- 1Key Laboratory of Marine Environment and Ecology, Ministry of Education, Ocean University of China, Qingdao, China
- 2Marine Ecology and Environmental Science Laboratory, Pilot National Laboratory for Marine Science and Technology, Qingdao, China
Understanding the diel vertical migration (DVM) patterns of zooplankton has important implications for biological pumping and pelagic food webs. The functional traits of zooplankton generally determine their distribution in the environment. However, knowledge about the DVM patterns of zooplankton with different functional traits is limited. Here, we used a trait-based approach to study the vertical distributions of zooplankton in the North Pacific Subtropical Gyre (NPSG). Four functional traits, namely, body length, feeding type, trophic group, and reproductive mode, were selected in this study. A high biodiversity of zooplankton (165 taxa) was recorded in the NPSG. Zooplankton was mainly concentrated above 100 m and exhibited normal DVM. Zooplankton with small and medium body sizes (<2 mm, 69% ± 16%), current feeding (43% ± 14%), and omnivore–herbivores (54% ± 14%) were dominant in the NPSG. The proportion of sac spawners in total abundance (52% ± 14%) was higher than that of broadcast spawners (48% ± 14%), which differed from that in the coastal regions. Sixteen functional groups of zooplankton were identified. Different functional groups exhibited distinct DVM patterns due to the influence of different factors. The DVM patterns of current-feeding omnivore–herbivores were significantly correlated with chlorophyll a, whereas giant ambush-feeding carnivores exhibited normal DVM patterns and were substantially correlated with the vertical distribution of their food organisms. However, the small omnivore–carnivores showed reverse DVM patterns. Overall, this study provided a new perspective for studies on zooplankton DVM from the aspect of functional traits.
Introduction
Zooplankton play crucial roles in marine ecosystems as they link the primary production of the pelagic food web to higher trophic levels (Banse, 1995; Wassmann et al., 2006). The diel vertical migration (DVM) of zooplankton is a common phenomenon in oceans, in which zooplankton feed in the surface layer at night and migrate to deeper layers during the day (Putzeys and Hernández-León, 2005; Steinberg and Landry, 2017). Zooplankton DVM plays a vital role in the downward flux of particulate and dissolved matters. Migrators could accelerate the vertical flux of carbon by feeding in superficial layers and defecating at depth (Longhurst and Harrison, 1989; Putzeys and Hernández-León, 2005).
Numerous studies in the field and laboratory have shown that zooplankton DVM can be initiated or influenced by environmental factors such as light, oxygen, and temperature (Hays, 2003; Putzeys and Hernández-León, 2005). The zooplankton community contains various organisms with different body sizes, feeding types, trophic groups, and reproductive modes (Pomerleau et al., 2015; Brun et al., 2016); thus, interactions such as predation and competition among organisms may also affect the DVM patterns of zooplankton (Pinti et al., 2019). Most previous studies on zooplankton DVM have used traditional taxonomic methods, but these methods are incapable of identifying potential zooplankton interactions. The trait-based method is also used to describe pelagic ecosystem dynamics and functions, which is to classify groups of species with similar functional traits (Pomerleau et al., 2015; Benedetti et al., 2018). Identifying and describing zooplankton functional groups allows us to move beyond reliance on taxonomical affiliation, providing greater insight into the distribution and functional roles of zooplankton in marine ecosystems (Pomerleau et al., 2015; Kiørboe et al., 2018). This approach has been proven useful for solving complex issues in marine ecosystems (Kiørboe et al., 2018). For instance, Venello et al. (2021) studied a 35-year time series of zooplankton communities off the west coast of Vancouver Island, Canada, indicating that the relative contribution of some functional groups changed with environmental variables such as sea surface temperature, the North Pacific Gyre Oscillation, and the Pacific Decadal Oscillation. Additionally, predator–prey interactions often occur simultaneously among different species, and the trait-based approach can be useful in these cases as it can significantly reduce system complexity (Pinti et al., 2019).
The North Pacific Subtropical Gyre (NPSG) ecosystem is the largest interconnected biome on earth, and zooplankton play vital roles in the food web of this open-sea system (Choy et al., 2015). The NPSG is characterized by low nutrient concentrations and high biodiversity (Hayward et al., 1983; Karl and Church, 2017). Studies on the zooplankton community and vertical distribution in the NPSG are extensively documented (Steinberg et al., 2008; Shimode et al., 2009; Hannides et al., 2015; Sun et al., 2019). Copepods are the dominant group of zooplankton in the NPSG, followed by ostracods and chaetognaths (Steinberg et al., 2008; Sun et al., 2019). Moreover, small-sized zooplankton (<2 mm) are abundant in the NPSG, and the proportions of zooplankton with distinct body sizes differ in each sampling layer (Steinberg et al., 2008; Hannides et al., 2015). Additionally, zooplankton are mainly distributed above 200 m depth in the NPSG and exhibit a substantial normal DVM (Shimode et al., 2009; Sun et al., 2019). Meanwhile, significant seasonality of biomass changes and the vertical scale of the diel migration of zooplankton were reported in the NPSG (Inoue et al., 2016; Valencia et al., 2016). Inoue et al. (2016) indicated that the bulk zooplankton biomass was higher in winter and spring in the NPSG, while biomass peaks were observed during the summer months of highest productivity at the ALOHA station (Landry et al., 2001). The peak biomass of zooplankton extended to 100 and 150 m in winter and autumn, respectively, whereas it was confined to between 0 and 50 m in spring (Inoue et al., 2016). However, the vertical distribution patterns of zooplankton functional traits in the NPSG remain unclear. Increasingly, zooplankton functional traits can be characterized by their diel vertical distribution. This can advance our understanding of zooplankton DVM patterns and their controlling factors in the NPSG.
In this study, we used a trait-based approach to study the functional traits, DVM patterns, and influence factors of zooplankton in the NPSG. The goals of this study were to (1) reveal the compositions of zooplankton functional traits and their diel variation, and (2) examine the DVM patterns of functional groups of zooplankton and controlling factors. This study could provide a new perspective for the study of zooplankton DVM from the aspect of functional traits and elucidate the DVM patterns and driving factors of zooplankton with different functional traits.
Materials and Methods
Field Sampling
Data were obtained on board RV “Dong Fang Hong 2” at a fixed station B9 with a water depth of 5,500 m (147°E, 30°N; Figure 1). Zooplankton were sampled by vertical tows using a Multi Plankton Sampler Multinet (Midi type, 0.25 m2 mouth, 200 μm mesh size), equipped with a calibrated flowmeter, from five strata (0–20, 20–50, 50–100, 100–150, and 150–200 m). In the NPSG, mesozooplankton mainly spread above 200 m, while their abundance was low in deeper layers (Hannides et al., 2015). Thus, we collected zooplankton samples above 200 m depth. Sampling began on 10 April 2015 at 05:00 am and ended on April 11 at 05:10 am (local time), totaling four times over a 24-h period (05:00, dawn; 13:00, daytime; 21:00, nighttime; 05:10, dawn). A total of 20 samples were collected, and zooplankton samples were preserved in a 5% formalin–seawater solution immediately after each towing. Temperature, salinity, and chlorophyll (Chl) a concentration vertical profiles were regularly recorded with a CTD system (Sea-Bird SBE 911) throughout the sampling period. The CTD system was calibrated at the National Center of Ocean Standards and Metrology in October 2014, and the fluorometer was calibrated in situ during the cruise following Lawrenz and Richardson (2011). Additionally, the effect of phytoplankton non-photochemical quenching on Chl a concentration was not considered in this study due to the lack of photosynthetically active radiation data.
Laboratory Work
Zooplankton samples were analyzed under a stereo microscope (Leica S8APO) in the laboratory. All zooplankton taxa present in the samples were identified at species level when possible. For this purpose, a subsample was obtained from each sample by using a Folsom plankton splitter. Its volume was determined on the basis of the density of organisms in the original sample, which include at least 500 adult individuals. To identify the presence of zooplankton DVM, zooplankton abundances in each layer were standardized as the number of individuals per 1 m2 (Dagg et al., 1998; Al-Mutairi and Landry, 2001; Madin et al., 2001). The wet weight biomass of zooplankton samples was measured using an analytical balance (SHPSI, JA2003N).
Functional Traits and Functional Groups
We selected four functional traits extracted from Benedetti et al. (2016): mean body length (mm), trophic group, feeding mode, and egg-spawning strategy. These traits cover distinct, crucial ecological functions and could influence marine ecosystem processes (Hébert et al., 2017). Trait information was primarily obtained from previous studies (Thompson, 1948; Zheng et al., 1984; Chen and Cao, 1990; Chen and Shi, 2002; Xiao, 2004; Xu et al., 2014; Benedetti et al., 2016), and online sources (http://www.eol.org; http://copepodes.obs-banyuls.fr/en). Pelagic larvae are very complex and their trait information is difficult to obtain (Krztoń and Kosiba, 2020). Additionally, the proportion of pelagic larvae was low in total zooplankton abundance in the NPSG (Steinberg et al., 2008; Sun et al., 2019). Thus, data on pelagic larvae were excluded from trait analyses.
a. Body size, a “master trait,” transcends and scales with various traits related to ecosystem processes, such as nutrient transfer, secondary productivity, and marine carbon cycling (Litchman et al., 2013; Hébert et al., 2016; Hébert et al., 2017). The mean adult body lengths were assigned to four length types: <1, 1–2, 2–5, and >5 mm (Steinberg et al., 2008; Hannides et al., 2015; Benedetti et al., 2016; Benedetti et al., 2018). We defined zooplankton with body lengths of <1, 1–2, 2–5, and >5 mm as small, medium, large, and giant zooplankton, respectively.
b. Prey selection, energy allocation, and nutrient cycling are all strongly influenced by feeding type (Litchman et al., 2013; Hébert et al., 2017). Feeding type was classified into four types: current feeding, ambush feeding, cruise feeding, and mixed feeding for species that could switch between two types (Benedetti et al., 2016). We defined current and cruise feeding as active-feeding strategy, and ambush feeding as a passive-feeding mode (Kiørboe, 2011; Brun et al., 2016).
c. The trophic group describes the primary food source of a species and thus its role in food-web dynamics (Pomerleau et al., 2015). Trophic group was classified into four types: carnivore, omnivore–herbivore, omnivore–carnivore, and omnivore–detritivore (Benedetti et al., 2018). Carnivores are predatory zooplankton that prey on small zooplankton, eggs, and larvae; omnivore–herbivores refer primarily to herbivorous species that occasionally feed on other small organisms or organic detritus; omnivore–carnivore refer primarily to carnivorous species that sometimes eat phytoplankton and organic detritus; and omnivore–detritivore refers to species that feed mostly on organic detritus and sometimes eat phytoplankton.
d. Spawning strategy influences zooplankton energy allocations (Litchman et al., 2013). The reproductive mode was defined as the egg-spawning strategy and was classified into three types: broadcast spawner, sac spawner, and binary fission (Benedetti et al., 2016). In this study, binary fission was mainly the reproductive mode of protozoans.
Zooplankton functional groups were assigned on the basis of four functional traits (Table S1). The trait types were assigned to each species, forming a trait matrix. The species were coded on the basis of the categories of each biological traits in terms of a character displayed using a binary code (Zhong et al., 2020). The score was 0 or 1, corresponding to no affinity or affinity for a trait modality, respectively. The trait matrix was used to calculate a dissimilarity matrix (with Gower distance). Then, agglomerative hierarchical clustering analysis (Ward’s method) was performed for species on the basis of similarity/dissimilarity of functional traits. This calculation was performed using the “factoextra” package in R (version 4.0.0) (Kassambara and Mundt, 2017). To examine the correlation of vertical migration between functional groups, the final cutting level was selected to ensure that groups were functionally homogeneous while also attempting to avoid functional redundancies between the groups (Benedetti et al., 2018). Functional dispersion (FDis) is the multivariate analog of the weighted mean absolute deviation (Laliberté and Legendre, 2010) and is calculated with the “dbFD” function in the FD package in R (version 4.0.0).
Correlations between each functional group and biotic and abiotic factors were conducted using the “ggcor” package, and were visualized using the “ggplot” packages in R (version 4.0.0). Redundancy analysis (RDA) was used to explain the relationship between functional groups and environmental factors in different stations and depth layers (Lepš and Šmilauer, 2003). The RDA results were visualized using CANOCO 5.0. Before the analysis, all environmental factors and the abundance of zooplankton were log10 (x + 1) transformed. Transformed abundances of zooplankton that belong to a functional group were aggregated, and the functional groups were incorporated into the analysis (Table S2). Environmental factors were used as explanatory variables. Average values of environmental factors in each stratum were used for the estimation.
Results
Hydrography
The vertical profiles of sea temperature and salinity showed obvious diel variations, especially at 20–120 m depth (Figure 2). Temperature and salinity decreased from the surface to the depths, with values ranging from 17.2–20.6°C and 34.8–34.9 psu, respectively. The vertical distribution of Chl a concentration showed diel variation. Deep Chl maxima were recorded at 50–70 m depth at four sampling times (Figure 2).
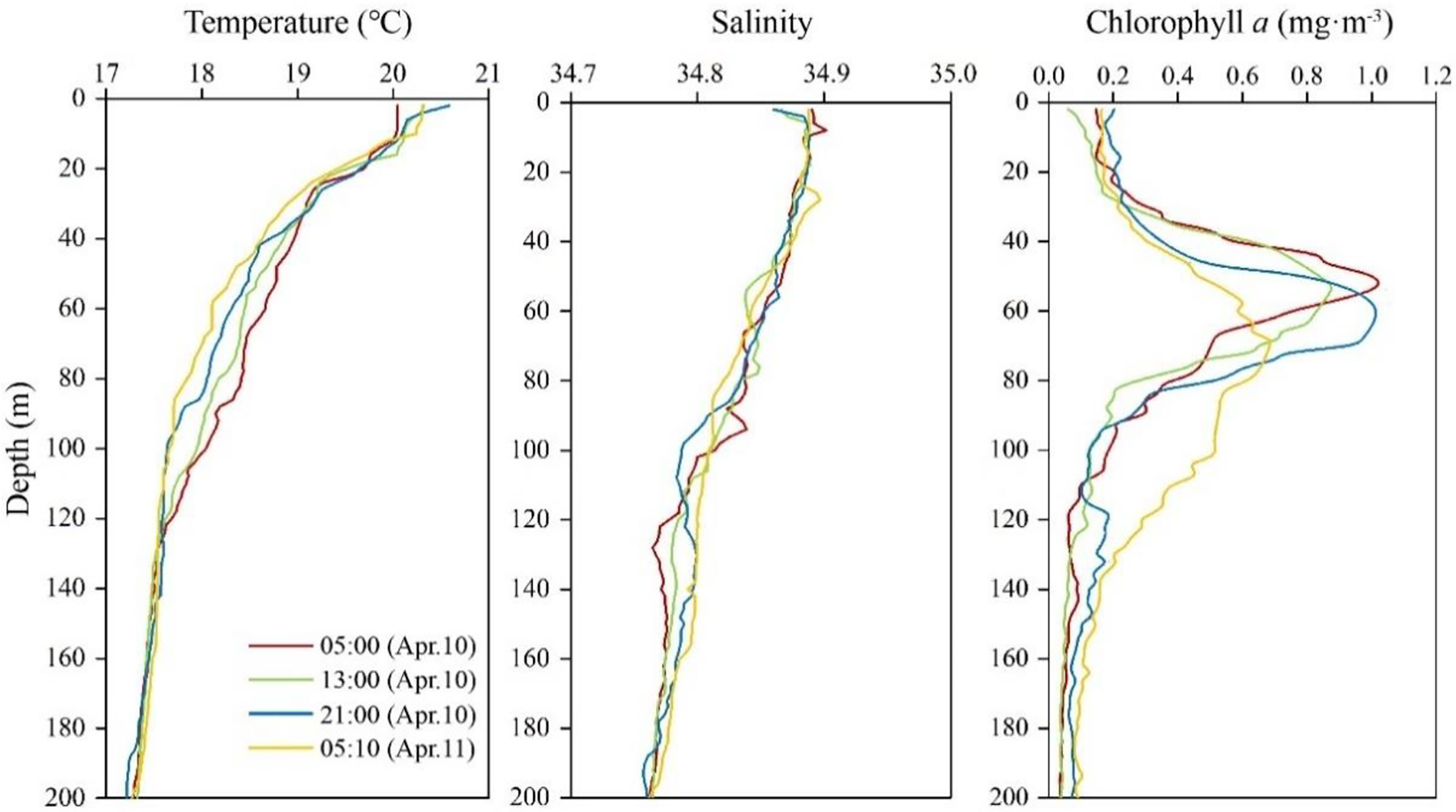
Figure 2 Vertical distribution of temperature, salinity, and chlorophyll a concentration above 200 m at the four sampling times.
Zooplankton Species Composition and Abundance
A total of 165 zooplankton taxa were recorded in the fixed station, and copepods were the dominant components (92 taxa; Figure S1A). The vertical distribution of species richness showed remarkable diel variations (Figure S1B). Except for the 20–50 m layer species richness above 200 m depth was greater at dawn and night than during the day,. Additionally, the vertical distributions of total zooplankton abundance also showed substantial diel variation (Figure 3). Zooplankton were primarily distributed in the 0–20 m layer at nighttime, and the maximum abundances of zooplankton at dawn and daytime were detected in the 20–50 and 50–100 m layers, respectively, attesting that zooplankton performed normal DVM. The diel vertical distribution of zooplankton biomass was similar to that of their abundances (Figure S2).
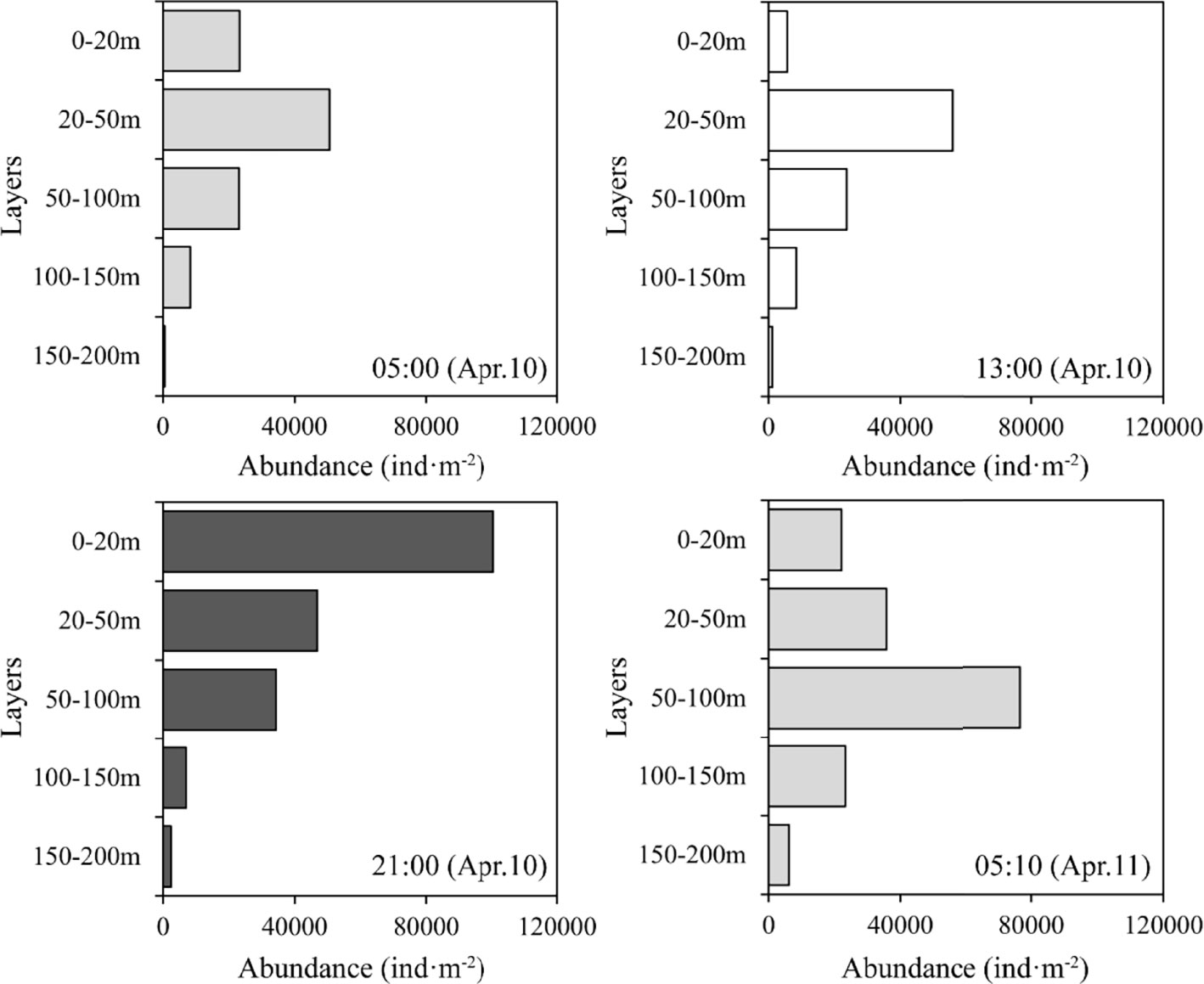
Figure 3 Diel vertical distribution of total zooplankton abundance. (gray: dawn; white: daytime; black: nighttime).
Vertical Distribution of Zooplankton Functional Traits
The percentages of zooplankton with distinct body lengths obviously differed among the layers. Small and medium zooplankton dominated each layer (69% ± 16%), whereas giant zooplankton accounted for small percentages (3% ± 4%) in each stratum (Figure 4A). The proportion of large zooplankton in the 0–20 m layer substantially increased at nighttime, whereas it was low at daytime and dawn (Figure 4A). The diel vertical distribution of large zooplankton abundance was similar to the diel variation in its proportion (Figure S3A). The distinct feeding types of zooplankton also occupied different proportions of the total abundance (Figure 4B): current-feeding zooplankton was prevalent (43% ± 14%), whereas mixed-feeding zooplankton was rare (<4%). The proportions of current feeders in the surface layer showed obvious increases at nighttime compared with those at daytime and dawn (Figure 4B; Figure S3B). As for trophic group, omnivore–herbivores were the most abundant components (54% ± 14%), followed by the omnivore–carnivores and carnivores, whereas omnivore–detritivores were rare (4% ± 6%) (Figure 4C). The proportion of omnivore–herbivores in the 0–20 m layer increased substantially at nighttime, whereas they were low at daytime and dawn (Figure 4C; Figure S3C). As for the reproductive mode, sac spawners (52% ± 14%) were an important group of zooplankton, followed by broadcast spawners (48% ± 14%). The proportion of zooplankton with a binary fission reproductive strategy was low in this study (Figure 4D). The proportions of broadcast spawners in the surface layer obviously increased at nighttime compared with those at daytime and dawn (Figure 4D; Figure S3D).
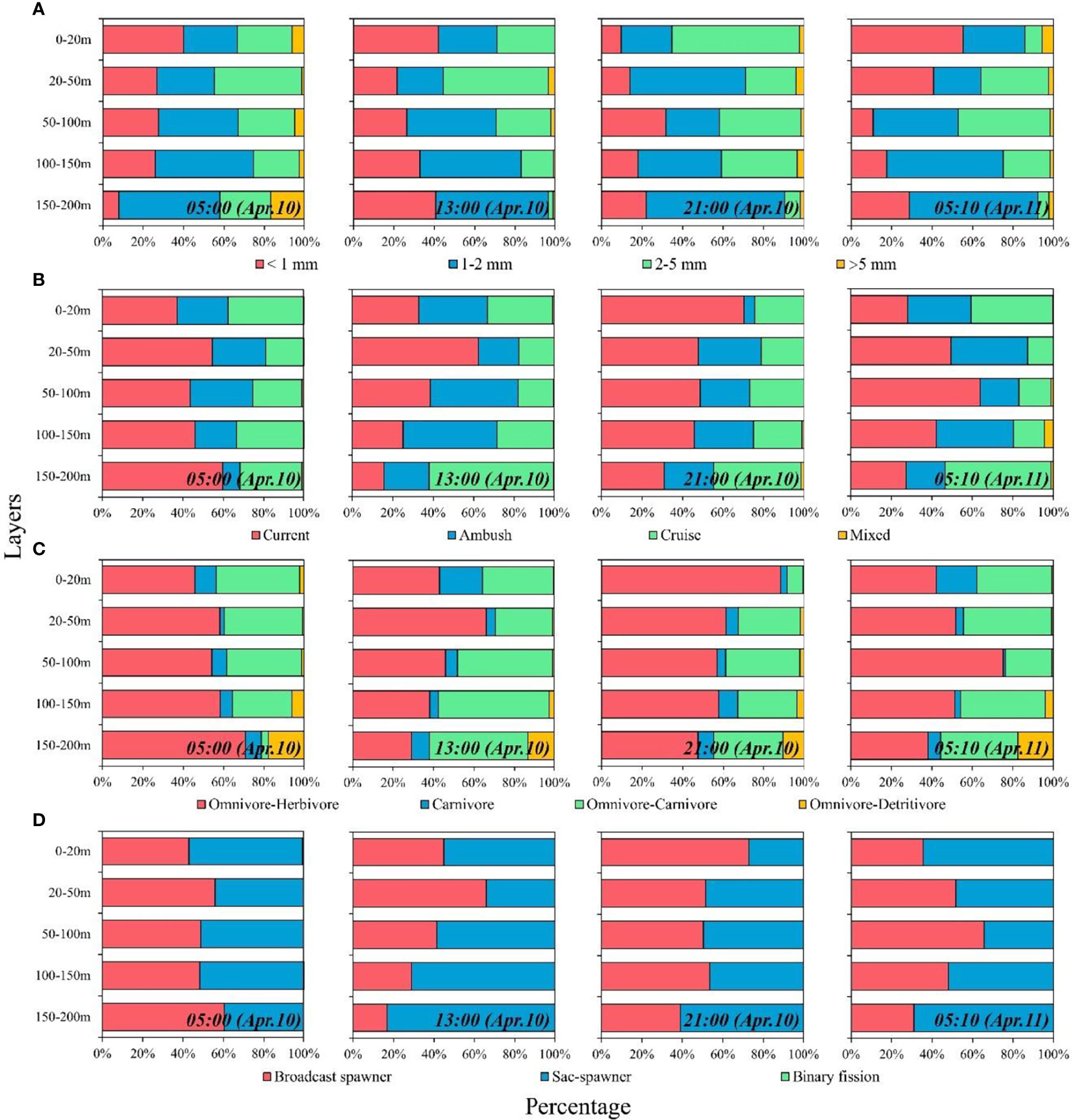
Figure 4 Diel vertical distribution of each functional trait. (A) Body length; (B) feeding type; (C) trophic group; and (D) reproductive mode.
Values of functional dispersion (FDis) were calculated to refer to the variability of traits (Table S3). The lowest FDis value in the NPSG was recorded at 21:00 in the 0–20 m (2.70), followed by FDis at 5:10 in the 50–100 m (3.14) and at 13:00 in the 20–50 m (3.27). FDis had relatively minor fluctuations at other sampling times.
Functional Groups and DVM
Zooplankton were classified into 16 functional groups based on a dendrogram resulting from cluster analysis (Figure 5; Table 1). Group 1 comprised large omnivore–herbivores that had the characteristics of current feeding. These species were broadcasters (Figure 5; Table 1). Group 1 was the most dominant group and was primarily distributed above 150 m depth, whereas these abundances were low in the 150–200 m layer (Figure S4). Group 1 exhibited obviously normal DVM patterns, which were primarily concentrated in the 0–20 m layer at nighttime, whereas they migrated to deep layers at dawn and daytime (Figure 6; Figures S4, S5). Group 2 comprised medium omnivore–herbivores (Figure 5; Table 1). Group 2 was primarily distributed in the 20–50 m layer and showed no obvious DVM (Figure 6; Figure S4). Group 3 was defined by medium and large cruise feeders, sac spawners, and mostly carnivore and omnivore–herbivore species (Figure 5; Table 1; Table S1). The abundances of the omnivore–herbivores species (Clausocalanus arcuicornis and C. furcatus) accounted for more than 98.0% of the total in Group 3. Group 3 showed an obvious normal DVM trend, the abundance in the surface layer substantially increased at nighttime compared with daytime (Figure 6; Figure S4).
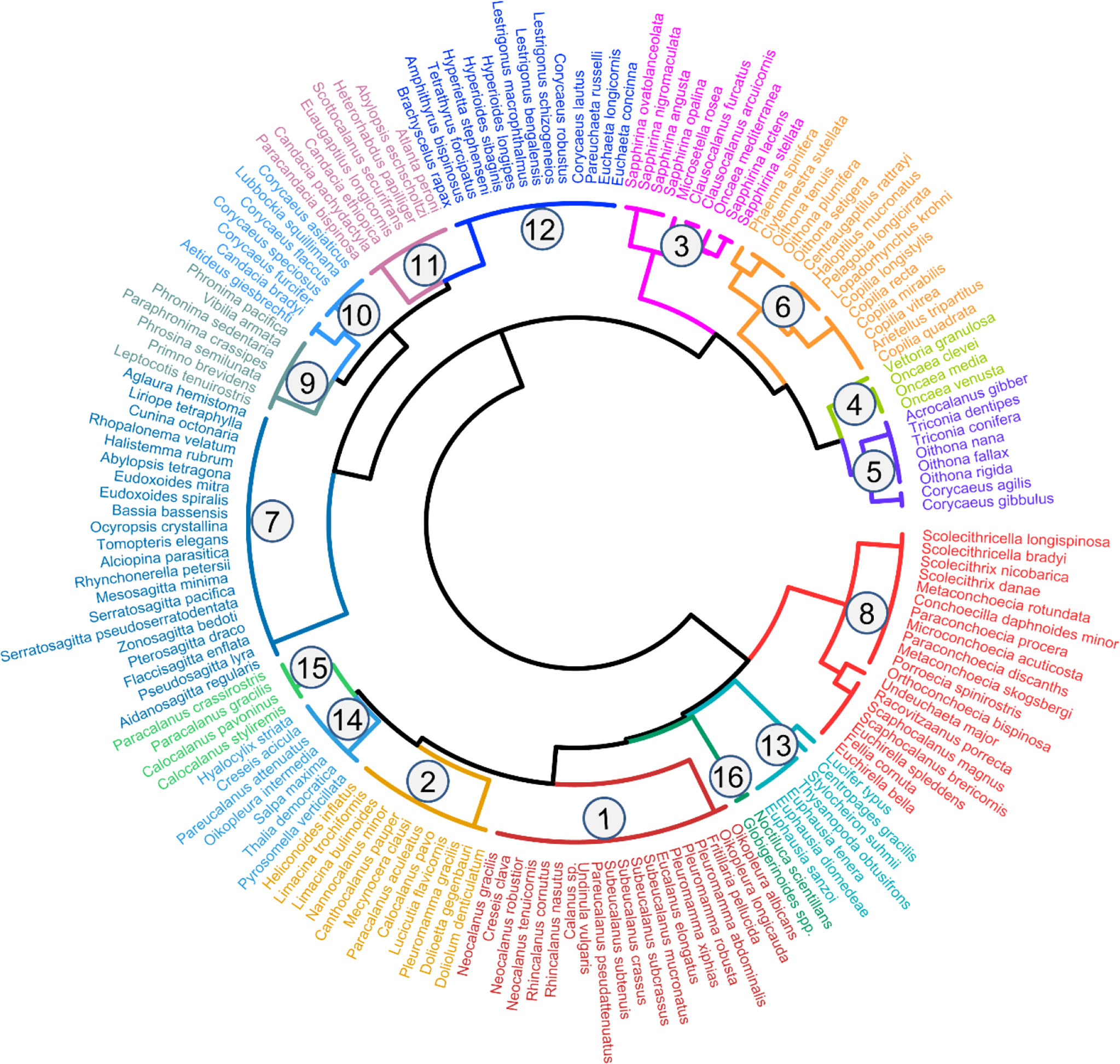
Figure 5 Dendrogram of functional groups of zooplankton identified on the basis of functional traits (numbers represent each group).
Group 4 gathered a lesser number of cyclopoid copepods, i.e., genera Vettoria and Oncaea. These species were small omnivore–carnivore, sac spawners, and a exhibited cruise-feeding strategy (Figure 5; Table 1). Group 4 showed a normal DVM pattern (Figure 6; Figure S4). The functional traits of Group 5 were similar to those of Group 4 (except for feeding type), which exhibited an ambush-feeding strategy. However, Group 5 exhibited reverse DVM, and they were primarily spread in the 50–100 m layer at nighttime but migrated to the 0–20 m layer at dawn and daytime (Figure 6; Figure S4). Group 6 consists primarily of medium and large omnivore–carnivore species, ambush feeders, and primarily sac spawners (Figure 5; Table 1; Table S1). Group 6 was mostly distributed below 20 m depth, and an obviously normal DVM for this group was recorded between 20 and 150 m depth (Figure 6; Figure S4). The body size of Group 7 was the largest and comprised chaetognaths and medusae. These species were giant carnivore broadcasters and exhibited an ambush feeding strategy (Figure 5; Table 1; Table S1). Group 7 showed an evident normal DVM trend, which migrated to the 20–50 m layer at daytime, whereas they were primarily distributed in the surface layer at dawn and nighttime (Figure 6; Figure S4). Although Group 7 contained 21 species, abundance was low (<6%; Table S2).
Group 8 comprised omnivore–detritivore species, exhibited a current-feeding strategy, and were medium and large in size. The reproductive strategy of this group was primarily broadcaster (Figure 5; Table 1; Table S1). Group 8 showed no obvious diel vertical variations and accounted for a high proportion (>10%) of the total abundance in the 150–200 m layer compared with other layers (0.4%–5%) (Figure 6). The vertical distribution pattern of Group 8 may be related to their trophic type, which were omnivore–detritivores. Additionally, the abundances of Groups 9–16 were low, accounting for 8% ± 6% of the total abundance of zooplankton (Figure 6).
Correlation of Zooplankton With Biotic and Abiotic Factors
Correlation analyses showed that Groups 4 and 6 showed significantly positive correlations with Chl a (p <0.01; Figure 7). Group 7 showed a significantly positive correlation with many other groups, such as Groups 1, 2, and 3 (p <0.05; Table 1; Figure 7). Moreover, Group 7 was significantly positively correlated with Group 9 (p <0.05). Group 8 was only significantly correlated with Group 12, whose abundance was low (p <0.05; Figure 7).
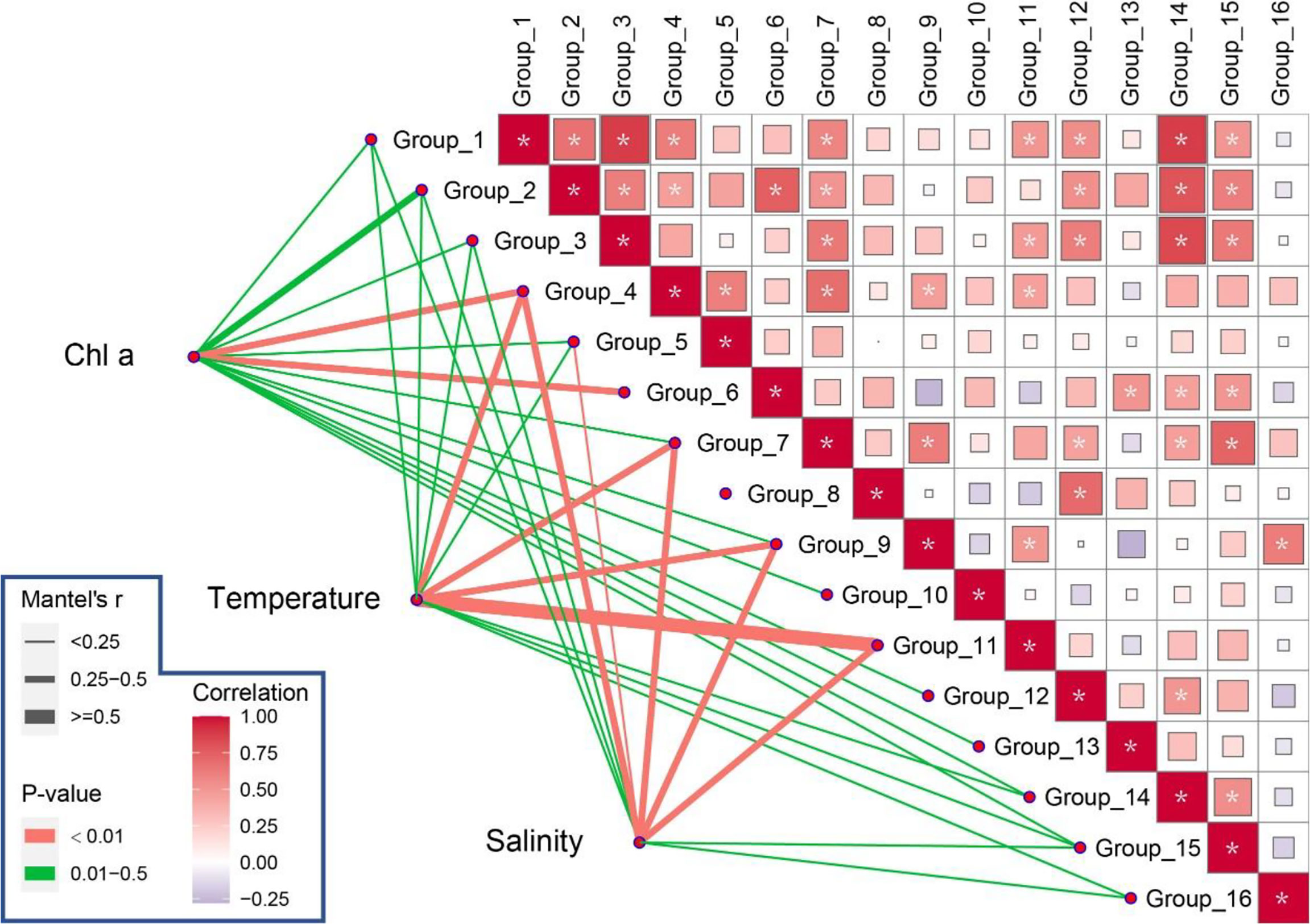
Figure 7 Interaction profile within and between groups and environmental factors by correlation analyses (*p <0.05).
Abiotic factors also affected the diel vertical distributions of zooplankton (Figure 7; Figure S6). Correlation analyses showed that Groups 4, 7, 9, and 11 had significant positive correlations with temperature (p <0.01; Figure 7), whereas Groups 4, 5, 7, 9, and 11 were significantly correlated with salinity (p <0.01; Figure 7). RDA results showed that the vertical distribution of zooplankton functional traits had the highest correlation with Chl a (F = 5.8, p = 0.008), while salinity (F = 8.9, p = 0.002) and Chl a (F = 5.4, p = 0.004) were crucial environmental factors affecting the vertical distributions of functional groups (Figure S6).
Discussion
This study revealed the composition of zooplankton functional traits, DVM patterns of functional groups, and their controlling factors in the NPSG. In the NPSG, zooplankton with small and medium body sizes, current feeding, omnivore–herbivores, and sac spawners were dominant. The distinct functional groups exhibited different DVM patterns, which were controlled by different factors. This study highlighted the advantages of the trait-based method in studying zooplankton DVM; that is, it can substantially reduce the complexity of the zooplankton community and can better analyze its controlling factors, especially the interaction between organisms.
Functional traits of marine zooplankton vary across the global ocean as a function of the environment (Brun et al., 2016). This study found that that small and medium zooplankton were dominant in oligotrophic NPSG, which agrees with previous findings that small-sized (<2 mm) zooplankton were abundant in the NPSG (Steinberg et al., 2008; Hannides et al., 2015). This could be explained by the size of phytoplankton size in the oligotrophic NPSG, where picophytoplankton contributed 74% of primary production on average (Li et al., 2011). Phytoplankton of small size was more suitable for the feeding of small and medium zooplankton (Boyce et al., 2015), thereby resulting in a higher proportion of small-sized zooplankton in oligotrophic NPSG. As for the feeding strategy of zooplankton, a substantially latitudinal pattern was observed as passive-feeding zooplankton was dominant in the high-latitude regions, while active-feeding zooplankton increased in low-latitude regions, such as NPSG (Prowe et al., 2018). The low Chl a concentration in the NPSG limited the food for zooplankton growth and reproduction (Steinberg et al., 2008). The encounter rates between high motility-predators and preys were higher than those of passive predators (Prowe et al., 2018), and active-feeding zooplankton may be more adaptable to the oligotrophic NPSG.
Additionally, it is worth noting that the reproductive mode of zooplankton in the oligotrophic NPSG was substantially different from that in coastal regions. This study showed that sac spawners were more abundant (52%) in the NPSG. However, Da Conceição et al. (2021) indicated that a high proportion of broadcasters (>70%) of the total abundance were detected in coastal regions. Generally, r-selected broadcasters live in variable or unstable habitats (Lalli and Parsons, 2004) and exhibit high fecundity in the marine ecosystem with a high Chl a concentration, whereas their fecundity is substantially reduced in oligotrophic regions (Bunker and Hirst, 2004). Thus, r-selected broadcasters may be more adapted to meso- and eutrophic marine ecosystems (Tundisi and Tundisi, 1995). However, K-selected sac spawners prefer to reside in stable environments (Lalli and Parsons, 2004), i.e., oligotrophic ecosystems, and they can effectively absorb and use limited resources in the oligotrophic environments, indicating that K-selected zooplankton could better tolerate such environments (Tundisi and Tundisi, 1995).
Generally, functional traits determine the fitness of marine organisms in the environment, namely, ecological selection and trophic interactions (Brun et al., 2016; Pinti and Visser, 2019). In this study, the large current-feeding omnivore–herbivores and giant ambush-feeding carnivores exhibited normal DVM patterns. Large zooplankton were more likely to be prey to visual predators compared with small and medium organisms (Hays, 2003; Pinti et al., 2019). Thus, these functional groups migrated onto the surface to feed at night and simultaneously avoid being hunted by visual predators. Ohman and Romagnan (2016) also found that zooplankton with 2.5–7 mm body length used deeper waters as a refuge by day and foraged in shallow strata by night. Moreover, giant ambush-feeding carnivores, such as medusae and chaetognaths, were significantly correlated with their prey in the NPSG, which was also recorded in many other marine systems (Nagasawa, 1991; Lučić et al., 2009; Júnior et al., 2015; Ge et al., 2021). As for medium omnivore–herbivores, their body sizes were small and it was difficult for them to be preyed on by visual predators even during the day (Ohman and Romagnan, 2016; Pinti et al., 2019), so they primarily distributed in the 20–50 m layer, where the Chl a concentration was abundant and they could concurrently avoid competition with large omnivore–herbivores. The small omnivore–carnivores exhibited obvious reverse DVM patterns, possibly to avoid predators such as giant ambush-feeding carnivores. The correlation analysis also indicated that the small omnivore–carnivores showed no significant positive correlation with its predators. Ohman et al. (1983) also indicated that the marine copepod Pseudocalanus sp. showed reverse diel vertical migration in Dabob Bay to reduce spatial overlap with their predators. Moreover, the small omnivore–carnivores feed primarily on ciliates, heterotrophic dinoflagellates, and bacterioplankton, all of which are abundant in the upper layer at day and night (Pérez et al., 2000; Turner, 2004; Wang et al., 2019). Thus, the reverse DVM patterns of the small omnivore–carnivores could not only avoid predators but also meet their feeding needs. Additionally, the giant carnivorous broadcast spawners showed a significant positive correlation with the giant carnivorous sac spawners (Figure 7), indicating that they might exist in ecological competition owing to similar ecological niches.
Zooplankton DVM increases vertical carbon export (Turner, 2015; Steinberg and Landry, 2017). Given the diversity of functional traits of different zooplankton taxa, the contributions of their DVM to carbon export substantially differ. This study showed that zooplankton with different functional traits may play different functions in the process of carbon export. For example, large current-feeding omnivore–herbivores and giant ambush-feeding carnivores may contribute to carbon flux by producing large fecal pellets and metabolism in their wide amplitude migration. Additionally, omnivore–detritivores in the deep layer may play a vital role in carbon export. They feed on sinking and suspended particles and repackage particles into new fecal pellets, thereby affecting vertical carbon export (Wilson et al., 2008; Cavan et al., 2017), although they showed no vertical migration. Conversely, small omnivore–carnivores with reverse DVM patterns and small migration amplitudes may contribute little to carbon flux despite their high abundance. A previous study has also suggested that high abundances of small-sized zooplankton such as Oithona spp. are negatively correlated with fecal pellet flux (Svensen and Nejstgaard, 2003), thereby reducing the efficiency of the biological pump, especially in oligotrophic ecosystems (Wilson et al., 2008). This study hints at the potential of trait-based analysis for carbon flux studies.
In this work, zooplankton samples were collected using a net with a mouth opening of 0.25 m2, which may not efficiently collect giant zooplankton such as euphausiids and decapods (Zhang and Dam, 1997). Thus, the abundance of giant zooplankton may be underestimated. Moreover, some gelatinous organisms such as pelagic tunicates and jellyfish are fragile during hauling the process, which may also be underestimated. Additionally, microzooplankton such as protozoans and ciliates are abundant in the oligotrophic Pacific (Uye et al., 1999), which also play vital roles in marine food webs. However, this study used a net with a mesh of 200 µm and examined mesozooplankton patterns, which may not efficiently collect microzooplankton. Their abundance may also be underestimated. Meanwhile, zooplankton DVM patterns are structured by many factors, such as UV light penetration, food availability, predation avoidance, and thermocline (Williamson et al., 2011; Palomares-García et al., 2013; Júnior et al., 2015; Sakinan and Gücü, 2016). Further research should pay attention to these influencing mechanisms and regulation of zooplankton functional groups. Additionally, high irradiance conditions can cause phytoplankton non-photochemical quenching, which leads to the measured values of Chl a concentration by the fluorometer being lower than the actual values (Falkowski and Kolber, 1995; Halverson and Pawlowicz, 2013). In this study, the surface Chl a concentration in the daytime (especially at 13:00) may be underestimated due to the effects of non-photochemical quenching, which consequently affects the correlation analysis and redundancy analysis.
In conclusion, zooplankton with small and medium body size, current feeding, and omnivore–herbivores were dominant in the NPSG. As for the reproductive mode, the proportion of sac spawners in total abundance was higher than that of broadcasters, which substantially differed from that in coastal ecosystems. Sixteen functional groups were identified in this study. The different functional groups exhibited distinct DVM patterns in the NPSG, and their controlling factors were also different. The distinct functional groups of zooplankton may play different potential roles in carbon export.
Data Availability Statement
The original contributions presented in the study are included in the article/Supplementary Material. Further inquiries can be directed to the corresponding author.
Author Contributions
HC conceived and coordinated the project. HC sampled and FL and HC classified zooplankton organisms. RG and YL measured biomass and analyzed the data with the help of HC. The manuscript was co-written by RG, YL, HC, and YZ. GL and FL helped to revise the work. All authors listed have made a substantial, direct, and intellectual contribution to the work and approved it for publication.
Funding
This study was funded by the National Natural Science Foundation of China (Nos. 42076146, 41876156).
Conflict of Interest
The authors declare that the research was conducted in the absence of any commercial or financial relationships that could be construed as a potential conflict of interest.
Publisher’s Note
All claims expressed in this article are solely those of the authors and do not necessarily represent those of their affiliated organizations, or those of the publisher, the editors and the reviewers. Any product that may be evaluated in this article, or claim that may be made by its manufacturer, is not guaranteed or endorsed by the publisher.
Acknowledgments
We are indebted to the captain and crew of the RV “Dong-Fang-Hong 2”, and appreciate the kind help of Yousong Huang, Weimin Wang, and Chenxi for sample collection on board. We are also grateful to the two reviewers for the highly constructive comments that improved the manuscript.
Supplementary Material
The Supplementary Material for this article can be found online at: https://www.frontiersin.org/articles/10.3389/fmars.2022.854642/full#supplementary-material
References
Al-Mutairi H., Landry M. R. (2001). Active Export of Carbon and Nitrogen at Station ALOHA by Diel Migrant Zooplankton. Deep Sea Res. PT II 48, 2083–2103. doi: 10.1016/S0967-0645(00)00174-0
Banse K. (1995). Zooplankton: Pivotal Role in the Control of Ocean Production. ICES J. Mar. Sci. 52, 265–277. doi: 10.1016/1054-3139(95)80043-3
Benedetti F., Gasparini S. P., Ayata S. E. (2016). Identifying Copepod Functional Groups From Species Functional Traits. J. Plankton Res. 38, 159–166. doi: 10.1093/plankt/fbv096
Benedetti F., Vogt M., Righetti D., Guilhaumon F., Ayata S. (2018). Do Functional Groups of Planktonic Copepods Differ in Their Ecological Niches? J. Biogeogr. 45, 604–616. doi: 10.1111/jbi.13166
Boyce D. G., Frank K. T., Leggett W. C. (2015). From Mice to Elephants: Overturning the ‘One Size Fits All’ Paradigm in Marine Plankton Food Chains. Ecol. Lett. 18, 504–515. doi: 10.1111/ele.12434
Brun P., Payne M. R., Kiørboe T. (2016). Trait Biogeography of Marine Copepods - an Analysis Across Scales. Ecol. Lett. 19, 1403–1413. doi: 10.1111/ele.12688
Bunker A. J., Hirst A. G. (2004). Fecundity of Marine Planktonic Copepods: Global Rates and Patterns in Relation to Chlorophyll a, Temperature and Body Weight. Mar. Ecol. Prog. Ser. 279, 161–181. doi: 10.3354/meps279161
Cavan E. L., Henson S. A., Belcher A., Sanders R. (2017). Role of Zooplankton in Determining the Efficiency of the Biological Carbon Pump. Biogeosciences 14, 177–186. doi: 10.5194/bg-14-177-2017
Chen Q., Shi C. (2002). Fauna Sinica, Invertebrata (Vol. 28), Phylum Arthropoda, Subphylum Crustacea, Order Amphipoda, Suborder Hyperiidea (Beijing: Science Press).
Choy C. A., Popp B. N., Hannides C. C. S., Drazen J. C. (2015). Trophic Structure and Food Resources of Epipelagic and Mesopelagic Fishes in the North Pacific Subtropical Gyre Ecosystem Inferred From Nitrogen Isotopic Compositions. Limnol. Oceanogr. 60, 1156–1171. doi: 10.1002/lno.10085
Da Conceição L. R., Souza C. S., de Oliveira Mafalda P., Schwamborn R., Neumann-Leitão S. (2021). Copepods Community Structure and Function Under Oceanographic Influences and Anthropic Impacts From the Narrowest Continental Shelf of Southwestern Atlantic. Reg. Stud. Mar. Sci. 47, 101931. doi: 10.1016/j.rsma.2021.101931
Dagg M. J., Frost B. W., Newton J. (1998). Diel Vertical Migration and Feeding in Adult Female Calanus Pacificus, Metridia Lucens and Pseudocalanus Newmani During a Spring Bloom in Dabob Bay, a Fjord in Washington USA. J. Marine Syst. 15, 503–509. doi: 10.1016/S0924-7963(97)00093-6
Falkowski P. G., Kolber Z. (1995). Variations in Chlorophyll Fluorescence Yields in Phytoplankton in the World Oceans. Funct. Plant Biol. 22, 341–355. doi: 10.1071/pp9950341
Ge R., Chen H., Liu G., Zhu Y., Jiang Q. (2021). Diel Vertical Migration of Mesozooplankton in the Northern Yellow Sea. J. Ocean. Limnol. 39, 1373–1386. doi: 10.1007/s00343-020-0170-y
Halverson M. J., Pawlowicz R. (2013). High Resolution Observations of Chlorophyll-a Biomass From an Instrumented Ferry: Influence of the Fraser River Plume From 2003-2006. Cont. Shelf Res. 59, 52–64. doi: 10.1016/j.csr.2013.04.010
Hannides C. C. S., Drazen J. C., Popp B. N. (2015). Mesopelagic Zooplankton Metabolic Demand in the North Pacific Subtropical Gyre. Limnol. Oceanogr. 60, 419–428. doi: 10.1002/lno.10032
Hays G. C. (2003). A Review of the Adaptive Significance and Ecosystem Consequences of Zooplankton Diel Vertical Migrations. Hydrobiologia 503, 163–170. doi: 10.1023/B:HYDR.0000008476.23617.b0
Hayward T. L., Venrick E. L., Mcgowan J. A. (1983). Environmental Heterogeneity and Plankton Community Structure in the Central North Pacific. J. Mar. Res. 41, 711–729. doi: 10.1357/002224083788520441
Hébert M. P., Beisner B. E., Maranger R. (2016). A Compilation of Quantitative Functional Traits for Marine and Freshwater Crustacean Zooplankton. Ecology 97, 1081. doi: 10.1890/15-1275.1
Hébert M. P., Beisner B. E., Maranger R. (2017). Linking Zooplankton Communities to Ecosystem Functioning: Toward an Effect-Trait Framework. J. Plankton Res. 39, 3–12. doi: 10.1093/plankt/fbw068
Inoue R., Kitamura M., Fujiki T. (2016). Diel Vertical Migration of Zooplankton at the S1 Biogeochemical Mooring Revealed From Acoustic Backscattering Strength. J. Geophys. Res. Oceans 121, 1031–1050. doi: 10.1002/2015JC011352
Júnior M. N., Brandini F. P., Codina J. C. U. (2015). Diel Vertical Dynamics of Gelatinous Zooplankton (Cnidaria, Ctenophora and Thaliacea) in a Subtropical Stratified Ecosystem (South Brazilian Bight). PloS One 10, e144161. doi: 10.1371/journal.pone.0144161
Karl D. M., Church M. J. (2017). Ecosystem Structure and Dynamics in the North Pacific Subtropical Gyre: New Views of an Old Ocean. Ecosystems 20, 433–457. doi: 10.1007/s10021-017-0117-0
Kassambara A., Mundt F. (2017) Factoextra: Extract and Visualize the Results of Multivariate Data Analyses. Available at: https://CRAN.R-project.org/package=factoextra.
Kiørboe T. (2011). How Zooplankton Feed: Mechanisms, Traits and Trade-Offs. Biol. Rev. 86, 311–339. doi: 10.1111/j.1469-185X.2010.00148.x
Kiørboe T., Visser A., Andersen K. H. (2018). A Trait-Based Approach to Ocean Ecology. ICES J. Mar. Sci. 75, 1849–1863. doi: 10.1093/icesjms/fsy090
Krztoń W., Kosiba J. (2020). Variations in Zooplankton Functional Groups Density in Freshwater Ecosystems Exposed to Cyanobacterial Blooms. Sci. Total Environ. 730, 139044. doi: 10.1016/j.scitotenv.2020.139044
Laliberté E., Legendre P. (2010). A Distance-Based Framework for Measuring Functional Diversity From Multiple Traits. Ecology 91, 299–305. doi: 10.1890/08-2244.1
Lalli C. M., Parsons T. R. (2004). Biological Oceanography: An Introduction (Oxford: Butterworth-Heinemann).
Landry M. R., Al-Mutairi H., Selph K. E., Christensen S., Nunnery S. (2001). Seasonal Patterns of Mesozooplankton Abundance and Biomass at Station ALOHA. Deep Sea Res. II 48, 2037–2061. doi: 10.1016/S0967-0645(00)00172-7
Lawrenz E., Richardson T. L. (2011). How Does the Species Used for Calibration Affect Chlorophyll a Measurement by in Situ Fluorometry? Estuar. Coast. 34, 872–883. doi: 10.1007/s12237-010-9346-6
Lepš J., Šmilauer P. (2003). Multivariate Analysis of Ecological Data Using CANOCO (Cambridge: Cambridge University Press).
Li B., Karl D. M., Letelier R. M., Church M. J. (2011). Size-Dependent Photosynthetic Variability in the North Pacific Subtropical Gyre. Mar. Ecol. Prog. Ser. 440, 27–40. doi: 10.3354/meps09345
Litchman E., Ohman M. D., Kiørboe T. (2013). Trait-Based Approaches to Zooplankton Communities. J. Plankton Res. 35, 473–484. doi: 10.1093/plankt/fbt019
Longhurst A. R., Harrison W. G. (1989). The Biological Pump: Profiles of Plankton Production and Consumption in the Upper Ocean. Prog. Oceanogr. 22, 47–123. doi: 10.1016/0079-6611(89)90010-4
Lučić D., Benović A., Morović M., Batistić M., Onofri I. (2009). Diel Vertical Migration of Medusae in the Open Southern Adriatic Sea Over a Short Time Period (July 2003). Mar. Ecol. Evol. Persp. 30, 16–32. doi: 10.1111/j.1439-0485.2008.00264.x
Madin L. P., Horgan E. F., Steinberg D. K. (2001). Zooplankton at the Bermuda Atlantic Time-Series Study (BATS) Station: Diel, Seasonal and Interannual Variation in Biomas-1998. Deep Sea Res. PT II 48, 2063–2082. doi: 10.1016/S0967-0645(00)00171-5
Nagasawa S. (1991). Vertical Distribution, Life Cycle and Production of the Chaetognath Sagitta Crassa in Tokyo Bay, Japan. J. Plankton Res. 13, 1325–1338. doi: 10.1093/plankt/13.6.1325
Ohman M., Frost B. W., Cohen E. B. (1983). Reverse Diel Vertical Migration: An Escape From Invertebrate Predators. Science 220, 1404–1407. doi: 10.1126/science.220.4604.1404
Ohman M., Romagnan J. B. (2016). Nonlinear Effects of Body Size and Optical Attenuation on Diel Vertical Migration by Zooplankton. Limnol. Oceanogr. 61, 765–770. doi: 10.1002/lno.10251
Palomares-García R. J., Gómez-Gutiérrez J., Robinson C. J. (2013). Winter and Summer Vertical Distribution of Epipelagic Copepods in the Gulf of California. J. Plankton Res. 35 (5), 1009–1026. doi: 10.1093/plankt/fbt052
Pérez M. T., Dolan J. R., Vidussi F., Fukai E. (2000). Diel Vertical Distribution of Planktonic Ciliates Within the Surface Layer of the NW Mediterranean (May 1995). Deep Sea Res. PT II 47, 479–503. doi: 10.1016/S0967-0637(99)00099-0
Pinti J., Kiørboe T., Thygesen U. H., Visser A. W. (2019). Trophic Interactions Drive the Emergence of Diel Vertical Migration Patterns: A Game-Theoretic Model of Copepod Communities. P. R. Soc B Bio. Sci. 286, 20191645. doi: 10.6084/m9.figshare
Pinti J., Visser A. W. (2019). Predator-Prey Games in Multiple Habitats Reveal Mixed Strategies in Diel Vertical Migration. Am. Nat. 193, E65–E71. doi: 10.1086/701041
Pomerleau C., Sastri A. R., Beisner B. E. (2015). Evaluation of Functional Trait Diversity for Marine Zooplankton Communities in the Northeast Subarctic Pacific Ocean. J. Plankton Res. 37, 712–726. doi: 10.1093/plankt/fbv045
Prowe A. E. F., Visser A. W., Andersen K. H., Chiba S., Kiørboe T. (2018). Biogeography of Zooplankton Feeding Strategy. Limnol. Oceanogr. 64, 661–678. doi: 10.1002/lno.11067
Putzeys S., Hernández-León S. (2005). A Model of Zooplankton Diel Vertical Migration Off the Canary Islands: Implication for Active Carbon Flux. J. Sea Res. 53, 213–222. doi: 10.1016/j.seares.2004.12.001
Sakinan S., Gücü A. C. (2016). Spatial Distribution of the Black Sea Copepod, Calanus Euxinus, Estimated Using Multifrequency Acoustic Backscatter. ICES J. Mar. Sci. 74 (3), 832–846. doi: 10.1093/icesjms/fsw183
Shimode S., Hiroe Y., Hidaka K., Takahashi K., Tsuda A. (2009). Life History and Ontogenetic Vertical Migration of Neocalanus Gracilis in the Western North Pacific Ocean. Aquat. Biol. 7, 295–306. doi: 10.3354/ab00201
Steinberg D. K., Cope J. S., Wilson S. E., Kobari T. (2008). A Comparison of Mesopelagic Mesozooplankton Community Structure in the Subtropical and Subarctic North Pacific Ocean. Deep Sea Res. Part II 55, 1615–1635. doi: 10.1016/j.dsr2.2008.04.025
Steinberg D. K., Landry M. R. (2017). Zooplankton and the Ocean Carbon Cycle. Annu. Rev. Mar. Sci. 9, 413–444. doi: 10.1146/annurev-marine-010814-015924
Sun D., Zhang D., Zhang R., Wang C. (2019). Different Vertical Distribution of Zooplankton Community Between North Pacific Subtropical Gyre and Western Pacific Warm Pool: Its Implication to Carbon Flux. Acta Oceanol. Sin. 38, 32–45. doi: 10.1007/s13131-018-1237-x
Svensen C., Nejstgaard J. C. (2003). Is Sedimentation of Copepod Fecal Pellets Determined by Cyclopoids? Evidence From Enclosed Ecosystems. J. Plankton Res. 25, 917–926. doi: 10.1093/plankt/25.8.917
Thompson H. (1948). Pelagic Tunicates of Australia (Melbourne: Commonwealth Council for Scientific and Industrial Research).
Tundisi T. M., Tundisi J. G. (1995). Plankton Diversity in a Warm Monomictic Lake (Dom Helvecio, Minas Gerais) and a Polymictic Reservoir (Barra Bonita): A Comparative Analysis of the Intermediate Disturbance Hypothesis. An. Acad. Bras. Cienc. 66, 15–28.
Turner J. T. (2004). The Importance of Small Planktonic Copepods and Their Roles in Pelagic Marine Food Webs. Zool. Stud. 43, 255–266.
Turner J. T. (2015). Zooplankton Fecal Pellets, Marine Snow, Phytodetritus and the Ocean's Biological Pump. Prog. Oceanogr. 130, 205–248. doi: 10.1016/j.pocean.2014.08.005
Uye S., Iwamoto N., Ueda T., Tamaki H., Nakahira K. (1999). Geographical Variations in the Trophic Structure of the Plankton Community Along a Eutrophic-Mesotrophic-Oligotrophic Transect. Fish. Oceanogr. 8, 227–237. doi: 10.1046/j.1365-2419.1999.00110.x
Valencia B., Landry M. R., Décima M., Hannides C. C. S. (2016). Environmental Drivers of Mesozooplankton Biomass Variability in the North Pacific Subtropical Gyre. J. Geophys. Res. Biogeosci. 121, 3131–3143. doi: 10.1002/2016JG003544
Venello T. A., Sastribc A. R., Galbraithb M. D., Dowerca J. F. (2021). Zooplankton Functional Group Responses to Environmental Drivers Off the West Coast of Vancouver Island, Canada. Prog. Oceanogr. 190, 102482. doi: 10.1016/j.pocean.2020.102482
Wang C., Li H., Zhao L., Zhao Y., Dong Y., Zhang W., et al. (2019). Vertical Distribution of Planktonic Ciliates in the Oceanic and Slope Areas of the Western Pacific Ocean. Deep Sea Res. Part II 167, 70–78. doi: 10.1016/j.dsr2.2018.08.002
Wassmann P., Reigstad M., Haug T., Rudels B., Carroll M. L., Hop H., et al. (2006). Food Webs and Carbon Flux in the Barents Sea. Prog. Oceanogr. 71, 232–287. doi: 10.1016/j.pocean.2006.10.003
Williamson C. E., Fischer J. M., Bollens S. M., Overholt E. P., Breckenridge J. K. (2011). Toward a More Comprehensive Theory of Zooplankton Diel Vertical Migration: Integrating Ultraviolet Radiation and Water Transparency Into the Biotic Paradigm. Limnol. Oceanogr. 56, 1603–1623. doi: 10.4319/lo.2011.56.5.1603
Wilson S. E., Steinberg D. K., Buesseler K. O. (2008). Changes in Fecal Pellet Characteristics With Depth as Indicators of Zooplankton Repackaging of Particles in the Mesopelagic Zone of the Subtropical and Subarctic North Pacific Ocean. Deep Sea Res. Part II 55, 1636–1647. doi: 10.1016/j.dsr2.2008.04.019
Xiao Y. (2004). Fauna Sinica, Invertebrata (Vol.38), Chaetognatha: Sagittoidea (Beijing: Science Press).
Xu Z., Huang J., Lin M., Guo D., Wang C. (2014). The Superclass Hydrozoa of the Phylum Cnidaria in China (Beijing: Ocean Press).
Zhang X., Dam H. G. (1997). Downward Export of Carbon by Diel Migrant Mesozooplankton in the Central Equatorial Pacific. Deep Sea Res. PT II 44, 2191–2202. doi: 10.1016/S0967-0645(97)00060-X
Keywords: zooplankton, functional traits, functional group, diel vertical migration, North Pacific Subtropical Gyre
Citation: Ge R, Li Y, Chen H, Lei F, Zhuang Y and Liu G (2022) Diel Vertical Distribution of Mesozooplankton Functional Groups in the North Pacific Subtropical Gyre: A Case Study. Front. Mar. Sci. 9:854642. doi: 10.3389/fmars.2022.854642
Received: 14 January 2022; Accepted: 04 April 2022;
Published: 02 May 2022.
Edited by:
Suzanne Jane Painting, Centre for Environment, Fisheries and Aquaculture Science (CEFAS), United KingdomReviewed by:
Akash R. Sastri, Department of Fisheries and Oceans, CanadaEnrique Nogueira, Spanish Institute of Oceanography (IEO), Spain
Copyright © 2022 Ge, Li, Chen, Lei, Zhuang and Liu. This is an open-access article distributed under the terms of the Creative Commons Attribution License (CC BY). The use, distribution or reproduction in other forums is permitted, provided the original author(s) and the copyright owner(s) are credited and that the original publication in this journal is cited, in accordance with accepted academic practice. No use, distribution or reproduction is permitted which does not comply with these terms.
*Correspondence: Hongju Chen, aG9uZ2p1Y0BvdWMuZWR1LmNu
†These authors have contributed equally to this work and share first authorship