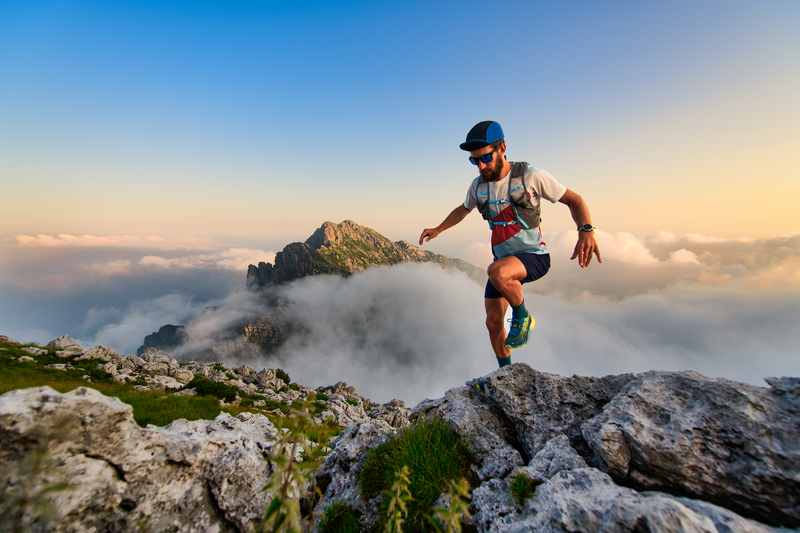
95% of researchers rate our articles as excellent or good
Learn more about the work of our research integrity team to safeguard the quality of each article we publish.
Find out more
ORIGINAL RESEARCH article
Front. Mar. Sci. , 20 July 2022
Sec. Marine Biology
Volume 9 - 2022 | https://doi.org/10.3389/fmars.2022.861945
This article is part of the Research Topic Protists as Model Ecological and Evolutionary Study Systems: Emerging Methodologies of the 21st century View all 8 articles
Nitrogen and sulfur are key elements in the biogeochemical cycles of marine ecosystems to which benthic foraminifera contribute significantly. Yet, cell-specific assimilation of ammonium, nitrate and sulfate by these protists is poorly characterized and understood across their wide range of species-specific trophic strategies. For example, detailed knowledge about ammonium and sulfate assimilation pathways is lacking and although some benthic foraminifera are known to maintain intracellular pools of nitrate and/or to denitrify, the potential use of nitrate-derived nitrogen for anabolic processes has not been systematically studied. In the present study, NanoSIMS isotopic imaging correlated with transmission electron microscopy was used to trace the incorporation of isotopically labeled inorganic nitrogen (ammonium or nitrate) and sulfate into the biomass of twelve benthic foraminiferal species from different marine environments. On timescales of twenty hours, no detectable 15N-enrichments from nitrate assimilation were observed in species known to perform denitrification, indicating that, while denitrifying foraminifera store intra-cellular nitrate, they do not use nitrate-derived nitrogen to build their biomass. Assimilation of both ammonium and sulfate, with corresponding 15N and 34S-enrichments, were observed in all species investigated (with some individual exceptions for sulfate). Assimilation of ammonium and sulfate thus can be considered widespread among benthic foraminifera. These metabolic capacities may help to underpin the ability of benthic foraminifera to colonize highly diverse marine habitats.
Marine protists are extremely diverse, comprising the majority of eukaryotic lineages (Massana, 2015). These single-cell organisms occur in most, if not all, aquatic habitats and are recognized as key members of marine ecosystems. They exhibit complex relationships with other taxa and are an integral part of the food web and, thus, contribute to the transfer of energy between trophic levels (Arístegui et al., 2009; Edgcomb, 2016). As significant contributors of marine ecosystems, it is important to better define their role in biogeochemical cycles and predict their response to future climate and environmental change. Among marine protists, benthic foraminifera are nearly ubiquitous in aquatic environments where they represent up to 47% of the eukaryotic benthic biomass and, therefore, represent a major component of the meiofauna in marine sediments (e.g., Gooday et al., 2000; Moodley et al., 2000; Pascal et al., 2009). These organisms exhibit diverse life strategies, including heterotrophy, bacterial and/or algal symbioses, kleptoplasty (sequestered chloroplasts), anaerobic (nitrate) respiration, and dormancy (e.g., Goldstein, 1999; Nomaki et al., 2006; Jauffrais et al., 2016; Bernhard et al., 2018; Piña-Ochoa et al., 2010a; Ross and Hallock, 2016). This diversity of life strategies enables them to colonize a wide variety of benthic environments, where they are often considered substantial contributors to nitrogen biogeochemical cycling (Høgslund et al., 2008; Piña-Ochoa et al., 2010a; Glock et al., 2013; Choquel et al., 2021). The ability to denitrify (Risgaard-Petersen et al., 2006; Woehle et al., 2018; Gomaa et al., 2021) is widespread among foraminiferal species (Piña-Ochoa et al., 2010a) and represents a major metabolic pathway that can contribute up to 100% of the total denitrification in sediments (Choquel et al., 2021). Likely related to this, many benthic foraminifera are found to uptake and store nitrate intracellularly both in the presence and absence of oxygen (Piña-Ochoa et al., 2010b; Koho et al., 2011; Glock et al., 2019). On the other hand, whether benthic foraminifera possess a metabolic pathway that allows them to assimilate nitrate-derived nitrogen into their biomass is still unknown. A few benthic foraminiferal species are known to actively assimilate ammonium (NH4+) (LeKieffre et al., 2018b; Jauffrais et al., 2019a; Bird et al., 2020; Gomaa et al., 2021), but it remains unclear whether this assimilation pathway is widespread among benthic foraminifera from contrasting environments.
It is becoming generally accepted that marine nitrogen and sulfur cycles play a paramount role in driving marine primary productivity. These cycles are interconnected through the regulation of dimethyl sulfide (DMS), which is produced by the enzymatic breakdown of DMSP (dimethylsulfoniopropionate) and is one of the major oceanic S sources to the atmosphere (Quinn et al., 1990; Yvon et al., 1996; Herbert, 1999; Sievert et al., 2007; Raina et al., 2017). DMSP biosynthesis was recently shown to occur in metazoans, breaking with the paradigm that this molecule is solely produced by marine algae and intertidal plants (Raina et al., 2013). High DMSP concentrations were found in symbiotic planktonic foraminifera harboring microalgae, making them a potentially significant source of marine DMS(P) (Gutierrez-Rodriguez et al., 2017). However, it remains unclear whether DMSP is produced only by the symbiotic microalgae and subsequently translocated to the host or directly produced by the host itself. Overall, sulfur metabolism has received little attention in benthic foraminifera (Nomaki et al., 2016). Sulfate (SO42-) assimilation is the first step in DMSP production, as well as in sulfated amino acids (such as cysteine) and glutathione synthesis; the latter being a reduced S-containing molecule serving as a reservoir for S in most living cells (Mendoza-Cózatl et al., 2005). Yet, to date, direct sulfate assimilation was shown in only one benthic kleptoplastic foraminiferal species, Nonionellina labradorica (Jauffrais et al., 2019a).
For both N and S cycling, it is essential to evaluate the ability of benthic foraminifera to assimilate nitrate, ammonium and/or sulfate from their sedimentary habitats to better constrain their role in these environmentally relevant biogeochemical cycles. Are these abundant protists able to actively assimilate inorganic nutrients such as ammonium, nitrate and/or sulfate? And by doing so, do they significantly contribute to nitrogen and sulfur remobilization in sediments? To better constrain the nitrogen and sulfur assimilation processes in benthic foraminifera, we used NanoSIMS (nanoscale secondary ion mass spectrometry) to visualize the anabolic incorporation of isotopically labeled compounds in these organisms. NanoSIMS is an ion microprobe that enables the mapping of the chemical and isotopic distribution in biological samples at the subcellular scale by bombarding the sample surface with a primary beam of ions that sputter secondary ions from the sample (Hoppe et al., 2013). This primary ion beam is focused to a beam spot of about 100 nm on the flat sample surface and a high-resolution mass spectrometry resolves specific ions from isobaric interferences in the mass spectrum of the emitted secondary ions. High analytical sensitivity is achieved for certain elements depending on the efficiency with which the element (and all its isotopes) ionizes from (the sample. Used in correlation with electron microscopy, and in combination with pulse-chase isotopic labeling experiments, the NanoSIMS allows to follow the spatio-temporal dynamics of isotopically labeled compound assimilation in specific tissue structures and/or cellular compartments. These assets makes the NanoSIMS a valuable tool in many research fields, including the study of nutrient assimilation dynamics in protist cells (e.g. Carpenter et al., 2018; LeKieffre et al., 2020; Decelle et al., 2021). Here, we combined NanoSIMS imaging with transmission electron microscopy (TEM) observations to investigate the assimilation of 15N-ammonium or 15N-nitrate, and 34S-sulfate at a sub-cellular scale in twelve different benthic foraminifera species from four different habitats.
Foraminifera were sampled in contrasted environments at different locations (Table 1): intertidal mudflats, a fjord, a silled basin and an Arctic methane emitting site. Ammonia sp. and Elphidium williamsoni were collected from the upper sediment layers (ca. 1 to 2 cm deep) in a shallow mudflat flanking the Gullmar Fjord (Sweden) in May 2016 and Haynesina germanica in the upper sediment layer (ca. 1 to 2 cm deep) of an intertidal mudflat in the Bay of Bourgneuf (France) in April 2015. All the species from the Gullmar Fjord (Bulimina marginata, Cassidulina laevigata, Nonionella sp. T1, Nonionella turgida and Nonionellina labradorica) were collected in September 2018 from box-cored surface sediments (ca. 2 cm) at 51-m water depth. Species from the Santa Barbara Basin (SBB), Globobulimina pacifica, N. stella and Stainforthia fusiformis, were sampled from the surface, ca 1 to 2 cm, of Soutar boxcores in February 2017 from > 500 m water depth. Finally, Globobulimina sp. and N. labradorica were also collected from the upper sediment layer (ca. 1 to 2 cm deep) close to an active methane emission site off southern Svalbard in October 2018 using an ROV-operated corer. Details of the sampling sites are given in the Supplementary Table S1 and Supplementary Figure 1.
Table 1 Species investigated in this study from four different marine environments, with indications of key life strategies per prior publications (perhaps from different locales).
Living specimens were isolated from surface sediments under a stereomicroscope based on their cytoplasm color and their pseudopodial activity (movement or cyst formation), and then transferred to new Petri dishes filled with artificial seawater (Red Sea salt, salinity: 35) spiked with either 10µM 15NH4+ or 10µM 15NO3- (Cambridge Isotope Inc.), and, for some species, with 25mM 34SO42- (Cambridge Isotope Inc.). All the incubations lasted 20 h, at room temperature for H. germanica and at 10°C for all other species, with a light source set at 90 µmol photon m-2 s-1 for all species from intertidal environments, and in the dark for species from SBB, Gullmar Fjord and Svalbard. After incubation, all specimens were chemically fixed and embedded into resin (for details see Jauffrais et al., 2019; LeKieffre et al., 2018b; LeKieffre et al., 2018a). Details of the experimental conditions are given in Supplementary Table S1.
The embedded specimens were processed for TEM observations and NanoSIMS analysis as described in Jauffrais et al. (2019) and LeKieffre et al. (2018b). TEM imaging was performed either with a Philips 301 CM100 (80 kV) at the Electron Microscopy Facility of the University of Lausanne (Switzerland) or with JEOL JEM 1400 at the SCIAM platform of the University of Angers (France). NanoSIMS analysis was performed with a Cameca NanoSIMS 50L ion microprobe in Lausanne, Switzerland (Hoppe et al., 2013). Note that the sample preparation method for TEM involved multiple steps that remove the soluble components of the cell. However, the structural components, such as proteins or fatty acids, which represent the products of anabolic metabolism, are generally fixed so they remain in the cell (Nomaki et al., 2018; Gibbin et al., 2020; Loussert-Fonta et al., 2020). Both 15N/14N and 34S/32S ratios were measured by simultaneously collecting the molecular ions 12C14N-, 12C15N-, 32S- and 34S-. Quantified 15N/14N and 34S/32S ratios were obtained as follows:
where 15/14Nmeas and 34/32Smeas are the 12C15N-/12C14N- and 34S-/32S- ratios measured in the isotopically labeled samples, and 15/14Ncontrol and 34/32Scontrol are the 12C15N-/12C14N- and 34S-/32S- ratios measured in control samples from unspiked filtered seawater, prepared and handled in an identical manner. Isotopic images of the 15N/14N and 34S/32S ratio distributions were processed using the software L’IMAGE (developed by Dr. Larry Nittler, Carnegie Institution of Washington DC, USA).
Our TEM-NanoSIMS analyses provide the first demonstration of widespread ammonium and sulfate assimilation among benthic foraminifera irrespective of their life strategy (e.g., heterotrophy, mixotrophy, kleptoplasty, nitrate respiration). Individuals of twelve benthic foraminiferal species with different life strategies from four contrasted environments (intertidal mudflats, fjord, silled basin or methane emitting site, see Table 1), were analyzed for ammonium, nitrate and sulfate assimilation with correlated TEM-NanoSIMS imaging. Note that the NanoSIMS imaging produced in this study only quantifies anabolic incorporation of 15N or 34S into cellular biomass. Soluble components not retained by sample preparation procedures were lost (c.f., Material and Methods).
All specimens of all species incubated with 15N-ammonium exhibited clear cellular 15N-enrichments (Supplementary Table S1). 15N-ammonium was anabolically assimilated into a suite of organelles in all species and specimens analyzed (Figure 1 and Supplementary Figures S3, S5, S7, S9, S11, S14, S17, S19 and S20). These organelles included common structures found in benthic foraminifera cytoplasm: electron-opaque bodies, fibrillar vesicles, nuclei and nucleoli (Figures 1, 2) (LeKieffre et al., 2018a). This is in agreement with previous isotope-uptake observations in benthic foraminiferal species (LeKieffre et al., 2018b; Jauffrais et al., 2019a; Bird et al., 2020). Except for the nucleus and nucleolus, the roles of these organelles are poorly known (LeKieffre et al., 2018a) and interpretations of their role in N-assimilation pathways are therefore difficult to ascertain. It should be noted that these structures were not always 15N-enriched within a given cell, and that inter- and intra-species variations were substantial (Supplementary Figures S2 − S20). In addition, a number of other organelles were occasionally enriched in 15N in certain species, such as the apertural collars in S. fusiformis (Figure 1 and Supplementary Figure S17), peroxisome-endoplasmic reticulum complexes (P-ER complexes, Figure 2 and Supplementary Figure S19) in G. pacifica and prokaryote-like structures in B. marginata cytoplasm (Figures 1, 2 and Supplementary Figure S5).
Figure 1 TEM micrographs of different benthic foraminiferal species’ cytoplasm and corresponding NanoSIMS images of 15N-ammonium assimilation (expressed as δ15N in ‰). Arrows: electron-opaque bodies, arrowheads: fibrillar vesicles, thick arrow: apertural collars, circles: prokaryote-like vesicles, c, kleptoplasts; li, lipid droplets; n, nucleus; nu, nucleoli; v, vacuoles. Inset 1 is presented in Figure 2A.
Figure 2 TEM micrographs of organelles commonly found 15N- or 34S-enriched in our benthic foraminiferal cells after incubation with 15N-ammonium and/or 34S-sulfate. (A) Close-up of a putative prokaryote-like structure observed in B marginata endoplasm in inset 1 of Figure 1. (B, C) Electron opaque bodies and fibrillar vesicles, two structures commonly found enriched in 15N and 34S, here in N. turgida (B) and N. labradorica Gullmar fjord (C). (D)34S-enriched vesicles with thick membranes observed in E williamsoni. (E) Peroxisome – endoplasmic reticulum (P-ER) complex in Globobulimina pacifica. Arrows: electron-opaque bodies, arrowheads: fibrillar vesicles, asterisks: thick-membrane vesicles, li, lipid droplets; m, mitochondria; p, peroxisome.
Overall, 15N-enrichment for ammonium assimilation was higher in intertidal species with 15N-enrichment exceeding 10,000 ‰ in some organelles such as fibrillar vesicles or electron opaque bodies, while 15N-enrichment in species from deeper-water sites (fjord, silled basin or methane emitting site) did not exceed 5000 ‰. The highly contrasted environmental conditions (e.g., presence/absence of sunlight, temperature, dissolved oxygen concentration) faced by the foraminifera likely affect their metabolic processes. In this study, the different foraminifera species were incubated with light and temperature conditions as similar as possible to those of their habitats (see Supplementary Table S1, note that all incubations were carried in oxygenated conditions). Lower N assimilation rates were observed in specimens challenged by low temperatures or incubated in the dark. One notable exception is B. marginata from the Gullmar Fjord that has cytoplasmic structures identified as putative prokaryotes that displayed 15N-enrichment values reaching ca. 30,000 ‰, thus supporting the hypothesis that these structures are prokaryotes. B. marginata is not known to harbor symbiotic prokaryotes and its 15N-enriched prokaryote-like structures were always confined to vacuoles. But foraminiferal specimens were cleaned with a fine brush prior incubation to remove most sedimentary material, thus removing most of the prokaryotes from their environment. Besides, these 15N-enriched prokaryote-like structures were observed in chambers n-8 to n-6 (n being the last chamber calcified, so the chamber closest to the external environment), while digestive vacuoles are typically observed in the most external (youngest) chambers (LeKieffre et al., 2018a). Thus, it remains unclear whether these prokaryote-like structures were associated with the calcite shell exterior, assimilating 15N-ammonium before being ingested by foraminifera (– the 15N-enrichment of these structures would then result from a feeding strategy independent of foraminiferal processes), – or if they act as putative symbionts, actively assimilating 15N-nitrogen from the cytoplasm of B. marginata (putatively exchanging metabolites with their foraminiferal host). Note that ammonium assimilation mediated by prokaryotes has been previously suggested for H. germanica, in which highly 15N-labeled prokaryote-like structures not enclosed in digestion vacuoles were observed (LeKieffre et al., 2018b).
In contrast, no 15N assimilation was observed in foraminifera incubated with 15N-nitrate (Figure 3 and Supplementary Table S1). The absence of nitrate assimilation in our material (Figure 3 and Supplementary Figures S4, S6, S8, S10, S12, S13,S15, S16 and S18) contrasts with one of the few studies that investigated 15N-nitrate assimilation by benthic foraminifera, finding enrichment in their cytoplasm (Nomaki et al., 2016). In that study, however, the foraminifer Ammonia sp. was incubated with 15N-nitrate in sediment for 14 days with a high likelihood of nitrate-derived 15N transfer into other compounds (possibly mediated by prokaryotes) that could in turn have been assimilated by the foraminifera. Some foraminiferal species are known to actively uptake and store nitrate within their cell, in both oxygenated and anoxic conditions (Piña-Ochoa et al., 2010b; Koho et al., 2011; Glock et al., 2019). In our study, even foraminiferal species that are known to store nitrate intracellularly (e.g., Globobulimina sp., N. stella, Stainforthia fusiformis) were not observed to assimilate detectable amounts of nitrate-derived 15N in their biomass, suggesting that nitrate stored in denitrifying species is exclusively used as an electron acceptor and is not involved in anabolic processes.
Figure 3 TEM micrographs of different benthic foraminiferal species’ cytoplasm and corresponding NanoSIMS images of 15N-nitrate assimilation (expressed as δ15N in ‰). c, kleptoplasts; dv, degradation vacuoles; re, residual bodies; v, vacuoles.
34S-sulfate assimilation was observed in all investigated species except Globobulimina sp. sampled from the methane emitting site (Figure 4; Supplementary Table S1). In other species, all specimens exhibited significant 34S enrichment, except Globobulimina pacifica (2 of 6 from the silled basin), N. labradorica (1 of 2 from the methane emitting site) and S. fusiformis (1 of 2 from the silled basin). Neither Globobulimina sp. (n=2) sampled from the methane emission site exhibited significant 34S enrichment (Supplementary Figure S19). However, some Globobulimina pacifica from the silled basin were able to assimilate sulfate effectively (Supplementary Figure S14), demonstrating that specimens of the Globobulimina genus can assimilate sulfate. As a result, the absence of assimilation in Globobulimina sp. might be attributed to metabolic adaptations specific to methane emitting sites, such as a lower metabolic activity.
Figure 4 TEM micrographs of different benthic foraminiferal species’ cytoplasm and corresponding NanoSIMS images of 34S-assimilation (expressed as δ34S in ‰). Arrows: electron-opaque bodies, arrowheads: fibrillar vesicles, asterisk: vesicles with thick membranes, circles: unidentified 34S-labeled structures, c, kleptoplasts; li, lipid droplets; v, vacuoles.
In specimens that assimilated sulfate-derived 34S, 34S-enriched organelles were similar between species and included fibrillar vesicles and electron-opaque bodies (Figures 2,4, Supplementary Figures S2, S3, S5, S14–S18 and S20). Additionally, a few subcellular structures with unknown function, including vesicles with thick membranes, were also observed to be 34S-enriched in E. williamsoni (Figures 2,4; Supplementary Figure S3). These observations are in good agreement with previous TEM-NanoSIMS observations on Ammonia sp. and N. labradorica (Nomaki et al., 2016; Jauffrais et al., 2019a). However, it should be noted that in these previous experiments, N. labradorica specimens were incubated with a protocol similar to that used here (Jauffrais et al., 2019a), whereas Ammonia sp. were incubated in sediment for 14 days, so 34S-sulfate might have been reprocessed into other 34S-containing compounds that could subsequently have been assimilated by the foraminifera (Nomaki et al., 2016).
Sulfate can be used in a variety of metabolic pathways such as sulfated amino acids (e.g., cysteine) and glutathione synthesis; the latter being a reduced sulfur containing molecule used as a S-reservoir in most living cells (Mendoza-Cózatl et al., 2005). Its assimilation is also the first step of DMSP production, a molecule involved in the regulation of the cytoplasmic osmotic environment (Yoch, 2002). DMSP biosynthesis has recently been shown to occur in other organisms besides marine algae and intertidal plants. For example, coral larvae lacking endosymbionts were able to produce DMSP (Raina et al., 2013). Our data show that all investigated foraminiferal species actively assimilated sulfate. This raises the possibility that benthic foraminifera, kleptoplastic species in particular since their sequestered chloroplasts presumably have the enzymatic machinery involved in DMSP biosynthesis (Stefels, 2000), play a significant role in the production of DMSP in their respective sediments and thus contribute strongly to the marine S-cycle, particularly with respect to S-removal from the sediment.Here, we demonstrate that ammonium and sulfate assimilation are widespread among benthic foraminifera irrespective of their life strategy, suggesting that multiple metabolic pathways (co-)exist for ammonium and sulfate assimilation in foraminifera (Figure 5). Based on the life traits of the studied foraminifera and our observations, different putative assimilation pathways can be inferred: (1) foraminiferal assimilation via cytoplasmic GDH (glutamate dehydrogenase) and ATP-sulfurylase enzymatic pathways for ammonium and sulfate, respectively, or (2) kleptoplastidial assimilation via the plastidial enzymes GS/GOGAT (glutamate synthase and glutamine oxoglutarate aminotransferase) and plastidial production of sulfated amino acids (cysteine, methionine) and sulfolipids. Ammonium assimilation being mediated either by a cytoplasmic or a kleptoplastic pathway in benthic foraminifera is supported by a recent metatranscriptomic study investigating a non-symbiotic heterotrophic species (Bolivina argentea) and a kleptoplastic species (N. stella) (Gomaa et al., 2021). This analysis of foraminiferal transcriptomes revealed the presence of transcripts coding for the enzymes GS and GOGAT in the kleptoplastic species, while these enzyme expressions were very low in B. argentea, which expressed instead high levels of the cytoplasmic enzymes GDH In our study, sulfate assimilation was shown in all species, even those lacking endobionts or kleptoplasts, such as Ammonia sp. or Cassidulina laevigata, supporting the existence of a foraminiferal cytoplasmic sulfate assimilation pathway. Further, kleptoplasts were previously hypothesized to play a role in sulfate assimilation in a kleptoplastic foraminiferal species inhabiting aphotic areas but there was no evidence supporting presence of a plastidial pathway (Jauffrais et al., 2019a). Finally, (3) assimilation of ammonium and sulfate could occur via endosymbionts. Although data presented in this study do not show evidence of prokaryotic endosymbionts, symbiotic associations with prokaryotes seem to be a common feature in certain (but not all) benthic foraminifera (reviewed in Bernhard et al., 2018). These pathways were previously suggested for sulfate and ammonium assimilation in the species Virgulinella fragilis, which may harbor putative sulfate-reducing bacteria endosymbionts (Tsuchiya et al., 2015), and in H. germanica, which harbors prokaryote-like structures that become highly enriched in 15N after incubation with 15N-ammonium (LeKieffre et al., 2018b), similar to our observations of B. marginata. Future studies should focus on the characterization of the metabolic pathways and the identification of the key metabolites involved in N and S assimilation processes, for example through transcriptomic surveys as it was done for ammonium assimilation by Gomaa et al. (2021), and/or through metabolomic studies that would address the identity of the compounds involved in the assimilation pathways.
Figure 5 Schematic diagram of the putative nitrogen and sulfate assimilation pathways in benthic foraminifera. Ammonium assimilation pathways are displayed in blue and sulfate assimilation pathways in red. In the cytoplasmic pathways, kleptoplastic and prokaryotic pathways, the assimilation of ammonium and sulfate result in N and S incorporation into the foraminiferal biomass. For nitrate uptake occurring in denitrifying foraminifer (shown in grey), N that enters the cell is reduced to gaseous nitrogen and exits the cell without being incorporated into the foraminiferal biomass. Green: kleptoplast; dashed black lines: prokaryotic endosymbionts. GDH, glutamate dehydrogenase; GS-GOGAT, glutamate synthase and glutamine oxoglutarate aminotransferase; ATPS, ATP-sulfurylase.
Benthic foraminifera have already been shown to be major contributors to the nitrogen cycle via their ability to denitrify (Risgaard-Petersen et al., 2006; Høgslund et al., 2008; Piña-Ochoa et al., 2010a; Bernhard et al., 2012; Glock et al., 2013; Glock et al., 2019; Choquel et al., 2021). However, not all foraminifera are able to denitrify, while our study shows ammonium assimilation in biomass for all the foraminiferal species investigated to date. Ammonium assimilation could therefore constitute another pathway for benthic foraminifera to act as major contributors to N cycling, especially considering their high abundance in some environments (e.g., Murray, 1991; Bernhard et al., 1997). We also document via these uptake experiments that these twelve species from geographically widespread disparate environments all assimilate sulfate, suggesting benthic foraminifera in general are key contributors to marine sulfur cycling. A next step is to scale up the ammonium and sulfate assimilation quantification at the population level in foraminifera to estimate their importance in the N and S nutrient cycling in marine sediments, and to decipher the effect(s) of environmental conditions on these assimilation processes.
The original contributions presented in the study are included in the article/Supplementary Material. Further inquiries can be directed to the corresponding author.
CL, TJ, JB, HF, CS, OM, and EG collected the samples from the field. CL, TJ, EG, GP, and AM designed the experiment. CL, HR, CS, and EG performed sample preparation and microscopy observations. CL performed NanoSIMS analysis. CL, TJ, JB, and EG interpreted the data and discussed the results. CL wrote the manuscript and prepared the figures. All authors edited and reviewed the manuscript. All authors contributed to the article and approved the submitted version.
This work was supported by the Swiss National Science Foundation (grant no. 200021_149333), and a postdoctoral fellowship allowed to CL by the University Loire-Bretagne. SBB sampling was funded by US National Science Foundation grant BIO IOS 1557430 to JMB, who also acknowledges NASA grant #80NSSC21K0478 for partial support. HF acknowledges funding from the Swedish Research Council VR (grant number 2017-04190). Svalbard sampling was supported by the Research Council of Norway through CAGE (Center for Excellence in Arctic Gas Hydrate Environment and Climate, project number 223259) and NORCRUST (project number 255150) to GP and the fellowship MOPGA (Make Our Planet Great Again) by CAMPUS France to CS.
The authors declare that the research was conducted in the absence of any commercial or financial relationships that could be construed as a potential conflict of interest.
All claims expressed in this article are solely those of the authors and do not necessarily represent those of their affiliated organizations, or those of the publisher, the editors and the reviewers. Any product that may be evaluated in this article, or claim that may be made by its manufacturer, is not guaranteed or endorsed by the publisher.
Florence Manero from the SCIAM platform at the University of Angers (France), the electron microscopy facility (EMF) at the University of Lausanne (Switzerland), the crews of the R/V Skagerak, R/V Kronprins Haakon, R/V Robert Gordon Sproul and ROV Ægir team are thanked for help and technical assistance. Inda Brinkmann, Constance Choquel, Sami Jokinen, Aurélia Mouret and Hanna Nilsson are thanked for their help with foraminiferal sampling in the Gullmar Fjord. Stephane Escrig is thanked for expertise and help with NanoSIMS imaging.
The Supplementary Material for this article can be found online at: https://www.frontiersin.org/articles/10.3389/fmars.2022.861945/full#supplementary-material
Arístegui J., Gasol J. M., Duarte C. M., Herndld G. J. (2009). Microbial Oceanography of the Dark Ocean’s Pelagic Realm. Limnol. Oceanogr. 54, 1501–1529. doi: 10.4319/lo.2009.54.5.1501
Barras C., Geslin E., Duplessy J.-C., Jorissen F. J. (2009). Reproduction and Growth of the Deep-Sea Benthic Foraminifer Bulimina Marginata Under Different Laboratory Conditions. J. Foraminiferal. Res. 39, 155–165. doi: 10.2113/gsjfr.39.3.155
Bernhard J. M., Alve E. (1996). Survival, ATP Pool, and Ultrastructural Characterization of Benthic Foraminifera From Drammensfjord (Norway): Response to Anoxia. Mar. Micropaleontol. 28, 5–17. doi: 10.1016/0377-8398(95)00036-4
Bernhard J. M., Bowser S. S. (1999). Benthic Foraminifera of Dysoxic Sediments: Chloroplast Sequestration and Functional Morphology. Earth-Science. Rev. 46, 149–165. doi: 10.1016/S0012-8252(99)00017-3
Bernhard J. M., Casciotti K. L., McIlvin M. R., Beaudoin D. J., Visscher P. T., Edgcomb V. P. (2012). Potential Importance of Physiologically Diverse Benthic Foraminifera in Sedimentary Nitrate Storage and Respiration. J. Geophys. Res. 117. doi: 10.1029/2012JG001949
Bernhard J. M., Sen Gupta B. K., Borne P. F. (1997). Benthic Foraminiferal Proxy to Estimate Dysoxic Bottom-Water Oxygen Concentrations; Santa Barbara Basin, U.S. Pacific. continent. margin. J. Foraminiferal. Res. 27, 301–310. doi: 10.2113/gsjfr.27.4.301
Bernhard J. M., Tsuchiya M., Nomaki H. (2018). Ultrastructural Observations on Prokaryotic Associates of Benthic Foraminifera: Food, Mutualistic Symbionts, or Parasites? Mar. Micropaleontol. 138, 33–45. doi: 10.1016/j.marmicro.2017.09.001
Bird C., LeKieffre C., Jauffrais T., Meibom A., Geslin E., Filipsson H. L., et al. (2020). Heterotrophic Foraminifera Capable of Inorganic Nitrogen Assimilation. Front. Microbiol. 11. doi: 10.3389/fmicb.2020.604979
Carpenter K. J., Bose M., Polerecky L., Lie A., Heidelberg K. B., Caron D. A. (2018). Single-Cell View of Carbon and Nitrogen Acquisition in the Mixotrophic Alga Prymnesium Parvum (Haptophyta) Inferred From Stable Isotope Tracers and NanoSIMS. Front. Mar. Sci. 5, 157. doi: 10.3389/fmars.2018.00157
Cedhagen T. (1991). Retention of Chloroplasts and Bathymetric Distribution in the Sublittoral Foraminiferan Nonionellina Labradorica. Ophelia 33, 17–30. doi: 10.1080/00785326.1991.10429739
Choquel C., Geslin E., Metzger E., Filipsson H. L., Risgaard-Petersen N., Launeau P., et al. (2021). Denitrification by Benthic Foraminifera and Their Contribution to N-loss from a Fjord Environment. Biogeosciences, 18, 327–341. doi: 10.5194/bg-18-327-2021
Decelle J., Veronesi G., LeKieffre C., Gallet B., Chevalier F., Stryhanyuk H., et al. (2021). Subcellular Architecture and Metabolic Connection in the Planktonic Photosymbiosis Between Collodaria (Radiolarians) and Their Microalgae. Environ. Microbiol. 23, 6569–6586. doi: 10.1111/1462-2920.15766
Edgcomb V. (2016). Marine Protist Associations and Environmental Impacts Across Trophic Levels in the Twilight Zone and Below. Curr. Opin. Microbiol. Environ. microbiol. Special. Section.: Megaviromes. 31, 169–175. doi: 10.1016/j.mib.2016.04.001
Gibbin E., Banc-Prandi G., Fine M., Comment A., Meibom A. (2020). A Method to Disentangle and Quantify Host Anabolic Turnover in Photosymbiotic Holobionts With Subcellular Resolution. Commun. Biol. 3, 1–6. doi: 10.1038/s42003-019-0742-6
Glock N., Roy A.-S., Romero D., Wein T., Weissenbach J., Revsbech N. P., et al. (2019). Metabolic Preference of Nitrate Over Oxygen as an Electron Acceptor in Foraminifera From the Peruvian Oxygen Minimum Zone. Proc. Natl. Acad. Sci. 116, 2860–2865. doi: 10.1073/pnas.1813887116
Glock N., Schönfeld J., Eisenhauer A., Hensen C., Mallon J., Sommer S. (2013). The Role of Benthic Foraminifera in the Benthic Nitrogen Cycle of the Peruvian Oxygen Minimum Zone. Biogeosciences 10, 4767–4783. doi: 10.5194/bg-10-4767-2013
Goldstein S. T. (1999). Foraminifera: A Biological Overview, in: Sen Gupta, B.K. (Ed.). Modern. Foraminifera. Springer-Verlag. New-York. pp, 37–55. doi: 10.1007/0-306-48104-9_3
Gomaa F., Utter D. R., Powers C., Beaudoin D. J., Edgcomb V. P., Filipsson H. L., et al. (2021). Multiple Integrated Metabolic Strategies Allow Foraminiferan Protists to Thrive in Anoxic Marine Sediments. Sci. Adv. 7, eabf1586. doi: 10.1126/sciadv.abf1586
Gooday A. J., Bernhard J. M., Levin L. A., Suhr S. B. (2000). Foraminifera in the Arabian Sea Oxygen Minimum Zone and Other Oxygen-Deficient Settings: Taxonomic Composition, Diversity, and Relation to Metazoan Faunas. Deep-Sea. Res. Part II.: Topical. Stud. Oceanogr. 47, 25–54. doi: 10.1016/S0967-0645(99)00099-5
Grzymski J., Schofield O. M., Falkowski P. G., Bernhard J. M. (2002). The Function of Plastids in the Deep-Sea Benthic Foraminifer, Nonionella Stella. Limnol. oceanogr. 47, 1569–1580. doi: 10.4319/lo.2002.47.6.1569
Gutierrez-Rodriguez A., Pillet L., Biard T., Said-Ahmad W., Amrani A., Simó R., et al. (2017). Dimethylated Sulfur Compounds in Symbiotic Protists: A Potentially Significant Source for Marine DMS(P). Limnol. Oceanogr. 62, 1139–1154. doi: 10.1002/lno.10491
Høgslund S., Revsbech N. P., Cedhagen T., Nielsen L. P., Gallardo V. A. (2008). Denitrification, Nitrate Turnover, and Aerobic Respiration by Benthic Foraminiferans in the Oxygen Minimum Zone Off Chile. J. Exp. Mar. Biol. Ecol. 359, 85–91. doi: 10.1016/j.jembe.2008.02.015
Herbert R. A. (1999). Nitrogen Cycling in Coastal Marine Ecosystems. FEMS Microbiol. Rev. 23, 563–590. doi: 10.1111/j.1574-6976.1999.tb00414.x
Hoppe P., Cohen S., Meibom A. (2013). NanoSIMS: Technical Aspects and Applications in Cosmochemistry and Biological Geochemistry. Geostandards. Geoanalytical. Res. 37, 111–154. doi: 10.1111/j.1751-908X.2013.00239.x
Jauffrais T., Jesus B., Metzger E., Mouget J.-L., Jorissen F., Geslin E. (2016). Effect of Light on Photosynthetic Efficiency of Sequestered Chloroplasts in Intertidal Benthic Foraminifera (Haynesina Germanica and Ammonia Tepida). Biogeosciences 13, 2715–2726. doi: 10.5194/bg-13-2715-2016
Jauffrais T., LeKieffre C., Schweizer M., Geslin E., Metzger E., Bernhard J. M., et al. (2019a). Kleptoplastidic Benthic Foraminifera From Aphotic Habitats: Insights Into Assimilation of Inorganic C, N and S Studied With Sub-Cellular Resolution. Environ. Microbiol. 21, 125–141. doi: 10.1111/1462-2920.14433
Jauffrais T., LeKieffre C., Schweizer M., Jesus B., Metzger E., Geslin E. (2019b). Response of a Kleptoplastidic Foraminifer to Heterotrophic Starvation: Photosynthesis and Lipid Droplet Biogenesis. FEMS Microbiol. Ecol. 95, fiz046. doi: 10.1093/femsec/fiz046
Koho K. A., Piña-Ochoa E., Geslin E., Risgaard-Petersen N. (2011). Vertical Migration, Nitrate Uptake and Denitrification: Survival Mechanisms of Foraminifers (Globobulimina Turgida) Under Low Oxygen Conditions: Survival Mechanisms of Foraminifers. FEMS Microbiol. Ecol. 75, 273–283. doi: 10.1111/j.1574-6941.2010.01010.x
LeKieffre C., Bernhard J. M., Mabilleau G., Filipsson H. L., Meibom A., Geslin E. (2018a). An Overview of Cellular Ultrastructure in Benthic Foraminifera: New Observations of Rotalid Species in the Context of Existing Literature. Mar. Micropaleontol. 138, 12–32. doi: 10.1016/j.marmicro.2017.10.005
LeKieffre C., Jauffrais T., Geslin E., Jesus B., Bernhard J. M., Giovani M.-E., et al. (2018b). Inorganic Carbon and Nitrogen Assimilation in Cellular Compartments of a Benthic Kleptoplastic Foraminifer. Sci. Rep. 8, 10140. doi: 10.1038/s41598-018-28455-1
LeKieffre C., Spangenberg J. E., Mabilleau G., Escrig S., Meibom A., Geslin E. (2017). Surviving Anoxia in Marine Sediments: The Metabolic Response of Ubiquitous Benthic Foraminifera (Ammonia Tepida). PloS One 12, e0177604. doi: 10.1371/journal.pone.0177604
LeKieffre C., Spero H. J., Fehrenbacher J. S., Russell A. D., Ren H., Geslin E., et al. (2020). Ammonium is the Preferred Source of Nitrogen for Planktonic Foraminifer and Their Dinoflagellate Symbionts. Proc. R. Soc. B.: Biol. Sci. 287, 20200620. doi: 10.1098/rspb.2020.0620
Lopez E. (1979). Algal Chloroplasts in the Protoplasm of Three Species of Benthic Foraminifera: Taxonomic Affinity, Viability and Persistence. Mar. Biol. 53, 201–211. doi: 10.1007/BF00952427
Loussert-Fonta C., Toullec G., Paraecattil A. A., Jeangros Q., Krueger T., Escrig S., et al. (2020). Correlation of Fluorescence Microscopy, Electron Microscopy, and NanoSIMS Stable Isotope Imaging on a Single Tissue Section. Commun. Biol. 3, 1–9. doi: 10.1038/s42003-020-1095-x
Massana R. (2015). “Protistan Diversity in Environmental Molecular Surveys,” in Marine Protists, Diversity and Dynamics. Eds. Ohtsuka S., Suzaki T., Horiguchi T., Suzuki N., Not F. (Springer Tokyo: Springer), 3–21.
Mendoza-Cózatl D., Loza-Tavera H., Hernández-Navarro A., Moreno-Sánchez R. (2005). Sulfur Assimilation and Glutathione Metabolism Under Cadmium Stress in Yeast, Protists and Plants. FEMS Microbiol. Rev. 29, 653–671. doi: 10.1016/j.femsre.2004.09.004
Moodley L., Boschker H. T., Middelburg J. J., Pel R., Herman P. M., De Deckere E., et al. (2000). Ecological Significance of Benthic Foraminifera: 13C Labelling Experiments. Mar. Ecology-Progress. Ser. 202, 289–295. doi: 10.3354/meps202289
Murray J. W. (1991). Ecology and Distribution of Benthic Foraminifera (London: Biology of Foraminifera. Academic Press), 221–253.
Nomaki H., Bernhard J. M., Ishida A., Tsuchiya M., Uematsu K., Tame A., et al. (2016). Intracellular Isotope Localization in Ammonia Sp. (Foraminifera) of Oxygen-Depleted Environments: Results of Nitrate and Sulfate Labeling Experiments. Front. Microbiol. 163. doi: 10.3389/fmicb.2016.00163
Nomaki H., Heinz P., Nakatsuka T., Shimanaga M., Ohkouchi N., Ogawa N. O., et al. (2006). Different Ingestion Patterns of 13C-Labeled Bacteria and Algae by Deep-Sea Benthic Foraminifera. Mar. Ecol. Prog. Ser. 310, 95–108. doi: 10.3354/meps310095
Nomaki H., LeKieffre C., Escrig S., Meibom A., Yagyu S., Richardson E. A., et al. (2018). Innovative TEM-Coupled Approaches to Study Foraminiferal Cells. Mar. Micropaleontol. 138, 90–104. doi: 10.1016/j.marmicro.2017.10.002
Pascal P.-Y., Dupuy C., Richard P., Mallet C., du Chatelet E. A., Niquil N. (2009). Seasonal Variation in Consumption of Benthic Bacteria by Meio-and Macrofauna in an Intertidal Mudflat. Limnol. Oceanogr. 54, 1048. doi: 10.4319/lo.2009.54.4.1048
Piña-Ochoa E., Hogslund S., Geslin E., Cedhagen T., Revsbech N. P., Nielsen L. P., et al. (2010a). Widespread Occurrence of Nitrate Storage and Denitrification Among Foraminifera and Gromiida. Proc. Natl. Acad. Sci. 107, 1148–1153. doi: 10.1073/pnas.0908440107
Piña-Ochoa E., Koho K., Geslin E., Risgaard-Petersen N. (2010b). Survival and Life Strategy of the Foraminiferan Globobulimina Turgida Through Nitrate Storage and Denitrification. Mar. Ecol. Prog. Ser. 417, 39–49. doi: 10.3354/meps08805
Quinn P. K., Bates T. S., Johnson J. E., Covert D. S., Charlson R. J. (1990). Interactions Between the Sulfur and Reduced Nitrogen Cycles Over the Central Pacific Ocean. J. Geophys. Research.: Atmospheres. 95, 16405–16416. doi: 10.1029/JD095iD10p16405
Raina J.-B., Clode P. L., Cheong S., Bougoure J., Kilburn M. R., Reeder A., et al. (2017). Subcellular Tracking Reveals the Location of Dimethylsulfoniopropionate in Microalgae and Visualises its Uptake by Marine Bacteria. eLife 6, e23008. doi: 10.7554/eLife.23008
Raina J.-B., Tapiolas D. M., Forêt S., Lutz A., Abrego D., Ceh J., et al. (2013). DMSP Biosynthesis by an Animal and its Role in Coral Thermal Stress Response. Nature 502, 677–680. doi: 10.1038/nature12677
Risgaard-Petersen N., Langezaal A. M., Ingvardsen S., Schmid M. C., Jetten M. S. M., Op den Camp H. J. M., et al. (2006). Evidence for Complete Denitrification in a Benthic Foraminifer. Nature 443, 93–96. doi: 10.1038/nature05070
Ross B. J., Hallock P. (2016). Dormancy in the Foraminifera: A Review. J. Foraminiferal. Res. 46, 358–368. doi: 10.2113/gsjfr.46.4.358
Schmidt C., Geslin E., Bernhard J. M., LeKieffre C., Svenning M. M., Roberge H., et al. (2021). Deposit Feeding of a Foraminifera From an Arctic Methane Seep Site and Possible Association With a Methanotroph Revealed by Transmission Electron Microscopy. Biogeosciences. Discussions., 1–28. doi: 10.5194/bg-2021-284
Sievert S. M., Kiene R. P., Schultz-Vogt H. N. (2007). The Sulfur Cycle. Oceanography 20, 117–123. doi: 10.5670/oceanog.2007.55
Stefels J. (2000). Physiological Aspects of the Production and Conversion of DMSP in Marine Algae and Higher Plants. J. Sea. Res. 43, 183–197. doi: 10.1016/S1385-1101(00)00030-7
Tsuchiya M., Toyofuku T., Uematsu K., Brüchert V., Collen J., Yamamoto H., et al. (2015). Cytologic and Genetic Characteristics of Endobiotic Bacteria and Kleptoplasts of Virgulinella Fragilis (Foraminifera). J. Eukaryotic. Microbiol. 62, 454–469. doi: 10.1111/jeu.12200
Woehle C., Roy A.-S., Glock N., Wein T., Weissenbach J., Rosenstiel P., et al. (2018). A Novel Eukaryotic Denitrification Pathway in Foraminifera. Curr. Biol. 28, 2536–2543.e5. doi: 10.1016/j.cub.2018.06.027
Wukovits J., Oberrauch M., Enge A. J., Heinz P. (2018). The Distinct Roles of Two Intertidal Foraminiferal Species in Phytodetrital Carbon and Nitrogen Fluxes – Results From Laboratory Feeding Experiments. Biogeosciences 15, 6185–6198. doi: 10.5194/bg-15-6185-2018
Yoch D. C. (2002). Dimethylsulfoniopropionate: Its Sources, Role in the Marine Food Web, and Biological Degradation to Dimethylsulfide. Appl. Environ. Microbiol. 68, 5804–5815. doi: 10.1128/AEM.68.12.5804-5815.2002
Keywords: marine protists, coastal environments, biogeochemical cycles, NanoSIMS, nitrogen, sulfur
Citation: LeKieffre C, Jauffrais T, Bernhard JM, Filipsson HL, Schmidt C, Roberge H, Maire O, Panieri G, Geslin E and Meibom A (2022) Ammonium and Sulfate Assimilation Is Widespread in Benthic Foraminifera. Front. Mar. Sci. 9:861945. doi: 10.3389/fmars.2022.861945
Received: 25 January 2022; Accepted: 06 June 2022;
Published: 20 July 2022.
Edited by:
Anieke Brombacher, University of Southampton, United KingdomCopyright © 2022 LeKieffre, Jauffrais, Bernhard, Filipsson, Schmidt, Roberge, Maire, Panieri, Geslin and Meibom. This is an open-access article distributed under the terms of the Creative Commons Attribution License (CC BY). The use, distribution or reproduction in other forums is permitted, provided the original author(s) and the copyright owner(s) are credited and that the original publication in this journal is cited, in accordance with accepted academic practice. No use, distribution or reproduction is permitted which does not comply with these terms.
*Correspondence: Charlotte LeKieffre, Y2hhcmxvdHRlLmxla2llZmZyZUBnbWFpbC5jb20=
†Present address: Charlotte LeKieffre, Cell and Plant Physiology Laboratory, University of Grenoble Alpes – CNRS – CEA – INRAE, Grenoble, France
Disclaimer: All claims expressed in this article are solely those of the authors and do not necessarily represent those of their affiliated organizations, or those of the publisher, the editors and the reviewers. Any product that may be evaluated in this article or claim that may be made by its manufacturer is not guaranteed or endorsed by the publisher.
Research integrity at Frontiers
Learn more about the work of our research integrity team to safeguard the quality of each article we publish.