- 1Department of Oceanography, Dalhousie University, Halifax, NS, Canada
- 2Department of Earth Sciences, Memorial University of Newfoundland, St. John’s, NL, Canada
There has been increasing interest in mining polymetallic sulfide deposits at deep-sea inactive hydrothermal vents, leading to the development of regulations to minimize risk to the marine environment. While an extensive body of literature exists on the ecological communities at active vents, fauna at inactive hydrothermal vents and the vent periphery are poorly described and their vulnerability to disturbance is unknown. We examined patterns in abundance of non-vent epibenthic megafauna on two segments of the Juan de Fuca Ridge, Northeast Pacific Ocean. Video footage was collected by the remotely operated vehicle ROPOS during four dives at the Endeavour Segment and two dives at Middle Valley in August 2016. At the Endeavour Segment, the substrate is characterized predominantly by basalt and edifices of hydrothermal sulfide that range in hydrothermal activity from inactive to vigorous, high-temperature venting. In contrast, Middle Valley is heavily sedimented and most hydrothermal activity is low-temperature diffuse flow. While inactive substrates at both sites harboured slow-growing sessile fauna, the dominant members of the community differed between sites. At Endeavour, the most abundant morphotaxa included rossellid vase sponges, alcyonacean corals, and crinoids. Estimated richness and total abundance of morphotaxa was higher on hard substrates than sedimented substrates and highest on inactive chimneys. At Middle Valley, the most abundant morphotaxa included antipatharian corals, anemones, and ascidians. Species richness was higher on inactive chimneys and mixed substrates than sediment. The abundance of some megafauna varied with proximity to active vents. At Endeavour, deep-water corals were nearly absent within 25 m of active chimneys and very few occurred between 26 and 50 m from active chimneys. Rossellid vase sponges were in low abundance within 25 m of active chimneys but were more abundant than corals at 26-50 m from active chimneys. This project contributes baseline data on megafaunal assemblages on inactive hydrothermal vents and can provide the basis for more focused research on the structure and function of inactive vent ecosystems.
Introduction
At deep-sea hydrothermal vents, hot, metal-rich, fluid is discharged into the ocean from beneath the seafloor. Upon mixing with ambient seawater, sulfide and sulfate minerals precipitate out of the hydrothermal fluid and accumulate on the seabed (Haymon, 1983). Hydrothermal vents occur along seafloor tectonic boundaries such as mid-ocean ridges, volcanic arcs, and back arc basins, where shallow magmatic heat sources drive convective circulation of seawater through oceanic crust (Hannington et al., 2005). These polymetallic sulfide-rich deposits accumulate over the lifetime of the active vent, forming chimney structures that can be 10s of meters tall and mounds with diameters of up to several hundred meters (Hannington et al., 2010). Vents typically cluster to form vent fields consisting of several chimneys and/or mounds spread over 1,000s of square meters. Vents, and vent fields, have a finite lifetime that, depending on the tectonic setting, can be up to hundreds of thousands of years, but will eventually become inactive (Cherkashov et al., 2017; Jamieson and Gartman, 2020). However, even within an active vent field, the locations of venting can change over time, and active vent fields typically contain a mixture of active and inactive chimneys and/or mounds. Once vent chimneys become inactive, they can persist as upright structures, but will weather over time and collapse into sulfide rubble or boulders on the seafloor.
Active hydrothermal vents support highly productive and dense communities of endemic species that rely on symbiotic chemoautotrophic microbes and fluid flow (Tunnicliffe et al., 1998; Van Dover, 2000). Community succession and dynamics on active hydrothermal vents is primarily driven by changes in fluid flow and temperature (Sarrazin et al., 1997; Marcus et al., 2009; Sen et al., 2014). When fluid flow wanes and eventually ceases, many vent-endemic species, particularly those with symbiont requirements, such as tubeworms can no longer survive, and non-vent fauna can colonize the deposits and nearby area. The high temperature, low oxygen, high sulfide and high metal fluid conditions at active hydrothermal vents are thought to be toxic to non-vent taxa which will not colonize a vent site until fluid flow has subsided (Van Dover, 2000).
The sphere of influence of the hydrothermal conditions beyond their immediate area of occurrence is currently poorly understood (Levin et al., 2016). ‘Peripheral’ areas to hydrothermal vents fall outside the direct impact of active hydrothermal fluid and are thought to be a transition zone between the active environments and the rest of the deep sea (Arquit, 1990; Sen et al., 2016). Closer to active vents, faunal communities comprise both vent associated and non-vent fauna, but non-vent taxa dominate at increasing distances (Arquit, 1990). Non-vent communities in the peripheral zone are higher in biomass and density than the surrounding deep sea, likely benefitting from increased primary productivity closer to vents (Arquit, 1990; Galkin, 1997). Non-vent fauna include species that are found on substrates throughout the deep sea and utilize nutritional resources derived from the photosynthetic food chain.
While an extensive body of literature exists on the ecological communities associated with active vents (ex. Tunnicliffe, 1991; Tunnicliffe et al., 1998; Van Dover, 2000; Mullineaux et al., 2018; Dasgupta et al., 2021), fauna at inactive hydrothermal vents are poorly described (Van Dover et al., 2020). Generally, inactive vents support communities of non-vent ‘background fauna’ that occur on hard substrates throughout the deep sea (Galkin, 1997; Erickson et al., 2009; Collins et al., 2012; Sen et al., 2014; Boschen et al., 2015; Boschen et al., 2016; Gerdes et al., 2019; Van Dover, 2019), but it is possible that inactive vents harbor unique species assemblages adapted to the conditions of weathering sulfide minerals (Van Dover, 2011; Van Dover et al., 2020). In the western South Pacific, these non-vent faunal communities associated with inactive vents include large gorgonian corals, actiniarian anemones, hydroids, brisingid sea stars, carnivorous sponges, and stalked barnacles (Galkin, 1997; Erickson et al., 2009; Collins et al., 2012). In addition to these taxa, assemblages in the South Pacific and Indian Oceans also include comatulid crinoids, hexactinellid sponges, ascidians, and brachiopods (Boschen et al., 2015; Boschen et al., 2016; Gerdes et al., 2019). These assemblages on inactive deposits are unique compared to assemblages on both active vents (Boschen et al., 2015; Boschen et al., 2016) and adjacent hard substrates (Gerdes et al., 2019). Large corals, possibly up to 200 years old, are a dominant member of inactive vent communities (Boschen et al., 2016), and aggregate on the tallest and most exposed surfaces of inactive chimneys (Galkin, 1997). While some inactive chimneys are densely populated, others are barren, indicating that colonization may be impacted by factors such as the proximity to active vents or ocean currents (Boschen et al., 2016).
In the Northeast Pacific, faunal communities on the vent periphery closely resemble typical benthic non-vent fauna in the surrounding area. On Cobb Seamount, away from active hydrothermal vents, deep-sea (up to 1150 m) fauna included actiniarian anemones, Alcyonacea and Antipatharia corals, holothurians, crabs, and glass sponges (Rossellidae) (Du Preez et al., 2016). On the periphery of active vents on the Juan de Fuca Ridge, sponges, crabs, sea stars, and holothurians were common (Arquit, 1990; Milligan and Tunnicliffe, 1994). On the Cleft segment, the most abundant groups included alcyonacean corals and sea pens, crinoids, and holothurians (Milligan and Tunnicliffe, 1994). On the vent periphery on Gorda Ridge in the Northeast Pacific, filter feeders such as crinoids and deep-water corals dominated hard substrate, while deposit feeders and scavengers such as asteroids and holothurians were more abundant on sediment (Carey et al., 1990).
There is increasing interest in mining polymetallic sulfide deposits that form at hydrothermal vent fields due to their potential high concentrations of metals such as copper, zinc, gold and silver (Petersen et al., 2016). Hydrothermally active polymetallic sulfide deposits are detected by chemical and physical anomalies in the seawater caused by the plumes of black smokers. However, active deposits are considered ecologically vulnerable and their protection from mining activities has been proposed (Van Dover et al., 2018). Hydrothermally inactive sulfide deposits may provide an alternative target for mining, but they are much more difficult to locate as they do not have an associated hydrothermal plume. In addition to the impacts on communities inhabiting inactive deposits themselves, inactive deposits and active vents often cluster together (Clague et al., 2020), thus mining inactive deposits could still harm nearby active vent ecosystems (Jamieson and Gartman, 2020).
No commercial mining is currently being undertaken in the deep sea, but Japan conducted a testing of mining equipment on an inactive hydrothermal mound off the coast of Okinawa in 2017 (Okamoto et al., 2018). Before commercial mining starts, there is a critical need for baseline ecological studies on inactive vents to fully understand how faunal communities will be impacted by mining sulfide deposits on the seafloor (Van Dover et al., 2020). Further, better data are required to inform a mining code that is currently in development by the International Seabed Authority (ISA) (Earth Negotiations Bulletin, 2020).
The Endeavour and Middle Valley segments form part of the Juan de Fuca Ridge, which is spreading at an intermediate full rate of 6 cm yr-1 (full rate; Riddihough, 1984). The segments host two geologically distinct hydrothermal systems, with differences in associated substrate and style of venting. The Endeavour vent field has five main hydrothermally active vent clusters, spaced ~2 km apart along the unsedimented basaltic floor of a 0.5 -1 km wide axial valley (Kelley et al., 2012). A wide range of hydrothermal venting conditions occur within this vent field, from tall (> 20 m), high-temperature active chimneys to areas of low-temperature diffuse flow. Abundant (> 500) inactive chimneys and mounds occur between the active vent clusters, indicating a dynamic evolution of hydrothermal venting over the ~6,000 years of recorded hydrothermal activity along the axial valley (Jamieson et al., 2013; Clague et al., 2020). The vent field, located in Canada’s exclusive economic zone, is a designated Marine Protected Area (MPA). The Middle Valley vent field is located 60 km northeast of Endeavour. A key difference between Middle Valley and Endeavour is the nearly 500 m of continental-derived turbidite sediments that have accumulated along Middle Valley, compared to the exposed basalt at Endeavour (Delaney et al., 1992; Juniper et al., 1992). There are two known locations of active venting at Middle Valley: Area of Active Venting (AAV) and Bent Hill (Ames et al., 1993). Unlike Endeavour, there is no evidence for abundant past (i.e. now inactive) venting outside of the active venting areas. Hydrothermal activity is primarily characterized by low-temperature diffuse flow, although there are a few focused, higher-temperature active chimneys in the area.
In this study, we quantified the type, abundance, and distribution of non-vent epibenthic megafaunal assemblages at the Endeavour and Middle Valley vent fields using data collected by the remotely operated vehicle ROPOS in August 2016. We described the distribution of select taxa relative to active venting using patterns in faunal abundance along transects that extended from active chimneys to inactive sulfide deposits and peripheral hard substrate. We expected that non-vent taxa would include deep-water corals, crinoids, sponges, and anemones at both Endeavour and Middle Valley, but also expected differences in the specific composition and abundance of these faunal communities between the two locations because of the differences in substrate and style of venting. Additionally, we predicted that the abundance of non-vent fauna would increase with distance from active hydrothermal flow. This study will contribute to a better understanding of the ecology at inactive vent sites on two types of hydrothermal systems, ultimately supporting the development of conservation and resource management plans for polymetallic sulfide deposits.
Materials and Methods
Data Collection
Video footage was collected with the remotely operated vehicle (ROV) ROPOS aboard the CCGS John P. Tully during four dives at the Endeavour segment (R1938, R1939, R1940 and R1941) and two dives at Middle Valley (R1942 and R1943), between 7 and 13 August 2016 (Figure 1). At Endeavour, dives R1938 and R1941 traversed along the axial valley floor near the active Sasquatch and High Rise vent clusters, while dives R1939 and R1940 started at the valley floor near Main Endeavour, and traversed up the eastern and western axial valley walls, respectively. At Middle Valley, dives R1942 and R1943 traversed Bent Hill and the Dead Dog vent field (located in the AAV), respectively. Individual dive tracks ranged in length between 2814 and 4646 m at the Endeavour vent field, and between 3180 and 3751 m at Middle Valley (Table 1).
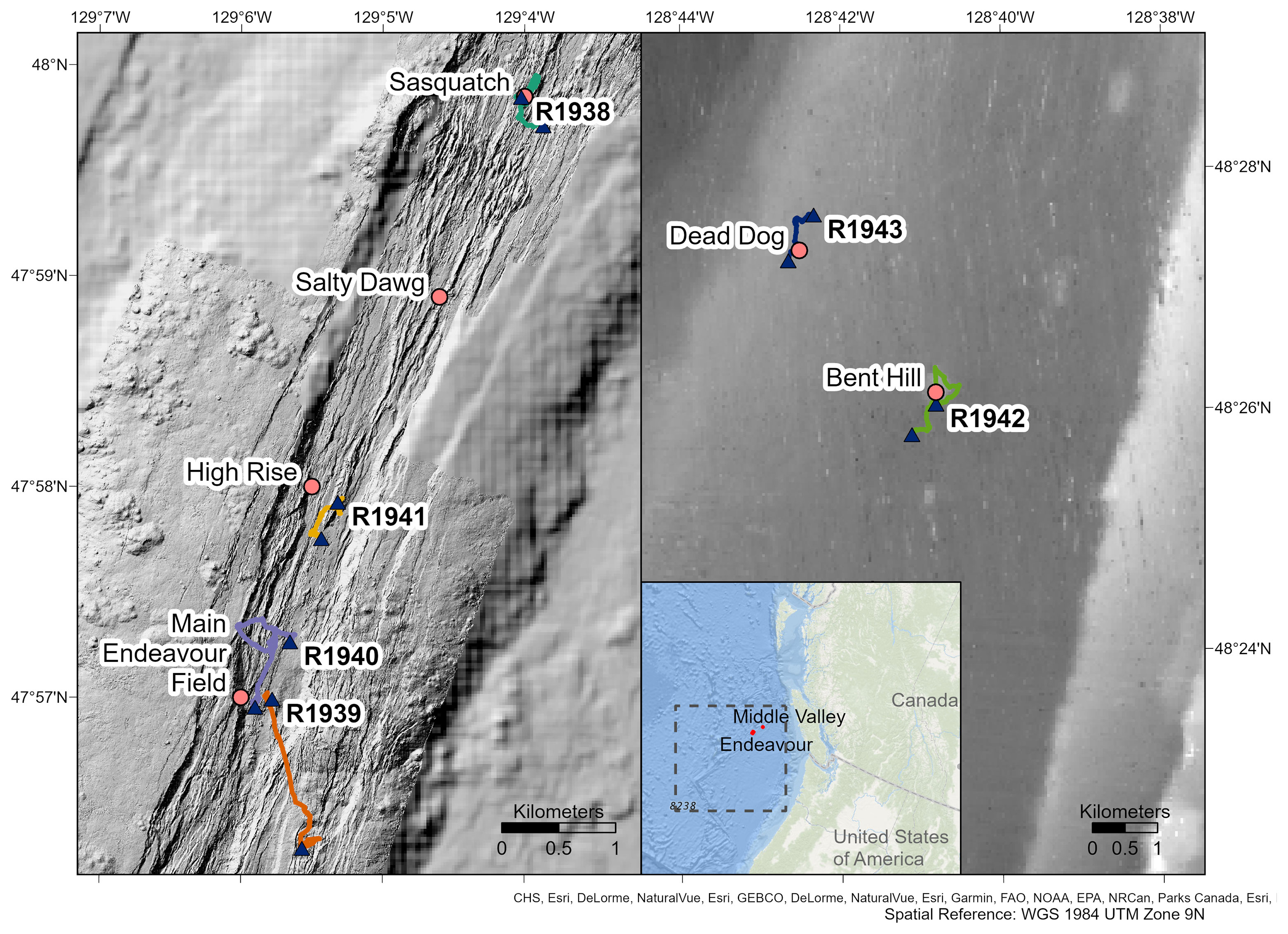
Figure 1 Track lines of dives R1938-R1943 conducted by the remotely operated vehicle ROPOS on the Endeavour (left) and Middle Valley (right) segments of the Juan de Fuca Ridge. Triangles represent the start and end points of dives. Known vent fields are shown. The general location of the Juan de Fuca Ridge (dashed box) and position of Endeavour and Middle Valley are shown in the inset map. Maps created in ArcGIS Pro, bathymetry and vent locations provided by ONC, MBARI, and the University of Washington.
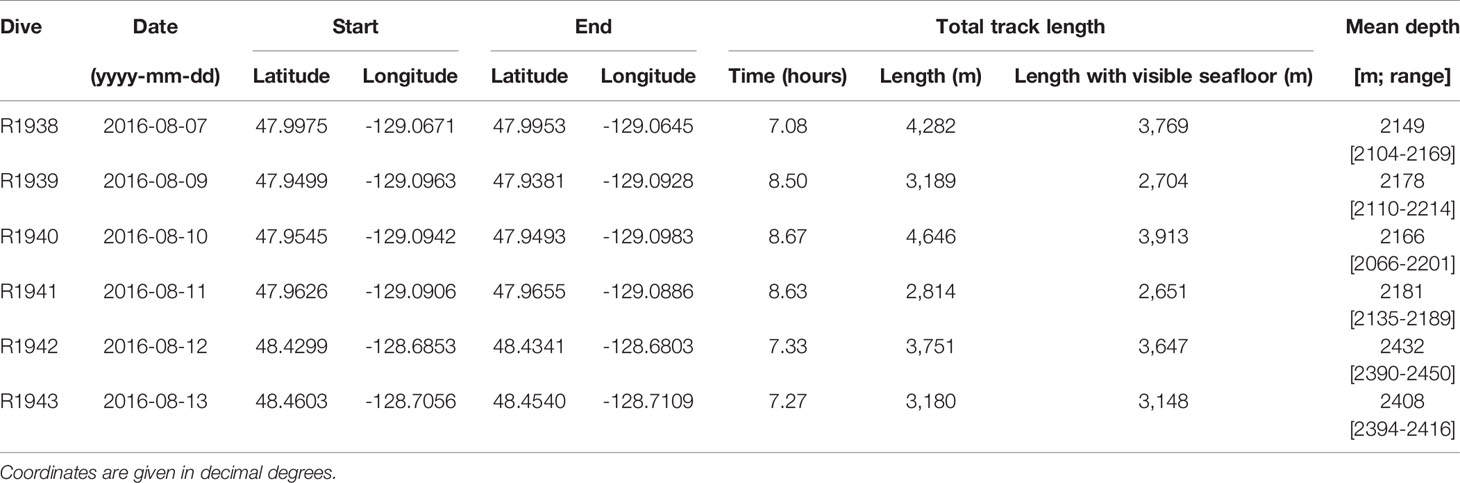
Table 1 Characteristics of dives performed by the remotely operated vehicle ROPOS at the Endeavour vent field (R1938-R1941) and Middle Valley (R1942 and R1943) on the Juan de Fuca Ridge.
ROPOS is equipped with two HD (1,920 × 1,080 pixels) video cameras: a forward-facing Insite Pacific Zeus-Plus with pan, tilt, and zoom capabilities and a downward-facing Insite Pacific Mini-Zeus with tilt only. Both cameras were equipped with a set of parallel scaling lasers that were spaced 10 cm apart into the field of view. Metadata associated with the dives included date, time, geographical coordinates, depth, heading, and altitude from the seafloor of ROPOS. An IXSEA GAPS ultra-short baseline system, combined with a Sonardyne SYRINX Doppler velocity log and an IXSEA OCTANS III survey-grade gyrocompass using the Canadian Scientific Submersible Facility’s LOKI Kalman filter were used for vehicle positioning at the seafloor, providing absolute positioning at better than 5-m accuracy.
Imagery Analysis
Two different analytical approaches were used to estimate the abundance of non-vent morphotaxa (typical vent taxa were excluded from the analysis). The occurrence of large-sized megafauna of relatively low abundance, such as deep-water alcyonacean and black corals and rossellid vase sponges (Rossellidae), were recorded using the forward-facing Zeus video. The video was annotated during playback using the Ocean Floor Observation Protocol software (OFOP 3.3.8c, Huetten and Greinert, 2008; Scientific Abyss Mapping Services, 2009). OFOP synchronizes the video file with the location data recorded during the dive, georeferencing all observations along the dive track. During playback, all visible megafauna greater than ~ 5 cm in the largest dimension (identified to the highest taxonomic resolution) were annotated, along with the substrate type to which they were attached and the location of every active and inactive chimney over the entire dive track. We ensured that duplicate recordings of the same individuals were avoided.
For all other morphotaxa, abundance was measured using images that were extracted from the downward-facing Mini Zeus video at approximately 20-m intervals using the OFOP software (Supplementary Figure 1). Suitable images were selected based on several criteria: (1) the area of the image did not exceed 20 m2; (2) the substrate was perpendicular to the camera angle (± ~ 10˚); (3) the scaling lasers were visible and on the same plane as the substrate; and (4) the seafloor was clearly visible. Images were cropped to include only the extent of seafloor that was visible as a single horizontal plane and to exclude any areas obstructed by fish, the ROPOS vehicle, lens distortions, or suspended sediment. Cropped images were not included in the analysis if the area after cropping was < 1 m2. If an image was deemed unsuitable, the next available image was used. If the distance between suitable images exceeded ~ 50 m, images were captured manually in OFOP to maintain the ~ 20-m spacing where possible. However, there were large segments of the dives where there were no suitable images because the ROV was travelling too far above the seafloor. To capture data from the vertical walls of inactive chimneys, images were extracted in a similar fashion but from the forward-facing Zeus footage because it was perpendicular to the substrate. All suitable images were imported into ImageJ, an image processing and analysis software, and the 2D area of the image was calculated using the scaling lasers. All visible megafauna > 2 cm were enumerated and identified to the highest taxonomic resolution. Additionally, the substrate in each image was classified by measuring the percent cover of each of 4 substrate types (basalt, hydrothermally derived, sediment, and actively venting) and their combinations if more than one type was present in an image. A substrate was classified as actively venting if live tubeworms or microbial matting were present in the image. If a substrate accounted for > 75% of the total image area, it was classified as the dominant type. If multiple substrate types were present, each accounting for > 25% of the image area, the image was classified as a mixed substrate type.
Data Analysis
All records of deep-water corals and rossellid vase sponges (Rossellidae) from the forward-facing video extracted from OFOP were loaded into the mapping software ArcGIS Pro, where the data were plotted along the dive tracks and summed over a 10 x 10 m square grid superimposed onto the dive track. For the dives at Endeavour only, the distance from each coral and vase sponge record to the nearest active vent was measured in ArcGIS Pro to determine the influence of active hydrothermal fluid flow on the abundance of these groups. To do this, the georeferenced positions of all known active vents and their areal extent within the Endeavour segment (Jamieson et al., 2014; Clague et al., 2020; this study) were combined into a single polygon layer in ArcGIS Pro. The polygons represent the extent of the sulfide edifices (i.e. the physical vent structures) and we assumed that fluid discharge would occur primarily within the polygon boundaries. It is possible that active venting occurs in areas outside the polygons, but we assumed it to be insignificant compared to the venting inside the polygon. For each of the active vents recorded in this study, we applied a 10-m buffer as an estimate of the extent of fluid flow at each chimney (Kelly et al., 2007). Areal extent was used to account for the presence of multiple chimneys at a single vent (e.g., Big Bad Ben) and positional error of the navigation system (5 m, Auger - CSSF personal communication). The distance from each coral or sponge record to the edge of the nearest vent polygon was measured. This analysis was not done for Middle Valley because, while there were a few focused chimneys (8 total), most hydrothermal activity was characterized as diffuse flow that spanned a large area with no distinct point source. For all dives at Endeavour, total abundance of morphotaxa was calculated for each substrate type.
The abundance data from the image capture analysis was used to construct individual-based rarefaction curves for each dive and each substrate type at Endeavour and at Middle Valley. We combined images from all dives on each segment, assuming a similar species pool within each location, to maximize the number of individuals recorded on each substrate type. The richness of morphotaxa was compared between dives and substrate types using a common number of individuals sampled (Hurlbert, 1971). Analyses were conducted in RStudio using the iNEXT software package (Chao et al., 2014; Hsieh et al., 2016).
Results
In total, 101 morphotaxa from 8 different phyla were identified at Endeavour and Middle Valley (Supplementary Table 1). At Endeavour, the most abundant morphotaxa were demosponges, comatulid crinoids, rossellid vase sponges, alcyonacean corals, and holothurians (Family Laetmogonidae). At Middle Valley, the most abundant morphotaxa were black corals, holothurians (Family Psychropotidae), anemones, ascidians, and stalked barnacles.
Substrate
At Endeavour, the most common substrate type was basalt, often as lobate basalt or sheet flows. This segment of the ridge is not heavily sedimented, resulting in mostly exposed hard substrate. Inactive sulfide deposits occurred as large upright chimneys and talus (Figure 2). Chimneys were defined as active if there was visible fluid flow in the video footage, or if there were vent obligate species present. There were 3 active chimneys recorded on dive R1938, 9 on dive R1939, 9 on dive R1940, and 4 on dive R1941. In contrast, Middle Valley was characterized primarily by thick sediment and diffuse hydrothermal flow was present over large areas without an identifiable point source. The few active chimneys (2 on dive R1942, 6 on dive R1943) that were present were much smaller than the large edifices on the Endeavour segment. Small patches of hydrothermal material were dispersed across the sedimented seafloor.
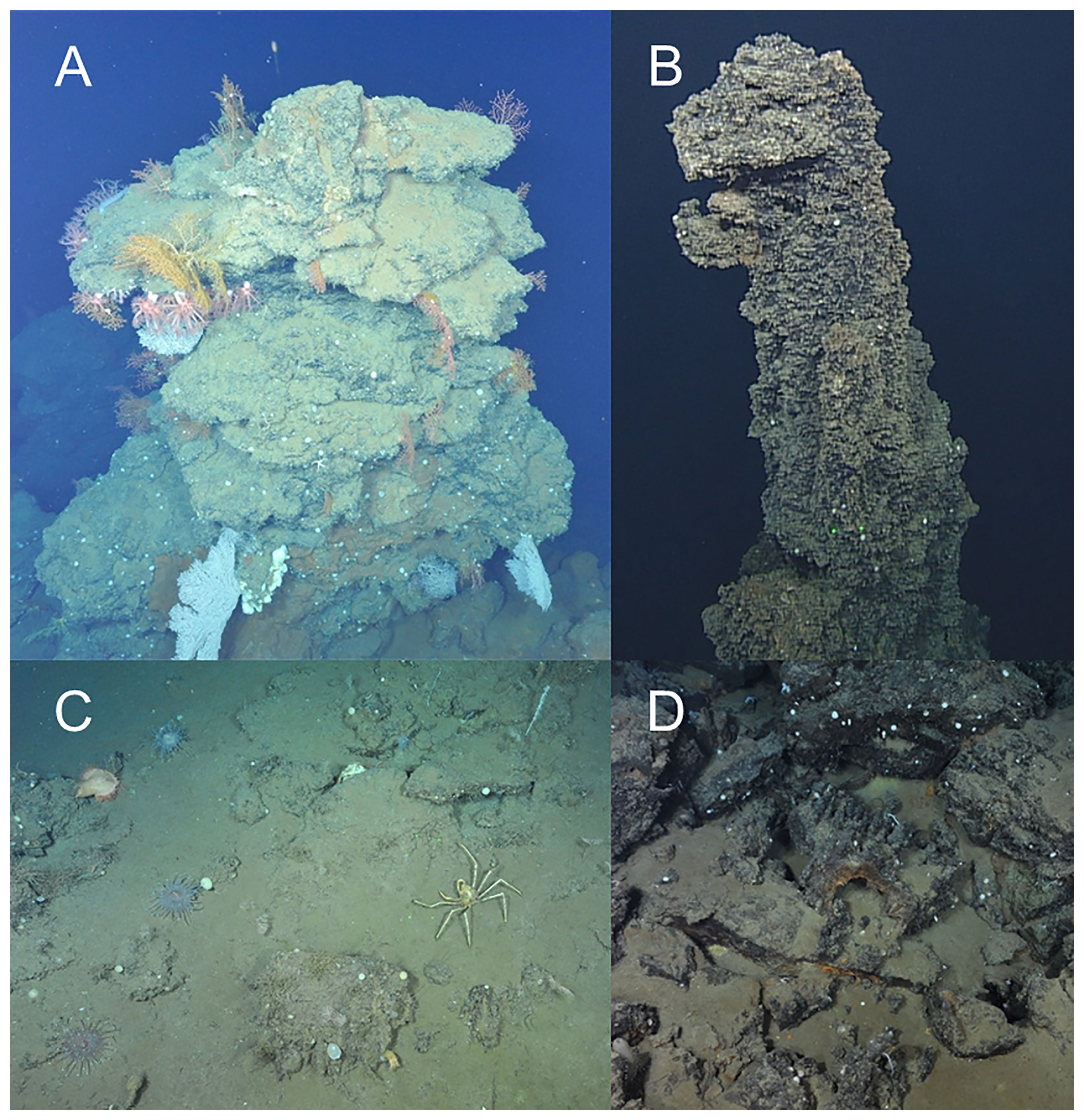
Figure 2 Photographs of inactive sulfide deposits at Endeavour and Middle Valley captured by ROPOS in August 2016. (A) An inactive chimney (dive R1939) populated with corals (Alcyoniidae spp., Plexauridae spp., Primnoidae sp. 1 and Primnoidae sp. 3), sponges (Demospongiae sp. 1 and white encrusting), and a holothurian (Pannychia moseleyi); (B) ‘Big Bad Ben’, the > 30-m tall inactive chimney (dive R1938); (C) Sedimented sulfide rubble (dive R1943) colonized by jewelled and fly trap anemones (Corallimorphus sp. 1 and Actinoscyphia aurelia), stalked barnacles (Scalpellidae sp. 1), bamboo coral (Lepidisis sp. 1), schizopathid corals (Umbellapathes sp. 1), ascidians, and an oregoniid (Macroregonia sp. 1) crab; (D) Sulfide rubble with fragments of a toppled chimney (dive R1938).
Large Mega-Epifauna
At Endeavour, 814 deep-water corals were recorded, mostly in the Order Alcyonacea (86.7% Alcyonacea, 11.7% Antipatharia). The most represented groups included Alcyoniidae (37%), Primnoidae (21%), and various small antipatharian corals (Antipatharia spp., 21%, likely Schizopathidae) on dive R1938; Plexauridae (60%) and Primnoidae (15%) on dive R1939; and Plexauridae (64%) and Alcyoniidae (32%) on dive R1940. No corals were recorded on dive R1941. Corals were always observed attached to hard substrate (basalt and inactive hydrothermal deposits) and were often seen on elevated and more exposed surfaces of basalt ridges and inactive chimneys.
Overall, the abundance of deep-water corals was highest on the walls of inactive chimneys and basalt ridges, and at distances > 50 m from active chimneys (Figures 3, 4). Corals were nearly absent within 25 m of active chimneys and sparse at 26-50 m despite the availability of hard substrate, except on dives R1938 and R1939 where a few soft corals (Alcyoniidae spp.) were recorded (Figure 4). On dive R1939, many corals were clustered on inactive sulfide deposits and chimneys at the south end of the dive (at the farthest distances from active chimneys and from the ridge axis) and on a basalt ridge located 201-300 m from active chimneys. The highest abundances of corals on dives R1938 and R1940 also correspond with the locations of inactive chimneys and basalt ridges.
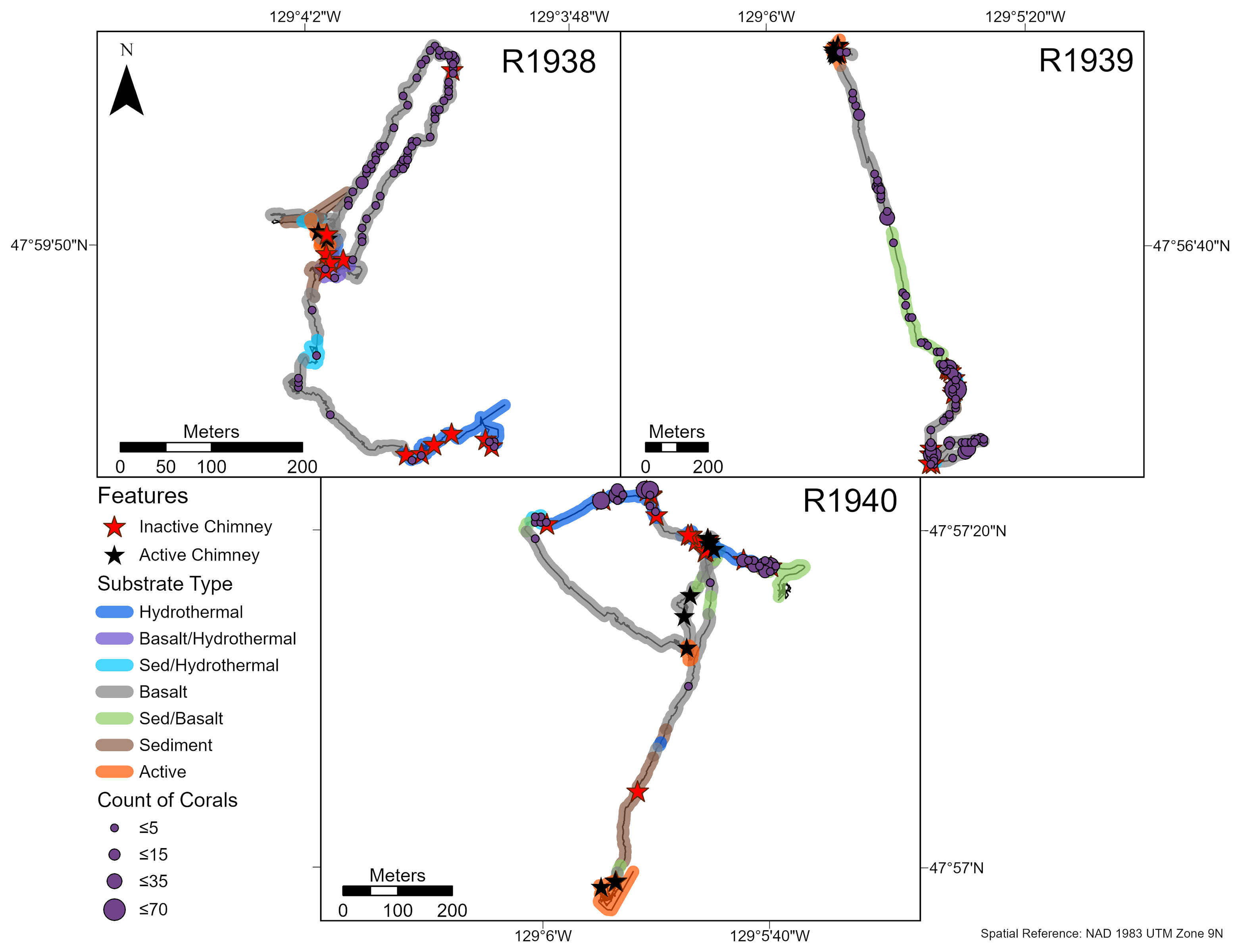
Figure 3 Abundance and distribution of deep-water corals (see Results for dominant morphotaxa) on dives R1938-R1940 at the Endeavour segment, Juan de Fuca Ridge in August 2016. Inactive and active chimney locations and broad scale substrate classifications are also plotted onto the track. “Hydrothermal” substrate refers to inactive substrate that is hydrothermally derived. Counts were summed over a 10 x 10 m tessellation in ArcGIS Pro.
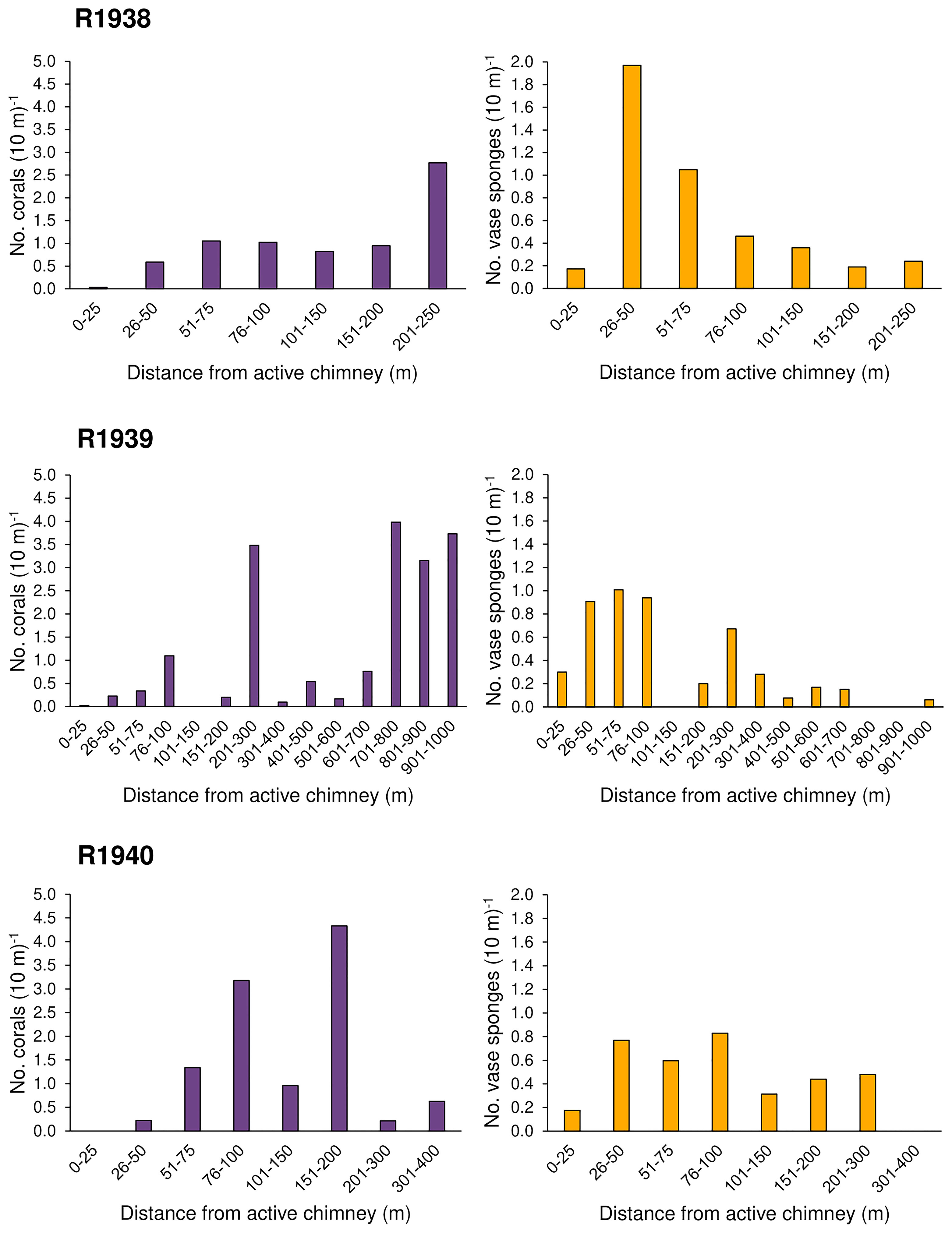
Figure 4 Number of deep-water corals and rossellid vase sponges (Rossellidae) (per 10 m of dive track) occurring within distance categories from active hydrothermal chimneys for each of 3 dives at the Endeavour segment of the Juan de Fuca Ridge, in August 2016. Dive R1941 was not included as no deep-water corals and only three rossellid vase sponges were recorded. Distance to the closest active feature was calculated in ArcGIS Pro (see Methods).
There did not appear to be a difference in the occurrence of deep-water corals between basalt and hydrothermally derived substrate (Figure 5). A greater number of corals were recorded on basalt than on hydrothermally derived substrate on dive R1938 while the opposite was the case on dive R1940. There was no difference between the two on dive R1939.
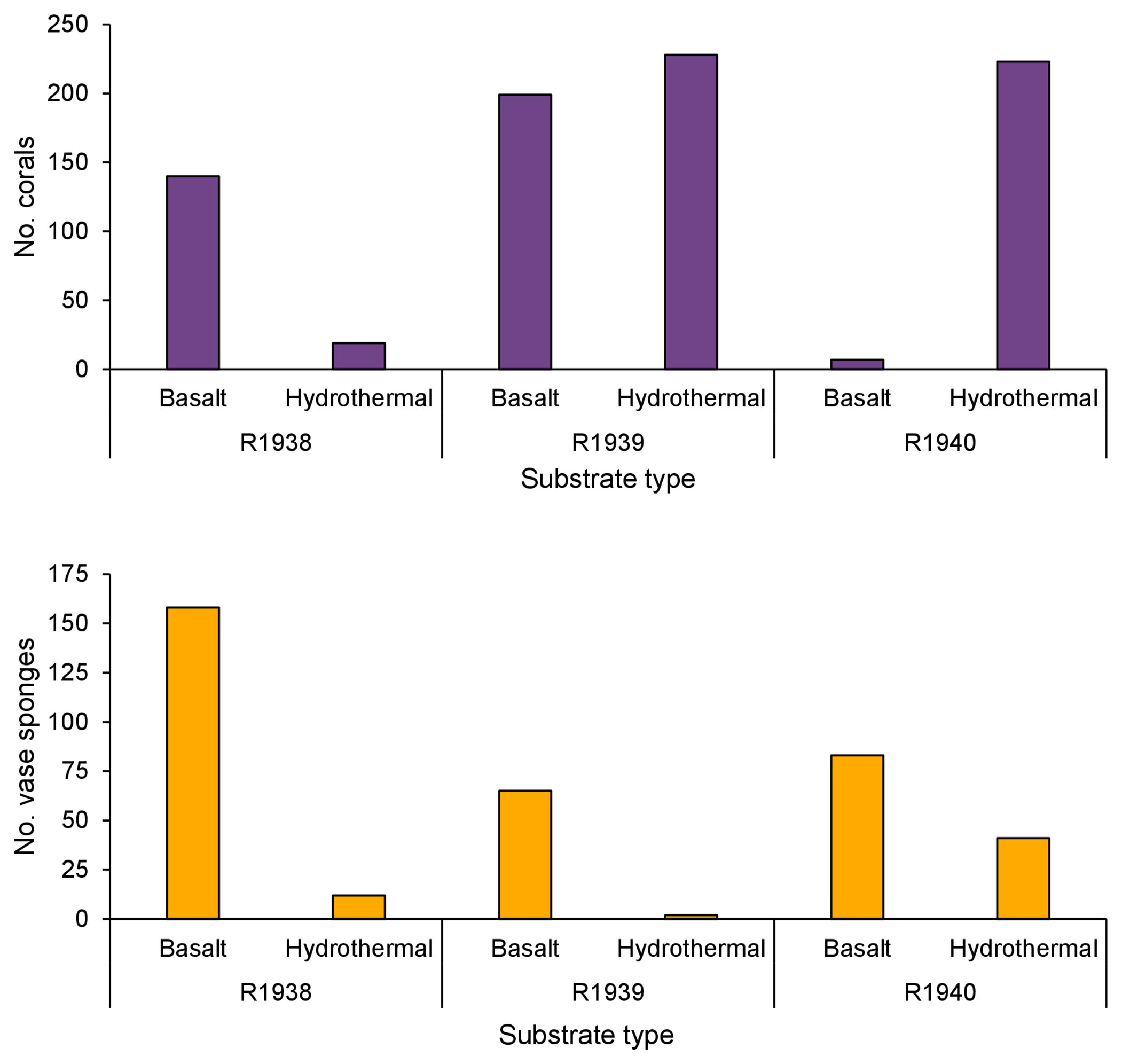
Figure 5 The occurrence of deep-water corals and rossellid vase sponges on basalt and hydrothermally derived substrate on dives R1938, R1939, and R1940. Dive R1941 was not included as no deep-water corals and only three vase sponges were recorded. Dives were conducted by remotely operated vehicle ROPOS at the Endeavour segment of the Juan de Fuca Ridge, NE Pacific, in August 2016.
At Endeavour, rossellid vase sponges (Rossellidae) were the other numerically dominant large mega-epifaunal morphotaxon and were present almost everywhere on the dive tracks except directly on active chimneys (Figure 6). In all dives, they occurred in low abundance within 25 m of active chimneys but were more abundant than corals at 26-50 m from active chimneys (Figure 4). Very few rossellid vase sponges occurred on heavily sedimented regions of the dives at Endeavour. Unlike deep-water corals, there tended to be more records of rossellid vase sponges on basalt than hydrothermally derived substrate for all dives (Figure 5).
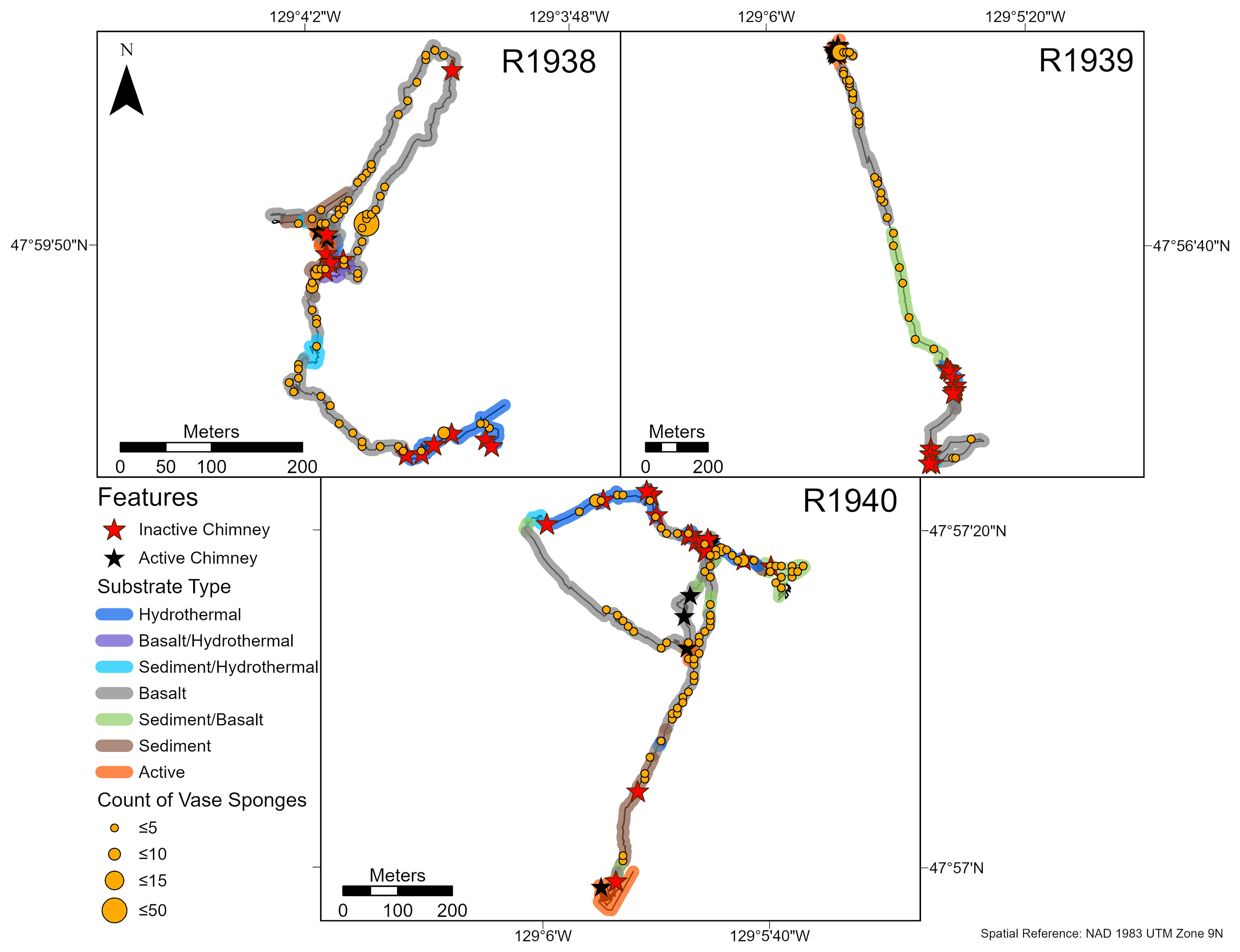
Figure 6 Abundance and distribution of rossellid vase sponges (Rossellidae) on dives R1938-R1940 at the Endeavour segment, Juan de Fuca Ridge in August 2016. Inactive and active chimney locations and broad scale substrate classifications are also plotted onto the track. “Hydrothermal” substrate refers to inactive substrate that is hydrothermally derived. Counts were summed over a 10 x 10 m tessellation in ArcGIS Pro.
At Middle Valley, 798 large deep-water corals were recorded, mostly from Antipatharia (94.9% Antipatharia, 5.1% Alcyonacea). Schizopathidae corals, specifically Umbellapathes sp. 1 were the most dominant group (92%) on both dives. These corals clustered on or around hard substrate (Supplementary Figures 2, 3), but also occurred in areas characterized as sediment. In sedimented areas, it is likely they were attached to smaller rocks or pieces of chimney debris that were buried. There did not seem to be a relationship between coral abundance and proximity to fluid flow since corals were recorded both near and far from active chimneys and diffuse flow. Very few rossellid vase sponges were recorded on dive R1942 (Supplementary Figure 2) and they were absent from dive R1943.
Small Mega-Epifauna
At Endeavour, small white demosponges (Demospongiae sp. 1) and brittle stars (Ophiuroidea spp.) were common (occurred in ≥ 25% of captured images) on all dives (Supplementary Figure 4). Comatulid crinoids (Comatulida sp. 1) were common on dives R1938 and R1939, but not on dives R1940 and R1941. The greatest abundances of these groups were found at distances > 100 m from active chimneys on dives R1938 (Figure 7) and R1940 (Figure 8). Common morphotaxa were either absent or occurred in low abundance around active chimneys (Supplementary Figures 5, 6). On dive R1940, the small white demosponges were often absent or at low densities on highly sedimented substrate.
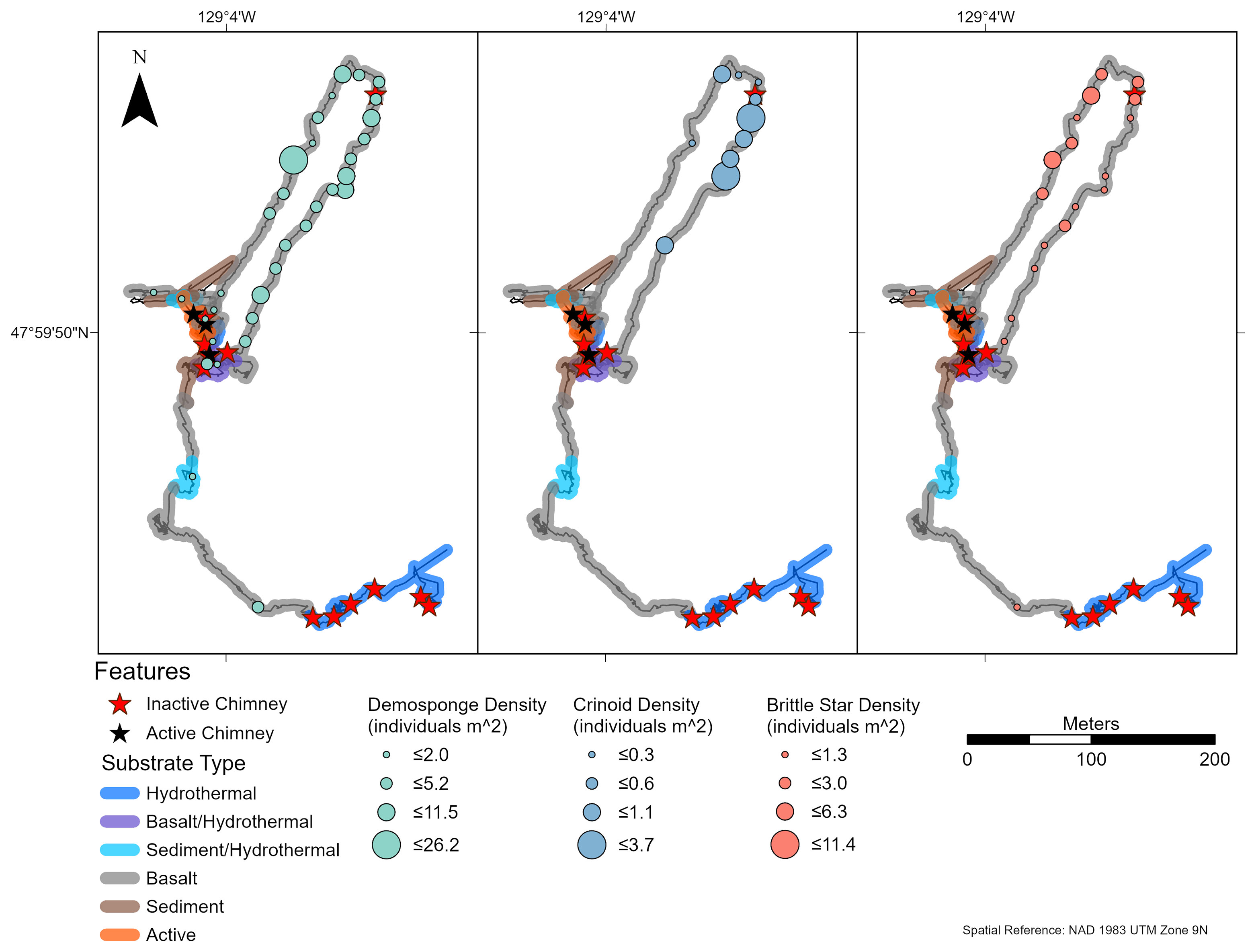
Figure 7 Density of brittle stars (Ophiuroidea spp.), comatulid crinoids (Comatulida sp. 1), and demosponges (Demospongiae sp. 1) on dive R1938, at the Endeavour Segment of the Juan de Fuca Ridge sampled in August 2016. Each symbol indicates density in an image captured at that location. These three morphotaxa were identified as the numerically dominant faunal groups (present in ≥ 25% of images) (n = 20, 10, 33 for Ophiuroidea spp., Comatulida sp. 1, and Demospongiae sp.1, respectively). “Hydrothermal” substrate refers to inactive substrate that is hydrothermally derived.
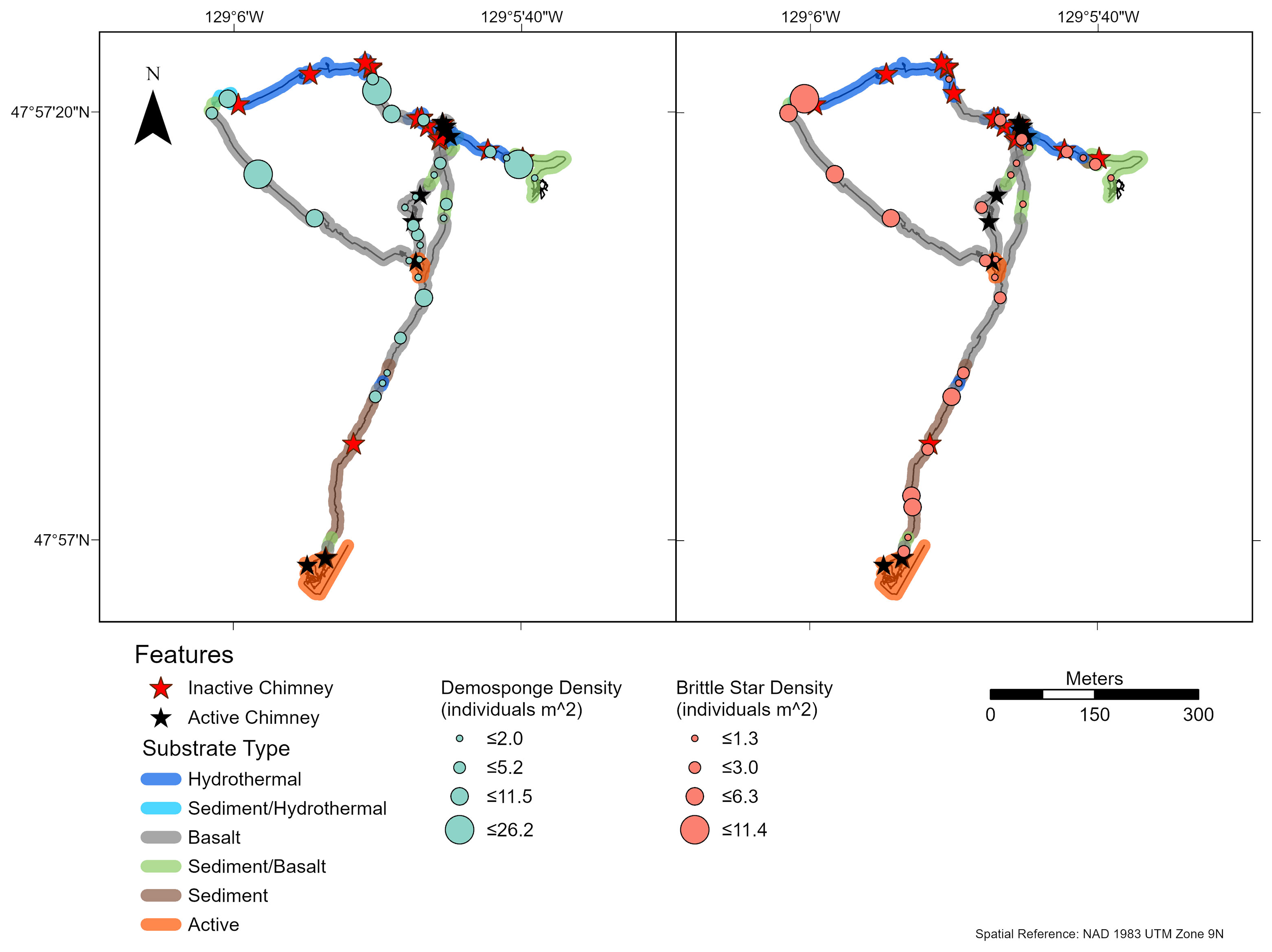
Figure 8 Density of brittle stars (Ophiuroidea spp.) and demosponges (Demospongiae sp. 1) on dive R1940, at the Endeavour Segment of the Juan de Fuca Ridge sampled in August 2016. Each symbol indicates density in an image captured at that location. These two morphotaxa were identified as the numerically dominant faunal groups (present in ≥ 25% of images) (n = 28, 29 for Ophiuroidea spp. and Demospongiae sp.1, respectively). “Hydrothermal” substrate refers to inactive substrate that is hydrothermally derived.
Total abundance of non-vent megafauna varied among the seven recorded substrate types (Figure 9). At Endeavour, the highest total abundance was on inactive chimneys, but was much more variable than on any other substrate type. The lowest total abundance was measured on sedimented substrates. For some substrate types, we were only able to capture a single image, making those estimates unreliable (Figure 9).
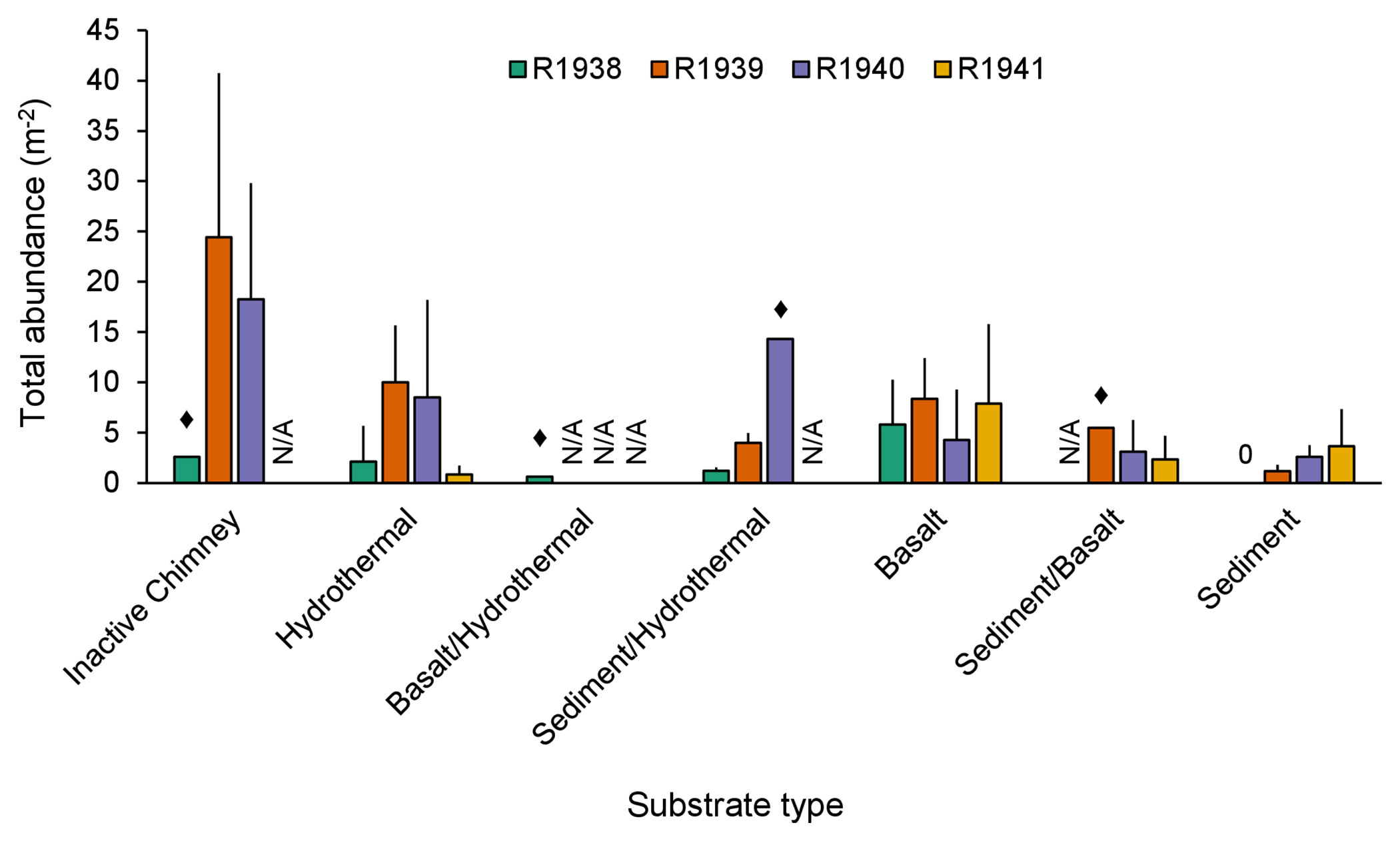
Figure 9 Average total abundance (+SD) of all non-vent morphotaxa on each substrate type for dives R1938, R1939, R1940, and R1941 at the Endeavour segment of the Juan de Fuca Ridge. Abundances were averaged across image captures of the same substrate type. ♦: n=1. “N/A”: indicates absence of that substrate type from the particular dive; 0 indicates true absence. “Hydrothermal” substrate refers to inactive substrate that is hydrothermally derived.
At Middle Valley, brittle stars (Ophiuroidea spp.) were the only morphotaxon commonly observed (occurred in ≥ 25% of captured images) on both dives; however, small white demosponges (Demospongiae sp. 1), a schizopathid coral (Umbellapathes sp.1), and jewelled anemones (Corallimorphus sp. 1) were also common on dive R1943 (Supplementary Figure 7). All four faunal groups were observed on both sediment and sulfide substrate types (Supplementary Figures 8, 9). On dive R1943, the highest abundance of brittle stars, demosponges, and Umbellapathes corals occurred on hard sulfide substrate, while the lowest abundances over areas of sediment or directly on diffuse flow.
Richness
The individual-based rarefaction curves did not reach an asymptote for any of the dives at Endeavour or Middle Valley (Figure 10). At Endeavour, for a common sample size of 246 individuals, there was no significant difference in expected richness among dives R1938, R1939, and R1940, but the expected richness for dive R1941 was significantly lower. At Middle Valley, for a common sample size of 413 individuals, the estimated richness of morphotaxa was significantly higher on dive R1943 than R1942.
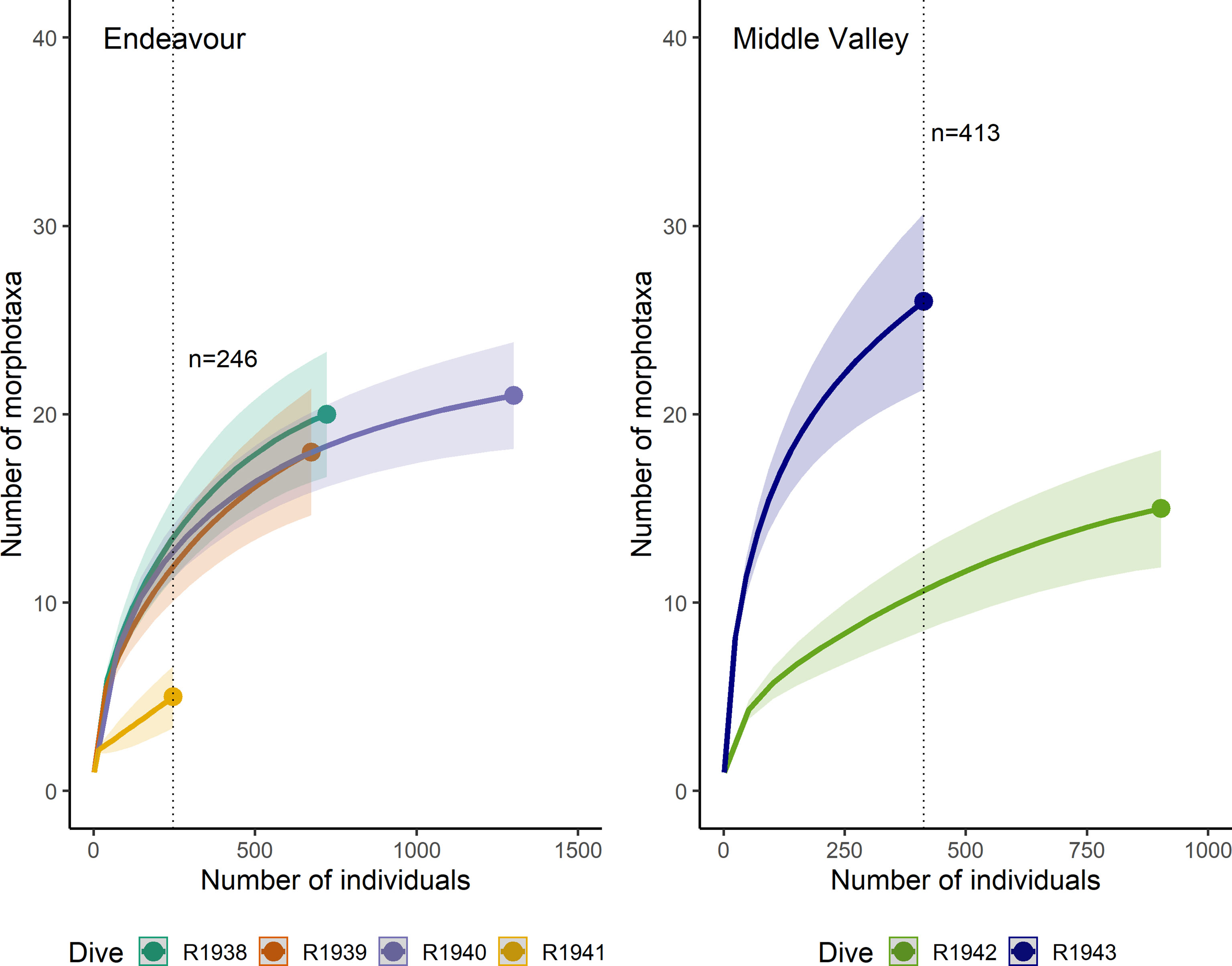
Figure 10 Individual-based rarefaction curves for the dives at Endeavour (R1938-R1941) and Middle Valley (R1942 and R1943). The dotted vertical line indicates the minimum number of individuals sampled across all dives at each site.
Individual-based rarefaction curves also did not reach an asymptote for any of the substrate types at Endeavour or Middle Valley (Figure 11; Supplementary Figures 10, 11). At Endeavour, for a common sample size of 69 individuals, the expected richness of ‘inactive chimney’ was significantly greater than both ‘basalt’ and ‘hydrothermal’, which in turn were not significantly different from one another, but were significantly greater than ‘sediment/basalt’ and ‘sediment’. The expected richness of ‘sediment/hydrothermal’ was significantly higher than “sediment” but was not significantly different from the other substrate types. At Middle Valley, for a common sample size of 32 individuals, the expected richness of ‘inactive chimney’ and ‘sediment/hydrothermal’ were significantly higher than ‘sediment’ but were not significantly different from each other. The expected richness of ‘hydrothermal’ was significantly lower than ‘sediment/hydrothermal’ but was not different from the other substrate types.
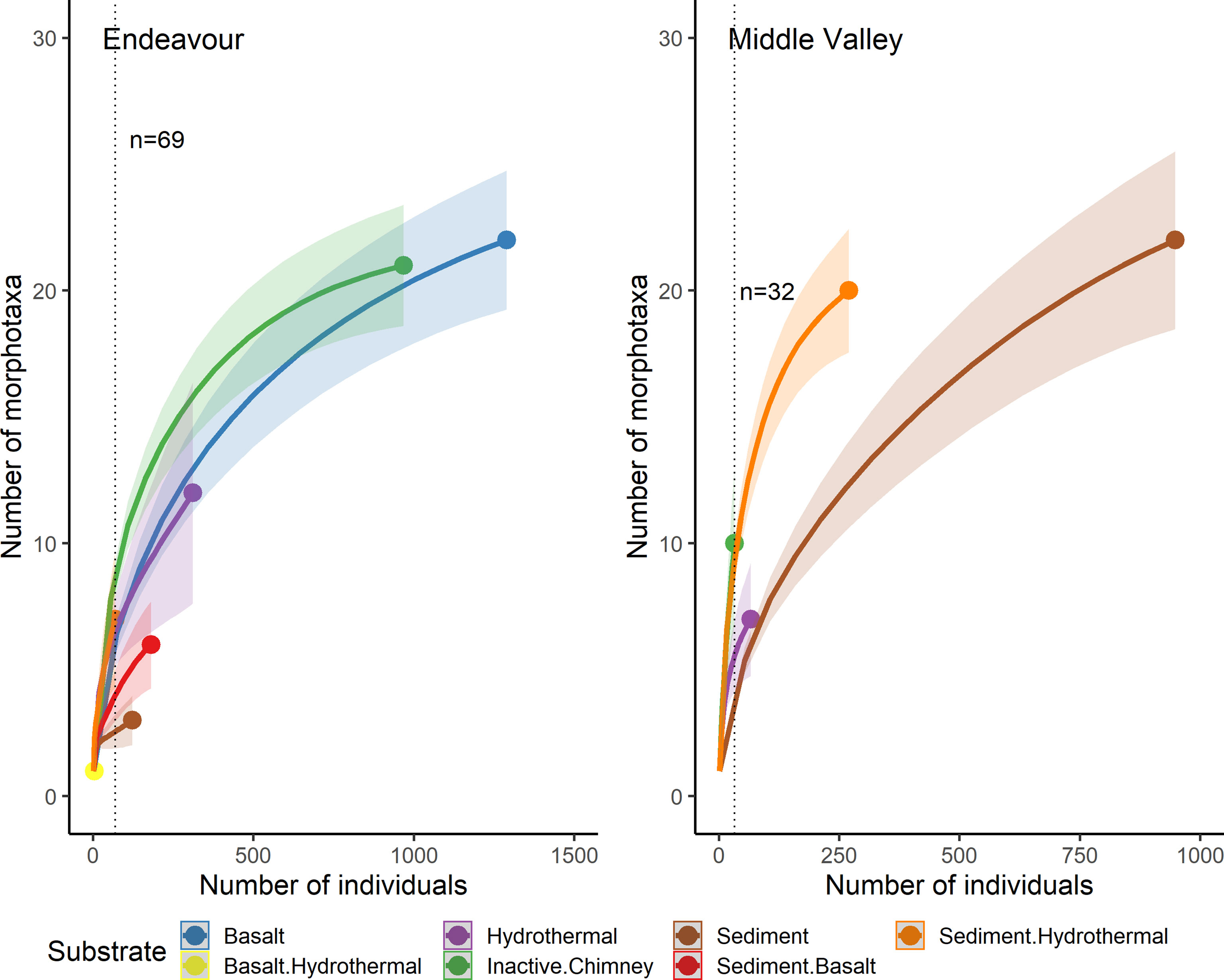
Figure 11 Individual-based rarefaction curves for each substrate type, pooled across all dives sampled at Endeavour (R1938, R1939, R1940 and R1941) and Middle Valley (R1942 and R1943) on the Juan de Fuca Ridge, NE Pacific. “Hydrothermal” substrate refers to inactive substrate that is hydrothermally derived. The substrate ‘basalt/hydrothermal’ was omitted from the rarefaction analysis because only 3 individuals of a single morphotaxon were recorded.
Discussion
Our study characterized non-vent faunal communities on two distinct hydrothermal systems on the Juan de Fuca Ridge. Overall, inactive vents harboured slow growing sessile mega-epifauna, but abundance varied widely among sites. Faunal assemblages at Endeavour were dominated by alcyonacean corals, stalked and comatulid crinoids, sponges, and holothurians. This pattern was very similar to that on the Cleft segment of the Juan de Fuca Ridge, where the most abundant non-vent taxa were alcyonacean corals and sea pens, stalked and comatulid crinoids, and holothurians (Milligan and Tunnicliffe, 1994). The Cleft segment is located further south on the Juan de Fuca Ridge and is also characterized by basalt lava flows (Milligan and Tunnicliffe, 1994). At Middle Valley, which is heavily sedimented, black corals, anemones, holothurians and ascidians were abundant. This community was different from that observed in the heavily sedimented environment of Mohn’s Treasure mound on Mohn’s Ridge, where echinoderms and sponges were the most common morphotaxa, and fields of stalked crinoids were observed in the sediment (Ramirez-Llodra et al., 2020). However, similar to Mohn’s Treasure, small patches or outcrops of hard substrate were dominated by suspension feeding taxa, while the sediment was typically inhabited by more mobile fauna such as echinoderms.
Deep-water coral communities were recorded at both Endeavour and Middle Valley, but there were notable differences in the composition and their distribution on the substrate. At Endeavour, alcyonacean corals dominated, and they aggregated on elevated and exposed hard surfaces, such as walls of basalt ridges and chimneys. Similarly, in the Southwest Pacific, non-vent suspension feeders, including large gorgonian corals, were more abundant at elevated surfaces of inactive chimneys (Galkin, 1997). The presence of corals on chimneys and vertical walls is likely related to nutrition since elevated substrates provide access to faster water flow, and, therefore, increased food supply (Genin et al., 1986; Levin et al., 2016). At Middle Valley, black corals (Antipatharia) of the genus Umbellapathes were the most abundant taxon on both sedimented and exposed substrates. Umbellapathes attach to hard substrate by a basal plate at the bottom of a long and thin upright stalk (Opresko and Wagner, 2020). This stalk can extend more than 50 cm from the seafloor before branching. It is possible that the tall and flexible structure of these corals is better adapted to areas of high sedimentation, such as Middle Valley, and allows the corals to occur on both exposed and buried hydrothermal substrates. Umbellapathes was not recorded at Endeavour, and none of the most abundant groups of alcyonacean corals at Endeavour were recorded at Middle Valley. In fact, only a few alcyonacean corals were recorded at Middle Valley, and most were unbranched Isididae which also have a tall structure. The morphology and distribution of these corals suggests that the differences in community composition of deep-water corals at Endeavour and Middle Valley were influenced by available substrate and level of sedimentation.
At Endeavour, the abundance of soft corals was low at distances < 50 m from active chimneys. Active hydrothermal vents can be inhospitable for non-vent taxa because of the composition of the hydrothermal fluid (Corliss et al., 1979) and frequent physical disturbance (Grigg, 1997). At Endeavour, hydrothermal plumes extend roughly 150-300 m above active vent sites and chemical anomalies have been detected as much as 5 km away from central vent sites (Thomson et al., 1992). It is likely that corals colonize areas at distances from vents that maximize increased availability of vent-derived nutrients (Erickson et al., 2009) but also minimize exposure to toxic chemicals (Grigg, 1997; Sen et al., 2016). Interestingly, the greatest number of corals at Endeavour were recorded on and around inactive chimneys (on dive R1939) that were located furthest from the ridge axis. Radioisotope dating of inactive vents at Endeavour has shown that, in general, the oldest vents occur on the axial valley walls or outside the axial valley (Jamieson et al., 2013). Older chimneys that are further away from the ridge axis, and have been inactive for a longer period, provide a stable environment for slow growing deep-water corals (Andrews et al., 2001; Roark et al., 2009; Sherwood and Edinger, 2009). Therefore, the particularly dense assemblage of corals found on dive R1939 could be related to the age of the sulfide deposit and the distance from active fluid flow. Since there was no notable difference in the total number of corals between basalt and hydrothermally derived hard substrates, the observed patterns were not related to the geological composition of the substrate but rather to the topography and distance from vent activity.
Rossellid vase sponges at Endeavour occurred mostly on hard substrates (basalt and sulfide). Although their abundance was also low within 25 m of active chimneys, sponges were more abundant than corals at distances of 26-50 m from active chimneys. Due to the difficulty in identifying species of morphotaxa from imagery, it is possible that more than one species is represented within this morphotaxon. However, their proximity to active vents suggests this morphotaxon overall is more tolerant of toxic chemicals than corals and may be utilizing particulate organic carbon and microbes in the water column, which have increased concentrations near hydrothermal vents (Enright et al., 1981; Galkin, 1997). Other glass sponges rely on fluid flow (Erickson et al., 2009), possibly even housing endosymbiotic bacteria to derive chemosynthetic energy (Georgieva et al., 2020). The sponges that may house these bacteria typically occur within 20 m of vents (Georgieva et al., 2020); however, in our study, rossellid vase sponges occurred at all distances to active venting, suggesting that they are not likely to be reliant on fluid flow but benefitting from elevated nutrients and the hard substrate.
The most common small mega-epifauna were brittle stars, demosponges, and crinoids at Endeavour and brittle stars, Umbellapathes corals, demosponges, and jewelled anemones at Middle Valley. The absence of crinoids at Middle Valley may be attributed to the lack of exposed and elevated hard substratum (Boschen et al., 2015; Ramirez-Llodra et al., 2020). Generally, brittle stars, demosponges, and crinoids seemed to be more abundant further away from active venting. At Endeavour, the absence of fauna from available hard substrate near active chimneys indicated that these groups were not limited by available substrate, but rather avoided fluid discharge.
Richness of morphotaxa at Endeavour was comparable across three of the dives (R1938-R1940), which were of similar length and in regions of the ridge with similar hydrothermal conditions and substrate. Dive R1941, however, mainly sampled three large active chimneys and a few short (< 250 m) segments of inactive substrate, resulting in a lower number of suitable images, and significantly lower estimated richness. Although the total track length for this dive was 2651 m, visible inactive substrate was only surveyed along 923 m of the total length, and only to a maximum of 86 m away from vent activity. At Middle Valley, richness differed significantly between the two dives. Dive R1942 traversed the Bent Hill vent field which was largely characterized by sediment, while dive R1943 traversed the Dead Dog vent field and surveyed sediment, inactive hydrothermal deposits and chimneys. Sampling more substrate types each with different associated faunal assemblages, resulted in a higher estimated richness on dive R1943 than R1942.
Among all substrate types at Endeavour, faunal communities on inactive chimneys had the greatest estimated richness and total abundance. However, the greatest variation in total abundance was also measured on inactive chimneys. Similarly to the South Pacific (Boschen et al., 2016), not all inactive chimneys in our study were colonized by fauna; some hosted dense communities while others were devoid of megafauna. This variation, even between chimneys at similar distances from active vents, suggests that factors such as local currents, and the chemical and microbial composition of the chimneys could be influencing colonization (Boschen et al., 2016). Communities on basalt and hydrothermal substrates had the next highest richness and total abundance, exceeding that of sedimented substrate types. This may be due to the life history traits of the non-vent assemblages at Endeavour, which are largely composed of sessile and sedentary suspension feeders that attach to hard substrates. It is important to note that the difference in richness between substrates was recorded at a small common sample size which was constrained by the sampling effort on sedimented substrates. A similar comparison at 1000 individuals indicates no difference in richness between basalt and inactive chimney substrates.
At Middle Valley, the mixed sediment/hydrothermal substrate type exhibited higher estimated richness than either sediment or hydrothermal alone. This is probably because fauna associated with both substrate types were present. A similar trend was observed at a highly sedimented area of the Arctic Mid-Ocean Ridge, where higher richness was found in areas with mixed substrate (Ramirez-Llodra et al., 2020). Inactive chimneys exhibited a higher estimated richness than sediment, indicating that fauna also utilize elevated and exposed substrate at Middle Valley.
Our study was opportunistic and thus based on a non-systematic layout of transects on each dive. The ROV stopped to sample frequently, flew over the same area multiple times resulting in duplicate footage, and often flew too far above the seafloor to obtain suitable imagery, making large portions of the dive track unusable for this study. Often, the scaling lasers were turned off or moved out of the field of view, providing no size reference for analysis. Additionally, the quality of imagery from the downward-looking camera was variable because of frequent changes in altitude above the seafloor, speed and direction of flight of the ROV, and unsuitable camera angles. This resulted in a non-uniform distribution of suitable images along the dive tracks, likely misrepresenting the number and distribution of morphotaxa on each dive and under sampling small and rare species, as also evidenced by the rarefaction curves. To better understand the distribution of non-vent fauna at inactive vents, studies should follow a well-designed and systematic sampling regime that prioritizes image quality throughout the dive by maintaining a constant altitude above the seafloor, speed, and camera/laser angle.
The increasing interest in mining polymetallic sulfide deposits at deep-sea hydrothermal vents necessitates the development of environmental regulations to minimize risk to the marine environment. Mining operations will have substantial direct and indirect impacts on both the targeted vents and the vent periphery. Our study demonstrates that inactive hydrothermal vents at Endeavour and Middle Valley support non-vent assemblages that are dominated by suspension feeding taxa such as corals, crinoids, and rossellid vase sponges. These taxa are fragile and sensitive to indirect plume impacts from deep sea mining (Boschen-Rose et al., 2021). Deep-water corals and sponges are considered particularly vulnerable to mining impacts because they are long-lived and slow growing, and their recovery potential is uncertain (Clark et al., 2016). To ultimately assess the vulnerability of inactive vent ecosystems to mining operations and develop sustainable management plans, it is critical to gain a basic understanding of the structure and ecology of the communities that inhabit them and the surrounding area.
Data Availability Statement
The raw data supporting the conclusions of this article will be made available by the authors, without undue reservation.
Author Contributions
AM conceptualized this study. MN and AM designed the protocol for data analysis. MN conducted the analysis of the video and data and prepared the manuscript. JJ participated on the research cruise and co-directed the ROV dives, contributed the video and supported data analysis. All authors contributed to the article and approved the submitted version.
Funding
This project was supported by Natural Sciences and Engineering Research Council of Canada (NSERC) Discovery Grants to AM [RGPIN-2016-04878] and undergraduate summer research awards (Nancy Witherspoon Memorial Summer Research Award, Rob Stewart Undergraduate Summer Research Award in Marine Conservation) to MN. The 2016 research cruise to Endeavour and Middle Valley aboard the CCGS John P. Tully was co-led by researchers at Fisheries and Oceans Canada and the University of Victoria, with Memorial University of Newfoundland as a contributing partner. The cruise was funded in part by the NSERC Canadian Healthy Oceans Network and its Partners: Department of Fisheries and Oceans Canada and INREST (representing the Port of Sept-Îles and City of Sept-Îles) [NETGP 468437 – 14]. JJ acknowledges funding support from the Canada Research Chairs Program [950 - 233103] for the ROV dives and participation on the cruise.
Conflict of Interest
The authors declare that the research was conducted in the absence of any commercial or financial relationships that could be construed as a potential conflict of interest.
Publisher’s Note
All claims expressed in this article are solely those of the authors and do not necessarily represent those of their affiliated organizations, or those of the publisher, the editors and the reviewers. Any product that may be evaluated in this article, or claim that may be made by its manufacturer, is not guaranteed or endorsed by the publisher.
Acknowledgments
We thank the ROV ROPOS pilots and technical team, and crew of the CCGS John P. Tully for their critical contribution to the collection of the video data for this project. We thank Janelle Curtis (Fisheries and Oceans Canada), and Kim Juniper and Verena Tunnicliffe (University of Victoria) for their contribution to conceptualization of the 2016 research cruise, as well as cruise organization and logistics. We thank Ben Grupe for his expert role as Chief Scientist during the cruise. We would like to thank Cherisse Du Preez and Merlin Best (DFO – British Columbia) for offering their expertise and resources on the identification of deep-sea taxa; Adrienne Schumlich, Sean Mullan and Fabio De Leo (Ocean Networks Canada) for providing access to bathymetry data and main vent field locations used in figures; and Sarah de Mendonça, Kate Arpin, and Graeme Guy for offering their advice and assistance throughout the project.
Supplementary Material
The Supplementary Material for this article can be found online at: https://www.frontiersin.org/articles/10.3389/fmars.2022.849976/full#supplementary-material
References
Ames D. E., Franklin J. M., Hannington M. D. (1993). Mineralogy and Geochemistry of Active and Inactive Chimneys and Massive Sulfide, Middle Valley, Northern Juan De Fuca Ridge: An Evolving Hydrothermal System. Can. Mineral 31:4, 997–1024.
Andrews A. H., Cordes E. E., Mahoney M. M., Munk K., Coale K. H., Cailliet G. M., et al. (2001). Age, Growth and Radiometric Age Validation of a Deep-Sea, Habitat-Forming Gorgonian (Primnoa Resedaeformis) From the Gulf of Alaska. Hydrobiologia 471, 101–110. doi: 10.1023/A:1016501320206
Arquit A. M. (1990). Geological and Hydrothermal Controls on the Distribution of Megafauna in Ashes Vent Field, Juan De Fuca Ridge. J. Geophys. Res. – Sol Ea 95:B8, 12947–12960. doi: 10.1029/JB095iB08p12947
Boschen-Rose R. E., Clark M. R., Rowden A. A., Gardner J. P. A. (2021). Assessing the Ecological Risk to Deep-Sea Megafaunal Assemblages From Seafloor Massive Sulfide Mining Using a Functional Traits Sensitivity Approach. Ocean Coast Manage. 210. doi: 10.1016/j.ocecoaman.2021.105656
Boschen R. E., Rowden A. A., Clark M. R., Barton S. J., Pallentin A., Gardner J. P. A. (2015). Megabenthic Assemblage Structure on Three New Zealand Seamounts: Implications for Seafloor Massive Sulfide Mining. Mar. Ecol. Prog. Ser. 523, 1–14. doi: 10.3354/meps11239
Boschen R. E., Rowden A. A., Clark M. R., Pallentin A., Gardner J. P. A. (2016). Seafloor Massive Sulfide Deposits Support Unique Megafaunal Assemblages: Implications for Seabed Mining and Conservation. Mar. Environ. Res. 115, 78–88. doi: 10.1016/j.marenvres.2016.02.005
Carey A. G., Stein D. L., Rona P. L. (1990). Benthos of the Gorda Ridge Axial Valley (NE Pacific Ocean): Taxonomic Composition and Trends in Distribution. Prog. Oceanog 24, 47–57. doi: 10.1016/0079-6611(90)90018-W
Chao A., Gotelli N. J., Hsieh T. C., Sander E. L., Ma K. H., Colwell R. K., et al. (2014). Rarefaction and Extrapolation With Hill Numbers: A Framework for Sampling and Estimation in Species Diversity Studies. Ecol. Monogr. 84, 45–67. doi: 10.1890/13-0133.1
Cherkashov G., Kuznetsov V., Kuksa K., Tabuns E., Maksimov M., Bel'tenev V. (2017). Sulfide Geochronology Along the Northern Equatorial Mid-Atlantic Ridge. Ore Geol. Rev. 87, 147–154. doi: 10.1016/j.oregeorev.2016.10.015
Clague D. A., Martin J. F., Paduan J. B., Butterfield D. A., Jamieson J. W., Le Saout M., et al. (2020). Hydrothermal Chimney Distribution on the Endeavour Segment, Juan De Fuca Ridge. Geochem Geophys. Geosyst 21, 6. doi: 10.1029/2020GC008917
Clark M. R., Althaus F., Schlacher T. A., Williams A., Bowden D. A., Rowden A. A. (2016). The Impacts of Deep-Sea Fisheries on Benthic Communities: A Review. ICES J. Mar. Sci. 73, i51–i69. doi: 10.1093/icesjms/fsv123
Collins P. C., Kennedy R., Van Dover C. L. (2012). A Biological Survey Method Applied to Seafloor Massive Sulphides (SMS) With Contagiously Distributed Hydrothermal-Vent Fauna. Mar. Ecol. Prog. Ser. 452, 89–107. doi: 10.3354/MEPS09646
Corliss J. B., Dymond J., Gordon L. I., Edmond J. M., von Herzen R. P., Ballard R. D., et al. (1979). Submarine Thermal Springs on the Galápagos Rift. Science 203:4385, 1073–1083. doi: 10.1126/science.203.4385.1073
Dasgupta S., Peng X., Ta K. (2021). Interaction Between Microbes, Minerals, and Fluids in Deep-Sea Hydrothermal Systems. Minerals 11, 1–15. doi: 10.3390/min11121324
Delaney J. R., Robigou V., Mcduff R. E., Tivey M. K. (1992). Geology of a Vigorous Hydrothermal System on the Endeavour Segment, Juan De Fuca Ridge. J. Geophys. Res. 97:B13, 19663–19682. doi: 10.1029/92jb00174
Du Preez C., Curtis J. M. R., Clarke M. E. (2016). The Structure and Distribution of Benthic Communities on a Shallow Seamount (Cobb Seamount, Northeast Pacific Ocean). PloS One 11 (10), e0165513. doi: 10.1371/journal.pone.0165513
Earth Negotiations Bulletin (2020) Summary of the Twenty-sixth Annual Session of the International Seabed Authority (First Part): 17-21 February 2020. Available at: https://enb.iisd.org/events/1st-part-26th-annual-session-international-seabed-authority-isa/summary-report-17-21 (Accessed October 3, 2021).
Enright J. T., Newman W. A., Hessler R. R., McGowan J. A. (1981). Deep-Ocean Hydrothermal Vent Communities. Nature 289, 219–221. doi: 10.1038/289219a0
Erickson K. L., Macko S. A., Van Dover C. L. (2009). Evidence for a Chemoautotrophically Based Food Web at Inactive Hydrothermal Vents (Manus Basin). Deep-Sea Res. II 56, 1577–1585. doi: 10.1016/j.dsr2.2009.05.002
Galkin S. V. (1997). Megafauna Associated With Hydrothermal Vents in the Manus Back-Arc Basin (Bismarck Sea). Mar. Geol. 142, 197–206. doi: 10.1016/S0025-3227(97)00051-0
Genin A., Dayton P. K., Lonsdale P. F., Spiess F. N. (1986). Corals on Seamount Peaks Provide Evidence of Current Acceleration Over Deep-Sea Topography. Nature 322, 59–61. doi: 10.1038/322059a0
Georgieva M. N., Taboada S., Riesgo A., Diez-Vives C., De Leo F. C., Jeffreys R. M., et al. (2020). Evidence of Vent-Adaptation in Sponges Living at the Periphery of Hydrothermal Vent Environments: Ecological and Evolutionary Implications. Front. Microbiol. 11. doi: 10.3389/fmicb.2020.01636
Gerdes K. H., Martinez Arbizu P., Schwentner M., Freitag R., Schwarz-Schampera U., Brandt A., et al. (2019). Megabenthic Assemblages at the Southern Central Indian Ridge – Spatial Segregation of Inactive Hydrothermal Vents From Active-, Periphery- and Non-Vent Sites. Mar. Environ. Res. 151, 1–11. doi: 10.1016/j.marenvres.2019.104776
Grigg R. W. (1997). Benthic Communities on Lo’ihi Submarine Volcano Reflect High-Disturbance Environment. Pac Sci. 51, 209–220.
Hannington M., De Ronde C., Petersen S. (2005). “Sea-Floor Tectonics and Submarine Hydrothermal Systems” in One Hundredth Anniversary Volume. Eds. Hedenquist J., Thompson J., Goldfarb R., Richards J. (Littleton, CO, USA: Society of Economic Geologists), 111–141. doi: 10.5382/AV100.06
Hannington M. D., Jamieson J., Monecke T., Petersen S. (2010). ““Modern Seafloor Massive Sulfides and Base Metal Resources: Toward an Estimate of Global Seafloor Massive Sulfide Potential”,” in The Challenge of Finding New Mineral Resources: Global Metallogeny, Innovative Exploration, and New Discoveries. Eds. Goldfarb R., Marsh E., Monecke T. (Littleton, CO USA: Society of Economic Geologists), 317–338. doi: 10.5382/SP.15.2.001
Haymon R. M. (1983). Growth History of Black Smoker Hydrothermal Chimneys. Nature 301, 695–698. doi: 10.1038/301695a0
Hsieh T. C., Ma K. H., Chao A. (2016). iNEXT: An R Package for Interpolation and Extrapolation of Species Diversity (Hill Numbers). Methods Ecol. Evol. 7:12, 1451–1456. doi: 10.1111/2041-210X.12613
Huetten E., Greinert J. (2008). Software Controlled Guidance, Recording and Post-Processing of Seafloor Observations by ROV and Other Towed Devices: The Software Package OFOP. Geophys. Res. Abstr 10, EGU2008–A-03088.
Hurlbert S. H. (1971). The Nonconcept of Species Diversity: A Critique and Alternative Parameters. Ecology 52, 577–586. doi: 10.2307/1934145
Jamieson J. W., Clague D. A., Hannington M. D. (2014). Hydrothermal Sulfide Accumulation Along the Endeavour Segment, Juan De Fuca Ridge. Earth Planet Sc Lett. 395, 136–148. doi: 10.1016/j.epsl.2014.03.035
Jamieson J. W., Gartman A. (2020). Defining Active, Inactive, and Extinct Seafloor Massive Sulfide Deposits. Mar. Policy 117. doi: 10.1016/j.marpol.2020.103926
Jamieson J. W., Hannington M. D., Clague D. A., Kelley D. S., Delaney J. R., Holden J. F., et al. (2013). Sulfide Geochronology Along the Endeavour Segment of the Juan De Fuca Ridge, Geochem. Geophys. Geosyst 14, 2084–2099. doi: 10.1002/ggge.20133
Juniper S. K., Tunnicliffe V., Southward C. (1992). Hydrothermal Vents in Turbidite Sediments on a Northeast Pacific Spreading Centre: Organisms and Substratum at an Ocean Drilling Site. Can. J. Zool. 70, 1792–1809. doi: 10.1139/z92-247
Kelley D. S., Carbotte S. M., Caress D. W., Clague D. A., Delaney J. R., Gill J. B., et al. (2012). Endeavour Segment of the Juan De Fuca Ridge: One of the Most Remarkable Places on Earth. Oceanography 25:1, 44–61. doi: 10.5670/oceanog.2012.03
Kelly N., Metaxas A., Butterfield D. (2007). Spatial and Temporal Patterns of Colonization by Deep-Sea Hydrothermal Vent Invertebrates on the Juan De Fuca Ridge, NE Pacific. Aquat Biol. 1, 1–16. doi: 10.3354/ab00001
Levin L. A., Baco A. R., Bowden D. A., Colaco A., Cordes E. E., Cunha M. R., et al. (2016). Hydrothermal Vents and Methane Seeps: Rethinking the Sphere of Influence. Front. Mar. Sci. 3. doi: 10.3389/fmars.2016.00072
Marcus J., Tunnicliffe V., Butterfield D. A. (2009). Posteruption Succession of Macrofaunal Communities at Diffuse Flow Hydrothermal Vents on Axial Volcano, Juan De Fuca Ridge, Northeast Pacific. Deep-Sea Res. Part II 56, 1586–1598. doi: 10.1016/j.dsr2.2009.05.004
Milligan B. N., Tunnicliffe V. (1994). Vent and Nonvent Faunas of Cleft Segment, Juan De Fuca Ridge, and Their Relations to Lava Age. J. Geophys. Res. 99:B3, 4777–4786. doi: 10.1029/93JB03210
Mullineaux L. S., Metaxas A., Beaulieu S. E., Bright M., Gollner S., Grupe B. M., et al. (2018). Exploring the Ecology of Deep-Sea Hydrothermal Vents in a Metacommunity Framework. Front. Mar. Sci. 5. doi: 10.3389/fmars.2018.00049
Okamoto N., Shiokawa S., Kawano S., Sakurai H., Yamaji N., Kurihara M. (2018). Current Status of Japan's Activities for Deep-Sea Commercial Mining Campaign. In 2018 OCEANS - MTS/IEEE Kobe Techno-Oceans (OTO), 2018. 1–7. doi: 10.1109/OCEANSKOBE.2018.8559373
Opresko D. M., Wagner D. (2020). New Species of Black Corals (Cnidaria:Anthozoa: Antipatharia) From Deepsea Seamounts and Ridges in the North Pacific. Zootaxa 4868:4, 543–559. doi: 10.11646/zootaxa.4868.4.5
Petersen S., Krӓtschell A., Augustin N., Jamieson J., Hein J. R., Hannington M. D. (2016). News From the Seabed – Geological Characteristics and Resource Potential of Deep-Sea Mineral Resources. Mar. Policy 70, 175–187. doi: 10.1016/j.marpol.2016.03.012
Ramirez-Llodra E., Hilario A., Paulsen E., Costa C. V., Bakken T., Johnsen G., et al. (2020). Benthic Communities on the Mohn’s Treasure Mound: Implications for Management of Seabed Mining in the Arctic Mid-Ocean Ridge. Front. Mar. Sci. 7. doi: 10.3389/fmars.2020.00490
Riddihough R. (1984). Recent Movements of the Juan-de-Fuca Plate System. J. Geo- Phys. Res. 89:B8, 6980–6994. doi: 10.1029/JB089iB08p06980
Roark E. B., Guilderson T. P., Dunbar R. B., Fallon S. J., Mucciarone D. A. (2009). Extreme Longevity in Proteinaceous Deep-Sea Corals. Proc. Natl. Acad. Sci. U.S.A. 106:13, 5204–5208. doi: 10.1073/pnas.0810875106
Sarrazin J., Robigou V., Juniper S. K., Delaney J. R. (1997). Biological and Geological Dynamics Over Four Years on a High-Temperature Sulfide Structure at the Juan De Fuca Ridge Hydrothermal Observatory. Mar. Ecol. Prog. Ser. 153, 5–24. doi: 10.3354/meps153005
Scientific Abyss Mapping Services. (2009) Ofop Documentation: Version 3.2.0k. Available at: http://www.ofop-by-sams.eu/ (Accessed November 7, 2017).
Sen A., Kim S., Miller A. J., Hovey K. J., Hourdez S., Luther Iii G. W. (2016). Peripheral Communities of the Eastern Lau Spreading Center and Valu Fa Ridge: Community Composition, Temporal Change and Comparison to Near-Vent Communities. Mar. Ecol. 37, 3. doi: 10.1111/maec.12313
Sen A., Podowski E. L., Becker E. L., Shearer E. A., Gartman A., Yücel M. (2014). Community Succession in Hydrothermal Vent Habitats of the Eastern Lau Spreading Center and Valu Fa Ridge, Tonga. Limnol. Oceanogr. 59:5, 1510–1528. doi: 10.4319/lo.2014.59.5.1510
Sherwood O. A., Edinger E. N. (2009). Ages and Growth Rates of Some Deep-Sea Gorgonian and Antipatharian Corals of Newfoundloand and Labrador. Can. J. Fish Aquat Sci. 66, 142–152. doi: 10.1139/F08-195
Thomson R. E., Delaney J. R., McDuff R. E., Janecky D. R., McClain J. S. (1992). Physical Characteristics of the Endeavour Ridge Hydrothermal Plume During July 1988. Earth Planet Sci. Lett. 111:1, 141–154. doi: 10.1016/0012-821X(92)90175-U
Tunnicliffe V. (1991). The Biology of Hydrothermal Vents: Ecology and Evolution. Oceanogr Mar. Biol. Annu. Rev. 29, 319–407.
Tunnicliffe V., McArthur A. G., McHugh D. (1998). A Biogeographical Perspective of the Deep-Sea Hydrothermal Vent Fauna. Adv. Mar. Biol. 34, 353–442. doi: 10.1016/S0065-2881(08)60213-8
Van Dover C. L. (2000). The Ecology of Deep-Sea Hydrothermal Vents (Princeton, NJ, USA: Princeton University Press).
Van Dover C. L. (2011). Mining Seafloor Massive Sulphides and Biodiversity: What Is at Risk? ICES J. Mar. Sci. 68:2, 341–348. doi: 10.1093/icesjms/fsq086
Van Dover C. L. (2019). Inactive Sulfide Ecosystems in the Deep Sea: A Review. Front. Mar. Sci. 6. doi: 10.3389/fmars.2019.00461
Van Dover C. L., Arnaud-Haond S., Gianni M., Helmreich S., Huber J. A., Jaeckel A. L., et al. (2018). Scientific Rationale and International Obligations for Protection of Active Hydrothermal Vent Ecosystems From Deep-Sea Mining. Mar. Policy 90, 20–28. doi: 10.1016/j.marpol.2018.01.020
Keywords: deep-sea fauna, Juan de Fuca Ridge, polymetallic sulfide deposits, deep-sea mining, non-vent fauna, deep-water corals, vasiform sponges, inactive hydrothermal vents
Citation: Neufeld M, Metaxas A and Jamieson JW (2022) Non-Vent Megafaunal Communities on the Endeavour and Middle Valley Segments of the Juan de Fuca Ridge, Northeast Pacific Ocean. Front. Mar. Sci. 9:849976. doi: 10.3389/fmars.2022.849976
Received: 06 January 2022; Accepted: 27 April 2022;
Published: 27 May 2022.
Edited by:
David Billett, Deep Seas Environmental Solutions Ltd., United KingdomReviewed by:
Klaas Gerdes, INES - Integrated Environmental Solutions, GermanyTina Molodtsova, P.P. Shirshov Institute of Oceanology (RAS), Russia
Copyright © 2022 Neufeld, Metaxas and Jamieson. This is an open-access article distributed under the terms of the Creative Commons Attribution License (CC BY). The use, distribution or reproduction in other forums is permitted, provided the original author(s) and the copyright owner(s) are credited and that the original publication in this journal is cited, in accordance with accepted academic practice. No use, distribution or reproduction is permitted which does not comply with these terms.
*Correspondence: Monika Neufeld, TW9uaWthLk5ldWZlbGRAZGFsLmNh