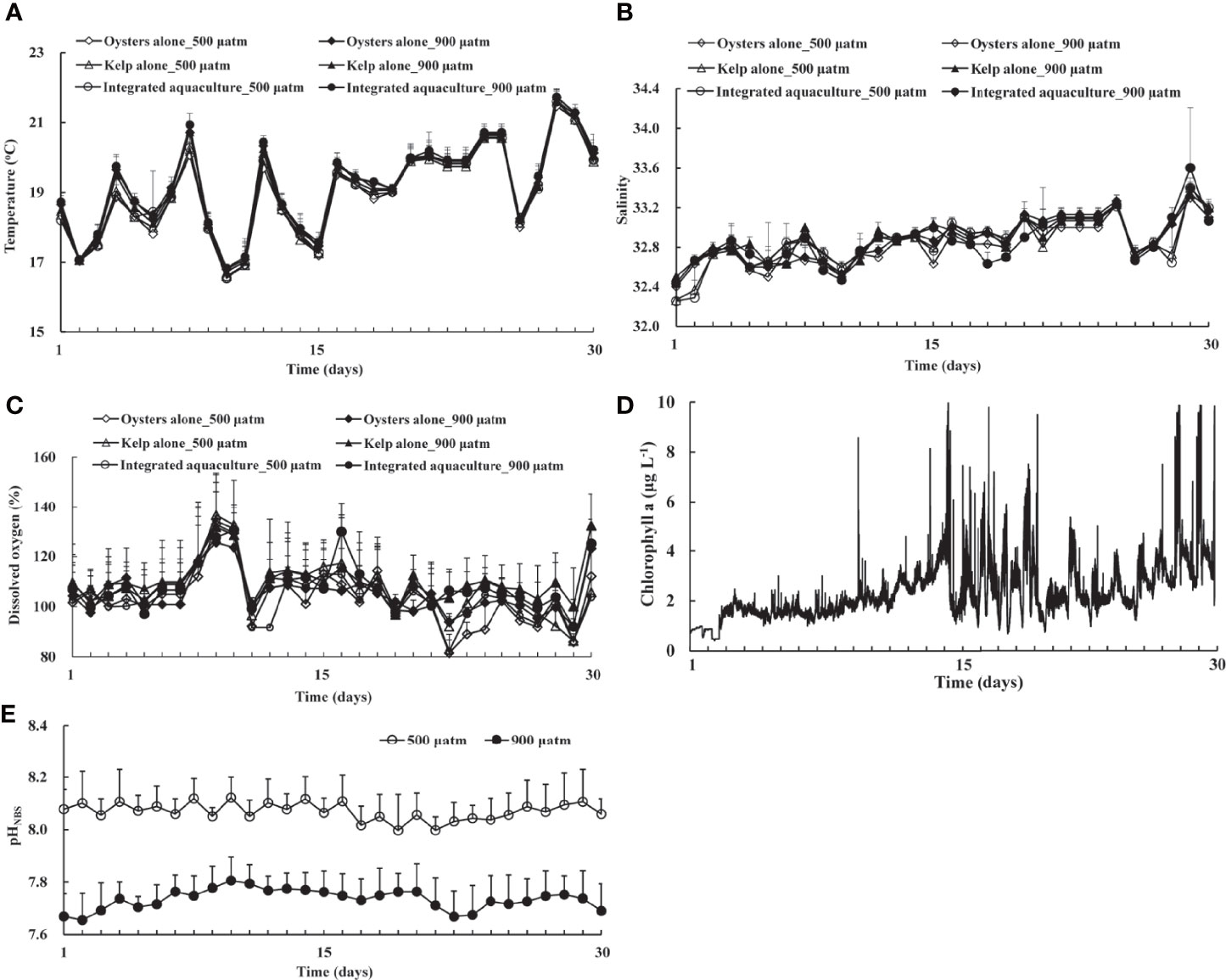
Figure 2 Daily variations of environmental conditions during the 30-day exposure from 14 May to 12 June 2017. (A) Temperature; (B) salinity; (C) dissolved oxygen; (D) chlorophyll a in the ambient seawater; and (E) pHNBS in the inflow of experimental units across the treatments. Means ± SD are shown.
The average change in daytime pHNBS between the inflow and outflow of the mesocosms for the different treatments during the 30 days of exposure is shown in Figure 3. Results showed that the seaweed alone treatment increased the daytime pHNBS by ~0.08 units, while oyster alone decreased ~0.05 units compared to the inflow under ambient conditions. The magnitude of change in daytime pHNBS was significantly greater in kelp alone treatment under elevated pCO2 condition than the ambient pCO2 condition. The kelp alone treatment increased daytime pHNBS by ~0.11 units, while the oyster and kelp integrated aquaculture treatment increased the daytime pHNBS by ~0.06 units.
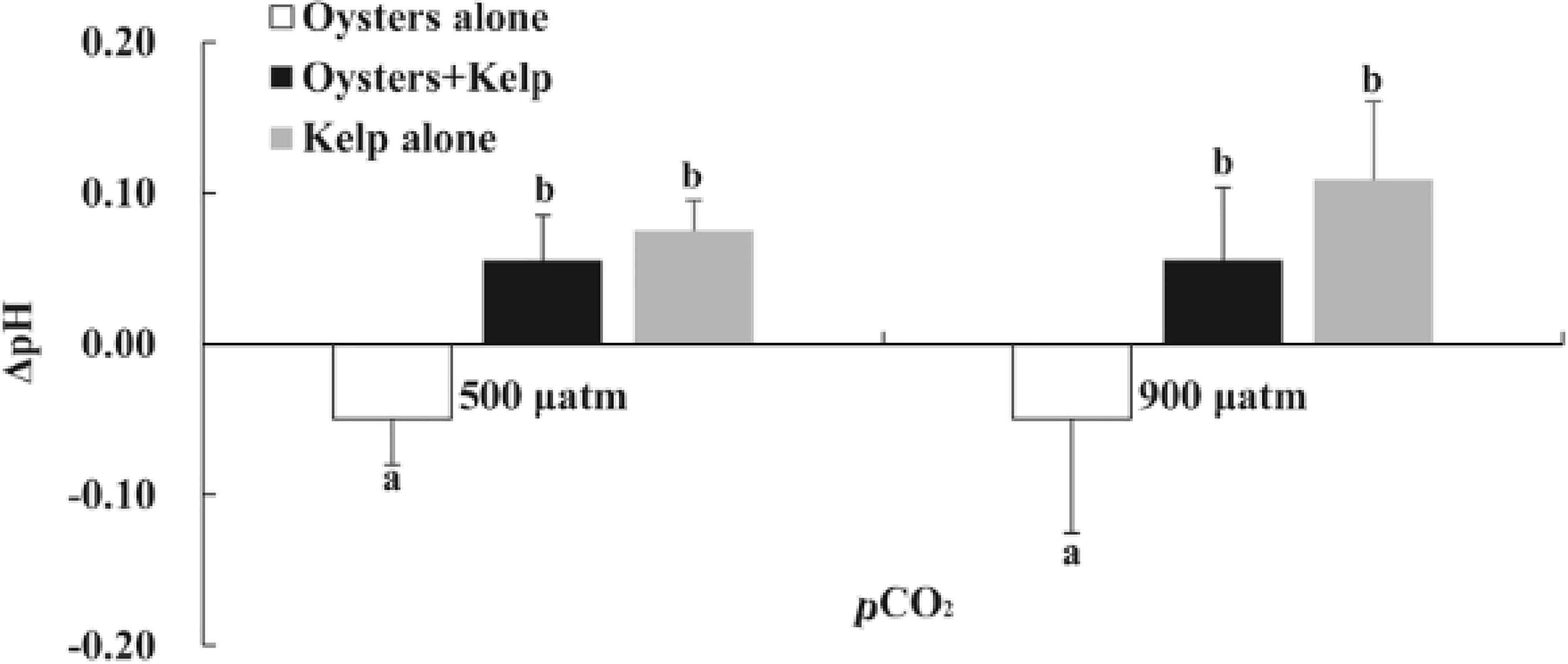
Figure 3 Average change in daytime pHNBS in the inflow and outflow of experimental units across the treatments during the 30 days. Means ± SD are presented. Different letters indicate significant variation between culture treatments (P < 0.05).
Clearance Rate and Absorption Efficiency
A significant effect of elevated pCO2 on the clearance rate of oysters was observed. After 30 days of exposure, the clearance rate of oysters was significantly reduced in the elevated pCO2 treatments and in the absence of kelp, as compared with ambient pCO2 treatments (P <0.05) (Figure 4A). The presence of kelp in elevated pCO2 treatment increased the clearance rate of oysters compared with oysters alone treated at the same elevated pCO2 level (P <0.01). Additionally, no significant difference in CR was found between oysters incubated with seaweed at elevated pCO2 and oysters incubated at ambient pCO2 levels (P >0.05).
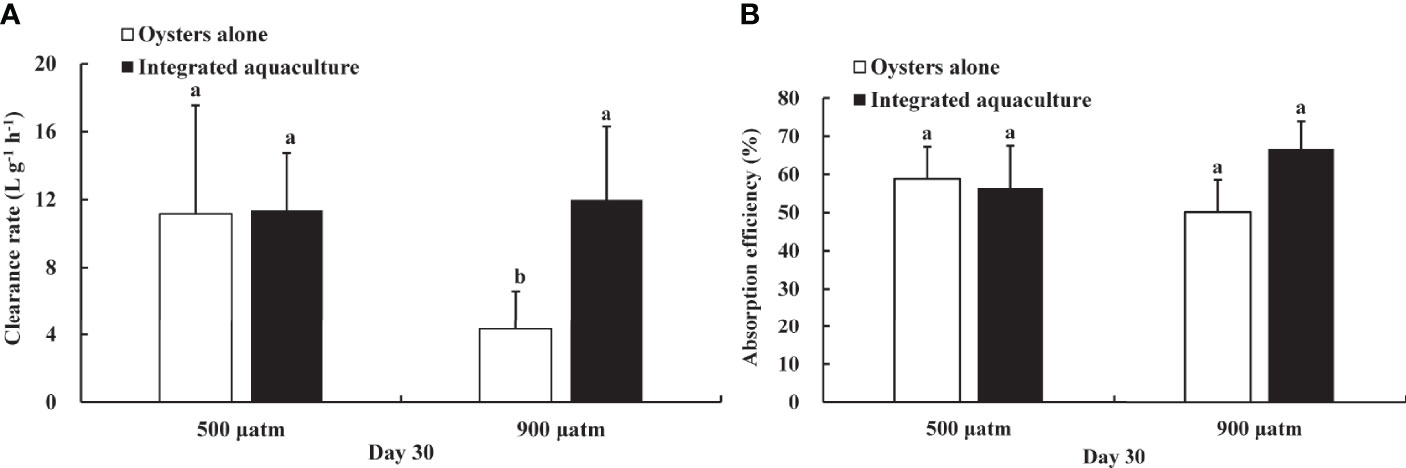
Figure 4 Clearance rate (A) and absorption efficiency (B) of C. gigas exposed to different treatments for 30 days. Means ± SD are shown. Different letters indicate significant variation between treatments (P < 0.05).
Absorption efficiency varied between 41 and 72% and was not affected significantly by elevated pCO2 during the experiment. The absorption efficiency was lower in the oyster alone treatment at elevated pCO2 treatment but showed no significant difference from the integrated aquaculture treatment (P >0.05) (Figure 4B).
Respiration Rate and Ammonia Excretion Rate
After 30 days of exposure, the respiration rate of oysters was significantly higher in the absence of kelp at elevated pCO2 compared with oysters in ambient pCO2 treatments (P <0.05). The presence of kelp in elevated pCO2 treatment lowered the respiration rate and ammonia excretion rate of oysters compared to ambient conditions, but no significant difference was found (P >0.05). Compared with all other treatments, the respiration rate and ammonia excretion rate were significantly increased in the oyster alone treatment (P <0.05) (Figure 5).
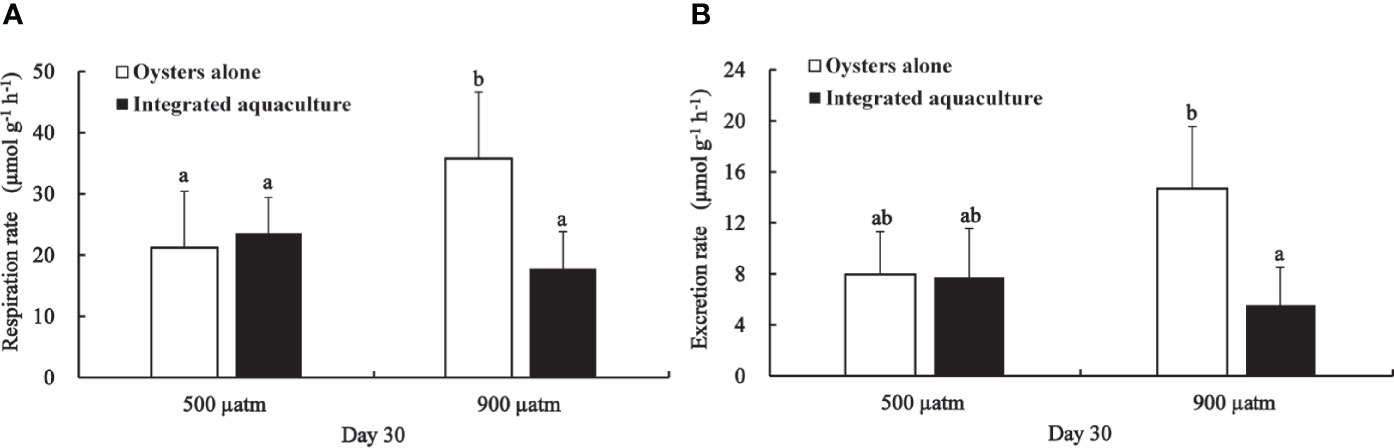
Figure 5 Respiration rate (A) and ammonia excretion rate (B) of C. gigas exposed to different treatments for 30 days. Means ± SD are shown. Different letters indicate significant variation between treatments (P < 0.05).
Scope for Growth and Specific Growth Rate
Elevated pCO2 exposure significantly reduced the SfG of C. gigas when unintegrated with kelp (Figure 6A). Compared to other treatments, SfG was negative (−9.45 J g−1 h−1) in the elevated pCO2 treatment in the absence of kelp (P <0.01). However, in the elevated pCO2 treatment in the presence of kelp, SfG (35.82 J g−1 h−1) was not significantly different to that of oysters in ambient pCO2 treatments (P >0.05). Moreover, after 30 days of exposure, the wet weight-specific growth rates of C. gigas were also observed to be dramatically reduced in the oyster alone treatment compared with the integrated aquaculture treatment at elevated pCO2 levels (Figure 6B).
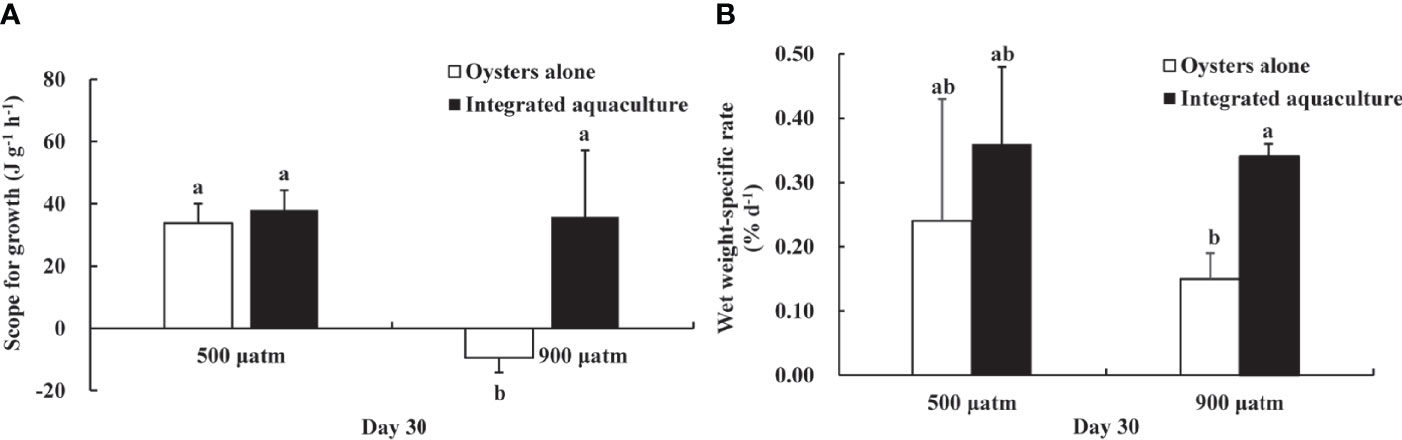
Figure 6 Scope for growth (A) and wet weight-specific rate (B) of C. gigas exposed to different treatments for 30 days. Means ± SD are shown. Different letters indicate significant variation between treatments (P < 0.05).
Discussion
The field mesocosms used in this study followed fluctuations in the natural environment, with CO2 controlled to give two natural but distinct treatments, maintaining a difference of about 0.25 pH units over time (Figure 2). Using this novel approach, our results indicate that medium-term elevated pCO2 exposure reduces the CR of Pacific oyster, C. gigas, while increasing the MO2 and ER, reducing energy available for growth. Importantly, the presence of kelp, S. japonica, in the mesocosms mitigates this negative physiological impact of OA on oysters.
CR is generally considered the most sensitive parameter for the feeding activity and energy acquisition of bivalves. In this study, elevated pCO2 induced a reduction in CR, limiting the capacity of energy acquisition from food sources. Zhao et al. (2017) and Xu et al. (2016) also demonstrated reductions of CR by the blood clam Tegillarca granosa and Manila clam R. philippinarum under similar acidified conditions. The gills are an important organ serving both a filter-feeding and respiration function in bivalves. The accumulation of GABA in the gill under elevated pCO2 conditions may be a potential explanation for the suppression of feeding activity (Jiang et al., 2019). However, Liu and He (2012) reported that CR of pearl oyster Pinctada fucata increased at extreme pH reductions of up to 0.7 units, and Sui et al. (2016) found that the physiological energetics of thick shell mussel Mytilus coruscus in terms of feeding, absorption and ultimately the energy available for growth remained unchanged by low pH conditions, perhaps due to the species-specific responses or the different experimental methods.
Here, AE was also not affected by acidified conditions, indicating the stable function of the digestive system, at least under the specific conditions and exposure time of this study. However, changes in CR, combined with increased MO2 and ER in response to elevated pCO2, resulted in a significant deficit in energy supply for C. gigas and ultimately less energy available for growth. For the mariculture industry, the significant reduction in energy available for growth (SfG) and actual growth in C. gigas under elevated pCO2 represents a potential challenge to oyster farming operations, which could lead to economic loss. Particularly during the season of this study, when spat is first introduced to the farm environment and when most growth occurs.
The wide variety of species and exposure-dependent responses reported for bivalves to elevated pCO2 (Navarro et al., 2013; Sui et al., 2016; Wang et al., 2016) may, in part, be due to differences in methodologies (e.g., measurement of CR via static or flow-through systems), which makes it difficult to compare results between studies. Most studies to date are also laboratory-based experiments, making it difficult to scale responses to the ecosystem level. Given the complexity of natural environments (such as food source and availability, daily fluctuation of environmental factors, etc.), particularly in coastal areas where bivalve aquaculture occurs, some recent studies, as here, have used field mesocosms (Jiang et al., 2019; Jiang et al., 2021) to advance the use of field experiments and improve the ecological relevance of data collected (e.g., Rastrick et al., 2018b).
Our results indicated that the presence of S. japonica under farm conditions helped to mitigate the negative effects of elevated pCO2 on C. gigas, at least at the mesocosm scale. Being highly effective autotrophic, S. japonica use dissolved CO2 in the seawater as the primary carbon source for photosynthesis. The rate of S. japonica photosynthetic carbon fixation was reported to 30 μmol g−1 h−1 (Han, 2013; Xu et al., 2016). Consequently, it may benefit from a future elevation in pCO2. For example, Xu et al. (2018) reported that projected pCO2 (1,000 μatm in 2,100 under RCP 8.5) may increase the growth of S. japonica. The increased photosynthesis rates of S. japonica improve the growing conditions for nearby C. gigas by removing sufficient amounts of CO2, reducing local seawater acidification, increasing pH, and providing dissolved oxygen at the local scale. Giving the CO2 released from the calcification and respiration processes of bivalves into account (Chauvaud et al., 2003; Mistri and Munari, 2013), S. japonica may also alleviate the potential self-acidification risk caused by C. gigas itself in smaller or more enclosed aquaculture settings. Here, S. japonica increased the mean daytime pH of the seawater by ~0.11 units in the elevated pCO2 treatment (Figure 3). Such biogenic pH fluctuations are important to the function of bivalves. Wahl et al. (2018) demonstrated that the blue mussel M. edulis can take advantage of these fluctuations by shifting most of their costly physiological activities to the daytime when the surrounding chemical conditions are more favorable. Microprofiling studies have shown that kelp E. radiata can modify the chemical environment in a micro-zone called the diffusive boundary layer (DBL), and DBL microenvironments at the blade surface may create potential refuges from OA for calcifying epifauna (Noisette and Hurd, 2018).
By using a novel in situ field mesocosm approach, this study demonstrates that elevated pCO2 can negatively affect the physiological energetics of C. gigas. Exposure to elevated pCO2 significantly reduced CR while increasing MO2 and ER, thereby reducing the energy allocated for reproduction and growth, presumably affecting production. However, similar energetic responses between C. gigas incubated at ambient CO2 levels and those incubated at elevated CO2 levels in the presence of S. japonica indicate that in integrated aquaculture systems, S. japonica may benefit the production of C. gigas by, in part, mitigating the negative physiological impact of elevated pCO2. However, further in situ investigations are needed to elucidate the mutual benefit mechanisms and quantify context-dependency, particularly at larger farm scales.
Data Availability Statement
The original contributions presented in the study are included in the article/Supplementary Material. Further inquiries can be directed to the corresponding author.
Author Contributions
ZJ: Conceptualization, methodology, writing—original draft, writing—review & editing, and funding acquisition. WJ: Investigation, data curation, formal analysis, writing—original draft, and writing—review & editing. SR: Conceptualization, methodology, investigation, and writing–review & editing. XW, JinF, MD, and YG: Investigation and formal analysis. ØS and JiaF: Supervision, resources, funding acquisition, and writing—review & editing. All authors listed have made a substantial, direct, and intellectual contribution to the work and approved it for publication.
Funding
This work was supported by grants from the Marine S&T Fund of Shandong Province for the Pilot National Laboratory for Marine Science and Technology (Qingdao) (2022QNLM040003-4), the Central Public-interest Scientific Institution Basal Research Fund, CAFS (2020TD50), the Young Taishan Scholars Program of Shandong Province (tsqn201909166), the Natural Science Foundation of Shandong Province (ZR2021QD035, ZR202102210486), the National Key R&D Program of China (2019YFE0103800), the Sino-Norway international collaboration project Environment and Aquaculture Governance (MFA, CHN 2152), and the China Agriculture Research System of MOF and MARA.
Conflict of Interest
The authors declare that the research was conducted in the absence of any commercial or financial relationships that could be construed as a potential conflict of interest.
Publisher’s Note
All claims expressed in this article are solely those of the authors and do not necessarily represent those of their affiliated organizations, or those of the publisher, the editors and the reviewers. Any product that may be evaluated in this article, or claim that may be made by its manufacturer, is not guaranteed or endorsed by the publisher.
Acknowledgments
The authors thank Mr. Junwei Wang, Senlin Wang, and Yitao Zhang of Chudao Fisheries Corporation for their assistance throughout the field experiment. The authors are also grateful for several anonymous reviewers whose constructive criticism improved the quality of this manuscript.
Supplementary Material
The Supplementary Material for this article can be found online at: https://www.frontiersin.org/articles/10.3389/fmars.2022.862172/full#supplementary-material
References
Barry J. P., Widdicombe S., Hall-Spencer J. M. (2011) “Effect of Ocean Acidification on Marine Biodiversity and Ecosystem Function,” in Ocean Acidific. Eds. Gattuso J. P., Hansson L. (Oxford: Oxford University Press), 192–209.
Barton A., Hales B., Waldbusser G. G., Langdon C., Feely R. A. (2012). The Pacific Oysters, Crassostrea Gigas, Shows Negative Correlation to Naturally Elevated Carbon Dioxide Levels: Implications for Near-Term Ocean Acidification Effects. Limnol. Oceanog. 57, 698–710. doi: 10.4319/lo.2012.57.3.0698
Barton A., Waldbusser G. G., Feely R. A., Weisberg S. B., Newton J. A., Hales B., et al. (2015). Impacts of Coastal Acidification on the Pacific Northwest Shellfish Industry and Adaptation Strategies Implemented in Response. Oceanography 28, 146–159. doi: 10.2307/24861877
Berge J. A., Bjerkeng B., Pettersen O., Schaanning M. T., Oxnevad S. (2006). Effects of Increased Sea Water Concentrations of CO2 on Growth of the Bivalve Mytilus Edulis L. Chemosphere 62, 681–687. doi: 10.1016/j.chemosphere.2005.04.111
Bibby R., Widdicombe S., Parry H., Spicer J., Pipe R. (2008). Effects of Ocean Acidification on the Immune Response of the Blue Mussel Mytilus Edulis. Aquat. Biol. 2, 67–74. doi: 10.3354/ab00037
Chauvaud L., Thompson J. K., Cloern J. E., Thouzeau G. (2003). Clams as CO2 Generators: The Potamocorbula Amurensis Example in San Francisco Bay. Limnol. Oceanog. 48, 2086–2092. doi: 10.4319/lo.2003.48.6.2086
Chopin T. (2013). “Aquaculture, Integrated Multi-Trophic,” in Encyclopedia of Sustainability Science and Technology. Ed. Myers R. A. (Dordrecht: Springer), 542–564.
Chopin T. (2014). Seaweeds: Top Mariculture Crop, Ecosystem Service Provider. Glob. Aquacult. Advoc. 17, 54–56.
Chung I. K., Oak J. H., Lee J. A., Shin J. A., Kim J. G., Park K. S. (2013). Installing Kelp Forests/Seaweed Beds for Mitigation and Adaptation Against Global Warming: Korean Project Overview. ICES J. Mar. Sci. 70, 1038–1044. doi: 10.1093/icesjms/fss206
Clements J. C., Hunt H.L. (2015). Marine Animal Behavior in a High CO2 Ocean. Mar. Ecol. Prog. Ser. 536, 259–279. doi: 10.3354/meps11426
Clements J. C., Chopin T. (2016). Ocean Acidification and Marine Aquaculture in North America: Potential Impacts and Mitigation Strategies. Rev. Aquacult. 9, 326–341. doi: 10.1111/raq.12140
Conover R. J. (1966). Assimilation of Organic Matter by Zooplankton. Limnol. Oceanogr. 11, 338e345. doi: 10.4319/lo.1966.11.3.0338
Cooley S. R., Kite-Powell H. L., Doney S. C. (2009). Ocean Acidification’s Potential to Alter Global Marine Ecosystems Services. Oceanography 22, 172–181. doi: 10.5670/oceanog.2009.106
Cranford P. J., Kamermans P., Krause G. H. M., Mazurie J. (2012). An Ecosystem-Based Approach and Management Framework for the Integrated Evaluation of Bivalve Aquaculture Impacts. Aquac. Environ. Interact. 2, 193–213. doi: 10.3354/aei00040
Cummings V., Hewitt J., Van Rooyen A., Currie K., Beard S., Thrush S., et al. (2011). Ocean Acidification at High Latitudes: Potential Effects on Functioning of the Antarctic Bivalve Laternula Elliptica. PLos One 61, e16069. doi: 10.1371/journal.pone.0016069
Dickson A. G., Millero F. J. (1987). A Comparison of the Equilibrium Constants for the Dissociation of Carbonic Acid in Seawater Media. Deep. Sea. Res. Part A. 34, 1733–1743. doi: 10.1016/0198-0149(87)90021-5
Fabry V. J., Seibel B. A., Feely R. A., Orr J. C. (2008). Impacts of Ocean Acidification on Marine Fauna and Ecosystem Processes. ICES J. Mar. Sci. 65, 414–432. doi: 10.1093/icesjms/fsn048
FAO (2020). The State of World Fisheries and Aquaculture 2020—Meeting the Sustainable Development Goals (Rome: Licence: CC BY-NC-SA 3.0 IGO).
Findlay H. S., Wood H. L., Kendall M. A., Spicer J. I., Twitchett R. J., Widdicombe S. (2011). Comparing the Impact of High CO2 on Calcium Carbonate Structures in Different Marine Organisms. Mar. Biol. Res. 7, 565–575. doi: 10.1080/17451000.2010.547200
Gazeau F., Gattuso J. P., Greaves M., Elderfield H., Peene J., Heip C. H. R., et al. (2011). Effect of Carbonate Chemistry Alteration on the Early Embryonic Development of the Pacific Oyster (Crassostrea Gigas). PLos One 6, e23010. doi: 10.1371/journal.pone.0023010
Gazeau F., Quibler C., Jansen J. M., Gattuso J. P., Middelburg J. J., Heip C. H. R. (2007). Impact of Elevated CO2 on Shellfish Calcification. Geophys. Res. Lett. 34, L07603. doi: 10.1029/2006GL028554
Gnaiger E. (1983). Heat Dissipation and Energetic Efficiency in Animal Anoxibiosis: Economy Contra Power. J. Exp. Zool. 228, 471–490. doi: 10.1002/jez.1402280308
Han T. (2013). Photosynthetic Physiological Responses of Macroalgae to Different CO2 Concentrations and Their Ecological Effects (Beijing: University of Chinese Academy of Science).
Hofmann G. E., Barry J. P., Enmunds P. J., Gates R. D., Hutchins D. A., Klinger T., et al. (2010). The Effect of Ocean Acidification on Calcifying Organisms in Marine Ecosystems: An Organism-to Ecosystem Perspective. Annu. Rev. Mar. Sci. 41, 127–147. doi: 10.1146/annurev.ecolsys.110308.120227
Jiang Z., Du M., Fang J., Gao Y., Li J., Zhao L., et al. (2017). Size Fraction of Phytoplankton and the Contribution of Natural Plankton to the Carbon Source of Zhikong Scallop Chlamys Farreri in Mariculture Ecosystem of the Sanggou Bay. Acta Oceanol. Sin. 36, 97–105. doi: 10.1007/s13131-017-0970-x
Jiang Z., Fang J., Han T., Li J., Mao Y., Du M. (2014). The Role of Gracilaria Lemaneiformis in Eliminating the Dissolved Inorganic Carbon Released From Calcification and Respiration Process of Chlamys Farreri. J. Appl. Phycol. 26, 545–550. doi: 10.1007/s10811-013-0110-8
Jiang Z., Fang J., Mao Y., Han T., Wang G. (2013). Influence of Seaweed Aquaculture on Marine Inorganic Carbon Dynamics and Sea-Air CO2 Flux. J. World Aquacult. Soc. 44, 133–140. doi: 10.1111/jwas.12000
Jiang Z., Li J., Qiao X., Wang G., Bian D., Jiang X., et al. (2015). The Budget of Dissolved Inorganic Carbon in the Shellfish and Seaweed Integrated Mariculture Area of Sanggou Bay, Shandong, China. Aquaculture 446, 167–174. doi: 10.1016/j.aquaculture.2014.12.043
Jiang Z., Wang X., Rastrick S. P. S., Fang J., Du M., Gao Y., et al. (2019). Metabolic Responses to Elevated Pco2 in the Gills of the Pacific Oyster (Crassostrea Gigas) Using a GC-TOF-MS-Based Metabolomics Approach. Comp. Biochem. Physiol. Part D. 29, 330–338. doi: 10.1016/j.cbd.2019.01.003
Jiang W., Wang X., Rastrick S. P. S., Wang J., Zhang Y., Stand Ø., et al. (2021). Effects of Elevated Pco2 on the Physiological Energetics of Pacific Oyster, Crassostrea Gigas. ICES J. Mar. Sci. 78, 2579–2590. doi: 10.1093/icesjms/fsab139
Kaladharan P., Amalu A. M., Revathy S. (2019). Role of Seaweeds in Neutralizing the Impact of Seawater Acidification- A Laboratory Study With Beached Shells of Certain Bivalves and Spines of a Sea Urchin. Mar. Biol. Ass. India. 61, 94–99. doi: 10.6024/jmbai.2019.61.1.2063-14
Kroeker K. J., Kordas R. L., Crim R. N., Hendriks I. E., Ramajo L., Singh G. S., et al. (2013). Impacts of Ocean Acidification on Marine Organisms: Quantifying Sensitivities and Interaction With Warming. Glob. Change Biol. 19, 1884–1896. doi: 10.1111/gcb.12179
Kurihara H., Kato S., Ishimatsu A. (2007). Effects of Increased Seawater Pco2 on Early Development of the Oyster Crassostrea Gigas. Aquat. Biol. 1, 91–98. doi: 10.3354/ab00009
Lannig G., Eilers S., Pörtner H. O., Sokolova I. M., Bock C. (2010). Impact of Ocean Acidification on Energy Metabolism of Oyster, Crassostrea Gigas—Changes in Metabolic Pathways and Thermal Response. Mar. Drugs 8, 2318–2339. doi: 10.3390/md8082318
Li S., Liu C., Zhan A., Xie L., Zhang R. (2017). Influencing Mechanism of Ocean Acidification on Byssus Performance in the Pearl Oyster Pinctada Fucata. Environ. Sci. Technol. 51, 7696–7706. doi: 10.1021/acs.est.7b02132
Lin F., Du M., Liu H., Fang J., Lars A., Jiang Z. (2020). A Physicalbiological Coupled Ecosystem Model for Integrated Aquaculture of Bivalve and Seaweed in Sanggou Bay. Ecol. Model. 431, 109181. doi: 10.1016/j.ecolmodel.2020.109181
Liu W., He M. (2012). Effects of Ocean Acidification on the Metabolic Rates of Three Species of Bivalve From Southern Coast of China. Chin. J. Oceanol. Limn. 30, 206–211. doi: 10.1007/s00343-012-1067-1
Mehrbach C., Culberson C. H., Hawley J. E., Pytkowicz R. M. (1973). Measurement of the Apparent Dissociation Constants of Carbonic Acid in Seawater at Atmospheric Pressure. Limnol. Oceanogr. 18, 897–907. doi: 10.4319/lo.1973.18.6.0897
Melzner F., Stange P., Trübenbach K., Thomsen J., Casties I., Panknin U., et al. (2011). Food Supply and Seawater Pco2 Impact Calcification and Internal Shell Dissolution in the Blue Mussel Mytilus Edulis. PLos One 6, e24223. doi: 10.1371/journal.pone.0024223
Mistri M., Munari C. (2013). The Invasive Bag Mussel Arcuatula Senhousia is a CO2 Generator in Near-Shore Coastal Ecosystems. J. Exp. Mar. Biol. Ecol. 440, 164–168. doi: 10.1016/j.jembe.2012.11.019
Navarro J. M., Torres R., Acuña K., Duarte C., Manriquez P. H., Lardies M., et al. (2013). Impact of Medium-Term Exposure to Elevated Pco2 Levels on the Physiological Energetics of the Mussel Mytilus Chilensis. Chemosphere 90, 1242–1248. doi: 10.1016/j.chemosphere.2012.09.063
Noisette F., Hurd C. (2018). Abiotic and Biotic Interactions in the Diffusive Boundary Layer of Kelp Blades Create a Potential Refuge From Ocean Acidification. Funct. Ecol. 32, 1329–1342. doi: 10.1111/1365-2435.13067
Peng C., Zhao X., Liu S., Shi W., Han Y., Guo C., et al. (2017). Ocean Acidification Alters the Burrowing Behaviour, Ca2+/Mg2+-ATPase Activity, Metabolism, and Gene Expression of a Bivalve Species, Sinonovacula Contricta. Mar. Ecol. Prog. Ser. 575, 107–117. doi: 10.3354/meps12224
Pierrot D., Lewis E., Wallace D. W. R. (2006). CO2SYS DOS Program Developed for CO2 System Calculations, ORNL/CDIAC-105 (Oak Ridge, Tennessee: Carbon Dioxide Information Analysis Center, Oak Ridge National Laboratory, U.S. Department of Energy).
Rastrick S. P. S., Calosi P., Calder-Potts R., Foggo A., Nightingale G., Widdicombe S., et al. (2014). Living in Warmer, More Acidic Oceans Retards Physiological Recovery From Tidal Emersion in the Velvet Swimming Crab, Necora Puber. J. Exp. Biol. 217, 2499–2508. doi: 10.1242/jeb.089011
Rastrick S. P. S., Collier V., Graham H., Strohmeier T., Whiteley N. M., Strand Ø. (2018a). Feeding Plasticity More Than Metabolic Rate Drives the Productivity of Economically Important Filter Feeders in Response to Elevated CO2 and Reduced Salinity. ICES J. Mar. Sci. 75, 2117–2128. doi: 10.1093/icesjms/fsy079
Rastrick S. S. P., Graham H., Azetsu-Scott K., Calosi P., Chierici M., Fransson A., et al. (2018b). Using Natural Analogues to Investigate the Effects of Climate Change and Ocean Acidification on Northern Ecosystems. ICES J. Mar. Sci. 75, 2299–2311. doi: 10.1093/icesjms/fsy128
Riebesell U., Bellerby R. G. J., Grossart H. P., Thingstad F. (2008). Mesocosm CO2 Perturbation Studies: From Organism to Community Level. Biogeosciences 5, 1157–1164. doi: 10.5194/bg-5-1157-2008
Ries J. B., Cohen A. L., McCorkle D. C. (2009). Marine Calcifiers Exhibit Mixed Responses to CO2-Induced Ocean Acidification. Geology 37, 1131–1134. doi: 10.1130/G30210A.1
Sui Y., Kong H., Huang X., Dupont S., Hu M., Storch D., et al. (2016). Combined Effects of Short-Term Exposure to Elevated CO2 and Decreased O2 on the Physiology and Energy Budget of the Thick Shell Mussel Mytilus Coruscus. Chemosphere 155, 207–216. doi: 10.1016/j.chemosphere.2016.04.054
Tang Q., Zhang J., Fang J. (2011). Shellfish and Seaweed Mariculture Increase Atmospheric CO2 Absorption by Coastal Ecosystems. Mar. Ecol. Prog. Ser. 424, 97–104. doi: 10.3354/meps08979
Tan K., Zheng H. (2019). “Climate Change and Bivalve Mass Mortality in Temperate Regions,” in Reviews of Environmental Contamination and Toxicology. Ed. de Voogt W. P. (Switzerland: Springer International Publishing), 109–129.
Tan K., Zheng H. (2020). Ocean Acidification and Adaptive Bivalve Farming. Sci. Total. Environ. 701, 134794. doi: 10.1016/j.scitotenv.2019.134794
Thomsen J., Gutowska M. A., Saphorster J., Heinemann A., Fietzke J., Hiebenthal C., et al. (2010). Calcifying Invertebrates Succeed in a Naturally CO2-Rich Coastal Habitat But are Threatened by High Levels of Future Acidification. Biogeosciences 7, 3879–3891. doi: 10.5194/bg-7-3879-2010
Wahl M., Schneider Covachã S., Saderne V., Hiebenthal C., Müller J. D., Pansch C., et al. (2018). Macroalgae may Mitigate Ocean Acidification Effects on Mussel Calcification by Increasing pH and its Fluctuations. Limnol. Oceanogr. 63, 3–21. doi: 10.1002/lno.10608
Wang Q., Cao R., Ning X., You L., Wang C., Wei L., et al. (2016). Effects of Ocean Acidification on Immune Responses of the Pacific Oyster Crassostrea Gigas. Fish. Shellf. Immunol. 49, 24–33. doi: 10.1016/j.fsi.2015.12.025
Wang Y., Li L., Hua M., Lu W. (2015). Physiological Energetics of the Thick Shell Mussel Mytilus Coruscus Exposed to Seawater Acidification and Thermal Stress. Sci. Total. Environ. 514, 261–272. doi: 10.1016/j.scitotenv.2015.01.092
Widdows J., Johnson D. (1988). Physiological Energetics of Mytilus Edulis: Scope for Growth. Mar. Ecol. Prog. Ser. 46, 113–121. doi: 10.3354/meps046113
Xu D., Brennan G., Xu L., Zhang X., Fan X., Han W., et al. (2018). Ocean Acidification Increases Iodine Accumulation in Kelp-Based Coastal Food Webs. Glob. Change Biol. 25, 629–639. doi: 10.1111/gcb.14467
Xu X., Yang F., Zhao L., Yan X. (2016). Seawater Acidification Affects the Physiological Energetics and Spawning Capacity of the Manila Clam Ruditapes Philippinarum During Gonadal Maturation. Comp. Biochem. Physiol. Part A. 196, 20–29. doi: 10.1016/j.cbpa.2016.02.014
Zha S., Liu S., Su W., Shi W., Xiao G., Yan M., et al. (2017). Laboratory Simulation Reveals Significant Impacts of Ocean Acidification on Microbial Community Composition and Host-Pathogen Interactions Between the Blood Clam and Vibro Harveyi. Fish. Shellf. Immunol. 71, 393–398. doi: 10.1016/j.fsi.2017.10.034
Keywords: Saccharina japonica, Crassostrea gigas, elevated seawater pCO2, mesocosm, physiological responses
Citation: Jiang Z, Jiang W, Rastrick SPS, Wang X, Fang J, Du M, Gao Y, Mao Y, Strand Ø and Fang J (2022) The Potential of Kelp Saccharina japonica in Shielding Pacific Oyster Crassostrea gigas From Elevated Seawater pCO2 Stress. Front. Mar. Sci. 9:862172. doi: 10.3389/fmars.2022.862172
Received: 25 January 2022; Accepted: 19 April 2022;
Published: 19 May 2022.
Edited by:
Wei Huang, Ministry of Natural Resources, ChinaCopyright © 2022 Jiang, Jiang, Rastrick, Wang, Fang, Du, Gao, Mao, Strand and Fang. This is an open-access article distributed under the terms of the Creative Commons Attribution License (CC BY). The use, distribution or reproduction in other forums is permitted, provided the original author(s) and the copyright owner(s) are credited and that the original publication in this journal is cited, in accordance with accepted academic practice. No use, distribution or reproduction is permitted which does not comply with these terms.
*Correspondence: Zengjie Jiang, amlhbmd6akB5c2ZyaS5hYy5jbg==
†These authors have contributed equally to this work