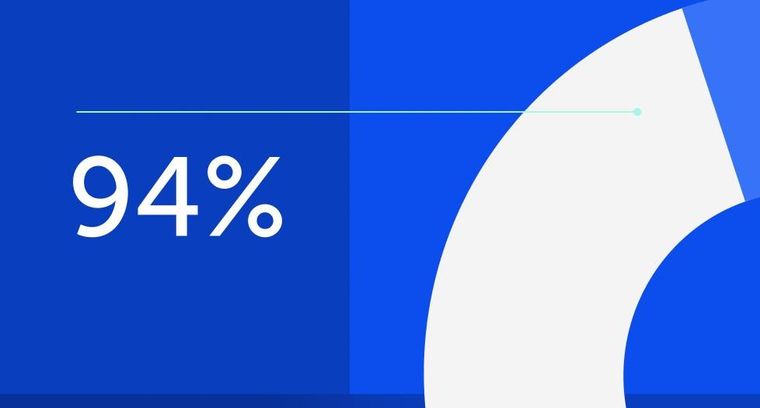
94% of researchers rate our articles as excellent or good
Learn more about the work of our research integrity team to safeguard the quality of each article we publish.
Find out more
ORIGINAL RESEARCH article
Front. Mar. Sci., 27 May 2022
Sec. Global Change and the Future Ocean
Volume 9 - 2022 | https://doi.org/10.3389/fmars.2022.860826
Seagrasses have experienced major losses globally mostly attributed to human impacts. Recently they are also associated with marine heat waves. The paucity of information on seagrass mortality thermal thresholds prevents the assessment of the risk of seagrass loss under marine heat waves. We conducted a synthesis of reported empirically- or experimentally-determined seagrass upper thermal limits (Tlimit) and tested the hypothesis that they increase with increasing local annual temperature. We found that Tlimit increases 0.42± 0.07°C per°C increase in in situ annual temperature (R2 = 0.52). By combining modelled seagrass Tlimit across global coastal areas with current and projected thermal regimes derived from an ocean reanalysis and global climate models (GCMs), we assessed the proximity of extant seagrass meadows to their Tlimit and the time required for Tlimit to be met under high (RCP8.5) and moderate (RCP4.5) emission scenarios of greenhouse gases. Seagrass meadows worldwide showed a modal difference of 5°C between present Tmax and seagrass Tlimit. This difference was lower than 3°C at the southern Red Sea, the Arabian Gulf, the Gulf of Mexico, revealing these are the areas most in risk of warming-derived seagrass die-off, and up to 24°C at high latitude regions. Seagrasses could meet their Tlimit regularly in summer within 50-60 years or 100 years under, respectively, RCP8.5 or RCP4.5 scenarios for the areas most at risk, to more than 200 years for the Arctic under both scenarios. This study shows that implementation of the goals under the Paris Agreement would safeguard much of global seagrass from heat-derived mass mortality and identifies regions where actions to remove local anthropogenic stresses would be particularly relevant to meet the Target 10 of the Aichi Targets of the Convention of the Biological Diversity.
Seagrass rank among the most threatened habitats in the biosphere, with about 19% to 29% of the global monitored area lost since the 1940’s (Waycott et al., 2009, Dunic et al., 2021). The bulk of losses are attributable to the wasting disease that devastated Zostera marina meadows in the 1930’s (Tutin 1942) and eutrophication-driven losses (Orth et al., 2006). However, there are growing reports of seagrass mortality associated with marine heat waves, including mortality of Posidonia oceanica in the Western Mediterranean following the 2003 heat wave (Díaz-Almela et al., 2009; Marbà and Duarte, 2010), mass mortality of Amphibolis antarctica in Shark Bay following an unprecedented marine heat wave in 2010/2011 (Thomson et al., 2015), and warming has been implicated in recent mass mortality of shallow Thalassia testudinum meadows in Florida Bay (Carlson et al., 2018) and Zostera marina and Ruppia maritima in Chesapeake Bay (Moore and Jarvis, 2008; Moore et al., 2014).
Knowledge on warming induced mortality on seagrasses is lagging behind that for other marine organisms (e.g. corals, Hughes et al., 2018; Lough et al., 2018), in part because reports of seagrass mass-mortality events are more recent. However, there is evidence that these impacts are propagating across the ocean and that marine heat waves will become more prevalent and intense (Frölicher et al., 2018; Oliver et al., 2018). A first step, required to determine the risk of seagrass mortality under marine heat waves, is to determine the thermal thresholds for seagrass mortality and morbidity. In contrast to reef-building corals, whose distribution is restricted to the subtropics and tropics and, therefore, experience relatively narrow climatic conditions and comparatively uniform thermal niches (Spalding et al., 2001), seagrasses occur from polar regions (in the northern hemisphere) to the Equator, thereby experiencing broad thermal regimes (e.g. Lee et al., 2007; Olesen et al., 2015). For shallow marine species, both upper and lower thermal limits generally decrease with latitude toward the poles and with decreasing seawater temperature (Stuart-Smith et al., 2017). This pattern has been observed to be consistent with respect to both fundamental thermal limits (i.e. based on laboratory experiments; Sunday et al., 2011), and realized thermal limits (i.e. based on species distributions; Stuart-Smith et al., 2017), across a range of animal taxa including fishes, benthic and pelagic invertebrates (Sunday et al., 2011; Stuart-Smith et al., 2017). To date, however, large-scale geographic patterns in the thermal limits of marine plants remain untested.
Here we test the hypothesis that seagrass upper thermal limits increase with geographically increasing annual mean seawater temperature populations are exposed to, and assess the proximity of extant seagrass meadows to their upper thermal limits as well as the time required for these thermal limits to be met under “business as usual” and moderate emission scenarios of greenhouse gases emissions (RCP8.5 and RCP4.5, respectively; Collins et al., 2013). The “business as usual” emission scenario is the one more closely reflecting realized emission trajectories. We do so by combining a synthesis of reported empirically- or experimentally- determined thermal limits for seagrass with current and future thermal regimes derived from an ocean reanalysis and global climate models (GCMs).
We compiled the available seagrass upper thermal limits (Tlimit) published in the literature by conducting a search using Web of Knowledge with the keywords combinations seagrass AND (temperature OR warming) and seagrass AND (“thermal limit” OR “thermal threshold” OR “critical temperature” OR “thermal niche”) and by screening the reference list of relevant papers found in these searches. We only included data of seagrass populations growing submersed within their native geographical range. We excluded the few studies of intertidal meadows because available evidence suggests they have higher thermal tolerance than submersed ones in order to cope with atmospheric temperatures (e.g. Massa et al., 2009). Tlimit were derived from empirical observations of seagrass die-off events attributed to heat waves, in combination with other simultaneous stressors (hypersalinity, Carlson et al., 2018; low light availability, Moore and Jarvis, 2008; Moore et al., 2014), or mesocosm experiments (Table 1). Seagrasses in mesocosm experiments were exposed to at least 2 temperature treatments above average in situ summer temperature that extended the experimental thermal range beyond the Tlimit. Seagrasses were exposed to experimental temperatures for 6 to 120 days depending on the study (see Dataset, Marbà et al 2022). The Tlimit was defined as: a) the upper temperature at which shoot survival, shoot growth or biomass above optimal temperature started to decline in experimental studies; or b) the seawater temperature during the heat wave that triggered die-off events. We assume that the thermal limit for growth is close to the temperature that induces mortality.
Table 1 Seagrass species, location and coordinates of the population studied, metric assessed, approach used to estimate the upper thermal limit (0: experimental, 1: mass die-off observation) and data source.
For each study, the compiled dataset (Marbà et al 2022) includes the species name, location and coordinates of the population studied, the Tlimit, the approach (i.e. experimental or empirical), the year the study was conducted and the data source. For experimental studies, the dataset also includes the temperature treatments seagrasses were exposed to. For each population studied, we obtained mean annual seawater temperature values for the 5 years before the thermal tolerance experiment or observation was conducted (Marbà et al 2022) from the ORAS4 ocean reanalysis (Balmaseda et al., 2013), which provides monthly 3D temperature global fields from 1958 to present with a spatial resolution of 1° in the horizontal and ~10 m in the vertical. Those temperatures aim at representing the regional characteristics, rather than the local features which cannot be captured by the coarse spatial resolution of ORAS4. We used least square regression analysis to fit the relationship between in situ 5 yr-mean annual temperature (x) and the seagrass Tlimit (y) and assess the deviation from the overall trend of temperate and tropical affinity seagrass floras by examining the residuals of this relationship. We used this empirical relationship to estimate Tlimit for seagrasses globally given in situ mean annual thermal regimes at present in all the locations potentially suitable for seagrass survival in terms of light availability as defined by Gattuso et al. (2006).
We characterised the present thermal conditions of coastal areas using the ORAS4 ocean reanalysis (Balmaseda et al., 2013). The mean maximum summer temperature (Tmax), defined as the temperature of the warmest month of the year at the model grid point closer to each location (in geographical coordinates and water depth), has been computed for all locations and averaged for the 1980-2005 period. For the future conditions we used 25 simulations from the CMIP5 ensemble of global climate simulations (Taylor et al., 2012) under the RCP8.5 (“business as usual”) and RCP4.5 (moderate) scenarios of greenhouse gases emissions. The temperature change projected by each model has been computed as the difference between the mean maximum summer temperature in the period 2075-2100 and 1980-2005. The ensemble of 3D anomaly fields was then interpolated to the suitable locations and averaged among models. This provides the most likely future mean maximum summer temperature at each location under the RCP8.5, which best reflects the emission trajectory to-date, and RCP4.5 scenarios.
We calculate the thermal safety margins of seagrass populations at present as the difference between the estimated population Tlimit and mean maximum summer temperature for the period 1980-2005 (Tmax, °C). We estimate the vulnerability of seagrass populations to global warming under RCP4.5 and RCP8.5 as the time (years) needed for in situ mean maximum summer temperature to equal population Tlimit.
The data set on seagrass thermal limits compiled included 35 estimates of Tlimit of seagrass species, of which 6 were empirical, determined from the seawater temperature at which die-offs were observed, and 29 were derived experimentally, under temperature-controlled experiments (Table 1). These estimates correspond to 15 species distributed from the subarctic (Zostera marina) to the tropics (e.g. Thalassodendron ciliatum, Thalassia hemprichii, Cymodocea serrulata and Enhalus acoroides) (Figure 1 and Table 1).
Figure 1 Average upper thermal limits, empirically- and experimentally- determined, across seagrass species with temperate (squares) and tropical (circles) affinity. Overall values for tropical and temperate affinity seagrasses are also provided. The number of observations (N) per species is indicated within brackets. Error bars show the standard error of the mean (N≥3) or standard deviation (N=2).
The observed Tlimit (°C) ranged 13°C, from 25°C to 38°C, across the data set, and increased strongly with in situ mean annual seawater temperature experimental and empirical populations were exposed to (Tannual, °C, Figure 2) as described by the fitted linear regression equation,
Figure 2 The relationship between local annual temperature (°C) (average 5 years before plant experiment/observation) and reported upper thermal limits (°C) of seagrass species with temperate (squares) and tropical (circles) affinity. The solid line represents the fitted regression line (Eq. 1), and the colours represent estimates for different seagrass species: AA, Amphibolis antarctica; AG, A. griffithii; CN, Cymodocea nodosa; CS, C. serrulata; EA, Enhalus acoroides; HS, Halophila stipulacea; PO, Posidonia oceanica; SF, Syringodium filiforme; TH, Thalassia hemprichii; TT, T. testudinum; TC, Thalassodendron ciliatum; ZJ, Zostera japonica; ZM, Z. marina; ZMu, Z.muelleri and ZM and RM, Z. marina and Ruppia maritima.
However, the upper thermal limits of seagrass flora of temperate (N=24) and tropical (N=11) affinity deviate by -1.15 ± 0.44°C and +2.53 ± 0.57°C (average ± SE) respectively, from those predicted by the overall seagrass flora relationship (Eq.1).
This equation (Eq.1) allows determination of Tlimit for seagrass meadows globally (Figure 3A), as well as the thermal scope with future climate change (i.e. current thermal safety margin or the degrees of warming required to reach Tlimit, Figure 3B) provided the mean maximum summer temperatures at present (1980-2005, Tmax °C, Supplementary Figure 1). This difference has a strong dependency on latitude (Supplementary Figure 2), as the realized Tmax is more than 20°C cooler than Tlimit at high latitude regions like the Barents and Labrador Seas or Patagonia (Figure 3B). Whereas the modal temperature gap between present Tmax and Tlimit is 5°C (Figure 3C), some regions have realized Tmax much closer (< 3°C) to the expected Tlimit. These regions include the Arabian Gulf, the Red Sea, the northern part of the Gulf of Mexico and the coasts of Florida and California (Figure 3B), where the risks of mass-mortality associated with warming are greatest for seagrass species.
Figure 3 (A) Distribution of predicted thermal limits around potential seagrass sites; (B) the temperature difference between the predicted thermal limits and the present mean maximum summer temperature around potential seagrass sites; and (C) the frequency distribution (expressed as percentage of global coastal area) of this difference.
Global climate simulations, under the current “business as usual” scenario, project sea-surface warming by 2100 ranging from about 1°C to 4 °C in potential seagrass habitat (Figure 4A). Calculation of the time required, under the “business as usual” scenario, for seawater annual maximum summer temperature in seagrass meadows to reach Tlimit ranges from decades to more than 300 years at high latitude areas (Figure 4B), being on average 187 years with a standard deviation of 70 years (Figure 4C), although the estimates for long-periods are less accurate (Supplementary Figure 3) because numerical simulations rarely exceed year 2100. The seagrass regions where Tmax will meet Tlimit sooner under “business as usual” emission scenarios are, as expected, the Arabian Gulf and southern Red Sea, along with the Gulf of Mexico and the coast of Florida, where Tlimit will be reached within 50-60 years (Figure 4B). In addition, Tlimit will be reached, on average, every summer before end of the 21st Century across the entire Coral Triangle, extending from northern Australia across SE Asia, as well the Arabian Sea and the Bay of Bengal, the Pacific coast of Central America, the Eastern Mediterranean and the Adriatic Sea. On the other hand, seagrass meadows growing in the boreal and subarctic regions will not meet their Tlimit within the next 200 years. Because the deviation of temperate and tropical affinity seagrass species from the overall relationship between seagrass Tlim and Tannual (Eq. 1), the time required for seawater mean maximum summer temperature to reach Tlim under the “business as usual” scenario in coastal areas where both floras co-exist (e.g. Mediterranean Sea, Queensland) will vary depending on species thermal affinity (Supplementary Figure 4). Conversely, under a moderate scenario of greenhouse gases emissions (RCP 4.5) climate models project Tmax will meet Tlim within few decades only in the Arabian Gulf and during the 21st Century in the Gulf of Mexico and South of the Red Sea (Supplementary Figure 5). In regions where temperate and tropical affinity flora co-exists, the time for Tmax meeting Tlim of temperate affinity seagrasses projected under a moderate warming scenario exceeds 100 years in most of the potential seagrass sites (Supplementary Figure 6).
Figure 4 (A) Predicted mean maximum summer temperature increase under the RCP8.5 scenario in 2100 relative to present; (B) the time (in years) to reach Tlimit at the warming rates predicted under the RCP8.5 scenario around potential seagrass sites; and (C) frequency distribution (expressed as percentage of global coastal area) of the time to reach Tlimit at the warming rates predicted under the RCP8.5 scenario.
Experimental and empirical assessments confirm that Tlimit, the thermal tolerance limit of seagrasses, varies from 25°C to 38°C. The distribution of residuals in the relationship between seagrass Tlimit and Tannual shows considerable variability in Tlimit in regions where in situ annual temperatures are between 16°C and 22°C (Figure 2). This corresponds to higher thermal limits than expected for the species of tropical affinity, such as Cymodocea nodosa, growing in the Mediterranean Sea. This variability is expected to drive major shifts in seagrass flora in regions where temperate and tropical affinity floras co-exist such are the Mediterranean Sea and the Eastern coast of Queensland, particularly under a “business as usual” scenario of greenhouse gases emissions (Supplementary Figures 4, 6). Indeed, recent projections, based on empirical thermal niche models, predict that Posidonia oceanica will be functionally extinct in the Mediterranean Sea, while C. nodosa will spread into some regions (Chefaoui et al., 2018), consistent with the higher Tlimit for this species.
The very high thermal limits (25°C) of subarctic seagrass, Zostera marina, are somewhat unexpected, given the low maximum seawater temperatures (9°C to 15°C) in western Greenland where eelgrass occurs (Olesen et al., 2015). The general pattern that species tend to have thermal optima close to the mean maximum temperature in the habitats they occupy (e.g. Thomas et al., 2012) does not apply to Z. marina in western Greenland. Indeed, the thermal limit of 25°C of Zostera marina in western Greenland is similar to that in Denmark, where summer temperature is 5-10°C warmer. Similarly, the thermal limit of Z. marina in western Greenland is only 5°C lower than the temperature reported to cause Z. marina die-off in Chesapeake Bay, where average summer temperatures (26-27°C, Moore and Jarvis, 2008; Moore et al., 2014) are 12 -18°C higher than those in Greenland. Comparable Tlimit of Z. marina in western Greenland to northern Europe is consistent with the inference that the subarctic stands are relatively recent (Marbà et al., 2018), and imply that future warming will propel the expansion of these meadows in the subarctic (Krause-Jensen and Duarte, 2014; Olesen et al., 2015), as supported by recent evidence that these meadows are indeed expanding (Marbà et al., 2018). However, common garden and transplant experiments with Z. marina have shown that equator-ward populations may recover faster from similar heat shock than pole-ward ones (Franssen et al., 2011; Jueterbock et al., 2016) and, therefore, these differences could alter seagrass vulnerability to warming. The information on how differences in recovery and resilience may affect seagrass vulnerability to warming is still scarce and a greater understanding of these dynamics will improve estimates of seagrass population risk of mortality at finer spatial and temporal scales.
The data set used to derive the in situ annual temperature-dependence of seagrass Tlimit suffers from a number of limitations, in terms of the number of species assessed (15 species out of some 60 extant seagrass species), the level of replication for species growing under different conditions of temperature (only Amphibolis antarctica, C. nodosa, P. oceanica, T. testudinum, and Z. marina were assessed across multiple locations), variability in experiment duration and the precision of the experimental and empirical Tlimit estimates, which respectively depend on the temperature interval experimentally assessed and population monitoring frequency. Despite these limitations, the current estimates represent the first effort at providing seagrass Tlimit estimates, which need be refined with additional estimates in the future, but are likely robust, since experimentally determined Tlimit estimates were comparable to empirically-determined ones for the same species when exposed to similar thermal regimes (P. oceanica, T. testudinum, Z marina, Marbà et al 2022). For instance, three experimentally-determined Tlimit estimates are available for P. oceanica shoots (Bennett et al., 2022, Olsen et al., 2012, Savva et al., 2018) and two estimates were derived from field monitoring observations (Díaz-Almela et al., 2009; Marbà and Duarte, 2010), with a very close agreement among them. Whereas Tlimit estimates are available for only one in 4 seagrass species, the species included in our study represent the dominant species across many regions (e.g. P. oceanica in the Mediterranean, Z. marina in the Atlantic, T. testudinum in the Caribbean, and Enhalus acoroides and T. hemprichii in the Indo-Pacific).
The thermal limits of tropical and subtropical seagrass species (34.7 ± 0.51°C, range 32 to 38°C, Figure 1) are somewhat warmer than those for reef-building corals, which are generally assumed to experience bleaching and mortality with seawater temperatures in excess of 29°C to 30°C (Hoegh-Guldberg, 1999). Indeed, we are not aware of any report of mass mortality of seagrass meadows in the Great Barrier Reef during the 2015/2016 massive coral bleaching that devastated much of the corals in this region (Hughes et al., 2017). In fact, Eq. 1 indicates that the Tlimit of the seagrasses growing at the region of the Great Barrier Reef would be 33.9°C given the average summer seawater temperature in the region for the period 1980 - 2016 (28.8°C, Hughes et al., 2017). Hence, we suggest that seagrass habitats are comparatively more robust to warming than reef-building coral are. Yet, mass mortalities of shallow seagrass meadows have been reported since the turn of the century, affecting Posidonia oceanica following the 2003 heat wave in the Western Mediterranean (Díaz-Almela et al., 2009; Marbà and Duarte, 2010), mass mortality of Amphibolis antarctica in Shark Bay following an unprecedented marine hat wave in 2010/2011 (Thomson et al., 2015; Arias-Ortiz et al., 2018), and shallow Thalassia testudinum meadows in Florida Bay in 2015 (Carlson et al., 2018) and Zostera marina and Ruppia maritima in Chesapeake Bay in 2005 (Moore and Jarvis, 2008; Moore et al., 2014.).
Extrapolation, using the relationship between seagrass Tlimit and Tmax, of the thermal scope of seagrass meadows worldwide showed a modal difference of 5°C between present Tmax and seagrass Tlimit. However, this comparison identifies the southern Red Sea, the Arabian Gulf, the Gulf of Mexico and the coast of Florida as the areas most at risk of warming-derived seagrass die-off. Because of their lower thermal thresholds, the time for coral reefs to approach seawater warming levels causing widespread mortality is far more proximal, if not reached already, than that of seagrass, which will meet their thermal limits regularly in summer in 50-60 years, for the areas most at risk, to more than 200 years for the Arctic under “business as usual” scenario of ocean warming. Seagrass vulnerability to global warming in the areas most at risk, however, will be largely reduced under a moderate scenario of greenhouse gases emissions. Hence, meeting the targets of the Paris agreement is likely to help prevent major losses from warming-derived die-off in seagrass meadows.
Our analysis, however, is focused on the mean maximum summer temperature and does not consider the intensity and duration heat waves, which have thus far generated mass die-off of seagrass (e.g. Thomson et al., 2015; Smale et al., 2019). Heat waves are indeed projected to become more frequent and intense in the future (Frölicher et al., 2018; Oliver et al., 2018) and knowledge on biological responses to heat wave physical attributes may refine future projections of seagrass mortality risk. However, this enhancement of heat waves will be mainly due to the increase in the mean temperature, while changes in the intra-seasonal variability are expected to play a secondary role (Jordà et al., 2012; Darmaraki et al., 2019). Therefore, a modelling approach based on mean maximum temperature is sound in terms of providing expectations for the future, where indeed episodic die-off events will become increasingly common. We used the “business as usual” (RCP8.5, IPCC, 2014) warming scenario and a more benign scenario consistent with the expectations of the Paris Agreement (RCP4.5), despite five years after this agreement was reached there is no evidence that emissions are curving below those in 2015 and indeed emissions reached a historical maximum in 2017 (Olivier et al., 2017; IEA, 2018). However, our results demonstrate that implementing the goals under the Paris Agreement will help safeguard much of global seagrass from heat-derived mass mortality. Moreover, our assessment does not consider the likely poleward migration of species, whether through natural propagation or assisted by human introductions. For instance, the occurrence of the exotic Halophila stipulacea from the Red Sea (Tlimit 38°C) in the Eastern Mediterranean may ensure continuity of ecological functions and services (Wesselmann et al., 2021) associated with seagrass habitats against the predicted loss of Posidonia oceanica (average Tlimit 28°C) with future warming (Jordà et al., 2012; Chefaoui et al., 2018). Moreover, the decadal time scales involved before seagrass reach Tlimit values even under the “business as usual” scenario, may allow adaptation, particularly so for the small, fast-growing species, such as Z. noltii or Halophila species (Duarte et al., 2018; Wesselmann et al., 2020). The vulnerability of P. oceanica to further Mediterranean warming is unlikely to be mitigated by rapid evolution, due to the very long generation times and slow evolutionary rates of this species (Aires et al., 2011; Arnaud-Haond et al., 2012). However, C. nodosa, also growing in the Mediterranean, has Tlimit values 4°C to 6°C above those of P. oceanica, and may, if warming continues to progress following “business as usual” trajectories, replace areas from which P. oceanica may be lost (Chefaoui et al., 2018). The likelihood that species losses may be compensated by climate migrants adapted to warmer regimes, and thus wider thermal safety margins, does not apply, however, to the regions identified as closest to reaching Tlimit values, such as the southern Red Sea, Arabian Gulf and Gulf of Mexico, which flora is already of tropical origin and present the highest Tlimit values among the seagrass flora.
The vulnerability of seagrass meadows to global warming is assessed here considering the thermal sensitivity of seagrass growth and survival and future scenarios of global seawater temperature. However, the demographic dynamics of seagrass populations, despite being clonal plants, also relies on sexual reproduction. The response of seagrass reproductive effort to warming has been examined for few species with contrasting results. Examination of sensitivity Z marina reproduction to global warming for the period (2002-2018) in Korea (Qin et al., 2020) demonstrated a negative trend in reproductive intensity with increasing temperature and experimental evidence suggests that warming may limit seed-based recruitment of H. ovalis (Statton et al., 2017). Conversely, field (Díaz-Almela et al., 2007) and experimental (Ruiz et al., 2018) observations demonstrated that P. oceanica flowering (and recruitment of new clones) was enhanced by increasing temperature when the upper thermal limit for seagrass survival is exceeded. However, the temperature-driven increase of P. oceanica sexual recruits was far insufficient to compensate for adult mass mortality (Díaz-Almela et al., 2007). Despite current evidence suggests little scope for reproductive effort to compensate seagrass mass-mortality driven by climate change, improved understanding of thermal dependence of reproductive effort across seagrass flora is needed to improve predictions of seagrass vulnerability to climate change, particularly for fast-growing species with clones rapidly spreading and shorter generation times that may allow for thermal adaptation within few decades (Wesselmann et al., 2020).
The experimental thermal limits used here are derived from seagrass growth and survival responses to temperature under full light conditions and no other stressors. However, there is evidence of additive or synergetic seagrass responses to temperature and irradiance (Moreno-Marín et al., 2018), salinity (e.g. Koch et al., 2007; Ontoria et al., 2020), sediment sulphide concentration (e.g., Koch and Ersike, 2001; García et al., 2012) or nutrient availability (Moreno-Marín et al., 2018) suggesting that seagrass thermal limits could vary under other stressing conditions. Indeed, thermal tolerance of Z. marina decreases when sediment sulphides increase (Pulido and Borum, 2010) and under low light availability (Beca-Carretero et al., 2018).
The in situ temperature-dependence of seagrass thermal limits reported here represents a useful resource to assess the risk of warming-derived die-offs of seagrass meadows. The seagrass meadows most at risk are also identified here at a coarse, regional, level. Within these regions, shallow meadows often experience temperatures close to their Tlimit sooner than deep meadows, although rapid downward penetration of warm surface isotherms is also threatening deeper habitats (Jorda et al., 2020). This may require adapting monitoring programs to encompass this risk, as monitoring programs across Europe and other regions focus on the depth limit of seagrass meadows, since this is most vulnerable to eutrophication, the main driver of seagrass decline in the past 40 years (Orth et al., 2006). The Target 10 of the Aichi Targets of the Convention of the Biological Diversity calls for reducing multiple anthropogenic pressures on ecosystems vulnerable to be impacted by climate change, so as to maintain their integrity and functioning by 2015 (https://www.cbd.int/sp/targets/). Our assessment also serves this goal by identifying regions (the southern Red Sea, Arabian Gulf and Gulf of Mexico), where seagrasses are closest to their Tlimit. Hence removing anthropogenic stresses, such as pollutants derived from industrial and domestic sources as well as mechanical damages from fishing and coastal development, is particularly important in these areas.
The dataset compiled is publicly available at Digital.CSIC repository: http://hdl.handle.net/10261/265646 (Marbà et al 2022).
NM, CD, SB, and GJ conceived the study. NM compiled and analyzed experimental and empirical seagrass data. GJ computed temperature data, seagrass thermal safety margins and seagrass vulnerability under GHG emission scenarios. CD and NM wrote the manuscript with contributions from all authors. All authors contributed to the article and approved the submitted version.
This work was funded by the Spanish Ministry of Economy, Industry and Competivness with the projects MedShift (CGL2015-71809-P), SumaEco (RTI2018-095441-B-C21) and Clifish (CTM2015-66400-C3-2-R), the European Union’s Horizon 2020 SOCLIMPACT project (grant agreement No 776661) and the King Abdullah University of Science and Technology (KAUST subaward number 3834). SB was supported by a Juan de la Cierva Formación contract funded by the Spanish Ministry of Economy, Industry and Competitiveness.
The authors declare that the research was conducted in the absence of any commercial or financial relationships that could be construed as a potential conflict of interest.
All claims expressed in this article are solely those of the authors and do not necessarily represent those of their affiliated organizations, or those of the publisher, the editors and the reviewers. Any product that may be evaluated in this article, or claim that may be made by its manufacturer, is not guaranteed or endorsed by the publisher.
We thank Oscar Serrano for providing the seawater temperature recorded when Amphibolis antarctica die-off was observed at Shark Bay (Australia).
The Supplementary Material for this article can be found online at: https://www.frontiersin.org/articles/10.3389/fmars.2022.860826/full#supplementary-material
Abe M., Kurashima A., Maegawa M. (2008). High Water-Temperature Tolerance in Photosynthetic Activity of Zostera Marina Seedlings from Ise Bay, Mie Prefecture, Central Japan. Fish. Sci. 74, 1017–1023. doi: 10.1111/j.1444-2906.2008.01619.x
Abe M., Yokota K., Kurashima A., Maegawa M. (2009). High Water Temperature Tolerance in Photosynthetic Activity of Zostera Japonica Ascherson and Graebner Seedlings From Ago Bay, Mie Prefecture, Central Japan. Fish. Sci. 75 (5), 1117–1123. doi: 10.1007/s12562-009-0141-x
Aires T., Marbà N., Cunha R. L., Kendrick G. A., Walker D. I., Serrão E. A., et al. (2011). Evolutionary History of the Seagrass Genus Posidonia. Mar. Ecol. Prog. Ser. 421, 117–130. doi: 10.3354/meps08879
Arias-Ortiz A., Serrano O., Masqué P., Lavery P. S., Mueller U., Kendrick G. A., et al. (2018). A Marine Heat Wave Drives Massive Losses From the World’s Largest Seagrass Carbon Stocks. Nat. Climate Change 8, 338–344. doi: 10.1038/s41558-018-0096-y
Arnaud-Haond S., Duarte C. M., Diaz-Almela E., Marbà N., Sintes T., Serrão E. A. (2012). Implication of Extreme Life Span in Clonal Organisms: Millenary Clones in Meadows of the Threatened Seagrass Posidonia Oceanica. PlosONE 7 (2), e30454. doi: 10.1371/journal.pone.0030454
Balmaseda M. A., Mogensen K., Weaver A. T. (2013). Evaluation of the ECMWF Ocean Reanalysis System ORAS4. Q. J. R. Meteorol. Soc. 139, 1132–1161. doi: 10.1002/qj.2063
Beca-Carretero P., Olesen B., Marbà N., Krause-Jensen D. (2018). Response to Experimental Warming in Northern Eelgrass Populations: Comparison Across a Range of Temperature Adaptations. Mar. Ecol. Prog. Ser. 589, 59–72. doi: 10.3354/meps12439
Bennett S., Vaquer-Sunyer R., Jorda G., Forteza M., Roca G., Marbà N. (2022). Thermal Performance of Seaweeds and Seagrasses Across a Regional Climate Gradient. Front. Mar. Sci. 9. doi: 10.3389/fmars.2022.733315
Carlson D. F., Yarbro L. A., Scolaro S., Poniatowski M., McGee-Absten V., Carlson Jr. P. R. (2018). Sea Surface Temperatures and Seagrass Mortality in Florida Bay: Spatial and Temporal Patterns Discerned From MODIS and AVHRR Data. Remote Sens. Envi. 208, 171–188. doi: 10.1016/j.rse.2018.02.014
Chefaoui R. M., Duarte C. M., Serrao E. A. (2018). Dramatic Loss of Seagrass Habitat Under Projected Climate Change in the Mediterranean Sea. Global Change Biol. 24, 4919 – 4928. doi: 10.1111/gcb.14401
Collins M., Knutti R., Arblaster J., Dufresne J. L., Fichefet T., Friedlingstein P., et al. (2013). “Long-Term Climate Change: Projections, Commitments and Irreversibility,” in Climate Change 2013: The Physical Science Basis. Contribution of Working Group I to the Fifth Assessment Report of the Intergovernmental Panel on Climate Change. Eds. Stocker T. F., Qin D., Plattner G. K., Tignor M., Allen S. K., Boschung J., Midgley P. M. (Cambridge: Cambridge University Press), 1029–1136.
Darmaraki S., Somot S., Sevault F., Nabat P., Cabos Narvaez W. D., Cavicchia L., et al. (2019). Future Evolution of Marine Heatwaves in the Mediterranean Sea. Clim. Dyn. 53, 1371–1392. doi: 10.1007/s00382-019-04661-z
Díaz-Almela E., Marbà N., Duarte C. M. (2007). Consequences of Mediterranean Warming Events in Seagrass (Posidonia Oceanica) Flowering Records. Global Change Biol. 13, 224–235. doi: 10.1111/j.1365-2486.2006.01260.x
Díaz-Almela E., Marbà N., Martínez R., Santiago R., Duarte C. M. (2009). Seasonal Dynamics of Posidonia Oceanica in Magalluf Bay (Mallorca, Spain): Temperature Effects on Seagrass Mortality. Limnol. Oceanog. 54 (6), 2170–2182. doi: 10.4319/lo.2009.54.6.2170
Duarte B., Martins I., Rosa R., Matos A. R., Roleda M. Y., Reusch T., et al. (2018). Climate Change Impacts on Seagrass Meadows and Macroalgal Forests: An Integrative Perspective on Acclimation and Adaptation Potential. Front. Mar. Sci. 5:190. doi: 10.3389/fmars.2018.00190
Dunic J. C., Brown C. J., Connolly R. M., Turschwell M. P., Côté I. M. (2021). Long-Term Declines and Recovery of Meadow Area Across the World’s Seagrass Bioregions. Glob. Change Biol. 27, 4096–4109. doi: 10.1111/gcb.15684
Franssen S. U., Gu J., Bergmann N., Winters G., Klostermeier U. C., Rosenstiel P., et al. (2011). Transcriptomic Resilience to Global Warming in the Seagrass Zostera Marina, a Marine Foundation Species. Proc. Natl. Acad. Sci. 108, 19276–19281. doi: 10.1073/pnas.1107680108
Frölicher T. L., Fischer E. M., Gruber N. (2018). Marine Heatwaves Under Global Warming. Nature 560, 360–364. doi: 10.1038/s41586-018-0383-9
García R., Sánchez-Camacho M., Duarte C. M., Marbà N. (2012). Warming Enhances Sulphide Stress of Mediterranean Seagrass (Posidonia Oceanica). Estuar. Coast. Shelf. Sci. 113, 240–247. doi: 10.1016/j.ecss.2012.08.010
Gattuso J. P., Gentili B., Duarte C. M., Kleypas J. A., Middelburg J. J., Antoine D. (2006). Light Availability in the Coastal Ocean: Impact on the Distribution of Benthic Photosynthetic Organisms and Their Contribution to Primary Production. Biogeosciences 3, 489–513. doi: 10.5194/bg-3-489-2006
George R., Gullström M., Mangora M. M., Mtolera M. S. P., Björk M. (2018). High Midday Temperature Stress has Stronger Effects on Biomass Than on Photosynthesis: A Mesocosm Experiment on Four Tropical Seagrass Species. Ecol. Evol. 8, 4508–4517. doi: 10.1002/ece3.3952
Guerrero-Meseguer L., Marín A., Sanz-Lázaro C. (2017). Futrue Heat Waves Due to Climate Change Threaten the Survival of Posidonia Oceanica Seedlings. Environ. Pollut. 230, 40–45. doi: 10.1016/j.envpol.2017.06.039
Hammer K. J., Borum J., Hasler-Sheetal H., Shields E. C., Sand-Jensen K., Moore K. A. (2018). High Temperatures Case Reduced Growth, Plant Death and Metabolic Changes in Eelgrass Zostera Marina. Mar. Ecol. Prog. Ser. 604, 121–132. doi: 10.3354/meps12740
Hernán G., Ortega M. J., Gánada A. M., Castejón I., Terrados J., Tomas F. (2017). Future Warmer Seas: Increased Stress and Susceptibility to Grazing in Seedlings of a Marine Habitat-Forming Species. Global Change Biol. 23, 4530–4543. doi: 10.1111/gcb.13768
Hoegh-Guldberg O. (1999). Climate Change, Coral Bleaching and the Future of the World’s Coral Reefs. Mar. Freshwat. Res. 50, 839–866. doi: 10.1071/MF99078
Höffe H., thomsen M. S., Holmer M. (2011). High Mortality of Zostera Marina Under High Temperature Regimes But Minor Effects of the Invasive Macroalgae Graciliaria Vermiculophylla. Estur. Coast. Shelf. Sci. 92, 25–46. doi: 10.1016/j.ecss.2010.12.017
Hughes T. P., Kerry J. T., Álvarez-Noriega M., Álvarez-Romero J. G., Anderson K. D., Baird A. H., et al. (2017). Global Warming and Recurrent Mass Bleaching of Corals. Nature 543, 373–377. doi: 10.1038/nature21707
Hughes T. P., Kerry J. T., Baird A. H., Connolly S. R., Dietzel A., Eakin C. M., et al. (2018). Global Warming Transforms Coral Reef Assemblages. Nature 556, 492–496. doi: 10.1038/s41586-018-0041-2
International Energy Agency (IEA) (2018) Global Energy and CO2 Status Report 2017. Available at: http://www.iea.org/publications/freepublications/publication/GECO2017.pdf.
IPCC. (2014). Climate Change 2014: Synthesis Report. Contribution of Working Groups I, II and III to the Fifth Assessment Report of the Intergovernmental Panel on Climate Change [Core Writing Team, Pachauri R. K., Meyer L. A. (eds.)]. IPCC, Geneva, Switzerland, 151 pp.
Jorda G., Marbà N., Bennett S., Santana-Garcon J., Agusti S., Duarte C. M. (2020). Ocean Warming Compresses the Three-Dimensional Habitat of Marine Life. Nat. Ecol. Evol. 4, 109–114. doi: 10.1038/s41559-019-1058-0
Jordà G., Marbà N., Duarte C. M. (2012). Mediterranean Seagrass Vulnerable to Regional Climate Warming. Nat. Climate Change 2, 821–824. doi: 10.1038/Nclimate1533
Jueterbock A., Franssen S. U., Bergmann N., Gu J., Coyer J. A., Reusch T. B. H., et al. (2016). Phylogeographic Differentiation Versus Transcriptomic Adaptation to Warm Temperatures in Zostera Marina, a Globally Important Seagrass. Mol. Ecol. 25, 5396–5411. doi: 10.1111/mec.13829
Koch M. S., Ersike J. M. (2001). Sulfide as a Phytotoxin to the Tropical Seagrass Thalassia Testudinum: Interactions With Light, Salinity and Temperature. J. Expriment. Biol. Ecol. 266, 81–95. doi: 10.1016/S0022-0981(01)00339-2
Koch M. S., Schopmeyer S., Kyhn-Hansen C., Madden C. J. (2007). Synergistic Effects of High Temperatura and Sulfide on Tropical Seagrass. J. Exp. Mar. Biol. Ecol. 341, 91–101. doi: 10.1016/j.jembe.2006.10.004
Krause-Jensen D., Duarte C. M. (2014). Expansion of Vegetated Coastal Ecosystems in the Future Arctic. Front. Mar. Sci. 1. doi: 10.3389/fmars.2014.00077
Lee K. S., Park S. R., Kim Y. K. (2007). Effects of Irradiance, Temperature, and Nutrients on Growth Dynamics of Seagrasses: A Review. J. Exp. Mar. Biol. Ecol. 350, 144–175. doi: 10.1016/j.jembe.2007.06.016
Lough J. M., Anderson K. D., Hughes T. P. (2018). Increasing Thermal Stress for Tropical Coral Reefs: 1871–2017. Sci. Rep. 8, 6079. doi: 10.1038/s41598-018-24530-9
Marbà N., Duarte C. M. (2010). Mediterranean Warming Triggers Seagrass (Posidonia Oceanica) Shoot Mortality. Global Change Biol. 16, 2366–2375. doi: 10.1111/j.1365-2486.2009.02130.x
Marbà N., Krause-Jensen D., Masqué P., Duarte C. M. (2018). Expanding Greenland Seagrass Meadows Contribute New Sediment Carbon Sinks. Sci. Rep. 8, 14024. doi: 10.1038/s41598-018-32249-w
Marbà N., Gabriel J., Scott B., Duarte C. M. (2022). Seagrass Thermal Limits [dataset]; DIGITAL.CSIC. doi: 10.20350/digitalCSIC/14572
Massa S. I., Arnaud-Haond S., Pearson G. A., Serrao E. A. (2009). Temperature Tolerance and Survival of Intertidal Populations of the Seagrass Zostera Noltii (Hornemann) in Southern Europe (Ria Formosa, Portugal). Hydrobiologia 619, 195–201. doi: 10.1007/s10750-008-9609-4
McMillan C. (1984). The Distribution of Tropical Seagrasses With Relation to Their Tolerance of High Temperatures. Aquat. Bot. 19, 369–379. doi: 10.1016/0304-3770(84)90049-4
Moore K. A., Jarvis J. C. (2008). Environmental Factors Affecting Recent Summertime Eelgrass Diebacks in the Lower Chesapeake Bay: Implications for Long-Term Persistence. J. Coast. Res. 55, 135–147. doi: 10.2112/SI55-014
Moore K. A., Shields E. C., Parrish D. B. (2014). Impacts of Varying Esturaine Temperature and Light Conditions on Zostera Marina (Eelgrass) and its Interactions With Ruppia Maritima (Widgeongrass). Estuar. Coast. 37 (suppl1), S20–S30. doi: 10.1007/s12237-013-9667-3
Moreno-Marín F., Brun F. G., Pedersen M. F. (2018). Additive Response to Multiple Environmental Stressors in the Seagrass Zostera Marinal. Limnol. Oceanog. 63, 1528–1544. doi: 10.1002/lno.10789
Nejrup L. B., Pedersen M. F. (2008). Effects of Salinity and Water Temperature on the Ecological Performance of Zostera Marina. Aquat. Bot. 88, 239–246. doi: 10.1016/j.aquabot.2007.10.006
Niu S., Zhang P., Liu J., Guo D., Zhang X. (2012). The Effect of Temperature on the Survival, Growth, Photosynthesis, and Respiration of Young Seedlings of Eelgrass Zostera Marina L. Aquaculture 350-353, 98–108. doi: 10.1016/j.aquaculture.2012.04.010
Olesen B., Krause-Jensen D., Marbà N., Christensen P. B. (2015). Eelgrass (Zostera Marina L.) in Subarctic Greenland: Dense Meadows With Slow Biomass Turnover. Mar. Ecol. Prog. Ser. 518, 107–121. doi: 10.3354/meps11087
Oliver E. C. J., Donat M. G., Burrows M. T., Moore P. J., Smale D. A., Alexander L. V., et al. (2018). Longer and More Frequent Marine Heatwaves Over the Past Century. Nat. Commun. 9, 1324. doi: 10.1038/s41467-018-03732-9
Olivier J. G. J., Schure K. M., Peters J. A. H. W. (2017). Trends in Global CO2 and Total Greenhouse Gas Emissions: 2017 Report. The Hague: Pbl Netherlands Environmental Assessment Agency (The Hague). Available at: www.pbl.nl/en..
Olsen Y. S., Sánchez-Camacho M., Marbà N., Duarte C. M. (2012). Mediterranean Seagrass Growth and Demography Responses to Experimental Warming. Estuar. Coast. 35 (5), 1205–1213. doi: 10.1007/s12237-012-9521-z
Ontoria Y., Webster C., Said N., Ruiz J. M., Pérez M., Romero J., et al. (2020). Positive Effects of High Salinity can Buffer the Negative Effects of Experimental Warming on Functional Traits of the Seagrass Halophila Ovalis. Mar. Pollut. Bull. 158, 111404. doi: 10.1016/j.marpolbul.2020.111404
Orth R. J., Carruthers T. J. B., Dennison W. C., Duarte C. M., Fourqurean J. W., Heck Jr., et al. (2006). A Global Crisis for Seagrass Ecosystems. Bioscience 56, 987–996. doi: 10.1641/0006-3568(2006)56[987:AGCFSE]2.0.CO;2
Pulido C., Borum J. (2010). Eelgrass (Zostera Marina) Tolerance to Anoxia. J. Exp. Mar. Biol. Ecol. 385, 8–13. doi: 10.1016/j.jembe.2010.01.014
Qin L. Z., Kim S. H., Songa H. J., Kima H. G., Suonana Z., Kwona O., et al. (2020). Long Long-term Variability in the Flowering Phenology and Intensity of the Temperate Seagrass Zostera Marina in Response to Regional Sea Warming. Ecol. Indic. 119, 106821. doi: 10.1016/j.ecolind.2020.106821
Ruiz J. M., Marín-Guirao L., García-Muñoz R., Ramos-Segura A., Bernardeau-Esteller J., Pérez M., et al. (2018). Eperimental Evidence of Warming-Induced Flowering in the Mediterranean Seagrass Posidonia Oceanica. Mar. Pollut. Bull. 134, 49–54. doi: 10.1016/j.marpolbul.2017.10.037
Savva I., Bennett S., Roca G., Jordà G., Marbà N. (2018). Thermal Tolerance of Mediterranean Marine Macrophytes: Vulnerability to Global Warming. Ecol. Evol. 287, 20193001. doi: 10.1098/rspb.2019.3001
Smale D. A., Wernberg T., Oliver E. C. J., Thomsen M., Harvey B. P., Straub S. C., et al. (2019). Marine Heatwaves Threaten Global Biodiversity and the Provision of Ecosystem Services. Nat. Climate Change 9, 306–312. doi: 10.1038/s41558-019-0412-1
Spalding M. D., Ravilious C., Green E. P. (2001). World Atlas of Coral Reefs. Prepared at the UNEP World Conservation Monitoring Centre (Berkeley, USA: University of California Press), 424 pp.
Statton J., Sellers R., Dixon K. W., Kilminster K., Merritt D. J., Kendrick G. A. (2017). Seed Dormancy and Germination of Halophila Ovalis Mediated by Simulated Seasonal Temperature Changes. Estuar. Coast. Shelf. Sci. 198, 156–162. doi: 10.1016/j.ecss.2017.08.045
Stuart-Smith R. D., Edgar G. J., Bates A. E. (2017). Thermal Limits to the Geographic Distributions of Shallow-Water Marine Species. Nat. Ecol. Evol. 1, 1846–1852. doi: 10.1038/s41559-017-0353-x
Sunday J. M., Bates A. E., Dulvy N. K. (2011). Global Analysis of Thermal Tolerance and Latitude in Ectotherms. Proc. R. Soc. B.: Biol. Sci. 278, 1823–1830. doi: 10.1098/rspb.2010.1295
Taylor K. E., Stouffer R. J., Meehl G. A. (2012). An Overview of CMIP5 and the Experiment Design. Bull. Amer. Meteor. Soc 93, 485–498. doi: 10.1175/BAMS-D-11-00094.1
Thomas M. K., Kremer C. T., Klausmeier C. A., Litchman E. (2012). A Global Pattern of Thermal Adaptation in Marine Phytoplankton. Science 338, 1085–1088. doi: 10.1126/science.1224836
Thomson J. A., Burkholder D. A., Heithaus M. R., Fourqurean J. W., Fraser M. W., Statton J., et al. (2015). Extreme Temperatures, Foundation Species, and Abrupt Ecosystem Change: An Example From an Iconic Seagrass Ecosystem. Global Change Biol. 21, 1463–1474. doi: 10.1111/gcb.12694
Walker D. I., Cambridge M. L. (1995). An Experimental Assessment of the Temperature Responses of Two Sympatric Seagrasses, Amphibolis Antarctica and Ampibolis Griffithii, in Relation to Their Biogeography. Hydrobiologia 302, 63–70. doi: 10.1007/BF00006399
Waycott M., Duarte C. M., Carruthers T. J. B., Orth R. J., Dennison W. C., Olyarnik S., et al. (2009). Accelerating Loss of Seagrasses Across the Globe Threatens Coastal Ecosystems. Proc. Natl. Acad. Sci. U. S. A. 106, 12377–12381. doi: 10.1073/pnas.0905620106
Wesselmann M., Anton A., Duarte C. M., Hendriks I. E., Agustí S., Savva I., et al. (2020). Tropical Seagrass Halophila Stipulacea Shifts Thermal Tolerance During Mediterranean Invasion. Proc. R. Soc. B. 287, 20193001. doi: 10.1098/rspb.2019.3001
Wesselmann M., Geraldi N. R., Duarte C. M., Garcia-Orellana J., Díaz-Rúa R., Arias-Ortiz A., et al. (2021). Seagrass (Halophila Stipulacea) Invasion Enhances Carbon Sequestration in the Mediterranean Sea. Global Change Biol. 27, 2592–2607. doi: 10.1111/gcb.15589
York P. H., Gruber R. K., Hill R., Ralph P. J., Booth D. J., Macreadie P. I. (2013). Physiological and Morphological Responses of the Temperate Seagrass Zostera Muelleri to Multiple Stressors: Investigating the Interactive Effects of Light and Temperature. PloS One 8 (10), e76377. doi: 10.1371/journal.pone.0076377
Keywords: temperature, heat waves, climate change, seagrass, thresholds, mortality, growth
Citation: Marbà N, Jordà G, Bennett S and Duarte CM (2022) Seagrass Thermal Limits and Vulnerability to Future Warming. Front. Mar. Sci. 9:860826. doi: 10.3389/fmars.2022.860826
Received: 23 January 2022; Accepted: 27 April 2022;
Published: 27 May 2022.
Edited by:
Elvira S. Poloczanska, Alfred Wegener Institute Helmholtz Centre for Polar and Marine Research (AWI), GermanyReviewed by:
Gary Andrew Kendrick, University of Western Australia, AustraliaCopyright © 2022 Marbà, Jordà, Bennett and Duarte. This is an open-access article distributed under the terms of the Creative Commons Attribution License (CC BY). The use, distribution or reproduction in other forums is permitted, provided the original author(s) and the copyright owner(s) are credited and that the original publication in this journal is cited, in accordance with accepted academic practice. No use, distribution or reproduction is permitted which does not comply with these terms.
*Correspondence: Núria Marbà, bm1hcmJhQGltZWRlYS51aWItY3NpYy5lcw==
Disclaimer: All claims expressed in this article are solely those of the authors and do not necessarily represent those of their affiliated organizations, or those of the publisher, the editors and the reviewers. Any product that may be evaluated in this article or claim that may be made by its manufacturer is not guaranteed or endorsed by the publisher.
Research integrity at Frontiers
Learn more about the work of our research integrity team to safeguard the quality of each article we publish.