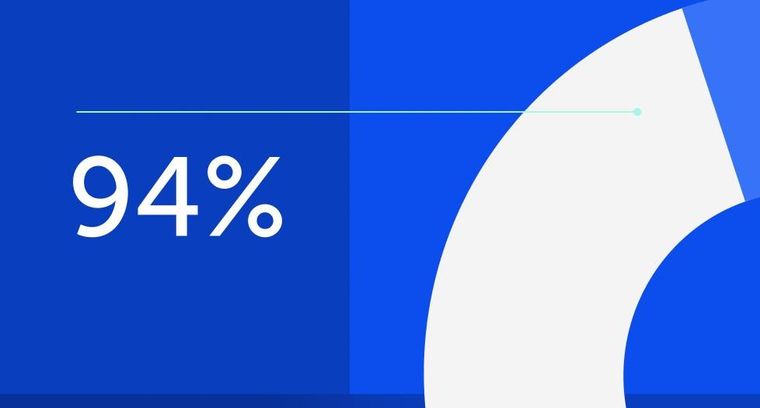
94% of researchers rate our articles as excellent or good
Learn more about the work of our research integrity team to safeguard the quality of each article we publish.
Find out more
ORIGINAL RESEARCH article
Front. Mar. Sci., 18 February 2022
Sec. Marine Molecular Biology and Ecology
Volume 9 - 2022 | https://doi.org/10.3389/fmars.2022.850120
This article is part of the Research TopicMarine Biological Materials: Functional Mechanisms and Environmental Impacts from the Molecular to the Macro-ScaleView all 11 articles
The development of protein anti-degradation strategies is important for storage at ambient conditions, for example in vaccine storage. Despite that it is known that biominerals, typical inorganic-organic composites, can preserve proteins at room temperature for a long time, it is unclear the extent of protein degradation under high temperatures. In this study, we examined remaining proteins in the toasted abalone shell under high temperatures (200 and 300°C) by biomineral proteomics method. Surprisingly, 21 proteins including carbonic anhydrase, hemocyanin, actin can still be identified from shells even after toasting under 300°C, not much decreased compared to that in the 200°C-treated and the native shell. However, the microstructure and composition (both mineral and organic matrix) of shells were altered significantly revealed by scanning electron microscopy, infrared spectroscopy, and X-ray diffraction. The well-preserved proteins may be partially due to the sacrifice of mineral/organic interfaces and the formation of nanopores in the shell at high temperatures. Moreover, the extracted proteins from both groups were able to affect calcium carbonate in vitro, indicating certain remaining bioactivities of proteins. This study has potential implications in various fields such as protein storage at high temperatures and palaeoproteomics.
Proteins are usually prone to be degraded under ambient temperatures let alone high temperatures (Bischof and He, 2006). The development of anti-degradation strategies is important for protein storage at ambient conditions, for example in vaccine storage (Zhang et al., 2012). Chemical modification of proteins such as crosslinking (Collins et al., 1992) and converting to polymers (Wiemann et al., 2018) may help to preserve proteins for a long time but these approaches will change the nature of proteins. Alternatively, proteins can be stabilized by organic macromolecules [e.g., glycerol (Vagenende et al., 2009) and arginine (Baynes et al., 2005)] that results from molecular interaction. In addition, minerals can also offer protection against degradation because of the isolation and stabilization effects (Demarchi et al., 2016).
Biominerals, typical inorganic-organic composite, are minerals formed by organisms (Addadi et al., 2006). In nature, commonly seen biominerals include bone, dental enamel, mollusk shell. Nacre, a typical mollusk shell biomineral, is composed of 95% calcium carbonate and 5% organics (proteins, polysaccharides, and lipids) (Addadi et al., 2006). In particular, proteins are found to be key players in the formation of biominerals and they are occluded in the biominerals (Marin et al., 2007). In recent past two decades, by proteomic methods, dozens of proteins were identified in various mollusk biominerals (Marie et al., 2012; Liao et al., 2015; Liu et al., 2015; Marin, 2020; Liu and Zhang, 2021). Proteins associated with the minerals have high stability (Demarchi et al., 2016). In fact, proteins in bone or dental enamel fossils, which have been preserved for as long as thousands of years or even millions of years (Weiner et al., 1976), are employed to perform phylogenetic analysis (Welker et al., 2015) of humans or to reconstruct people’s life in the past (Hendy et al., 2018; Cappellini et al., 2019; Sakalauskaite et al., 2019; Welker et al., 2019).
Despite that it is known that biominerals can preserve proteins at room temperature for a long time, it is unclear that the extent of protein degradation under high temperature. A recent study found that the shell proteins of the thorny oyster, Spondylus gaederopus can still be identified after heating the shell at 140°C (Sakalauskaite et al., 2021). In this study, to find out if proteins can be extracted and identified from seashells heated at even higher temperatures, we extracted proteins from toasted abalone shells (200, 300°C) and used liquid chromatography tandem-mass spectrometry (LC-MS/MS) to analyze their proteome. Scanning electron microscopy (SEM), fourier transform infrared spectroscopy (FTIR), X-ray diffraction (XRD), and thermogravimetric analysis (TGA) were complimentarily used for the characterization of mineral morphology, mineral composition, mineral crystallography, and thermal behavior, respectively (Figure 1).
Figure 1. The experimental process of toasting abalone shells. Shells were heated at 200 and 300°C for 10 min and native shells as the control. Then the shells were decalcified by EDTA and the remaining proteins were analyzed by LC-MS/MS. Additionally, the microstructure and composition of shells were examined by SEM, FTIR, and XRD.
Native abalone (Haliotis discus hannai) were purchased from local markets from Fuzhou, Fujiang province, China. The shell was obtained by sacrificing the abalone and cleaned with deionized (DI) water. Toasted shells were obtained by toasting the whole abalone shell in an oven at 200°C, 300°C for 10 min and then cleaned with DI water. Untoasted shells were used as the control. Two replicates were performed. It is assumed that the temperature reached up to the same degree in all layers of shells.
The experiment was based on a well-established protocol for the extraction of SMPs in the pearl oyster shell (Liu et al., 2015). Two cleaned toasted abalone shells were immersed in 5% sodium hydroxide for 24 h and were subsequently rinsed in DI water to avoid possible contamination from soft tissues adhering to the shell. The fragments were pulverized (∼50 g) and were decalcified with 50 mL of 0.8 M ethylenediaminetetraacetic acid (EDTA, pH 8.0) for 48 h at 4 °C with continuous agitation. AEBSF (Sangon Biotech, Shanghai, China), an irreversible serine protease inhibitor, was added to prevent protein degradation. For the extraction of EDTA-soluble matrix, the supernatant was collected by centrifugation at 10,000 rpm for 30 min at 4 °C and was then desalted by dialysis and ultrafiltration (cutoff 3 K). For the extraction of EDTA-insoluble matrix, the above insoluble pellet was thoroughly rinsed with water and boiled with a denaturing solution (pH 8.0, 30 mM Tris-HCl, 1% sodium dodecyl sulfate, 10 mM dithiothreitol) for 10 min. The EDTA-insoluble matrix was desalted by dialysis against DI water and then was concentrated by Millipore tubes (cutoff 3 kDa). The samples were applied on 12% precast SDS-polyacrylamide gels (Sangon Biotech, Shanghai, China). The protein concentration was quantified by a BCA assay kit (Pierce).
This process was based on a previously established protocol for the pearl oyster shell (Liu et al., 2015) and was performed in the Biomedical Analysis Center of Tsinghua University. Proteins were stained with Coomassie Blue and the entire protein lane was excised in two pieces, which were completely destained by washing with 50 μL of 50 mM NH4HCO3/acetonitrile (50/50) mixture for 30 min at 37 °C. Reduction was conducted with 50 μL of 10 mM DTT in 50 mM NH4HCO3 for 1 h at 57 °C, followed by alkylation with 50 μL of 100 mM iodoacetamide for 30 min at room temperature in the dark. The cut gels were dried in acetonitrile and then treated with 0.4 μg trypsin (Proteomics Grade, Sigma) in 10 μL of 50 mM NH4HCO3 for 12 h at 37 °C. The suspension was treated with 50 μL of 1% formic acid at 30 °C for 30 min under agitation. The digests were then lyophilized and suspended in 30 μL of 0.1% trifluoroacetic acid and 4% acetonitrile for LC-MS/MS analysis. Five microliter of the samples were injected into the LTQ Orbitrap Velos mass spectrometer with Dionex U-3000 Rapid Separation nano-LC system (Thermo Fisher Scientific) for analysis. MS data were acquired automatically using Analyst QS 1.1 software (Applied Biosystems) following an MS survey scan over m/z 350–1,500 at a resolution of 60,000 for full scan and 2, 000 for MS/MS measurements.
The LC-MS/MS data were searched against the protein database downloaded from Mollusca category in the Uniprot database using a Mascot 2.1 search engine with oxidized methionine and tryptophan as variable modifications. No other post-translation modification has been performed. The peptide MS and MS/MS tolerances were set to 0.5 Da. Finally, sequences with mascot scores of at least 5.0 and with at least two matched peptide fragments were considered valid. Conserved domains of proteins were predicted using SMART.1
Calcium carbonate crystallization experiment was performed by the slow diffusion of CO2 vapor into six cell-culture dishes (Nest, China) from ∼10 g solid ammonium carbonate in a closed desiccator for about 24 h at room temperature. Each well contained 180 μL of 20 mM CaCl2 and 20 μL of EDTA-soluble proteins (free of SDS) with a final concentration of 150 μg mL–1 on a silica cover glass. For the control, no proteins were added. The final samples were rinsed twice with DI water and air-dried before further evaluation.
The shells were fractured and the cross-sections were spurred with gold and then examined by SEM (Hitachi S4800, 5 kV). XRD of powdered shells was recorded on Bruker D8 ADVANCE diffractometer using CuKα radiation (λ = 0.154 nm) in the 2θ range 20–80°. FTIR of powdered shells was recorded at a resolution of 4 cm–1 with 64 scans with attenuated total reflectance (ATR) on a Thermo Scientific Nicolet IN10 instrument. TGA analysis of powdered shells (∼3 mg) was carried out using a TAQ 600 (TA Instruments, Inc., United States) in an air atmosphere with a temperature range from room temperature to 800°C.
Critical raw data of proteomics have been deposited into PRIDE, project accession: PXD030901.
In the native abalone shell, the classical “brick-and-motor” structure composed of stacking nanoscale tablets (thickness, ∼350 nm) was observed by SEM (Figures 2A,B). Macroscopically, the inner shell surfaces were similar after the toasting treatment at 200°C (inset of Figure 2C). By contrast, the inner shell surfaces lost the iridescence after the toasting treatment at 300°C (inset of Figure 2E), indicating microstructural changes of nacre tablets. Microscopically, although the thickness of tablets remained almost unchanged in both conditions, broken pieces of tablets were observed (Figures 2C–F) and nanoscale holes (diameters of ca. 50 nm) were generated (Figures 2D,F) after toasting. In addition, the surfaces of tablets appeared rougher (Figures 2D,F), indicating altered mineral/organic matrices. The nanoscale pores may be due to the collapse of organics inside the minerals after toasting since organics were known to form nanoscale ellipse-shaped forms inside biominerals and synthetic CaCO3 (Li et al., 2011; Rae Cho et al., 2016; Liu et al., 2017). Interestingly, these nanopores were also observed when sea urchin skeleton made of calcium carbonate was treated at high temperatures, in which the authors ascribed the pore formation to the transformation of amorphous calcium carbonate (ACC) to crystalline calcite (Albéric et al., 2018). It is also proposed that relatively thin ACC layers are adjacent to organic/inorganic interfaces, serving as buffer layers between intra-crystalline organics and crystalline calcite (Albéric et al., 2018). Given that ACC has been found in the abalone nacre tablets (Nassif et al., 2005) and other gastropod nacre (Macías-Sánchez et al., 2017), it is possible that ACC in the nacre tablets collapsed and pores formed when temperatures reached up to above 200°C, which might help to dissipate energy from the heat treatment.
Figure 2. Microstructure of the native and toasted abalone shell by SEM. (A) Native abalone shell and its magnification (B); toasted abalone shells at 200°C (C) and 300°C (E) and their magnification (D,F) respectively. Red arrows indicate the nanoscale holes in the nacre tablets. The insets in (A,C,E) are the optical images of shells.
Next, we sought to check whether proteins can be preserved in the toasted shells. In agreement with previous studies, smear protein bands appeared in both the EDTA-soluble and EDTA-insoluble matrix. Interestingly, when shells were toasted at 200°C, protein bands from both matrices appeared in the entire gel lane, indicating the existence of proteins (Figure 3A). When shells were toasted at 300°C, very weak protein bands from both matrices appeared in the entire gel lane (Figure 3A). To analyze the SMP proteome, the entire gels of both matrices were cut and subjected to LC-MS/MS. To our surprise, many proteins can still be identified in both toasted groups, compared to the native shell. 49 and 21 proteins (Table 1 and Supplementary Files) were found at the 200 and 300°C group, respectively, and in the control group, 38 proteins were identified. The three groups of abalone shells (200°C, 300°C, Control) shared 13 proteins, including Hemocyanin, Carbonic anhydrase, Lustrin A, Actin, and others (Figure 3B). In toasted shells at 300°C, there were three unique proteins, including proline-rich, Alpha-amylase, and Beta-catenin (Figure 3B and Table 1). Interestingly, the number of proteins in shells toasted at 200°C increased compared with the control group, which we speculated that it might be caused by the improved destabilization of proteins and/or the disruption of the peptide chains when the temperature increased.
Figure 3. Proteomic analysis of shell matrix proteins from the toasted shell. (A) SDS-PAGE of EDTA-soluble matrix and EDTA-insoluble matrix of toasted shells at 200, 300°C and the native shell (as the control). S: EDTA-soluble matrix; I: EDTA-insoluble matrix. (B) Venn diagram of identified proteins in the three groups by proteomics.
Table 1. Proteomic analysis of shell matrix proteins extracted from toasted abalone shells at 300°C for 10 min.
One of the most abundant proteins was hemocyanin, which contains tyrosinase domains. Tyrosinase was commonly found in shells and was thought to be involved in the cross-linking of organic matrix (Liu and Zhang, 2021). Interestingly, hemocyanin has been found in cuttlebone before (Liu et al., 2021). Another abundant protein found was a secreted carbonic anhydrase (CA), which can converse CO2 into HCO3– and therefore plays an important role in biomineralization (Cardoso et al., 2019). CA or proteins with similar functions have been found in various biominerals such as the skeleton of sponges, corals and shells of pearl oysters, abalones, oysters, clams, etc. (Le Roy et al., 2014). CA is also homologous to nacrein, one of the first identified matrix proteins in the seashell (Miyamoto et al., 1996). Lustrin A was a known shell matrix protein found in the abalone shell, which contained cysteine-rich repeat interspersed by proline-rich domains (Shen et al., 1997). Together with other known SMPs such as LIM protein, Fasciclin domain-containing protein, Proline-rich protein, Thioredoxin domain-containing protein, Alpha-amylase, Alginate lyase 2, they indicated the well-preserved proteins in the shell after 300°C treatment. In addition to the above proteins, others are cellular proteins including actin, tubulin, ubiquitin, histone, transcription factor, and GAPDH. It has been proposed that exosomes or some other vesicles carrying these cellular proteins participated in the shell formation and finally occluded inside the minerals (Song et al., 2019).
Previously, in the native abalone (Haliotis asinine) shell proteome, 51 SMPs were found including Lustrin A, Perlucin, Perlustrin, AP24, Perlwapin, Ependymin related proteins, Kunitz domain-containing protein, glutamine-rich protein (Mann et al., 2018). Partially in agreement with their results, we found 38 proteins in our control. The difference in terms of protein number might be due to the species difference, treatment methods, or instrument sensitivity. In our study, compared with unroasted abalone shells, the number of proteins increased by around 30% at 200°C and decreased by around 45% at 300°C. CA and other proteins might be occluded inside the mineral crystals and therefore can withstand high temperatures. In contrast, other intercrystalline proteins or proteins located between nacreous building blocks (e.g., BPTI/Kunitz domain-containing protein, Calponin) may be susceptible to high temperatures.
To better understand the underlying mineral and matrix change after toasting treatment, materials characterization was conducted. First, TGA was employed to examine the thermal behavior of the native shells (Figure 4A). Particularly, the loss of organics and structural water occurred in samples below 300°C (Figure 4B). The weight percent of organics was around 2 wt.% and 3.7 wt.% if calculated until 300 and 550°C, respectively, close to the amount of organics in biominerals measured by TGA (Albéric et al., 2018). This result seems to indicate that some organics can withstand high temperatures at even above 300°C. At about 600°C, the weight percent dropped dramatically, which is caused by the transformation process of CaCO3 to CaO (Balmain et al., 1999; Figure 4A). Further, to examine the potential compositional change during toasting treatment, XRD (for mineral crystallography) and FTIR (for mineral and organic matrix) were used. XRD showed similar peaks between the three samples (Figure 4C), which had all the characteristic diffraction lines of aragonite according to RRUFF ID R040078.1. In addition, the (104) peak of calcite was observed in all the group including the native shell. This indicates some prismatic layers made of calcite were in the samples. A close examination of (012) peaks at 33.2° of the toasted shell (200°C) indicated the left shift compared to the standard aragonite and native shell, but the (012) peak of toasted shells (300°C) just had a small shift and was close to the standard aragonite (Figure 4D). Previously, it is known that organics inside many biominerals cause the distortion of crystal lattice and heat treatment can eliminate this effect (Pokroy et al., 2004, 2006). The lattice distortion originates from strong atomic interactions at organic/inorganic interfaces (Pokroy et al., 2004). Thereby, it is possible that when proteins degraded with the increase of temperature to 300°C, some of the crystal deformation caused by occluded proteins disappeared. This assumption is supported by the above proteomics data, in which only 40% of proteins were lost. It is not hard to assume that when all proteins are degraded, the crystal lattice distortion caused by occluded proteins will disappear. The XRD results showed overall no change of crystal phase but suggested microscale changes of crystal domains caused by heat treatment. While XRD only reflected mineral phase, FTIR provides information on both the inorganic and organic compositional change between the two samples. Indeed, FTIR showed that the characteristic peaks of 700, 713, 864, 1,090, 1,490 cm–1 from aragonite (Balmain et al., 1999), 3,380 cm–1 from -OH bands of water, and 1,490–1,550 cm–1 from proteins and chitin in the native shell, however, with the increase of toasting temperature, many peaks were diminished. The right (blue) shift of wide bands around 1,490–1,550 cm–1 could be a result of changed interaction among chitin-proteins-CaCO3 composite. After treatment at 200 and 300°C, the peak at 2,520 cm–1 from HCO3– at the mineral/organic interfaces (Balmain et al., 1999) and the peak at 3,500 cm–1 from OH– of water (Balmain et al., 1999) were greatly diminished (Figures 4E,F), indicating the destruction of the mineral/organic interfaces and the loss of structural water, respectively. The destruction of the mineral/organic interfaces supports the observation of nanopores formation by SEM (Figures 2C–F). It is speculated that heat energy was partially dissipated by the sacrifice of mineral/organic interfaces, and thereby protected the occluded proteins from high temperatures.
Figure 4. Compositional analysis of native and toasted abalone shell. (A) TGA analysis of native shells and a close look at room temperature to 300°C (B). XRD patterns (C) and a close exanimation of (012) peaks (D); FTIR spectroscopy (E) and a close examination from wavenumbers at 650–1,300 cm– 1 (F).
To test whether the extracted SMPs still have certain bioactive abilities, the effects of SMPs on the crystallization of calcium carbonate in vitro were examined. In the control, when no proteins were added to the system, typical rhombohedral calcium carbonate crystals were formed (Figures 5A,B). In contrast, when proteins (150 μg/mL) extracted from native shells were added, dumbbell-shaped crystals with diameters of 2–5 μm were formed and small crystal units (diameters of ca. 30 nm) were found on the crystal surfaces (Figures 5C,D). Interestingly, in the 200°C-treated group, no dramatic change of crystal morphology has been observed although the small crystal units on the surfaces appeared to be slightly larger (diameters of ca. 90 nm) than that in the control group (Figures 5E,F). Moreover, in the 300°C-treated group, no dumbbell-shaped crystals but small crystals with diameters of 30–90 nm were formed (Figures 5G,H). It seems that crystal number and nucleation in the 200°C-treated group were similar to that in the native shell group. By contrast, the crystal number was higher in the 300°C-treated group compared to the 200°C-treated group and the native shell group. The crystal phase seems not affected by the proteins (data not shown). This data suggested that compared with untoasted shells, proteins in the 200°C-treated group still affect the crystallization of calcium carbonate in vitro and maintain similar bioactivities with that of control. Proteins in the 300°C-treated group lost certain abilities of control on crystal morphology but still can affect the calcium carbonate formation such as crystal nucleation and growth. This is also in agreement with our proteomic data.
Figure 5. Effects of SMPs of toasted shell on calcium carbonate crystallization. Representative SEM images of calcium carbonate crystals formed at different conditions. (A,B) Control condition without proteins; (C,D) SMPs extracted from native shells; (E,F) SMPs extracted from 200°C-toasted shells; (G,H) SMPs extracted from 300°C-toasted shells. All proteins are in the final concentration of 150 μg/mL.
The indications of this study are in four aspects:
1) Proteins or peptide-based drugs are growing drastically over the past few decades; however, they have low stability and high susceptibility to degradation during processing, storage, and distribution. The establishment and maintenance of a cold temperature environment from manufacturers to patients are therefore required, representing a significant economic burden and sometimes unrealistic in low-developed regions (Moreira et al., 2021). This study shows that proteins can be preserved in toasted seashells, indicating the potential application of protein storage in mineralized materials. In addition, it points out that proteins occluded inside the crystals are possibly more resistant to high temperatures, in agreement with previous concepts that amino acids are well preserved in shells or fossils (Abelson, 1957). Coincident with this idea, the encapsulation of organisms such as viruses, bacteria, yeast, and cells by biomineralized structures has been explored in the past 15 years (Liu et al., 2016; Wang et al., 2018). These studies have shown enhanced stability for preserving the encapsulated organisms (Liu et al., 2016; Wang et al., 2018).
2) Proteins can be preserved for a long time in biominerals such as teeth, bones, and shells. Extracting proteins and analyzing proteome from them (i.e., palaeoproteomics) are increasingly used as a powerful method in archeology and anthropology for the phylogenetic reconstruction of extinct species to the investigation of past human diets and ancient diseases (Hendy et al., 2018). Our study may inform that proteins can be identified in some fossils that are preserved in high-temperature regions, in agreement with previous studies that well-preserved protein sequences were found in ostrich (Struthionidae) eggshell, including from the paleontological sites of Laetoli (3.8 Ma) and Olduvai Gorge (1.3 Ma) in Tanzania (Demarchi et al., 2016).
3) Although shells of Mollusca are not commonly edible, this study indicated that the use of heated/cooked seashells as a food source may have some biomedical effects since the remaining proteins are inside the minerals. In fact, adding heated oyster shells to the diet of elderly patients appears to increase the bone quality (Fujita et al., 1996).
4) The temperature on Earth’s surface ranges from -98.6 to 495°C (Merino et al., 2019) and the upper temperature limit for life is 130°C (microorganism Geogemma barossii 121) (Kashefi and Lovley, 2003). Current environmental and theoretical studies suggest that the upper limit of life might lay near 150°C, due primarily to the instability of macromolecules above this temperature (Merino et al., 2019). This study raises a hypothesis that proteins may sustain even higher temperatures when in certain local environment such as minerals. Further experiment is needed to test this hypothesis.
At last, several interesting questions remain to be explored. Are the proteins such as enzymes extracted from the biominerals still be active to catalyze reactions after the heat treatment? How extreme is the extent of proteins can be preserved in biominerals? Can this preservation effect be applied to other biominerals such as bone and teeth? What is the mechanism of protein protection in biominerals?
Although toasted abalone shells (200°C, 300°C for 10 min) have altered microstructure and composition in the nacre tablets, many proteins can still be extracted and identified from shells and maintain certain bioactivities. Nearly 60% of proteins (e.g., hemocyanin, carbonic anhydrase, Lustrin A, actin, tubulin) can still be identified from shells after 300°C treatment revealed by proteomics analysis. The well-preserved proteins may be due to the incorporation of proteins inside the crystal lattices of nacre tablets. This study has some implications in several aspects such as protein storage at high temperatures, palaeoproteomics in archeology, and food nutrients.
The datasets presented in this study can be found in online repositories. The names of the repository/repositories and accession number(s) can be found below: ProteomeXchange (accession: PXD030901).
CL conceived the project. XJ and CL performed the experiments. CL and JH analyzed the data and wrote the manuscript. All authors revised the manuscript.
The authors declare that the research was conducted in the absence of any commercial or financial relationships that could be construed as a potential conflict of interest.
All claims expressed in this article are solely those of the authors and do not necessarily represent those of their affiliated organizations, or those of the publisher, the editors and the reviewers. Any product that may be evaluated in this article, or claim that may be made by its manufacturer, is not guaranteed or endorsed by the publisher.
CL gratefully acknowledges the support of Natural Science Foundation of Jiangsu Province BK20210363, the Fundamental Research Funds for the Central Universities B200201065, and the Jiangsu Innovation Talent Program JSSCBS20210250. JH gratefully acknowledges the National Natural Science Foundation of China Grant 42106091.
We thank Dingfei Yan and Haiteng Deng in Center of Protein Analysis Technology, Tsinghua University, for MS analysis.
The Supplementary Material for this article can be found online at: https://www.frontiersin.org/articles/10.3389/fmars.2022.850120/full#supplementary-material
Abelson, P. H. (1957). Some aspects of paleobiochemistry. Ann. N.Y. Acad. Sci. 69, 276–285. doi: 10.1111/j.1749-6632.1957.tb49663.x
Addadi, L., Joester, D., Nudelman, F., and Weiner, S. (2006). Mollusk shell formation: a source of new concepts for understanding biomineralization processes. Chem. Eur. J. 12, 980–987. doi: 10.1002/chem.200500980
Albéric, M., Caspi, E. N., Bennet, M., Ajili, W., Nassif, N., Azaïs, T., et al. (2018). Interplay between calcite, amorphous calcium carbonate, and intracrystalline organics in sea urchin skeletal elements. Cryst. Grow. Des. 18, 2189–2201. doi: 10.1021/acs.cgd.7b01622
Balmain, J., Hannoyer, B., and Lopez, E. (1999). Fourier transform infrared spectroscopy (FTIR) and X-ray diffraction analyses of mineral and organic matrix during heating of mother of pearl (nacre) from the shell of the mollusc Pinctada maxima. J. Biomed. Mater. Res. 48, 749–754. doi: 10.1002/(sici)1097-4636(1999)48:5<749::aid-jbm22gt;3.0.co;2-p
Baynes, B. M., Wang, D. I. C., and Trout, B. L. (2005). Role of arginine in the stabilization of proteins against aggregation. Biochemistry 44, 4919–4925. doi: 10.1021/bi047528r
Cappellini, E., Welker, F., Pandolfi, L., Ramos-Madrigal, J., Samodova, D., Ruther, P. L., et al. (2019). Early Pleistocene enamel proteome from Dmanisi resolves Stephanorhinus phylogeny. Nature 574, 103–107. doi: 10.1038/s41586-019-1555-y
Cardoso, J. C. R., Ferreira, V., Zhang, X., Anjos, L., Félix, R. C., Batista, F. M., et al. (2019). Evolution and diversity of alpha-carbonic anhydrases in the mantle of the Mediterranean mussel (Mytilus galloprovincialis). Sci. Rep. 9:10400. doi: 10.1038/s41598-019-46913-2
Collins, M. J., Westbroek, P., Muyzer, G., and De Leeuw, J. W. (1992). Experimental evidence for condensation reactions between sugars and proteins in carbonate skeletons. Geochim. Cosmochim. Acta 56, 1539–1544. doi: 10.1016/0016-7037(92)90223-6
Demarchi, B., Hall, S., Roncal-Herrero, T., Freeman, C. L., Woolley, J., Crisp, M. K., et al. (2016). Protein sequences bound to mineral surfaces persist into deep time. eLife 5:e17092. doi: 10.7554/eLife.17092
Fujita, T., Ohue, T., Fujii, Y., Miyauchi, A., and Takagi, Y. (1996). Heated oyster shell-seaweed calcium (AAA Ca) on osteoporosis. Calcified Tissue Int. 58, 226–230. doi: 10.1007/BF02508640
Hendy, J., Welker, F., Demarchi, B., Speller, C., Warinner, C., and Collins, M. J. (2018). A guide to ancient protein studies. Nat. Ecol. Evol. 2, 791–799.
Kashefi, K., and Lovley, D. R. (2003). Extending the upper temperature limit for life. Science 301, 934–934. doi: 10.1126/science.1086823
Le Roy, N., Jackson, D. J., Marie, B., Ramos-Silva, P., and Marin, F. (2014). The evolution of metazoan α-carbonic anhydrases and their roles in calcium carbonate biomineralization. Front. Zool. 11:75. doi: 10.1186/s12983-014-0075-8
Li, H., Xin, H. L., Kunitake, M. E., Keene, E. C., Muller, D. A., and Estroff, L. A. (2011). Calcite prisms from mollusk shells (Atrina Rigida): swiss-cheese-like organic–inorganic single-crystal composites. Adv. Funct. Mater. 21, 2028–2034.
Liao, Z., Bao, L.-F., Fan, M.-H., Gao, P., Wang, X.-X., Qin, C.-L., et al. (2015). In-depth proteomic analysis of nacre, prism, and myostracum of Mytilus shell. J. Proteomics 122, 26–40. doi: 10.1016/j.jprot.2015.03.027
Liu, C., and Zhang, R. (2021). Biomineral proteomics: a tool for multiple disciplinary studies. J. Proteomics 238:104171. doi: 10.1016/j.jprot.2021.104171
Liu, C., Du, J., Xie, L., and Zhang, R. (2017). Direct observation of nacre proteins in the whole calcite by super-resolution microscopy reveals diverse occlusion patterns. Cryst. Grow Des. 17, 1966–1976.
Liu, C., Ji, X., Huang, J., Wang, Z., Liu, Y., and Hincke, M. T. (2021). Proteomics of shell matrix proteins from the cuttlefish bone reveals unique evolution for cephalopod biomineralization. ACS Biomater. Sci. Eng. Online ahead of print, doi: 10.1021/acsbiomaterials.1c00693
Liu, C., Li, S., Kong, J., Liu, Y., Wang, T., Xie, L., et al. (2015). In-depth proteomic analysis of shell matrix proteins of Pinctada fucata. Sci. Rep. 5:17269. doi: 10.1038/srep17269
Liu, Z., Xu, X., and Tang, R. (2016). Improvement of biological organisms using functional material shells. Adv. Funct. Mater. 26, 1862–1880.
Macías-Sánchez, E., Willinger, M. G., Pina, C. M., and Checa, A. G. (2017). Transformation of ACC into aragonite and the origin of the nanogranular structure of nacre. Sci. Rep. 7:12728. doi: 10.1038/s41598-017-12673-0
Mann, K., Cerveau, N., Gummich, M., Fritz, M., Mann, M., and Jackson, D. J. (2018). In-depth proteomic analyses of Haliotis laevigata (greenlip abalone) nacre and prismatic organic shell matrix. Proteome Sci. 16:11. doi: 10.1186/s12953-018-0139-3
Marie, B., Joubert, C., Tayalé, A., Zanella-Cléon, I., Belliard, C., Piquemal, D., et al. (2012). Different secretory repertoires control the biomineralization processes of prism and nacre deposition of the pearl oyster shell. Proc. Natl. Acad. Sci. U.S.A. 109, 20986–20991. doi: 10.1073/pnas.1210552109
Marin, F. (2020). Mollusc shellomes: past, present and future. J. Struct. Biol. 212:107583. doi: 10.1016/j.jsb.2020.107583
Marin, F., Luquet, G., Marie, B., and Medakovic, D. (2007). Molluscan shell proteins: primary structure, origin, and evolution. Curr. Top. Dev. Biol. 80, 209–276. doi: 10.1016/S0070-2153(07)80006-8
Merino, N., Aronson, H. S., Bojanova, D. P., Feyhl-Buska, J., Wong, M. L., Zhang, S., et al. (2019). Living at the extremes: extremophiles and the limits of life in a planetary context. Front. Microbiol. 10:780. doi: 10.3389/fmicb.2019.00780
Miyamoto, H., Miyashita, T., Okushima, M., Nakano, S., Morita, T., and Matsushiro, A. (1996). A carbonic anhydrase from the nacreous layer in oyster pearls. Proc. Natl. Acad. Sci. U.S.A. 93, 9657–9660. doi: 10.1073/pnas.93.18.9657
Moreira, A., Lawson, D., Onyekuru, L., Dziemidowicz, K., Angkawinitwong, U., Costa, P. F., et al. (2021). Protein encapsulation by electrospinning and electrospraying. J. Controlled Release 329, 1172–1197. doi: 10.1016/j.jconrel.2020.10.046
Nassif, N., Pinna, N., Gehrke, N., Antonietti, M., Jäger, C., and Cölfen, H. (2005). Amorphous layer around aragonite platelets in nacre. Proc. Natl. Acad. Sci. U.S.A. 102, 12653–12655. doi: 10.1073/pnas.0502577102
Pokroy, B., Fitch, A. N., Marin, F., Kapon, M., Adir, N., and Zolotoyabko, E. (2006). Anisotropic lattice distortions in biogenic calcite induced by intra-crystalline organic molecules. J. Struct. Biol. 155, 96–103. doi: 10.1016/j.jsb.2006.03.008
Pokroy, B., Quintana, J. P., Caspi, E. A. N., Berner, A., and Zolotoyabko, E. (2004). Anisotropic lattice distortions in biogenic aragonite. Nat. Mater. 3, 900–902. doi: 10.1038/nmat1263
Rae Cho, K., Kim, Y.-Y., Yang, P., Cai, W., Pan, H., Kulak, A. N., et al. (2016). Direct observation of mineral–organic composite formation reveals occlusion mechanism. Nat. Commun. 7:10187. doi: 10.1038/ncomms10187
Sakalauskaite, J., Andersen, S. H., Biagi, P., Borrello, M. A., Cocquerez, T., Colonese, A. C., et al. (2019). ‘Palaeoshellomics’ reveals the use of freshwater mother-of-pearl in prehistory. eLife 8:e45644. doi: 10.7554/eLife.45644
Sakalauskaite, J., Mackie, M., Taurozzi, A. J., Collins, M. J., Marin, F., and Demarchi, B. (2021). The degradation of intracrystalline mollusc shell proteins: a proteomics study of Spondylus gaederopus. BBA Proteins Proteom. 1869:140718. doi: 10.1016/j.bbapap.2021.140718
Shen, X., Belcher, A. M., Hansma, P. K., Stucky, G. D., and Morse, D. E. (1997). Molecular cloning and characterization of Lustrin A, a matrix protein from shell and pearl nacre of Haliotis rufescens. J. Biol. Chem. 272, 32472–32481. doi: 10.1074/jbc.272.51.32472
Song, X., Liu, Z., Wang, L., and Song, L. (2019). Recent advances of shell matrix proteins and cellular orchestration in marine molluscan shell biomineralization. Front. Mar. Sci. 6:41. doi: 10.3389/fmars.2019.00041
Vagenende, V., Yap, M. G. S., and Trout, B. L. (2009). Mechanisms of protein stabilization and prevention of protein aggregation by glycerol. Biochemistry 48, 11084–11096. doi: 10.1021/bi900649t
Wang, X., Xiao, Y., Hao, H., Zhang, Y., Xu, X., and Tang, R. (2018). Therapeutic potential of biomineralization-based engineering. Adv. Ther. 1:1800079.
Weiner, S., Lowenstam, H. A., and Hood, L. (1976). Characterization of 80-million-year-old mollusk shell proteins. Proc. Natl. Acad. Sci. U.S.A. 73, 2541–2545. doi: 10.1073/pnas.73.8.2541
Welker, F., Collins, M. J., Thomas, J. A., Wadsley, M., Brace, S., Cappellini, E., et al. (2015). Ancient proteins resolve the evolutionary history of Darwin’s South American ungulates. Nature 522, 81–84. doi: 10.1038/nature14249
Welker, F., Ramos-Madrigal, J., Kuhlwilm, M., Liao, W., Gutenbrunner, P., De Manuel, M., et al. (2019). Enamel proteome shows that Gigantopithecus was an early diverging pongine. Nature 576, 262–265. doi: 10.1038/s41586-019-1728-8
Wiemann, J., Fabbri, M., Yang, T.-R., Stein, K., Sander, P. M., Norell, M. A., et al. (2018). Fossilization transforms vertebrate hard tissue proteins into N-heterocyclic polymers. Nat. Commun. 9:4741. doi: 10.1038/s41467-018-07013-3
Keywords: biomineralization, proteomics, proteins-degradation, shells (structures), materials characterisation
Citation: Ji X, Huang J, Wang Z, Xu Z and Liu C (2022) Proteins Are Well-Preserved in Shells Toasted at 300°C Revealed by Proteomics. Front. Mar. Sci. 9:850120. doi: 10.3389/fmars.2022.850120
Received: 07 January 2022; Accepted: 28 January 2022;
Published: 18 February 2022.
Edited by:
Shiguo Li, Research Center for Eco-Environmental Sciences, Chinese Academy of Sciences (CAS), ChinaReviewed by:
Zhi Liao, Zhejiang Ocean University, ChinaCopyright © 2022 Ji, Huang, Wang, Xu and Liu. This is an open-access article distributed under the terms of the Creative Commons Attribution License (CC BY). The use, distribution or reproduction in other forums is permitted, provided the original author(s) and the copyright owner(s) are credited and that the original publication in this journal is cited, in accordance with accepted academic practice. No use, distribution or reproduction is permitted which does not comply with these terms.
*Correspondence: Chuang Liu, Y2h1YW5nbGl1MjAyMEBoaHUuZWR1LmNu
Disclaimer: All claims expressed in this article are solely those of the authors and do not necessarily represent those of their affiliated organizations, or those of the publisher, the editors and the reviewers. Any product that may be evaluated in this article or claim that may be made by its manufacturer is not guaranteed or endorsed by the publisher.
Research integrity at Frontiers
Learn more about the work of our research integrity team to safeguard the quality of each article we publish.