- 1Key Laboratory of Mariculture, Ocean University of China, Ministry of Education, Qingdao, China
- 2Function Laboratory for Marine Fisheries Science and Food Production Processes, Qingdao National Laboratory for Marine Science and Technology, Qingdao, China
The present study evaluated the effects of dietary supplementation with poly-β-hydroxybutyrate (PHB) on the growth performance, non-specific immunity, immune-related gene expression in the Nuclear factor-κB (NF-κB) signaling pathway, and intestinal microbiota of the sea cucumber Apostichopus japonicus. During a 63-day feeding trial, the sea cucumber was fed with basal diet (control, C treatment) and treatment diets supplemented at different doses of PHB, i.e., 0.5% (P treatment), 1% (OP treatment), and 3% PHB (TP treatment) (w/w) followed by a stimulation test of inactivated Vibrio splendidus for 7 days. All PHB treatments significantly promoted the specific growth rate and weight gain rate of the sea cucumber (P < 0.05). The 1% PHB significantly enhanced phagocytic, respiratory burst, superoxide dismutase, alkaline phosphatase, acid phosphatase, catalase, and lysozyme activities in the coelomocytes of A. japonicus (P < 0.05). However, no elevated activities of catalase and lysozyme were observed in the P treatment (P < 0.05), and only increased catalase activity appeared in the TP treatment (P < 0.05). Quantitative real-time PCR revealed significantly higher expression levels of Aj-p105, Aj-p50, and Aj-rel in the mid-intestine tissue of the sea cucumber in the PHB treatments (P < 0.05), and the relative expression level Aj-lys gene was significantly higher in the OP treatment (P < 0.05) than that in the control. After injection of inactivated V. splendidus, the relative expression level of four immune-related genes in the OP treatment was significantly up-regulated at 24 h (P < 0.05). The richness of intestinal microbiota in PHB treatments significantly increased, while diversity in TP treatment significantly decreased (P < 0.05). The relative abundances of Rhodobacteraceae in the PHB treatments were significantly higher than that in the control (P < 0.05). Network analysis revealed that 0.5 and 1% PHB supplement enhanced the stability of the intestinal microbial ecosystem. Functional prediction revealed that the PHB diet significantly increased some potential functions of intestinal microbiota, involving amino acid, lipid, and nucleotide metabolisms. In summary, dietary supplementation with a suitable dose of PHB had shown multiple beneficial effects on A. japonicus. Considering collectively the above, the optimum dose of 1% PHB addition to the diet of A. japonicus is recommended.
Introduction
Antibiotic misuse for aquaculture disease control has caused severe environmental pollution (Sapkota et al., 2008). Uncontrolled antibiotic application heavily hindered the aquaculture industry’s sustainable development by drug residues, bacteria resistance, immune suppression, and intestinal microbiota imbalance (Sapkota et al., 2008; Das et al., 2009). Furthermore, antibiotic misuse increased the risk of antibiotic resistance transferred to aquatic animals and human pathogens, threatening human health (Cabello, 2006; Defoirdt et al., 2007). Therefore, the development of environmentally friendly biocontrol strategies to replace antibiotics is key to guaranteeing the healthy and sustainable development of the aquaculture industry.
Poly-β-hydroxybutyrate (PHB) is a biopolymer consisting of linear chains of (R)-3-hydroxybutyrate (3HB) units (Jendrossek and Pfeiffer, 2014). It is safe, non-toxic, biodegradable, and histocompatible, and it has been widely used in environmental protection materials, biomedical materials, and agricultural production (Liu et al., 2010; Zhang et al., 2018). PHB is insoluble in water, but it can be depolymerized into water-soluble short-chain fatty acid monomers in the intestine and absorbed by animals. PHB could also be a carbon source to provide energy for the growth of aquatic animals. Therefore, PHB is considered an environmentally friendly alternative biocontrol compound for some cultured aquatic animals. Previous studies have shown that PHB could enhance some animals’ growth, immunity, and survival rate (Hung et al., 2015). Furthermore, PHB maintains a slightly acidic environment in the intestine and promotes beneficial microorganisms’ proliferation (Halet et al., 2007; Nhan et al., 2010). PHB-supplemented diets enhance the growth performance and survival of juvenile European sea basses (Dicentrarchus labrax) (De Schryver et al., 2010), Siberian sturgeons (Acipenser baerii) (Najdegerami et al., 2012), Chinese mitten crabs (Eriocheir sinensis) (Sui et al., 2016), blue mussels (Mytilus edulis) (Hung et al., 2015), and white shrimps (Litopenaeus vannamei) (Gao et al., 2019). Moreover, Najdegerami et al. (2012), Sui et al. (2016) found that dietary PHB increased the intestinal microbial species richness and diversity of Siberian sturgeons and Chinese mitten crabs, respectively. Sui et al. (2012), Hung et al. (2015) found that dietary PHB increased disease resistance against pathogenic microorganisms in Chinese mitten crabs and blue mussels. At present, PHB is mostly used as a feed additive in fish and crustacean aquaculture; however, according to our knowledge, the effects of dietary PHB on sea cucumber Apostichopus japonicus is rarely reported.
The sea cucumber A. japonicus is an important economic resource and is an aquaculture species widely distributed in China, Japan, South Korea, and Russia (Li et al., 2020). As a deposit feeder, sea cucumbers ingest food more than their body weight such as inorganic mineral particles and indigestible humic substances daily (Lopez and Levinton, 1987). However, the composition of the digestive system is very simple in sea cucumber, and it relies mainly on the intestinal tract for food conversion and nutrient absorption. The ability of its gut to accomplish such heavy food digestion and nutrient absorption to provide itself with nutrients and energy may be closely related to the transformation and decomposition of the intestinal microbiota. Intestinal microorganisms are considered the second genome and play a vital role in the host’s digestion and absorption of nutrients, immune response, and organ development (Yamazaki et al., 2016; Liu et al., 2019). Yamazaki et al. (2016) reported an abundance of intestinal microbiome retaining PHB metabolism genes in larger A. japonicus individuals, which might be derived from Rhodobacterales (in more detail Rhodobacteraceae). Differences in intestinal microbiota between larger and smaller individuals might be related to the PHB synthetic metabolism (Yamazaki et al., 2016). Previous studies indicate that Rhodobacteraceae played active roles in maintaining the health and enhancing the growth of aquatic animals (Liu et al., 2019; Huang et al., 2020). In addition, Yang et al. (2015b) showed that the activation of the Nuclear factor-κB (NF-κB) signaling pathway enhances the immune response of sea cucumbers, and is related to a significant increase in the potential probiotic Rhodobacteraceae in A. japonicus intestines.
Hence, we considered that PHB-supplemented diets may improve the relative abundance of Rhodobacteraceae, which may be linked with the enhancement of the growth performance and immunity of sea cucumbers. This study was designed to investigate the effects of dietary supplementation with different doses of PHB on growth performance, non-specific immunity, immune-related genes (Aj-p105, Aj-p50, Aj-rel, and Aj-lys) expression profiles in the NF-κB signaling pathway, and intestinal microbiota for A. japonicus. The suitable additive dose of PHB in the diet of A. japonicus was explored to provide a practical reference for culture technology improvement, feed development, and disease control for A. japonicus.
Materials and Methods
Experimental Animals and Diets
The sea cucumbers were purchased from Yantai Anyuan Aquatic Products Co., Ltd. (Yantai, China). Before the feeding trial, the sea cucumbers were reared in tanks (volume: 1,000 l) and fed with a basal diet for 15 days to acclimate to the laboratory conditions (temperature, 18 ± 1°C; salinity, 28–30‰; pH, 7.8–8.2; dissolved oxygen, 8.0 ± 0.2 mg/l). After acclimation, the sea cucumbers were fasted for 24 h, then 200 similar-sized individuals (mean body weight approximately 6.48 g) were randomly distributed into 20 aquariums (55 cm × 30 cm × 35 cm). Each aquarium has 10 individuals, and each treatment includes five aquariums.
Poly-β-hydroxybutyrate (PHB) (particle size of 10–50 μm) was purchased from Ningbo Tianan Biological Material Co., Ltd. (Ningbo, China). The basal diet was prepared with 70% sea mud, 15% sargassum powder, and 15% commercial sea cucumber feed (dry weight). All ingredients were ground into powder through 320-mesh, weighed proportionately, and mixed thoroughly. The basal diet contains 20% crude protein and 2.5% crude lipid. Experimental diets were prepared by supplementing the basal diet at different doses of PHB on a weight basis (w/w), supplemented with 0, 0.5, 1, and 3% of PHB as the C treatment (control), P treatment, OP treatment, and TP treatment, respectively. Powdered experimental diets were mixed daily with sterile seawater (gathered into mass and not dispersed in water) and fed to the sea cucumbers within 1 h to guarantee the freshness of diets.
Feeding Trial and Management
The sea cucumbers were fed to satiation with experimental diets one time daily at 6:00 pm for 63 days. Uneaten feed and feces were removed by siphoning before the next feeding, and 1/3 of the water in each aquarium was exchanged with fresh seawater daily. The water conditions for the experiment were the same as for the maintenance stage. The sea cucumbers were reared under 14 h light and 10 h dark dial cycle photoperiod.
Measurement of Growth and Survival
At the end of the feeding trial, the final number and mean bodyweight of the sea cucumber in each aquarium were recorded. The specific growth rate (SGR) (%/d), weight growth rate (WGR) (%), and survival rate (SR) (%) were calculated by the following formulae:
where Wt and W0 are the final and initial weights, t is the feeding trial period in days (d), and Nt and N0 are the final and the initial number of sea cucumber in each aquarium, respectively.
Sample Collection
At the end of the feeding trial, the sea cucumbers were fasted for 24 h. Three sea cucumbers were obtained from each aquarium, and their surface skin was sterilized with 70% ethanol. Then coelomic fluid of each sea cucumber was collected from the abdominal with a 1 ml sterile syringe (25-gauge needle) and thoroughly mixed with an equal volume of anticoagulant (0.02 M EGTA, 0.48 M NaCl, 0.019 M KCl, and 0.068 M Tris-HCl; pH = 7.6). Coelomocytes in the coelomic fluid was counted and expressed as cells per ml. Fresh coelomic fluid was divided into two, one part was used for phagocytosis (PA) activity and respiratory burst (RB) activity tests, the other was centrifuged at 3,000 × g, 4°C for 10 min to collect the coelomocytes. The coelomocytes were resuspended in an equal volume of 0.85% sterile ice-cold saline and sonicated at 22 kHz, 0°C for 30 s. Then, the suspension was centrifuged at 4,000 × g, 4°C for 10 min to collect the cell lysate supernatant (CLS) for the superoxide dismutase (SOD), catalase (CAT), acid phosphatase (ACP), alkaline phosphatase (AKP), and lysozyme (LZM) activity tests.
The ventral surface of each sea cucumber was dissected with a sterile scalpel from the anus to expose the body cavity. The intestinal content and mid-intestine tissue were collected into sterile tubes, respectively. The samples were flash-frozen in liquid nitrogen and stored at –80°C. The intestinal contents were used for the analysis of the intestinal microbiota, and the mid-intestine tissues were used for the determination of the relative expression levels of immune-related genes (Aj-p105, Aj-p50, Aj-rel, and Aj-lys).
Inactivation Vibrio splendidus Stimulation Test
The purified V. splendidus was inoculated in a 2216E seawater liquid medium and cultured for 24 h at 28°C, 150 rpm in shaken bottles. The thallus of V. splendidus was harvested by centrifugation for 10 min at 5,000 × g, 4°C. Then, the thallus was washed three times with sterilized phosphate-buffered saline (PBS), and an equal volume of 0.85% sterile ice-cold saline was added to make a suspension. A formaldehyde solution was added to the suspension to a final concentration of 0.5% then incubated for 24 h at 28°C to inactivate V. splendidus. The 2216E solid plate medium was coated with the inactivated V. splendidus and cultured for 24 h at 28°C. The absence of bacterial colonies on the plates indicated that V. splendidus was completely inactivated. Then, the inactivated thallus was harvested and washed three times with sterile PBS to eliminate the residual formaldehyde. Finally, the inactivated thallus was made into 2.57 × 108 cfu/ml bacterial suspension with 0.85% sterile ice-cold saline.
At the end of the feeding trial, 30 sea cucumbers from each treatment were equally distributed into three aquariums. Then, 100 μl inactivated V. splendidus was injected into the coelomic cavity of each sea cucumber and reared for 168 h, consistent with the feeding trial. The mid-intestine tissue from three sea cucumbers was collected at 24, 72, and 168 h after injection, respectively. The mid-intestine tissues were immediately placed into liquid nitrogen and stored at –80°C for immune-related gene expression levels test.
Non-specific Immune Parameters Analysis
Phagocytosis Activity
The coelomocytes’ phagocytosis activity was evaluated by the neutral red method according to Yang et al. (2015a) with some modification. The 100 μl of fresh coelomic fluid from each replicate was added to a 96-well microplate and incubated for 30 min at 25°C. Then, the supernatant was removed, 100 μl 0.029% neutral red (G-CLONE, China) was added to each well, and incubated for 30 min at 25°C. Each well was washed three times with PBS carefully, then 100 μl cell lysis buffer (acetic acid: ethanol = 1: 1, v/v) was added and incubated for 20 min at room temperature. The optical density of each well was measured at 540 nm by a microplate reader (Thermo, BioTeK, Winooski, VT, United States). The phagocytosis activity of coelomocytes is expressed as the absorbance of 1 × 106 cells.
Respiratory Burst Activity
The respiratory burst activity of coelomocytes was evaluated by nitroblue tetrazolium (NBT) (Sigma Aldrich, St. Louis, MO, United States) staining following the method of Yang et al. (2015a) with some modifications. The 100 μl of 0.2% poly-L-lysine (PLL) (Sigma Aldrich, St. Louis, MO, United States) solution was pre-added to each well of the 96-well microplate, shaken for 30 s, and left for 2 h at room temperature. The 100 μl fresh coelomic fluid was added to each well, centrifuged for 10 min at 300 × g, 4°C. The supernatant was removed, and 100 μl phorbol 1, 2-myristate 1, 3-acetate (1 μg/ml; PMA, United States) was added to each well, and the microplate was incubated at 37°C for 30 min. Then, 100 μl 0.3% NBT was added to each well and incubated for 30 min at 37°C to stain the cells. Then, the 96-well microplate was centrifuged at 560 × g for 10 min at 4°C. The supernatant was discarded, and 200 μl of absolute methanol was added to terminate the reaction for 10 min at 4°C. Then, the 96-well microplate was centrifuged for 10 min at 700 × g, 4°C, and the supernatant was discarded. Each well was washed three times with 70% methanol and air-dried at room temperature. Then, 120 μl 2 M potassium hydroxide solution KOH and 140 μl dimethyl sulfoxide (DMSO, Sigma, United States) were added, and the color was determined at 630 nm using a microplate reader (Thermo, BioTeK, United States) with KOH/DMSO as a blank. The respiratory burst activity of coelomocytes is expressed as the absorbance of 1 × 106 cells.
Non-specific Immune Enzyme Activity
Non-specific immune enzymes kits (Nanjing Jiancheng Bioengineering Institute, China) were used to evaluate the activities of SOD, CAT, ACP, AKP, and LZM in CLS according to the manufacturer’s protocols. One unit of SOD activity was defined as the amount of enzyme needed to inhibit the xanthine reduction rate by 50% in a 1 ml reaction solution. One unit of CAT activity was defined as the amount of enzyme needed in 1 ml CLS to decompose 1 μmol H2O2 per second. One unit of ACP activity was defined as 1 mg of phenol released by 100 ml CLS reacted with the matrix in 30 min at 37°C. One unit of AKP activity was defined as 1 mg of phenol released by 100 ml CLS reacted with the matrix in 15 min at 37°C. One unit of LZM activity was defined as the amount of enzyme required to decrease absorbance at a rate of 0.001/min⋅ml.
Immune-Related Gene Expression in Mid-Intestine
Relative Expression Levels of Aj-p105, Aj-p50, Aj-rel, and Aj-lys Genes
Total RNA was extracted from the mid-intestine tissue of the sea cucumber in each aquarium with Trizol Reagent (Ambion, United States) following the manufacturer’s protocol. Total RNA concentration was determined by a microspectrophotometer Nano-300 (Allsheng, China), and the integrity was detected by 1.5% agarose gel electrophoresis. Then, the total RNA was reverse transcribed to complementary DNA (cDNA) by a PrimeScript™ RT reagent Kit (Takara, Japan). Real-time quantitative PCR(RT-qPCR) was performed in a quantitative thermal cycler (Applied Biosystems, United States). The PCR program was 95°C for 2 min, then 40 cycles of 95°C for 10 s, 60°C for 10 s, 72°C for 20 s, followed by 95°C for 15 s, 60°C for 1 min, 95°C for 1 s. Each sample was carried out with three duplicates. The quantitative primers of Aj-p105, Aj-p50, Aj-rel, and Aj-lys genes, and reference gene (β-actin) were shown in Table 1. At the end of each PCR reaction, a melting curve analysis was performed to verify the amplification of a single product. The relative expression levels of Aj-p105, Aj-p50, Aj-rel, and Aj-lys genes were calculated by the 2–ΔΔCT comparative CT method.
Relative Expression Levels of Aj-p105, Aj-p50, Aj-rel, and Aj-lys Genes After Inactivation Vibrio splendidus Stimulation Test
The relative expression levels of Aj-p105, Aj-p50, Aj-rel, and Aj-lys genes of the mid-intestine of the sea cucumbers were measured at 24, 72, and 168 h, respectively. The extraction and detection method of total RNA, RT-qPCR process, and the calculation method of genes expression level was the same as described in section “Relative Expression Levels of Aj-p105, Aj-p50, Aj-rel, and Aj-lys Genes.”
Analysis of Intestinal Microbiota
DNA Extraction and 16S rRNA Gene Sequencing
Total DNA was extracted from the intestinal contents of A. japonicas using the PowerFecal DNA Isolation Kit (Mobio, Carlsbad, United States) following the manufacturer’s protocol. The V3 - V4 region of the bacterial 16S rRNA gene was amplified by PCR (95°C for 3 min, followed by 25 cycles 95°C for 30 s, 55°C for 30 s, and 72°C for 45 s, with a final extension at 72°C for 10 min) using primer set 338F (ACTCCTACGGGAGGCAGCAG) and 806R (GGACTACHVGGGTWTCTAAT). PCR reactions were performed in triplicate with 20 μl mixture containing 4 μl of 5 × FastPfu Buffer, 2 μl of 2.5 mM dNTPs, 0.8 μl of each primer (5 μM), 0.4 μl of FastPfu polymerase, and 10 ng of template DNA. The quality of the PCR products was detected using 2% agarose gel electrophoresis. Then, the purified PCR products were sequenced on the Illumina MiSeq platform by Majorbio Bio-pharm Technology Co., Ltd (Shanghai, China). The raw data of the 16S rRNA sequence were submitted to the National Center for Biotechnology Information (NCBI) Sequence Read Archive (SRA) database under accession number PRJNA781084.
Sequencing Data Processing
Raw sequencing data were processed with the Quantitative Insights Into Microbial Ecology software (QIIME 2 version 2020.11; Bolyen et al., 2019). All chimeric and low-quality reads were removed, and the qualified sequences were clustered into operational taxonomic units (OTUs) with a 97% cut-off. The bacterial community’s alpha and beta diversities were analyzed by combining QIIME 2, SPSS 22.0 (IBM, Armonk, NY, United States), and R software packages (version 4.0.4; Free Software Foundation, Inc., Boston, United States). Alpha diversity analysis included Chao1, abundance-based coverage estimator (ACE), Shannon, Simpson, and Coverage index analysis. Beta diversity was analyzed by Non-metric Multidimensional Scaling (NMDS) based on unweighted UniFrac metrics. The different abundant taxa between the control and different PHB treatments were identified by linear discriminant analysis (LDA) effect size (LEfSe) in the Galaxy web1, based on the non-parametric factorial Kruskal–Wallis rank-sum test.
Molecular Ecological Network Construction
The molecular ecological networks of the intestinal microbiota were constructed based on OTUs relative abundance on treatment. Five biological replicates were used to create a network in each treatment. Networks construction and OTUs topological roles identification were performed based on the random matrix theory (RMT) approach using the online MENA pipeline2. In the network, one node represented one OTU. A fast-greedy modularity optimization procedure was used for module separation of the network. To compare different complex networks, 100 randomly rewired networks were constructed using the Maslov-Sneppen procedure for each network identified. The average clustering coefficient (avgCC), average path distance (GD), and modularity of all random networks were calculated to test the differences between the molecular ecological networks and random networks based on Z-test. The within-module connectivity (Zi) and among-module connectivity (Pi) describe how well a node is connected to other nodes in the same module and the degree of connectivity of that node with different modules, respectively. The topological roles of OTUs were divided into four categories: (i) Peripheral nodes (Zi ≤ 2.5, Pi ≤ 0.62), (ii) Connectors (Zi ≤ 2.5, Pi > 0.62), (iii) Module hubs (Zi > 2.5, Pi ≤ 0.62), and (iv) Network hubs (Zi > 2.5, Pi > 0.62) (Deng et al., 2012). Cytoscape 3.8.0 was utilized for network visualization (Cline et al., 2007).
Functional Prediction Analysis of Intestinal Microbiota
Functional predictions of the intestinal microbiota were based on individual OTU profiles classified against the Greengenes database (Greengenes 13.8). The Kyoto Encyclopedia of Genes and Genome (KEGG) pathway analysis of OTUs was inferred using Phylogenetic Investigation of Communities by Reconstruction of Unobserved States (PICRUSt 2.4.1; Langille et al., 2013). The statistical analysis of microbial functions across treatments was performed using the Statistical Analysis of Metagenomic Profiles software package (STAMP 2.1.3; Parks et al., 2014). The significantly different KEGG pathways between the control and other treatments were identified using a two-sided Welch’s t-test, where a P < 0.05 was considered significant.
Statistical Analysis
The results are presented as the mean ± SE. The data were analyzed with one-way ANOVA followed by Duncan’s multiple range test using SPSS 22.0. Significant differences were set at P < 0.05.
Results
Growth Performance and Survival
Dietary PHB significantly enhanced the final weight, specific growth rate (SGR), and weight gain (WG) of the sea cucumbers (P < 0.05). Meanwhile, the highest values for final weight, SGR, and WG were observed in the OP treatment. The final weight, SGR, and WG of sea cucumber in the P and OP treatments were significantly higher than TP treatment, and no significant difference between the P and OP treatments (P > 0.05) was observed. No difference in survival rate (SR) was observed among treatments (P > 0.05) (Table 2).
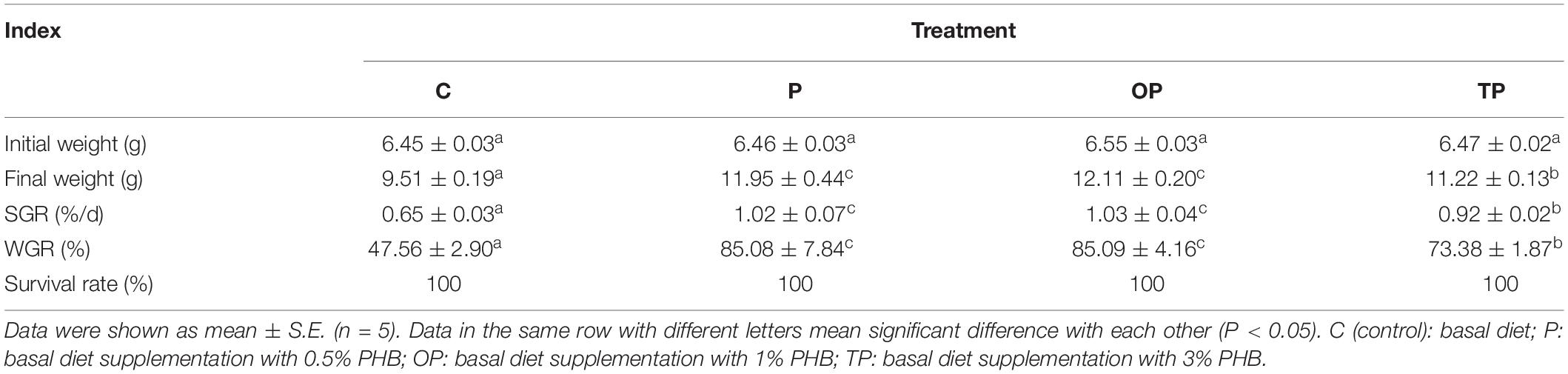
Table 2. Growth performance of sea cucumbers (Apostichopus japonicus) fed with diets with different levels of poly-β-hydroxybutyrate (PHB).
Non-specific Immune Parameters
Phagocytic Activity and Respiratory Burst Activity in Coelomocytes
Coelomocytes’ phagocytic activity and respiratory burst activity for sea cucumber in the P and OP treatments were significantly higher than the control and TP treatment (P < 0.05). However, no differences were observed between the control and TP treatment (P > 0.05), nor between the P and OP treatments (P > 0.05) (Figures 1A,B).
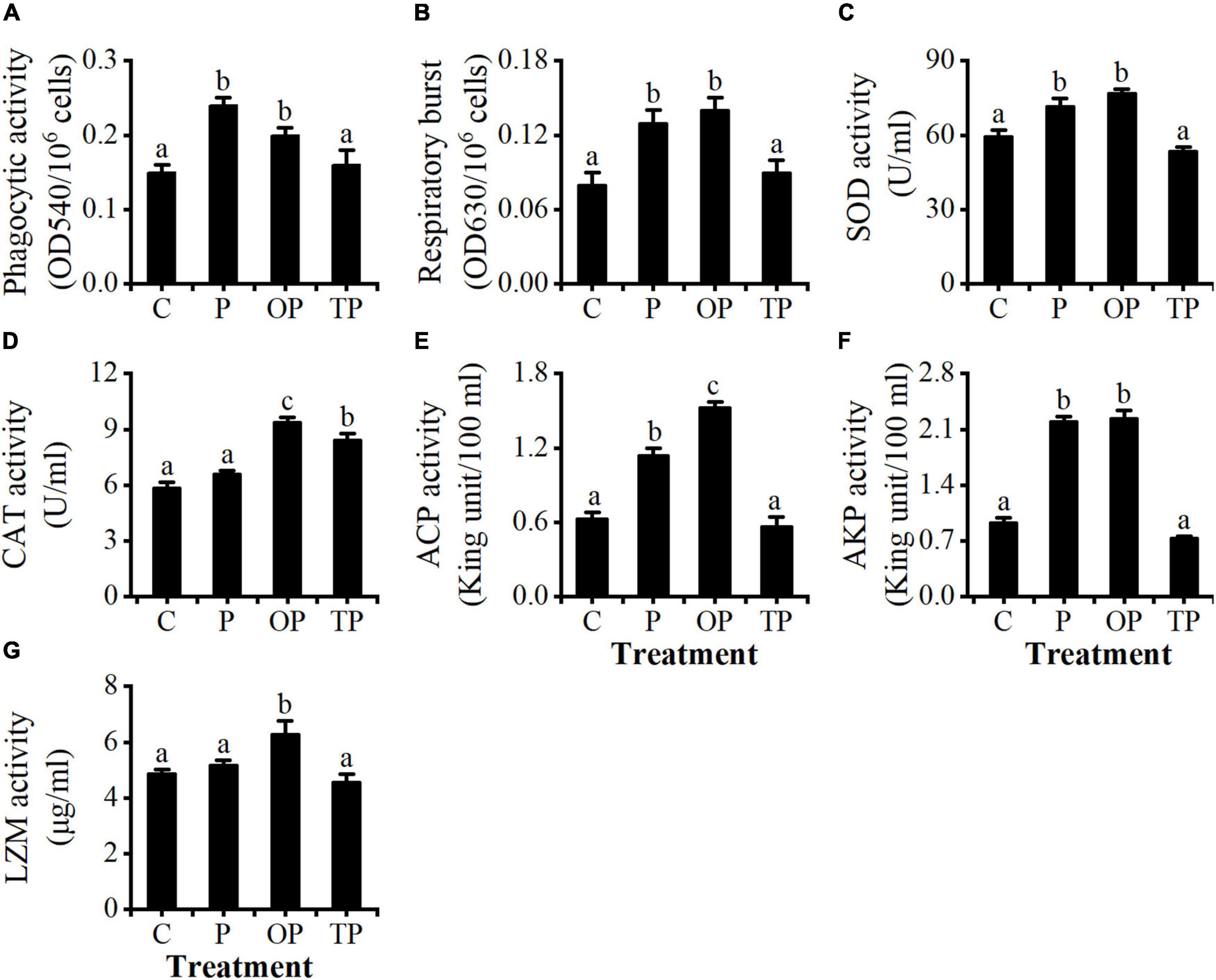
Figure 1. Effects of dietary poly-β-hydroxybutyrate (PHB) on (A) phagocytic (PA), (B) respiratory burst (RB), (C) superoxide dismutase (SOD), (D) catalase (CAT), (E) acid phosphatase (ACP), (F) alkaline phosphatase (AKP), and (G) lysozyme (LZM) activity in the coelomocytes of sea cucumber (Apostichopus japonicus). Bars represent mean ± SE (n = 5). Different letters in each figure represent significant differences (P < 0.05). C (control): basal diet; P: basal diet supplementation with 0.5% PHB; OP: basal diet supplementation with 1% PHB; TP: basal diet supplementation with 3% PHB.
Activities of Non-specific Immune Enzymes in Coelomocytes
The activities of SOD and AKP of coelomocytes in the P and OP treatments were significantly higher than the control and TP treatment (P < 0.05). However, no significant differences were observed between the P and OP treatments and between the control and TP treatments (P > 0.05) (Figures 1C,F). The CAT activity of the coelomocytes in the OP and TP treatments was significantly higher than the control and P treatments (P < 0.05). No difference was observed between the control and P treatments (P > 0.05), and the CAT activity in the OP treatment was significantly higher than that in the TP treatment (P < 0.05) (Figure 1D). The ACP activity of coelomocytes in the OP treatment was significantly higher than other treatments (P < 0.05), and the P treatment was significantly higher than the control and TP treatments (P < 0.05). There was no difference between the control and TP treatments (P > 0.05) (Figure 1E). The LZM activity in the OP treatment was significantly higher than that in the other treatments (P < 0.05), whereas no differences were observed among the control, P, and TP treatments (P > 0.05) (Figure 1G).
Relative Expression Levels of Aj-p105, Aj-p50, Aj-rel, and Aj-lys Genes in Mid-Intestine
As shown in Figure 2, the relative expression levels of the Aj-p105, Aj-p50, and Aj-rel genes in the PHB treatments were significantly higher than those in the control (P < 0.05). Meanwhile, the relative expression levels of the Aj-p105, Aj-p50, Aj-rel, and Aj-lys genes in the OP treatment were all significantly higher than other treatments (P < 0.05). Furthermore, the relative expression level of the Aj-p105 gene in the P treatment was significantly higher than in the TP treatment (P < 0.05). The relative expression level of the Aj-p50 gene in the TP treatment was significantly higher than that in the P treatment (P < 0.05). There was no difference in the relative expression levels of the Aj-rel gene between the P and TP treatments (P > 0.05). There was no difference in the relative expression levels of the Aj-lys gene among the control, P, and TP treatments (P > 0.05).
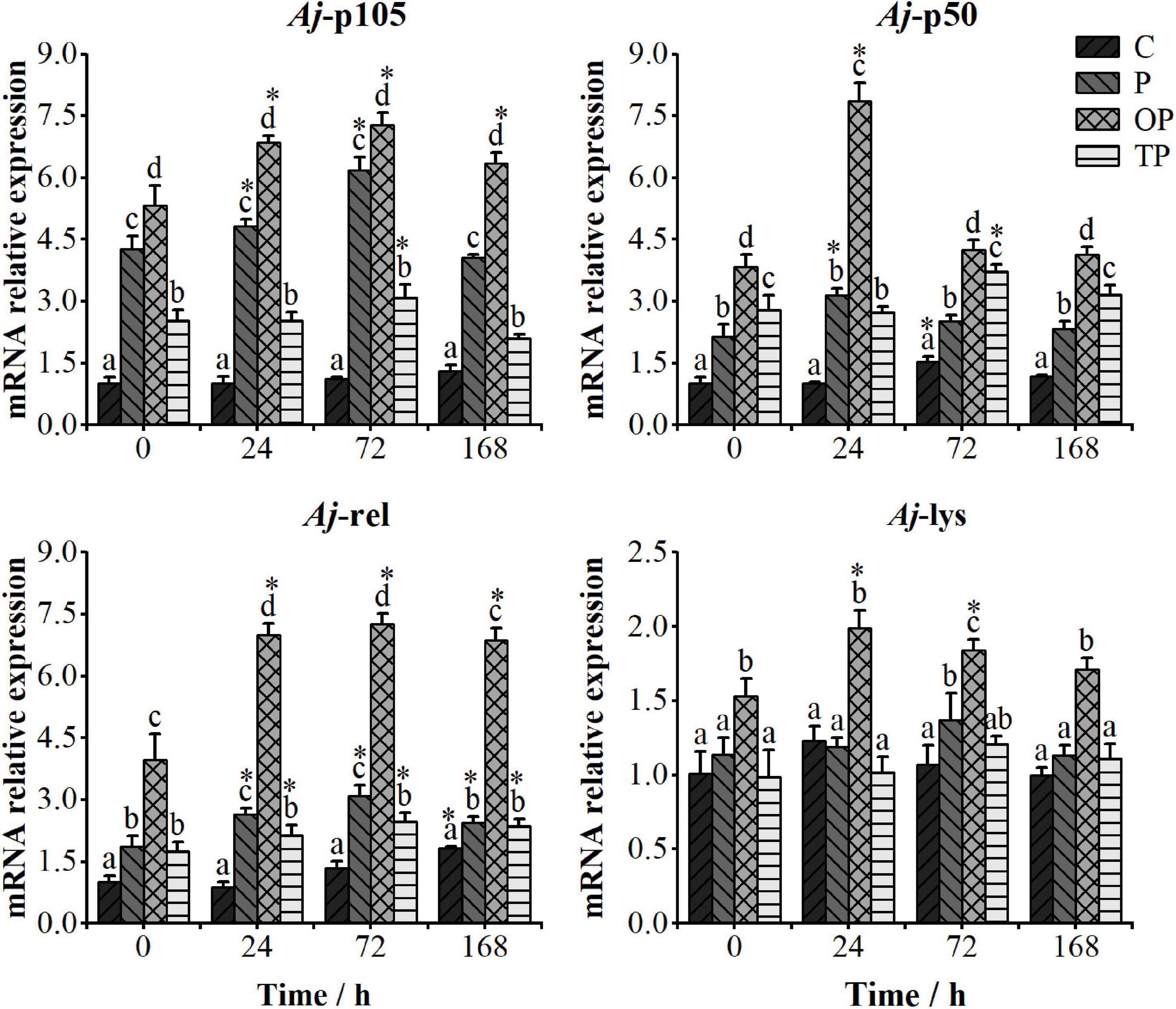
Figure 2. Relative expression levels of the Aj-p105, Aj-p50, Aj-rel, and Aj-lys genes in the mid-intestine tissue of the sea cucumbers at 0, 24, 72, and 168 h after stimulating by inactivated Vibrio splendidus. The time point of 0 h represents the relative expression level of immune-related genes at the end of the 63 days feeding trial. Bars represent mean ± SE (n = 5). Different letters at the same time point represent significant differences (P < 0.05). * stands for significantly different immune-related genes expression levels between the different time points and 0 h time point (P < 0.05). C (control): basal diet; P: basal diet supplementation with 0.5% PHB; OP: basal diet supplementation with 1% PHB; TP: basal diet supplementation with 3% PHB.
Relative Expression Levels of Aj-p105, Aj-p50, Aj-rel, and Aj-lys Genes in Mid-Intestine After Inactivated Vibrio splendidus Injection
The relative expression levels of the Aj-p105, Aj-p50, Aj-rel, and Aj-lys genes after the injection of inactivated V. splendidus are shown in Figure 2. The relative expression levels of the Aj-p105 gene were significantly up-regulated at 24 and 72 h in the P treatment, at 24, 72, and 168 h in the OP treatment, and at 72 h time point in the TP treatment (P < 0.05). The relative expression levels of the Aj-p50 gene were significantly up-regulated at 24 h in the P and OP treatments, and at 72 h time point in the TP treatment (P < 0.05). The relative expression levels of the Aj-rel gene in all PHB treatments were significantly up-regulated at 24, 72, and 168 h after injecting inactivated V. splendidus (P < 0.05). Meanwhile, the relative expression level of the Aj-rel gene in the OP treatment was significantly higher than other treatments at each time point (P < 0.05). The relative expression level of the Aj-lys gene was significantly up-regulated only in the OP treatment at 24 and 72 h after injecting inactivated V. splendidus (P < 0.05).
Analysis of Intestinal Microbiota
Bacterial Diversity of the Intestine
A total of 1,022,781 high-quality valid sequences was obtained after quality control and optimization of raw data from the 20 intestinal content samples with an average of 51,139 sequences per sample. The sequences ranged between 401 bp and 440 bp with an average length of 417 bp. From the 20 samples, a total of 2,741 OTUs obtained according to 97% sequence identity were assigned to 37 phyla, 98 classes, 245 orders, 421 families, 858 genera, and 1,474 species. The rarefaction curves tended to approach the saturation plateau in all samples, suggesting that the sequencing depth was enough to cover the whole bacterial diversity (Figure 3A).
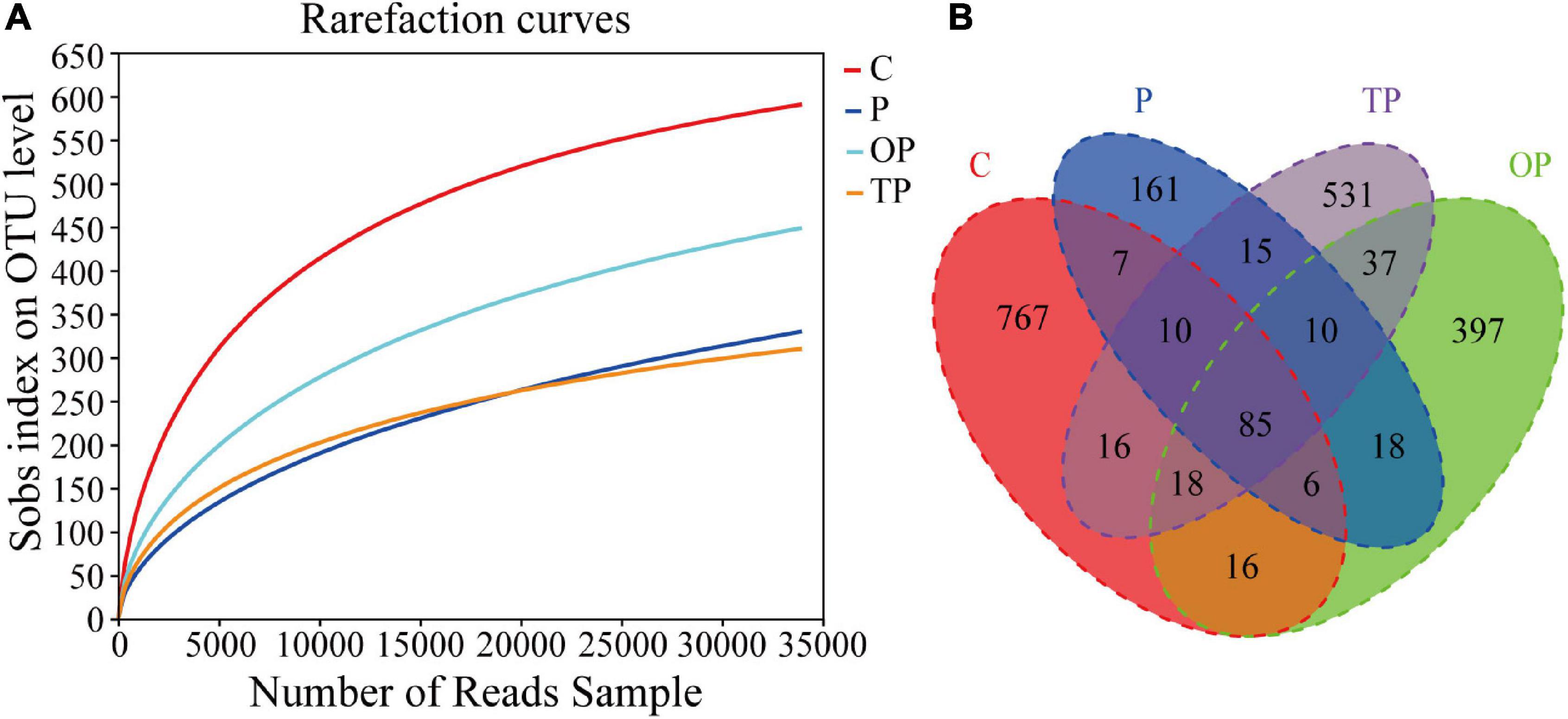
Figure 3. Rarefaction curves and Venn diagram analysis of intestinal microbiota for control and PHB treatments. (A) Rarefaction curves analyses of the different treatments and (B) Venn diagram. C (control): basal diet; P: basal diet supplementation with 0.5% PHB; OP: basal diet supplementation with 1% PHB; TP: basal diet supplementation with 3% PHB.
The Venn diagram showed that the four treatments shared 85 OTUs. There were 767, 161, 397, and 531 unique OTUs in the control, P, OP, and TP treatment, respectively (Figure 3B). The bacterial community alpha diversity indices are shown in Table 3. The alpha diversity analysis showed that the richness of intestinal microbiota in the P, OP, and TP treatments were significantly higher than that in the control (P < 0.05). The chao1 index in the OP treatment was significantly higher than that in the P and TP treatment (P < 0.05). The ACE index in the P and OP treatments was significantly higher than in the TP treatment (P < 0.05). Microbial diversity indices Shannon and Simpson in the OP treatment were significantly higher than those in the TP treatment (P < 0.05). As depicted in Figure 4, the NMDS ordination showed that dietary PHB altered the intestinal microbiota structure, especially the OP treatment had clear different characteristic bacterial community compared to the control.
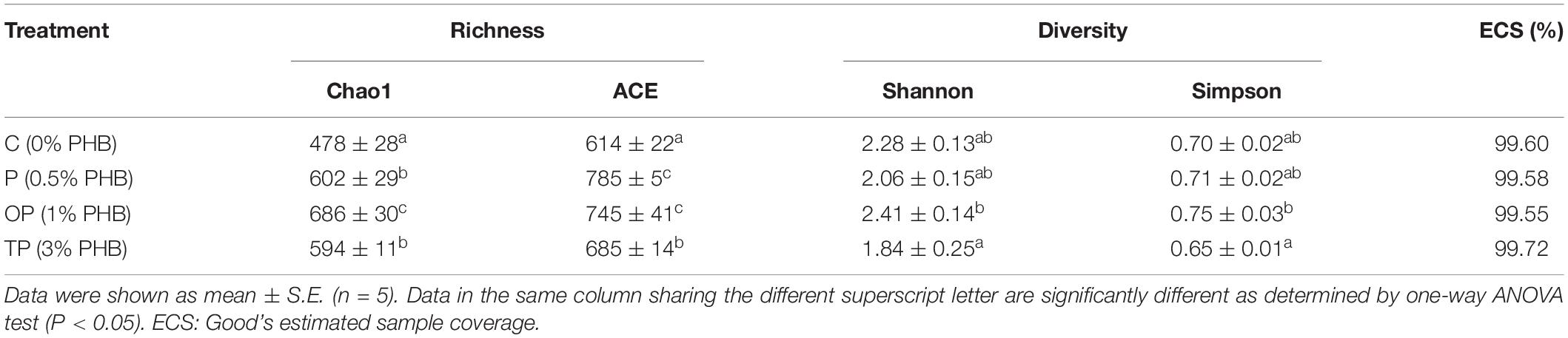
Table 3. Effects of diet supplementation with different levels of PHB on the alpha diversity index of intestinal microbiota for sea cucumbers.
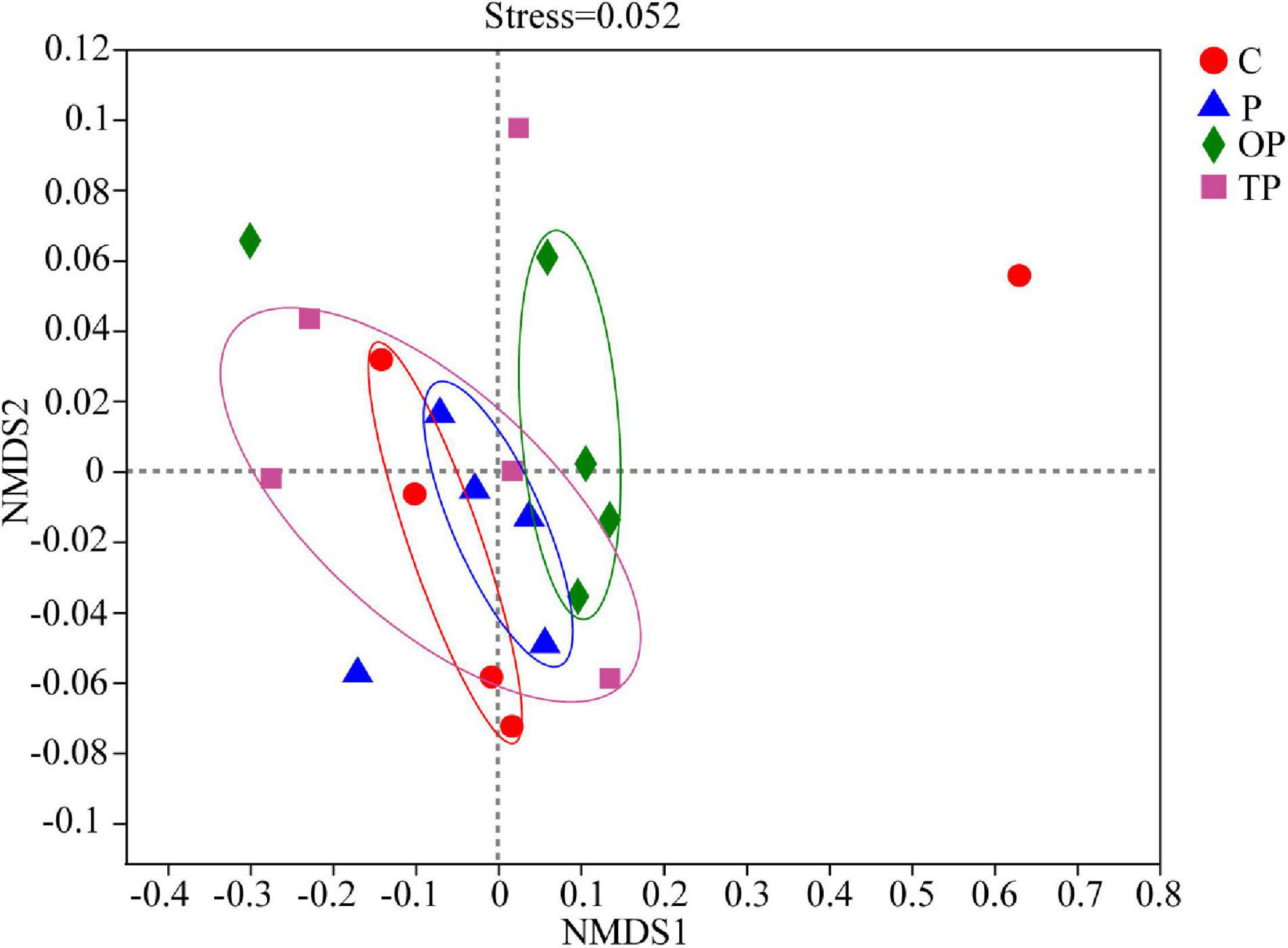
Figure 4. UniFrac distance-based Nonmetric Multidimensional Scaling (NMDS) analysis of intestinal microbial communities of sea cucumber fed diet supplementation with different levels of PHB. C (control): basal diet; P: basal diet supplementation with 0.5% PHB; OP: basal diet supplementation with 1% PHB; TP: basal diet supplementation with 3% PHB.
Intestinal Bacterial Community Composition
At the phylum level, Proteobacteria, Bacteroidota, Firmicutes, Verrucomicrobiota, and Actinobacteriota were the predominant bacterial phyla in the intestinal microbiota of sea cucumber (Figure 5A). The relative abundance of Verrucomicrobiota (9.25%) and Dependentiae (4.81%) in the OP treatment significantly increased (P < 0.05) compared to the control, however, the relative abundance of Firmicutes in all PHB treatments decreased.
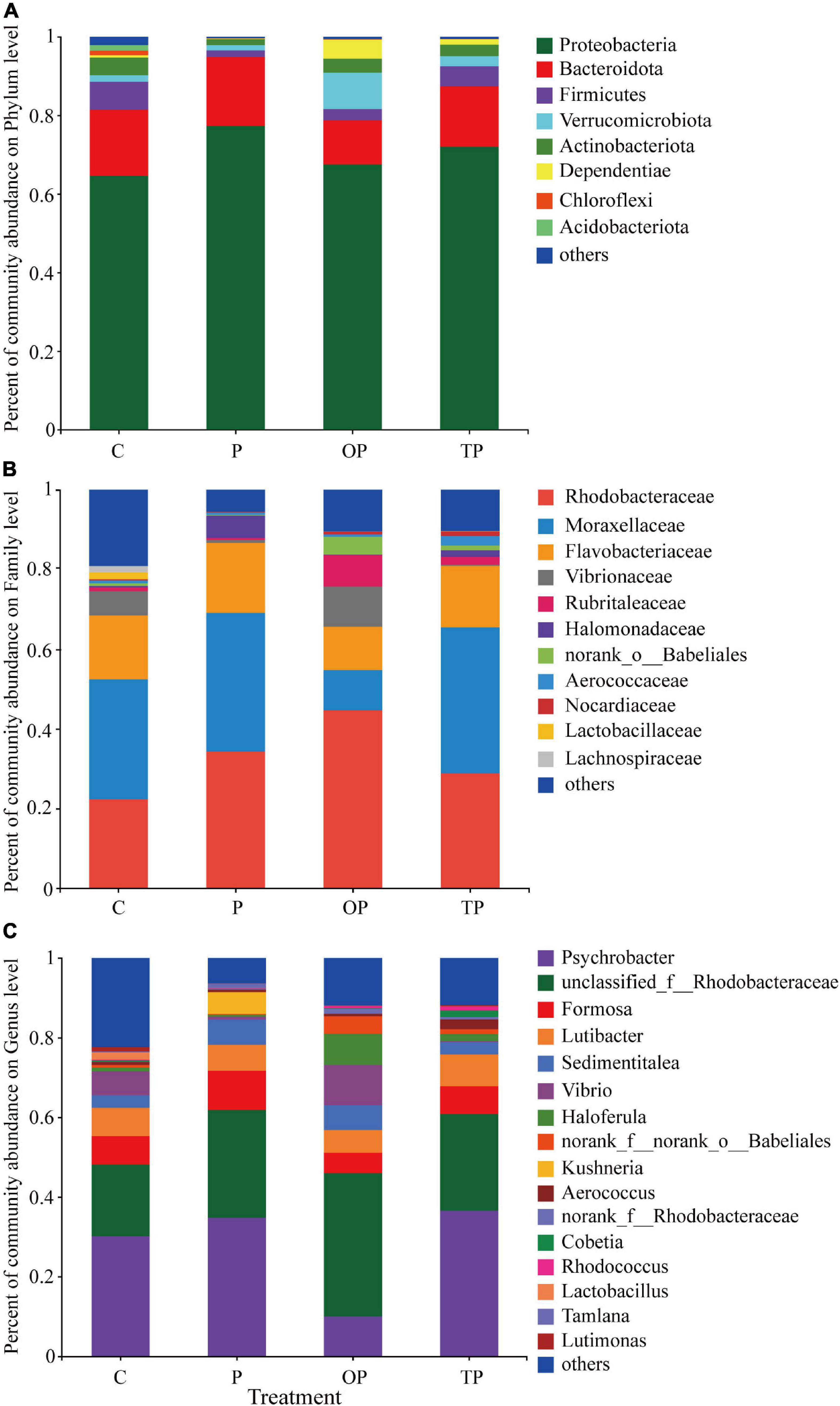
Figure 5. Effects of diet supplementation with different levels of PHB on microbial community composition. (A) At the phylum level, (B) at the family level, and (C) at the genus level. C (control): basal diet; P: basal diet supplementation with 0.5% PHB; OP: basal diet supplementation with 1% PHB; TP: basal diet supplementation with 3% PHB.
At the family level, the Rhodobacteraceae, Moraxellaceae, and Flavobacteriaceae were the predominant bacterial families in the intestinal microbiota of the sea cucumbers (Figure 5B). The relative abundance of Rhodobacteraceae was significantly increased in the P (34.56%), OP (43.29%), and TP treatments (28.79%) compared to the control (P < 0.05). The relative abundance of Halomonadaceae in the P (5.58%) and TP treatment (1.66%) significantly increased (P < 0.05). The relative abundance of Vibrarionaceae significantly decreased in the P (0.72%) and TP treatments (0.24%) (P < 0.05), while increased in the OP treatment (10.14%). In addition, the relative abundance of Rubritaleaceae and norank_o_Babeliales in the OP (8.37% and 4.64%, respectively) and TP treatment (2.03% and 1.31%, respectively) significantly increased (P < 0.05), whereas the Moraxellaceae in the OP treatment (10.04%) significantly decreased (P < 0.05).
At the genus level, Psychrobacter, unclassified_f_Rhodobacteraceae, Formosa, Lutibacter, and Sedimentitalea were the predominant bacterial genera in the intestinal microbiota of sea cucumber (Figure 5C). Compared to the control, the relative abundance of Psychrobacter in the OP treatment (9.98%) was significantly reduced (P < 0.05). The relative abundance of Sedimentitales significantly increased in the P (5.99%) and OP treatment (6.44%) (P < 0.05). The relative abundance of unclassified_f_Rhodobacteraceae in the P (27.68%), OP (34.41%), and TP treatment (24.17%) significantly increased (P < 0.05), and Haloferula and norank_f_norank_o_Babeliales was significantly increased in the OP treatment (8.29% and 4.64%, respectively) (P < 0.05). The relative abundance of Vibrio in the P (0.72%) and TP treatments (0.24%) significantly reduced (P < 0.05), while increased in the OP treatment (10.14%). The relative abundance of Kushneria was remarkably increased in the P treatment (5.53%) (P < 0.05).
The LEfSe analysis identified 12 differential OTUs responsible for the discrimination between the four treatments (Figure 6). When compared between treatments, one, eight, and two OTUs in the P, OP, and TP treatments were significantly increased (P < 0.05), respectively. In the P treatment, the OTU belongs to the Rhodobacteraceae family. In the OP treatment, the eight OTUs belong to the bacterial taxonomic families norank_o_Babeliales, Rhodobacteraceae, Rhizobiaceae, unclassified_c_Gammaproteobacteria, unclassified_o_Babeliales, unclassified_c_Alphaproteobacteria, Bacillaceae, and Legionellaceae. In the TP treatment, the two OTUs belong to the bacterial taxonomic families Clostridiaceae and Rubritaleaceae. One OTU belonging to the family Promicromonosporaceae was significantly decreased in all PHB treatments compared to the control (P < 0.05).
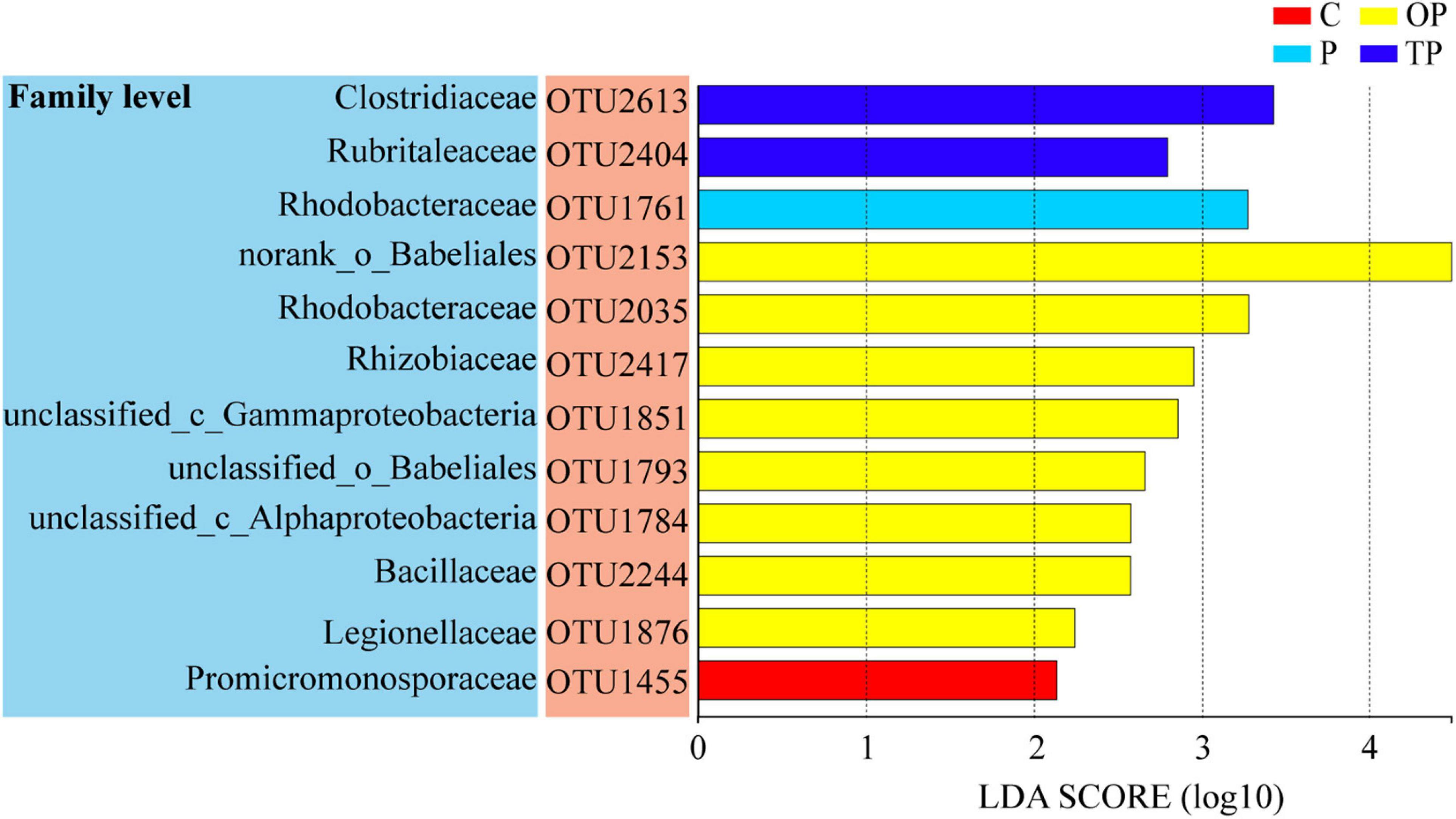
Figure 6. The linear discriminant analysis (LDA) effect size (LefSe) analysis identified the most differentially abundant operational taxonomic units (OTUs) for sea cucumber between the control and PHB treatments. C (control): basal diet; P: basal diet supplementation with 0.5% PHB; OP: basal diet supplementation with 1% PHB; TP: basal diet supplementation with 3% PHB.
Ecological Network Analysis
Four treatments of molecular ecological networks were constructed based on random matrix theory, red and blue links indicated positive and negative interactions, respectively (Figure 7). As shown in Figure 7 and Supplementary Table S1, in the control, the network was divided into four modules consisting of 109 nodes and 1,067 links (1,030 positive and 37 negative interactions). In the P treatment, the network was divided into six modules consisting of 157 nodes and 1,054 links (949 positive and 105 negative interactions). In the OP treatment, the network was divided into eight modules consisting of 204 nodes and 937 links (690 positive and 247 negative interactions). In the TP treatment, the network was divided into four modules consisting of 89 nodes and 1,014 links (987 positive and 27 negative interactions). Networks had more nodes and a higher proportion of negative and positive interactions in the OP treatment than the control, indicating a larger and more complex ecological network. As shown in Supplementary Table S2, the network in control, P, OP, and TP treatments consisted of different OTUs from 53, 73, 92, and 53 families, respectively. The dominant families were Bacillaceae, Flavobacteriaceae, and Rhodobacteraceae.
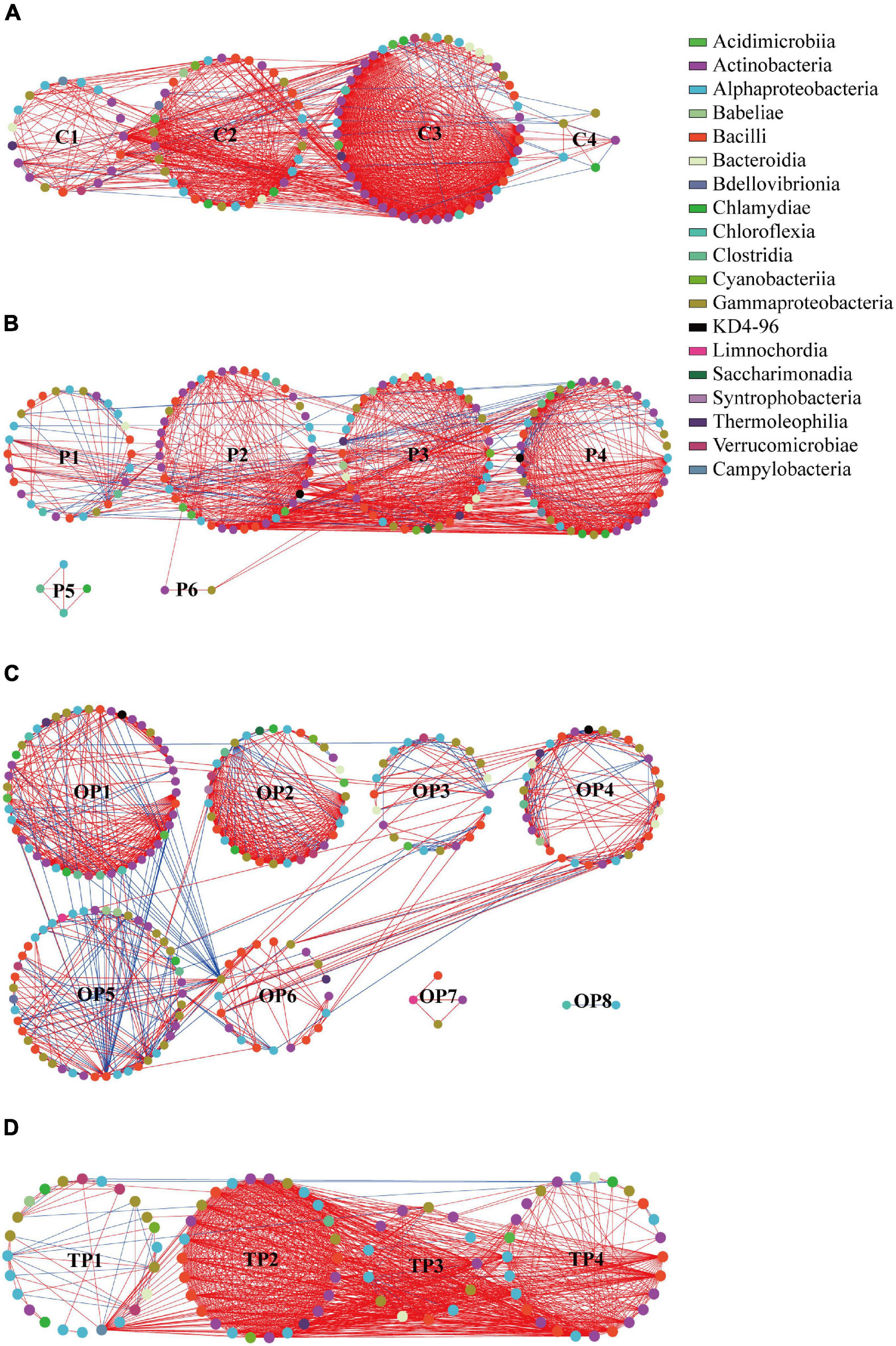
Figure 7. Network analysis of the intestinal microbiota in sea cucumbers. Each node indicates one OTU. Different colored nodes represent different classes. A red link indicates positive interaction between two individuals, while a blue link indicates negative interaction. (A) C treatment network, (B) P treatment network, (C) OP treatment network, and (D) TP treatment network. C (control): basal diet; P: basal diet supplementation with 0.5% PHB; OP: basal diet supplementation with 1% PHB; TP: basal diet supplementation with 3% PHB.
Ecological network indices are summarized in Supplementary Table S1. Average clustering coefficient (avgCC), average path distance (GD), and modularity of empirical networks were significantly different (P < 0.001) from their corresponding random networks in each treatment, indicating that each treatment network had a typical small-world property and modularity (Watts and Strogatz, 1998). In addition, the GD in the P (2.747), OP (5.381), and TP treatments (2.418), avgCC in the OP (0.620) and TP (0.707) treatments, and modularity in the P (0.482), OP (0.704), and TP (0.158) treatments were significantly different from the control (P < 0.001).
Different topological roles of OTUs in ecological networks are shown in Figure 8 and Supplementary Table S3. Most OTUs in the four networks were peripherals. The control, P, OP, and TP treatments showed four, ten, two, and five OTUs as connectors, respectively. In addition, one OTU served as module hubs in each of three PHB treatments, and one OTU served as net hubs in the P treatment.
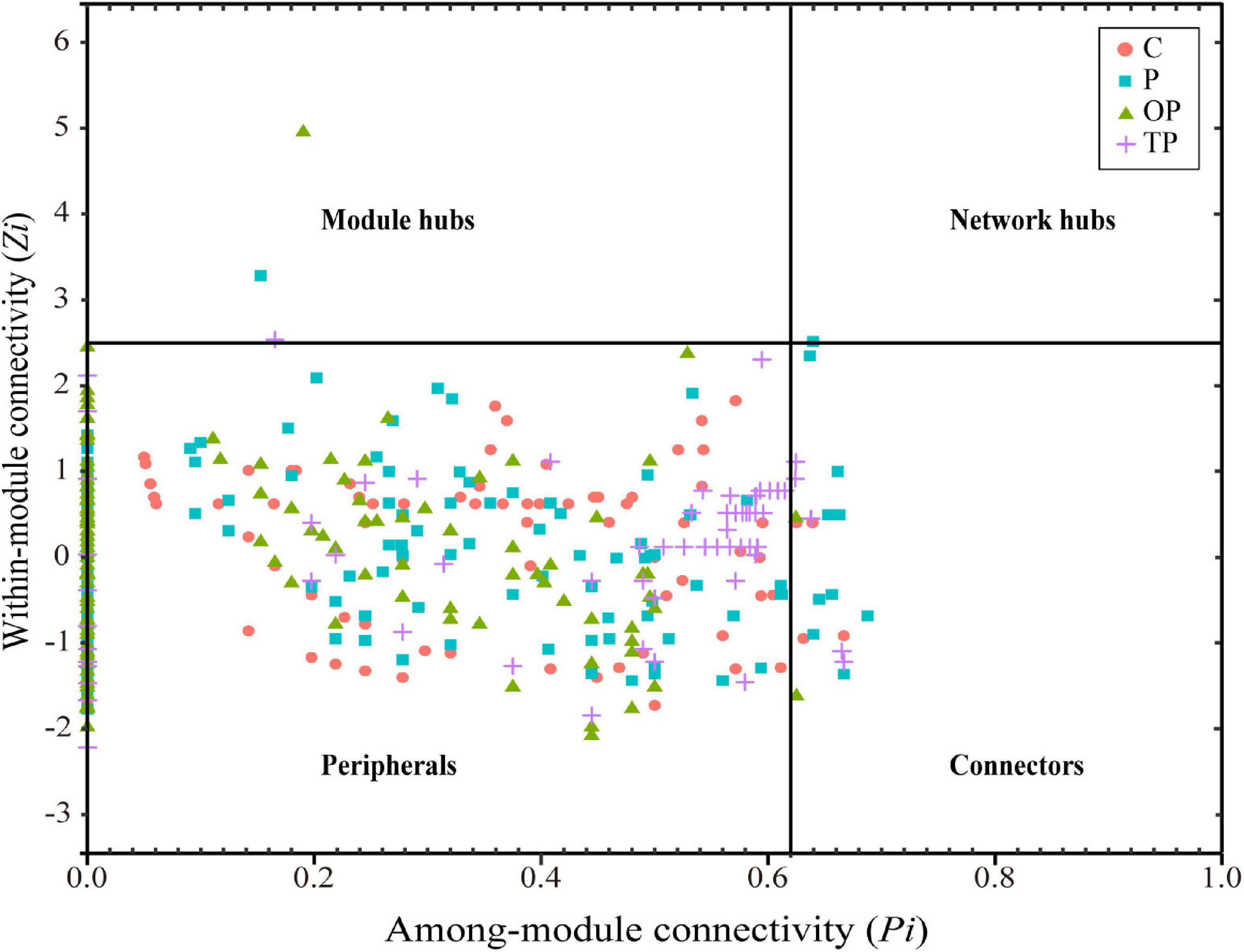
Figure 8. Distribution of sea cucumber intestinal bacterial taxa based on their topological roles. C (control): basal diet; P: basal diet supplementation with 0.5% PHB; OP: basal diet supplementation with 1% PHB; TP: basal diet supplementation with 3% PHB.
Predictive Functions of Intestinal Microbiota
As shown in Figure 9, compared to the control, ten functional pathways (amino acid metabolism, lipid metabolism, cell growth and death, metabolism of other amino acids, nucleotide metabolism, energy metabolism, carbohydrate metabolism, metabolism of terpenoids and polyketides, signaling molecules and interaction, glycan biosynthesis and metabolism) were enriched in the P treatment (P < 0.05), whereas, three functional pathways (endocrine system, sensory system, and global and overview maps) were significantly decreased (P < 0.05). In the OP treatment, eight functional pathways (signaling molecules and interaction, metabolism of other amino acids, amino acid metabolism, cell growth and death, global and overview maps, transport and catabolism, lipid metabolism, and nucleotide metabolism) were significantly higher than the control (P < 0.05). However, four functional pathways (signal transduction, “folding, sorting and degradation,” aging, and replication and repair) were significantly decreased (P < 0.05). In the TP treatment, eight functional pathways (metabolism of other amino acids, energy metabolism, cell growth and death, amino acid metabolism, global and overview maps, lipid metabolism, transport and catabolism, and nucleotide metabolism) were enriched (P < 0.05). On the contrary, five functional pathways (signal transduction, cell motility, circulatory system, “folding, sorting and degradation,” and drug resistance: antimicrobial) were significantly decreased (P < 0.05).
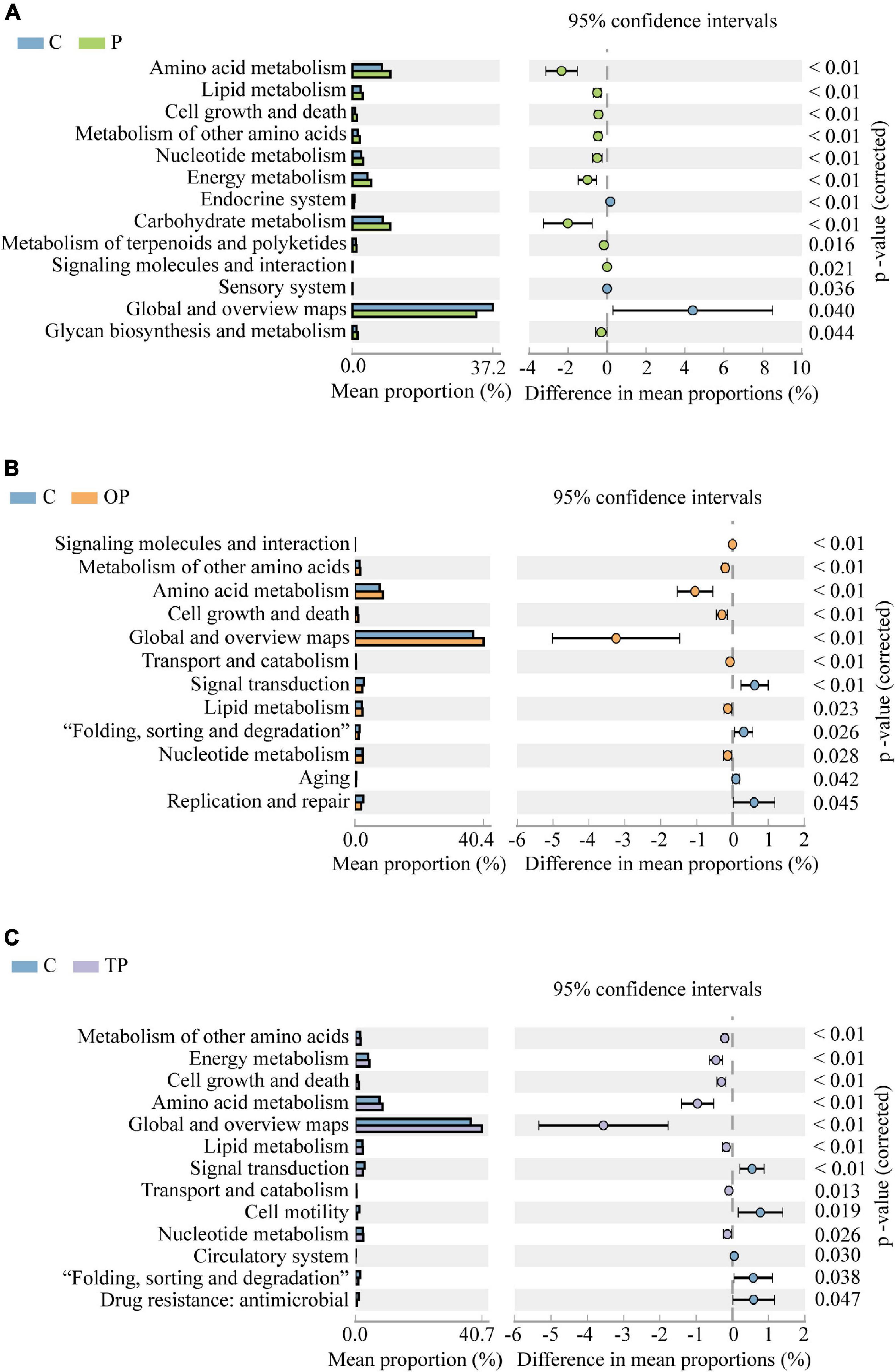
Figure 9. Differences of intestinal microbial functions predicted at level 2 of Kyoto Encyclopedia for Genes and Genomes (KEGG) pathways using PICRUSt. Only data with significant differences (P < 0.05) between treatments are shown. (A) C and P treatment, (B) C and OP treatment, (C) C and TP treatment. C (control): basal diet; P: basal diet supplementation with 0.5% PHB; OP: basal diet supplementation with 1% PHB; TP: basal diet supplementation with 3% PHB.
Discussion
Poly-β-hydroxybutyrate (PHB) is not only a carbon source that provides energy for the growth and development of some aquatic animals but degrades into water-soluble β-hydroxybutyric acid monomer in the intestine, which benefits the intestinal microbiota (De Schryver et al., 2010; Nhan et al., 2010; Sui et al., 2012, 2016). When supplemented with PHB, better growth performance has been observed in some aquatic animals, such as soiny mullets (Liza haematocheila) and Pacific white shrimps L. vannamei (Duan et al., 2017b; Qiao et al., 2019). In this study, significant enhancement in the growth performance of sea cucumbers supplemented with PHB was also demonstrated. However, the suitable dose of PHB for the growth promotion effect might be species-specific. The PHB optimal growth-promoting dose for Chinese mitten crabs (E. sinensis), European sea basses (D. labrax), and Siberian sturgeons (A. baerii) have been previously reported to be 1–3% (Sui et al., 2016), 2–5% (De Schryver et al., 2010), and 2% (Najdegerami et al., 2012), respectively. Comparatively, in this research, the specific growth rates of sea cucumbers fed with a diet supplemented with 0.5% and 1% PHB were significantly higher than those with 3% PHB, indicating that the promoting effect of PHB on growth performance is dose-dependent and species-specific for aquatic animals (De Schryver et al., 2010; Ng and Koh, 2017).
Coelomocytes can release bactericidal humoral factors and engulf invasive pathogenic microorganisms. Therefore, they are an important barrier in the non-specific immune system of sea cucumbers (Zhao et al., 2012). Generally, a series of parameters are used to assess the non-specific immune capacity of aquatic animals, including respiratory burst, phagocytosis, activities of SOD, CAT, ACP, AKP, and LZM (Adeshina et al., 2021; Guo et al., 2021; Jang et al., 2021). In this study, the responses of non-specific immune parameters to PHB supplementation were obviously dose-dependent. The dietary supplementation with 1% PHB significantly enhanced all parameters in the coelomocytes of sea cucumber. However, no elevated CAT and LZM activities were observed in the P treatment (0.5%), and only increased CAT activity appeared in the TP treatment (3%). By comparison, Duan et al. (2017b) found that dietary supplementation with 3% PHB significantly increased intestinal LZM activity in Pacific white shrimp L. vannamei. In another research, contrary to our findings, dietary PHB significantly decreased ACP and AKP activities in the hemolymph of Chinese mitten crabs (Sui et al., 2016). These results suggested that non-specific immune parameters’ responses to PHB supplementation were also dose-dependent and species-specific in aquatic animals.
The intestine is an important site for food digestion and nutrient absorption and a crucial barrier for the innate immune system to defend against pathogenic microorganisms (Li et al., 2020). NF-κB plays an important role in inflammation, immune response, development, and differentiation (Hoffmann and Baltimore, 2006). Wang et al. (2013) identified three important immune-related genes in the NF-κB signaling pathway of sea cucumber: Aj-p105, Aj-p50, and Aj-rel. Meanwhile, the NF-κB signaling cascade was active in sea cucumbers and played a crucial role in regulating the immune response (Wang et al., 2013). Lysozyme lyses pathogenic bacteria that invade the organisms. Therefore, the expression level of the Aj-pl05, Aj-p50, Aj-rel, and Aj-lys mRNAs in the intestine could reflect the immune status of A. japonicus (Yang et al., 2015a; Ahmadifar et al., 2019). In the present study, dietary PHB up-regulated these four genes expression levels to various extents. These indicated that dietary PHB stimulated intestinal immune-related genes expression and enhanced the transduction of the NF-κB signaling pathway. Among three treatments, those in the OP treatment were significantly higher than other treatments.
To assess the effects of PHB on the intestinal immune response of A. japonicus, the intestinal immune response of A. japonicus was stimulated via inactivated V. splendens, and the relative expression levels of the Aj-pl05, Aj-p50, Aj-rel, and Aj-lys genes were compared before and after stimulation in this study. Four immune-related gene expression levels in the OP treatment were significantly up-regulated rapidly at 24 h after being injected with inactivated V. splendens, and still higher than the control after 72 and 168 h. This indicated that the proper concentration of PHB enhanced the intestinal immune function of A. japonicus and accelerated its immune stress response to increase resistance to pathogenic bacteria.
The PHB supplement altered the richness, diversity, and composition of the intestinal microbiota of the sea cucumbers in the present study. The microbial richness of the three PHB supplement treatments was significantly higher than the control. Moreover, the effect of PHB on microbial richness was dose-dependent. The microbial richness in feeds supplemented with 0.5 and 1% PHB was significantly higher than that with 3%. On the other hand, the Shannon and Simpson indices in the OP treatment were the highest. A significant difference in intestinal microbial structure appeared between the OP treatment and the control by NMDS. LEfSe analysis identified 12 differential OTUs responsible for the discrimination between the four treatments. Eight of 12 OTUs were observed in the OP treatment, and their abundance increased significantly than other treatments. These results showed that the intestinal community in the OP treatment had different characteristics than the control, indicating the obviously regulatory effects of 1% PHB on the intestinal microbial structure of sea cucumber.
The intestinal microbiota of the sea cucumbers shifted after the PHB supplement. It was found that the relative abundance of Rhodobacteracea in the PHB supplement treatments significantly increased; this result well supported our presumption. Rhodobacteraceae is an important player in the marine ecosystem (Daniel et al., 2018). Previous studies indicated that Rhodobacteraceae played active roles in maintaining the health maintenance and growth enhancement for some aquatic animals, including Pacific white shrimp L. vannamei (Zheng et al., 2017; Liu et al., 2019; Wang et al., 2020), crab Portunus trituberculatus (Lu et al., 2022), Atlantic salmon (Salmo salar L.) (Hartviksen et al., 2014), and dusky kob Argyrosomus japonicus (Maapea et al., 2021). In this study, the highest relative abundance of Rhodobacteraceae was observed in the OP treatment, followed by the P and TP treatment was lowest among the three PHB treatments. LEfSe analysis identified two biomarkers belonging to Rhodobacteraceae with significantly increased abundance in the P (OTU1455) and OP treatment (OTU2035). This result was well consistent with the trend of growth enhancement in sea cucumbers supplemented PHB, indicating that the enhancement of growth performance in sea cucumbers might be closely linked to the increase of the relative abundance of Rhodobacteraceae. Intriguingly, it was observed that the relative abundance of Vibrio in the OP treatment was increased, while significantly decreased in the P and TP treatment. Vibrio is a common bacterium in the intestine of marine invertebrates, i.e., it was the predominant species in the intestine of A. japonicus (Wang et al., 2018; Zhao et al., 2018). However, it is usually considered an opportunistic pathogen in aquaculture (Thompson et al., 2004). In the present study, the increase in the relative abundance of Vibrio in the OP treatment did not negatively impact the sea cucumbers. On the contrary, the growth performance and non-specific immunity of the sea cucumbers in the OP treatment were better than the other treatments. Although some Vibrio species have been considered associated with disease, it has been reported that some Vibrio species are beneficial (Ninawe and Selvin, 2009). The beneficial Vibrio could inhibit pathogenic bacteria growth or directly kill pathogenic microorganisms, and they may be a major contributor to nutrient digestibility (Rajeev et al., 2021). Several Vibrio species have been successfully applied as biocontrol agents in aquaculture (Thompson et al., 2004; Gao et al., 2014). It could be presumed that 1% PHB addition might appropriately decrease the intestinal pH, create a favorable environment for benefiting the growth of beneficial Vibrio and inhibit the growth of pathogenic Vibrio spp. (Duan et al., 2017a). In addition, the relative abundance of Verrucomicrobiota and Rubritaleaceae in the OP treatment significantly increased while that of Moraxellaceae significantly decreased. The two formers could play an important role in polysaccharides degradation, and the latter was considered as a class of conditionally pathogenic bacteria or pathobionts (Embers et al., 2011; Cardman et al., 2014; Teixeira and Merquior, 2014; Ruzauskas et al., 2021). The above results suggested that the PHB supplement altered the intestinal microbiota, which might benefit the growth of beneficial bacteria and inhibit the pathogenic ones.
The positive (such as mutualism or synergy) and negative interactions (such as competition or predation) of interspecies within the intestinal microbial community construct a complex ecological network and maintain the stability of the intestinal microbial ecosystem (Deng et al., 2012; Coyte et al., 2015; Xiong et al., 2017; Venturelli et al., 2018). Generally, the cooperating networks of microbes can be efficient, but they are usually unstable, and hosts can benefit from the microbial competition when microbial competition dampens cooperative networks and increases stability (Coyte et al., 2015). In the present study, the larger and more complex ecological network was observed in the OP treatment with the biggest negative interactions/positive interactions ratio and the largest average path distance. These indicated that 1% PHB addition might enhance the stability of the intestinal microbial ecosystem. Meanwhile, topological role analysis showed more connectors and module hubs in the P treatment than other treatments. From the ecological viewpoint, connectors and module hubs may be linked to generalists, while the generalist decrease can cause the network to deteriorate (Olesen et al., 2007; Kaiser-Bunbury et al., 2010; Ramos-Jiliberto et al., 2012). Thus 0.5% PHB supplement might also be beneficial for enhancing the stability of the intestinal microbial ecosystem. A modularity value can be used to measure how well a network can be separated into modules. Each network module is considered a functional unit and performs an identifiable task (Newman, 2006; Deng et al., 2012). In this study, the network in the P (modularity 0.482, 6 sub-modules) and OP (modularity 0.704, 8 sub-modules) treatments had higher modularity and more sub-modules than the TP treatment (modularity 0.158, 4 sub-modules) and the control (modularity 0.286, 4 sub-modules). These results suggested that 0.5 and 1% PHB supplements could better regulate the intestinal microbiota to perform more biological functions, and the dose of 1% PHB was better than the 0.5% supplement.
Intestinal microbiota plays an important role in metabolism and nutrient absorption (Ramakrishna, 2013). Through amino acid-related metabolic pathways, the intestinal microbiota can produce some amino acids, absorbed as nutrients by the host’s intestine (Bairagi et al., 2002; Pandiyan et al., 2013). Moreover, intestinal microbiota may play an immunomodulatory role by regulating lipid metabolism (Liang et al., 2021). Therefore, the metabolic activities of the intestinal microbiota are closely involved in the hosts’ health (Shortt et al., 2018; Rastelli et al., 2019). In the present study, predicted functions of intestinal microbiota showed that ten, eight, and eight functional pathways in the P, OP, and TP treatments significantly enhanced, respectively. The amino acid metabolism, lipid metabolism, and nucleotide metabolism in all PHB treatments significantly increased. In the present study, the enhancement of multiple metabolic functions of the intestinal microbiota may directly improve the growth performance and non-specific immune enzyme activities in A. japonicus. The overall improvement in richness and diversity in the intestinal microbiota could allow more bacteria to thrive in the microbial community (Sui et al., 2016). Specifically, regarding changes in the gut microbiota, the relative abundance of Rhodobacteriaceae in all PHB treatments was significantly increased. Rhodobacteraceae bacteria possess complex metabolic pathways and perform various ecological functions in marine ecosystems (Luo and Moran, 2014; Simon et al., 2017). In addition, previous research reported that some secondary metabolites from Rhodobacteraceae had potential inhibitory effects on fish pathogens (Sonnenschein et al., 2017). Thus, increasing the relative abundance of Rhodobacteraceae might partially contribute to enhancing metabolism pathways in the PHB treatments.
However, it is worth noting that three functional pathways in the P treatment (endocrine system, sensory system, global and overview maps), four functional pathways in the OP treatment (signal transduction, “folding, sorting and degradation,” replication and repair, aging), and five functional pathways in the TP treatment (signal transduction, cell motility, circulatory system, “folding, sorting and degradation,” drug resistance: antimicrobial) significantly decreased. Overall, the number of enhanced functional pathways decreased, and the number of diminished functional pathways increased with raising the dose of PHB. This suggested that the PHB effects on the intestinal microbiota functional pathways were also dose-dependent, higher dose diminishing the effects of enhancement for functional pathways instead.
Conclusion
This study demonstrated that dietary supplementation with PHB significantly promoted growth performance, increased non-specific immune enzymes activities, stimulated intestinal immune-related gene expression, and enhanced the transduction of the NF-κB signaling pathway in A. japonicus. Dietary supplementation with 1% PHB showed better beneficial effects on the activities of non-specific immune enzymes and immune-related genes expression in A. japonicus. PHB supplement increased the richness of intestinal microbiota. A significant regulatory effect on the intestinal microbial structure of A. japonicus appeared at 1% PHB, and the stability of intestinal ecological networks was significantly enriched at 0.5 and 1% PHB. Dietary PHB significantly enhanced the pathways of metabolism functions of intestinal microbiota. The increasing relative abundance of Rhodobacteraceae might partially enhance growth performance and immune responses, and the metabolism pathways of A. japonicus intestinal microbiota. When the growth performance, immunity capacity, structure, and predicted functions of intestinal microbiota were considered together, 1% (w/w) PHB is the optimum dose as a feed additive for sea cucumber.
Data Availability Statement
The datasets presented in this study can be found in online repositories. The names of the repository/repositories and accession number(s) can be found below: NCBI (accession: PRJNA781084).
Ethics Statement
All animal experiments were conducted in accordance with the guidelines and approval of the respective Animal Research and Ethics Committees of Ocean University of China (Permit Number: 20141201. http://www.gov.cn/gongbao/content/2011/content_1860757.htm).
Author Contributions
LL performed experiments, analyzed the data, and wrote the manuscript. MW, CW, YL, MP, SW, and LC helped to LL for the animal collecting and maintaining. XT was the major instructor. All authors contributed to the article and approved the submitted version.
Funding
This work was financially supported by the National Natural Science Foundation of China (41976095) and the Natural Science Foundation of Shandong (ZR2017MC027).
Conflict of Interest
The authors declare that the research was conducted in the absence of any commercial or financial relationships that could be construed as a potential conflict of interest.
Publisher’s Note
All claims expressed in this article are solely those of the authors and do not necessarily represent those of their affiliated organizations, or those of the publisher, the editors and the reviewers. Any product that may be evaluated in this article, or claim that may be made by its manufacturer, is not guaranteed or endorsed by the publisher.
Acknowledgments
The authors thank the Laboratory of Aquaculture Ecology, Ocean University of China, for the support.
Supplementary Material
The Supplementary Material for this article can be found online at: https://www.frontiersin.org/articles/10.3389/fmars.2022.855938/full#supplementary-material
Footnotes
References
Adeshina, I., Abdel-Tawwab, M., Tijjani, Z. A., Tiamiyu, L. O., and Jahanbakhshi, A. (2021). Dietary Tridax procumbens leaves extract stimulated growth, antioxidants, immunity, and resistance of Nile tilapia, Oreochromis niloticus, to monogenean parasitic infection. Aquaculture 532:736047. doi: 10.1016/j.aquaculture.2020.736047
Ahmadifar, E., Dawood, M. A. O., Moghadam, M. S., Sheikhzadeh, N., Hoseinifar, S. H., and Musthafa, M. S. (2019). Modulation of immune parameters and antioxidant defense in zebrafish (Danio rerio) using dietary apple cider vinegar. Aquaculture 513:734412. doi: 10.1016/j.aquaculture.2019.734412
Bairagi, A., Ghosh, K. S., Kumar, S., Sen, S. K., and Ray, A. K. (2002). Enzyme producing bacterial flora isolated from fish digestive tracts. Aquacult. Int. 10, 109–121. doi: 10.1023/A:1021355406412
Bolyen, E., Rideout, J. R., Dillon, M. R., Bokulich, N. A., Abnet, C. C., Al-Ghalith, G. A., et al. (2019). Reproducible, interactive, scalable and extensible microbiome data science using QIIME 2. Nat. Biotechnol. 37, 852–857. doi: 10.1038/s41587-019-0209-9
Cabello, F. C. (2006). Heavy use of prophylactic antibiotics in aquaculture: a growing problem for human and animal health and for the environment. Environ. Microbiol. 8, 1137–1144. doi: 10.1111/j.1462-2920.2006.01054.x
Cardman, Z., Arnosti, C., Durbin, A., Ziervogel, K., Cox, C., Steen, A. D., et al. (2014). Verrucomicrobia are candidates for polysaccharide-degrading bacterioplankton in an arctic fjord of Svalbard. Appl. Environ. Microbiol. 80, 3749–3756. doi: 10.1128/AEM.00899-14
Cline, M. S., Smoot, M., Cerami, E., Kuchinsky, A., Landys, N., Workman, C., et al. (2007). Integration of biological networks and gene expression data using Cytoscape. Nat. Protoc. 2, 2366–2382. doi: 10.1038/nprot.2007.324
Coyte, K. Z., Schluter, J., and Foster, K. R. (2015). The ecology of the microbiome: networks, competition, and stability. Science 350, 663–666. doi: 10.1126/science.aad2602
Daniel, R., Simon, M., and Wemheuer, B. (2018). Editorial: molecular ecology and genetic diversity of the roseobacter clade. Front. Microbiol. 9:1185. doi: 10.3389/fmicb.2018.01185
Das, A., Saha, D., and Pal, J. (2009). Antimicrobial resistance and in vitro gene transfer in bacteria isolated from the ulcers of EUS-affected fish in India. Lett. Appl. Microbiol. 49, 497–502. doi: 10.1111/j.1472-765X.2009.02700.x
De Schryver, P., Sinha, A. K., Kunwar, P. S., Baruah, K., Verstraete, W., Boon, N., et al. (2010). Poly-β-hydroxybutyrate (PHB) increases growth performance and intestinal bacterial range-weighted richness in juvenile European sea bass, Dicentrarchus labrax. Appl. Microbiol. Biotechnol. 86, 1535–1541. doi: 10.1007/s00253-009-2414-9
Defoirdt, T., Boon, N., Sorgeloos, P., Verstraete, W., and Bossier, P. (2007). Alternatives to antibiotics to control bacterial infections: luminescent vibriosis in aquaculture as an example. Trends Biotechnol. 25, 472–479. doi: 10.1016/j.tibtech.2007.08.001
Deng, Y., Jiang, Y. H., Yang, Y., He, Z., Luo, F., and Zhou, J. (2012). Molecular ecological network analyses. BMC Bioinformatics 13:113. doi: 10.1186/1471-2105-13-113
Duan, Y., Zhang, Y., Dong, H., Wang, Y., and Zhang, J. (2017a). Effects of dietary poly-β-hydroxybutyrate (PHB) on microbiota composition and the mTOR signaling pathway in the intestines of litopenaeus vannamei. J. Microbiol. 55, 946–954. doi: 10.1007/s12275-017-7273-y
Duan, Y., Zhang, Y., Dong, H., Zheng, X., Wang, Y., Li, H., et al. (2017b). Effect of dietary poly-β-hydroxybutyrate (PHB) on growth performance, intestinal health status and body composition of Pacific white shrimp Litopenaeus vannamei (Boone, 1931). Fish Shellfish Immunol. 60, 520–528. doi: 10.1016/j.fsi.2016.11.020
Embers, M. E., Doyle, L. A., Whitehouse, C. A., Selby, E. B., Chappell, M., and Philipp, M. T. (2011). Characterization of a Moraxella species that causes epistaxis in macaques. Vet. Microbiol. 147, 367–375. doi: 10.1016/j.vetmic.2010.06.029
Gao, F., Tan, J., Sun, H., and Yan, J. (2014). Bacterial diversity of gut content in sea cucumber (Apostichopus japonicus) and its habitat surface sediment. J. Ocean Univ. China 13, 303–310. doi: 10.1007/s11802-014-2078-7
Gao, M., Du, D., Bo, Z., and Sui, L. (2019). Poly-β-hydroxybutyrate (PHB)-accumulating Halomonas improves the survival, growth, robustness and modifies the gut microbial composition of Litopenaeus vannamei postlarvae. Aquaculture 500, 607–612. doi: 10.1016/j.aquaculture.2018.10.032
Guo, K., Zhao, Z., Luo, L., Wang, S., Zhang, R., Xu, W., et al. (2021). Immune and intestinal microbiota responses to aerial exposure stress in Chinese mitten crab (Eriocheir sinensis). Aquaculture 541:736833. doi: 10.1016/j.aquaculture.2021.736833
Halet, D., Defoirdt, T., Van Damme, P., Vervaeren, H., Forrez, I., Van de Wiele, T., et al. (2007). Poly-β-hydroxybutyrate-accumulating bacteria protect gnotobiotic Artemia franciscana from pathogenic Vibrio campbellii. FEMS Microbiol. Ecol. 60, 363–369. doi: 10.1111/j.1574-6941.2007.00305.x
Hartviksen, M., Vecino, J. L. G., Ringo, E., Bakke, A. M., Wadsworth, S., Krogdahl, A., et al. (2014). Alternative dietary protein sources for Atlantic salmon (Salmo salar L.) effect on intestinal microbiota, intestinal and liver histology and growth. Aquac. Nutr. 20, 381–398. doi: 10.1111/anu.12087
Hoffmann, A., and Baltimore, D. (2006). Circuitry of nuclear factor κB signaling. Immunol. Rev. 210, 171–186. doi: 10.1111/j.0105-2896.2006.00375.x
Huang, L., Guo, H., Chen, C., Huang, X., Chen, W., Bao, F., et al. (2020). The bacteria from large-sized bioflocs are more associated with the shrimp gut microbiota in culture system. Aquaculture 523:735159. doi: 10.1016/j.aquaculture.2020.735159
Hung, N. V., De Schryver, P., Tam, T. T., Garcia-Gonzalez, L., Bossier, P., and Nevejan, N. (2015). Application of poly-β-hydroxybutyrate (PHB) in mussel larviculture. Aquaculture 446, 318–324. doi: 10.1016/j.aquaculture.2015.04.036
Jang, W. J., Hasan, M. T., Lee, G. H., Lee, B. J., Hur, S. W., Lee, S., et al. (2021). Comparison of spore or vegetative Bacillus sp. supplementation on physiological changes and gut microbiota of the olive flounder (Paralichthys olivaceus). Aquaculture 535:736355. doi: 10.1016/j.aquaculture.2021.736355
Jendrossek, D., and Pfeiffer, D. (2014). New insights in the formation of polyhydroxyalkanoate granules (carbonosomes) and novel functions of poly(3-hydroxybutyrate). Environ. Microbiol. 16, 2357–2373. doi: 10.1111/1462-2920.12356
Kaiser-Bunbury, C. N., Muff, S., Memmott, J., Muller, C. B., and Caflisch, A. (2010). The robustness of pollination networks to the loss of species and interactions: a quantitative approach incorporating pollinator behaviour. Ecol. Lett. 13, 442–452. doi: 10.1111/j.1461-0248.2009.01437.x
Langille, M. G., Zaneveld, J., Caporaso, J. G., McDonald, D., Knights, D., Reyes, J. A., et al. (2013). Predictive functional profiling of microbial communities using 16S rRNA marker gene sequences. Nat. Biotechnol. 31, 814–821. doi: 10.1038/nbt.2676
Li, Y., Yang, Y., Ji, Q., Song, J., Wang, L., Liu, B., et al. (2020). The function of Apostichopus japonicas catalase in sea cucumber intestinal immunity. Aquaculture 521:735103. doi: 10.1016/j.aquaculture.2020.735103
Liang, Q., Dong, J., Wang, S., Shao, W., Ahmed, A. F., Zhang, Y., et al. (2021). Immunomodulatory effects of Nigella sativa seed polysaccharides by gut microbial and proteomic technologies. Int. J. Biol. Macromol. 184, 483–496. doi: 10.1016/j.ijbiomac.2021.06.118
Liu, J., Wang, K., Wang, Y., Chen, W., Jin, Z., Yao, Z., et al. (2019). Strain-specific changes in the gut microbiota profiles of the white shrimp Litopenaeus vannamei in response to cold stress. Aquaculture 503, 357–366. doi: 10.1016/j.aquaculture.2019.01.026
Liu, Y., De Schryver, P., Van Delsen, B., Maignien, L., Boon, N., Sorgeloos, P., et al. (2010). PHB-degrading bacteria isolated from the gastrointestinal tract of aquatic animals as protective actors against luminescent vibriosis. FEMS Microbiol. Ecol. 74, 196–204. doi: 10.1111/j.1574-6941.2010.00926.x
Lopez, G. R., and Levinton, J. S. (1987). Ecology of deposit-feeding animals in marine sediments. Q. Rev. Biol. 62, 235–260. doi: 10.1086/415511
Lu, Z., Ren, Z. M., Lin, W., Shi, C., Mu, C., Wang, C., et al. (2022). Succession, sources, and assembly of bacterial community in the developing crab larval microbiome. Aquaculture 548:737600. doi: 10.1016/j.aquaculture.2021.737600
Luo, H., and Moran, M. A. (2014). Evolutionary ecology of the marine Roseobacter clade. Microbiol. Mol. Biol. Rev. 78, 573–587. doi: 10.1128/MMBR.00020-14
Maapea, A. D., Vine, N. G., and Macey, B. M. (2021). Bacterial microbiome of dusky kob Argyrosomus japonicus eggs and rearing water and the bacteriostatic effect of selected disinfectants. Aquaculture 542:736882. doi: 10.1016/j.aquaculture.2021.736882
Najdegerami, E. H., Tran, T. N., Defoirdt, T., Marzorati, M., Sorgeloos, P., Boon, N., et al. (2012). Effects of poly-β-hydroxybutyrate (PHB) on Siberian sturgeon (Acipenser baerii) fingerlings performance and its gastrointestinal tract microbial community. FEMS Microbiol. Ecol. 79, 25–33. doi: 10.1111/j.1574-6941.2011.01194.x
Newman, M. E. J. (2006). Modularity and community structure in networks. Proc. Natl. Acad. Sci. U.S.A. 103, 8577–8582. doi: 10.1073/pnas.0601602103
Ng, W. K., and Koh, C. B. (2017). The utilization and mode of action of organic acids in the feeds of cultured aquatic animals. Rev. Aquacult. 9, 342–368. doi: 10.1111/raq.12141
Nhan, D. T., Wille, M., De Schryver, P., Defoirdt, T., Bossier, P., and Sorgeloos, P. (2010). The effect of poly-β-hydroxybutyrate on larviculture of the giant freshwater prawn Macrobrachium rosenbergii. Aquaculture 302, 76–81. doi: 10.1016/j.aquaculture.2010.02.011
Ninawe, A. S., and Selvin, J. (2009). Probiotics in shrimp aquaculture: avenues and challenges. Crit. Rev. Microbiol. 35, 43–66. doi: 10.1080/10408410802667202
Olesen, J. M., Bascompte, J., Dupont, Y. L., and Jordano, P. (2007). The modularity of pollination networks. Proc. Natl. Acad. Sci. U.S.A. 104, 19891–19896. doi: 10.1073/pnas.0706375104
Pandiyan, P., Balaraman, D., Thirunavukkarasu, R., George, E. G. J., Subaramaniyan, K., Manikkam, S., et al. (2013). Probiotics in aquaculture. Drug Invent. Today 5, 55–59. doi: 10.1016/j.dit.2013.03.003
Parks, D. H., Tyson, G. W., Hugenholtz, P., and Beiko, R. G. (2014). STAMP: statistical analysis of taxonomic and functional profiles. Bioinformatics 30, 3123–3124. doi: 10.1093/bioinformatics/btu494
Qiao, G., Sun, Q., Zhang, M., Xu, C., Lv, T., Qi, Z., et al. (2019). Antioxidant system of soiny mullet (Liza haematocheila) is responsive to dietary poly-β-hydroxybutyrate (PHB) supplementation based on immune-related enzyme activity and de novo transcriptome analysis. Fish Shellfish Immunol. 95, 314–327. doi: 10.1016/j.fsi.2019.10.042
Rajeev, R., Adithya, K. K., Kiran, G. S., and Selvin, J. (2021). Healthy microbiome: a key to successful and sustainable shrimp aquaculture. Rev. Aquacult. 13, 238–258. doi: 10.1111/raq.12471
Ramakrishna, B. S. (2013). Role of the gut microbiota in human nutrition and metabolism. J. Gastroenterol. Hepatol. 28(Suppl. 4), 9–17. doi: 10.1111/jgh.12294
Ramos-Jiliberto, R., Valdovinos, F. S., Moisset de Espanes, P., and Flores, J. D. (2012). Topological plasticity increases robustness of mutualistic networks. J. Anim. Ecol. 81, 896–904. doi: 10.1111/j.1365-2656.2012.01960.x
Rastelli, M., Cani, P. D., and Knauf, C. (2019). The gut microbiome influences host endocrine functions. Endocr. Rev. 40, 1271–1284. doi: 10.1210/er.2018-00280
Ruzauskas, M., Armalyte, J., Lastauskiene, E., Siugzdiniene, R., Klimiene, I., Mockeliunas, R., et al. (2021). Microbial and antimicrobial resistance profiles of microbiota in common carps (Cyprinus carpio) from aquacultured and wild fish populations. Animals (Basel) 11:929. doi: 10.3390/ani11040929
Sapkota, A., Sapkota, A. R., Kucharski, M., Burke, J., McKenzie, S., Walker, P., et al. (2008). Aquaculture practices and potential human health risks: current knowledge and future priorities. Environ. Int. 34, 1215–1226. doi: 10.1016/j.envint.2008.04.009
Shortt, C., Hasselwander, O., Meynier, A., Nauta, A., Fernandez, E. N., Putz, P., et al. (2018). Systematic review of the effects of the intestinal microbiota on selected nutrients and non-nutrients. Eur. J. Nutr. 57, 25–49. doi: 10.1007/s00394-017-1546-4
Simon, M., Scheuner, C., Meier-Kolthoff, J. P., Brinkhoff, T., Wagner-Dobler, I., Ulbrich, M., et al. (2017). Phylogenomics of Rhodobacteraceae reveals evolutionary adaptation to marine and non-marine habitats. ISME J. 11, 1483–1499. doi: 10.1038/ismej.2016.198
Sonnenschein, E. C., Nielsen, K. F., D’Alvise, P., Porsby, C. H., Melchiorsen, J., Heilmann, J., et al. (2017). Global occurrence and heterogeneity of the Roseobacter-clade species Ruegeria mobilis. ISME J. 11, 569–583. doi: 10.1038/ismej.2016.111
Sui, L., Cai, J., Sun, H., Wille, M., and Bossier, P. (2012). Effect of poly-β-hydroxybutyrate on Chinese mitten crab, Eriocheir sinensis, larvae challenged with pathogenic Vibrio anguillarum. J. Fish Dis. 35, 359–364. doi: 10.1111/j.1365-2761.2012.01351.x
Sui, L., Ma, G., Lu, W., Deng, Y., Bossier, P., and De Schryver, P. (2016). Effect of poly-β-hydroxybutyrate on growth, enzyme activity and intestinal microbial community of Chinese mitten crab, Eriocheir sinensis (Milne-Edwards) juveniles. Aquac. Res. 47, 3644–3652. doi: 10.1111/are.12817
Teixeira, L. M., and Merquior, V. L. C. (2014). ‘‘The family Moraxellaceae,’’ in The Prokaryotes, eds E. Rosenberg, E. F. DeLong, S. Lory, E. Stackebrandt, and F. Thompson (Berlin: Springer), 443–476. doi: 10.1007/978-3-642-38922-1_245
Thompson, F. L., Iida, T., and Swings, J. (2004). Biodiversity of vibrios. Microbiol. Mol. Biol. Rev. 68, 403–431. doi: 10.1128/MMBR.68.3.403-431.2004
Venturelli, O. S., Carr, A. V., Fisher, G., Hsu, R. H., Lau, R., Bowen, B. P., et al. (2018). Deciphering microbial interactions in synthetic human gut microbiome communities. Mol. Syst. Biol. 14:e8157. doi: 10.15252/msb.20178157
Wang, L., Zhao, X., Xu, H., Bao, X., Liu, X., Chang, Y., et al. (2018). Characterization of the bacterial community in different parts of the gut of sea cucumber (Apostichopus Japonicus) and its variation during gut regeneration. Aquac. Res. 49, 1987–1996. doi: 10.1111/are.13654
Wang, T., Sun, Y., Jin, L., Thacker, P., Li, S., and Xu, Y. (2013). Aj-rel and Aj-p105, two evolutionary conserved NF-κB homologues in sea cucumber (Apostichopus japonicus) and their involvement in LPS induced immunity. Fish Shellfish Immunol. 34, 17–22. doi: 10.1016/j.fsi.2012.09.006
Wang, Y., Wang, K., Huang, L., Dong, P., Wang, S., Chen, H., et al. (2020). Fine-scale succession patterns and assembly mechanisms of bacterial community of Litopenaeus vannamei larvae across the developmental cycle. Microbiome 8:106. doi: 10.1186/s40168-020-00879-w
Watts, D. J., and Strogatz, S. H. (1998). Collective dynamics of ‘small-world’ networks. Nature 393, 440–442. doi: 10.1038/30918
Xiong, J., Dai, W., Zhu, J., Liu, K., Dong, C., and Qiu, Q. (2017). The underlying ecological processes of gut microbiota among cohabitating retarded, overgrown and normal shrimp. Microb. Ecol. 73, 988–999. doi: 10.1007/s00248-016-0910-x
Yamazaki, Y., Meirelles, P. M., Mino, S., Suda, W., Oshima, K., Hattori, M., et al. (2016). Individual Apostichopus japonicus fecal microbiome reveals a link with polyhydroxybutyrate producers in host growth gaps. Sci. Rep. 6:21631. doi: 10.1038/srep21631
Yang, G., Tian, X., Dong, S., Peng, M., and Wang, D. (2015a). Effects of dietary Bacillus cereus G19, B. cereus BC-01, and Paracoccus marcusii DB11 supplementation on the growth, immune response, and expression of immune-related genes in coelomocytes and intestine of the sea cucumber (Apostichopus japonicus Selenka). Fish Shellfish Immunol. 45, 800–807. doi: 10.1016/j.fsi.2015.05.032
Yang, G., Xu, Z., Tian, X., Dong, S., and Peng, M. (2015b). Intestinal microbiota and immune related genes in sea cucumber (Apostichopus japonicus) response to dietary β-glucan supplementation. Biochem. Biophys. Res. Commun. 458, 98–103. doi: 10.1016/j.bbrc.2015.01.074
Zhang, J., Shishatskaya, E. I., Volova, T. G., da Silva, L. F., and Chen, G. Q. (2018). Polyhydroxyalkanoates (PHA) for therapeutic applications. Mater. Sci. Eng. C 86, 144–150. doi: 10.1016/j.msec.2017.12.035
Zhao, Y., Yuan, L., Wan, J., Sun, H., Wang, Y., and Zhang, Q. (2018). Effects of a potential autochthonous probiotic Bacillus subtilis 2-1 on the growth and intestinal microbiota of Juvenile Sea cucumber, Apostichopus japonicus Selenka. J. Ocean Univ. China 17, 363–370. doi: 10.1007/s11802-018-3402-4
Zhao, Y., Zhang, W., Xu, W., Mai, K., Zhang, Y., and Liufu, Z. (2012). Effects of potential probiotic Bacillus subtilis T13 on growth, immunity and disease resistance against Vibrio splendidus infection in juvenile sea cucumber Apostichopus japonicus. Fish Shellfish Immunol. 32, 750–755. doi: 10.1016/j.fsi.2012.01.027
Keywords: growth performance, non-specific immune enzymes, immune-related genes, intestinal microbiota, Apostichopus japonicus, poly-β-hydroxybutyrate
Citation: Liu L, Wang M, Wei C, Liu Y, Pan M, Wang S, Cui L and Tian X (2022) Effects of Dietary Poly-β-Hydroxybutyrate Supplementation on the Growth, Non-specific Immunity, and Intestinal Microbiota of the Sea Cucumber Apostichopus japonicus. Front. Mar. Sci. 9:855938. doi: 10.3389/fmars.2022.855938
Received: 16 January 2022; Accepted: 27 January 2022;
Published: 25 February 2022.
Edited by:
Yun-Zhang Sun, Jimei University, ChinaReviewed by:
Chenghua Li, Ningbo University, ChinaYichao Ren, Qingdao Agricultural University, China
Copyright © 2022 Liu, Wang, Wei, Liu, Pan, Wang, Cui and Tian. This is an open-access article distributed under the terms of the Creative Commons Attribution License (CC BY). The use, distribution or reproduction in other forums is permitted, provided the original author(s) and the copyright owner(s) are credited and that the original publication in this journal is cited, in accordance with accepted academic practice. No use, distribution or reproduction is permitted which does not comply with these terms.
*Correspondence: Xiangli Tian, eGlhbmdsaXRpYW5Ab3VjLmVkdS5jbg==