- 1Key Laboratory of Tropical Marine Bio-resources and Ecology, South China Sea Institute of Oceanology, Chinese Academy of Sciences, Guangzhou, China
- 2Southern Marine Science and Engineering Guangdong Laboratory (Guangzhou), Guangzhou, China
- 3University of Chinese Academy of Sciences, Beijing, China
- 4Innovation Academy of South China Sea Ecology and Environmental Engineering, Chinese Academy of Sciences, Guangzhou, China
- 5Nansha Marne Ecological and Environmental Research Station, South China Sea Institute of Oceanology, Chinese Academy of Sciences, Guangzhou, China
Metabolisms of field plankton community, including gross primary production (GPP), community respiration (CR), and net community production (NCP), usually indicate the status of the health, resource production, and carbon budget of marine ecosystems. In this study, we explored the regional variance and driving forces of plankton metabolism in coastal waters of the Guangdong-Hong Kong-Macao Greater Bay Area (GGBA), a fast-developed area with complex hydrological and environmental states. The results showed that the maximum GPP and CR occurred in the estuarine plume of the GGBA in summer, while in winter the more active metabolisms of plankton community occurred in the Daya Bay, with the GPP and CR being respectively mediated by the nutrient level and temperature. Moreover, four regional zones were divided on the base of the environments and biological factors in surface water of the GGBA i.e., the river-runoff zone, river-plume zone, nearshore and far-offshore zones. The metabolic states in these zones varied significantly due to the regional and seasonal variations of, for example, the nutrient level, temperature, and turbidity driven by multiple factors including land-derived runoffs, anthropogenic activities, the Yuedong Coastal Current, and offshore seawater-intrusions. On the whole, the GGBA areas exhibited the weak heterotrophic processes in both summer (NCP = -24.9 ± 26.7 mg C m-3 d-1) and winter (NCP = -51.2 ± 8.51 mg C m -3 d-1). In addition, we found that the higher CR occurred to the bottom layers of the river plume and nearshore zones wherein hypoxia happened, indicating a possible attribution of plankton community respiration to the hypoxia in the GGBA.
Introduction
Rising atmospheric CO2 causes global climate change, which brings unprecedented challenges to human's sustainable development. As an important carbon reservoir on Earth, the ocean has been estimated to absorb 31 ± 4% of anthropogenic CO2 from 1994 to 2007 (Gruber et al., 2019), wherein the plankton metabolisms mediate the exchange of CO2 between air and sea (Odum, 1956; Falkowski, 1998; Duarte et al., 2013). The dynamics of gross primary production (GPP) and community respiration (CR) can directly reflect the carbon metabolism balance in marine ecosystems, as indicated by the net community production (NCP) (Duarte and Regaudie-de-Gioux, 2009; Cai, 2011). When the GPP is over the CR (i.e., NCP>0), marine ecosystem has a potential to absorb CO2 and export organic matters and is thus autotrophic. When NCP <0, the marine ecosystem emits CO2 and becomes a carbon source and is thus heterotrophic, and when NCP ≈ 0, the CO2 absorption and emission tends to be in equilibrium (Duarte and Regaudie-de-Gioux, 2009; Caffrey et al., 2014). The carbon metabolism of the plankton community that often regulates the distribution and variation of carbon flux in marine ecosystem usually influences the evaluations of the carbon cycle and nutrient dynamics in the oceans.
The metabolism status of the marine plankton community is susceptible to multiple factors and shows greatly spatial and temporal changes (Karl et al., 2003). Therefore, it is not surprising that the controversial results often occur among different regions and seasons depended on whether the marine ecosystem is autotrophic or heterotrophic (Ducklow and Doney, 2013; Serret et al., 2015). A famous controversy is about the judgment of autotrophy or heterotrophy in oligotrophic oceans (Duarte et al., 2013; Williams et al., 2013). Such a debate of metabolic status also occurs in estuaries or bays. Many studies suggested that the estuarine and nearshore areas are heterotrophic and are the sources of CO2 due to the land-derived inputs of excess organic carbon, while the continental shelves are autotrophic and the sinks of CO2 (Heip et al., 1995; Caffrey, 2004; Borges et al., 2005; Duarte and Prairie, 2005; Chen et al., 2013; Cloern et al., 2014). However, some recent studies showed that some estuaries, coastal and coral reef areas are autotrophic (Bauer et al., 2013; McKinnon et al., 2017; Agusti et al., 2018; Garcia Corral et al., 2020). Such a controversy demonstrates that the metabolic-balanced characteristics, and potential driving mechanisms need to be studied further in combination with specific biological and environmental factors because the regional estuarine and coastal ecosystems are usually affected by various human activities and environmental forces with a spatial variance.
As one of major urban agglomerations in the world, the Guangdong-Hong Kong-Macao Greater Bay Area (GGBA or Greater Bay Area) is one of the regions with the strongest economic vitality and fastest development in China. The coastal area of the GGBA covers the Pearl River Estuary (PRE) and its adjacent waters (Figure 1) and forms a coastal ecosystem with diverse habitats (estuary, bay, and offshore waters) and high biodiversity (Yang et al., 2019; Yu, 2019; Wang et al., 2021). Due to the inputs of nutrients and organic matters, the estuarine and nearshore waters are important junction of connecting land to open sea with active biochemical geochemical cycles (Gattuso et al., 1998). Previous studies showed that the greatly regional variances often occurred in physical and chemical environments of the PRE and its adjacent waters due to the Pearl River runoffs, human activities, and other physical processes (such as coastal current and coastal upwelling), which further affected the carbon fixation process of plankton assemblages (Cai et al., 2002; Song et al., 2004; Song et al., 2011). Many studies also showed that phytoplankton primary production and microbial metabolism mainly mediated the air–sea carbon flux in the PRE (Cai et al., 2004; Guo et al., 2009; Dai et al., 2014; Li et al., 2019), suggesting the importance of plankton metabolisms in this ecosystem. However, there are few studies on the couplings of the respiration and primary production of the plankton community in coastal waters of the GGBA, therefore, the balance of autotrophic and heterotrophic processes and their variations and regulation mechanisms are still unclear as the environments therein are very complicated.
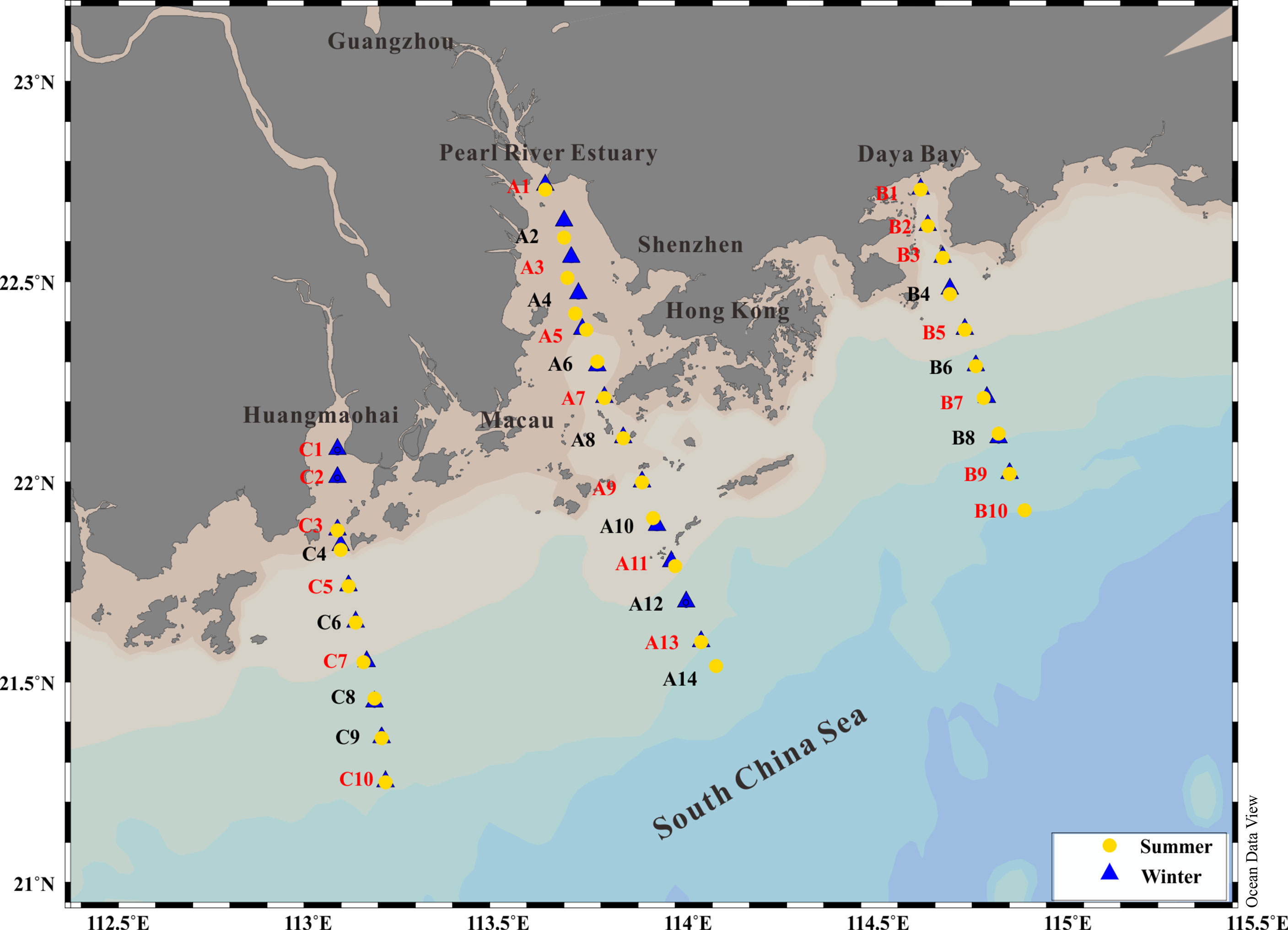
Figure 1 Map indicating the sampling stations during the summer (yellow dots; July 14–24, 2019) and winter periods (blue triangles; December 8–18, 2020). Stations marked in red show where the cell size-fractionated Chl a measurements and metabolic experiments (GPP, CR, and NCP) were carried out.
With rapid urbanization and economic growth, eutrophication, algal bloom, and hypoxia have become serious ecological and environmental problems in coastal waters of the GGBA (Yin et al., 2004a; Harrison et al., 2008; Song et al., 2009; Qian et al., 2018). As both algal bloom and hypoxia are closely related to the processes of plankton production and metabolism, studies on the balance between them and the influencing factors will help us to deeply understand the potential formation mechanism of these environmental and ecological events. Therefore, the GPP, CR, and NCP of the plankton community, as well as their associated environmental and biological factors were characterized in coastal waters of the GGBA during both wet (summer) and dry (winter) seasons, to clarify 1) the metabolic balance and carbon budget of plankton community in the GGBA and how the physical-environmental–biological factors shape their spatial and temporal variations; and 2) the relationship of plankton metabolic processes to the ecological risks of e.g., algal blooms and hypoxia events, as well as its potential indicative functions.
Material and Methods
Study Area and Sample Collection
In this study, we carried out the experiments in 3 transactions located in the PRE (A1-A14), Daya Bay (B1–B10), and Huangmaohai Bay (C1–C10) during wet (summer; July 14–24, 2019); and dry seasons (winter; December 8–18, 2020) (Figure 1). During the two investigations, the profiles of temperature, salinity, dissolved oxygen (DO), pH, turbidity, nutrients, and chlorophyll a (Chl a) concentration were obtained at all the 34 stations; and the cell size-fractionated Chl a, GPP, CR, and NCP in surface and bottom layers were measured at 20 of the 34 stations (labeled in red color). The seawater in the surface (~0.5 m depth), 5, 10, 15, 20, 30, 50 m, and bottom layers were collected with an acid-cleaned (1N HCl) 5-L polycarbonate bucket for Chl a and nutrient analysis as described below.
Environments and Chlorophyll a Measurements
At each station, the temperature, salinity, and DO profiles were measured with a multi-parameter water quality monitor Sonde (YSI 6600; Yellow Springs Instruments, Yellow Springs, OH, USA).
For nutrient analysis, the collected seawater from each depth was filtrated through 0.7 μm pore-sized glass fiber filters (25 mm, Whatman GF/F). The filtrate was then dispensed into an 80 ml polycarbonate bottle, frozen immediately, and stored at -20°C for subsequent analysis. Concentrations of , , , (DIP), and were measured with an automatic nutrient analyzer (AA3; Seal Instruments, Hamburg, Germany) using the traditional colorimetry methods (Hansen and Koroleff, 1999). In this study, the DIN concentration was calculated as the sum of , , and concentrations.
For the Chl a measurement, 250 ml of collected seawater was prefiltered through a 200 μm mesh and then sequentially filtrated through a 20 μm pore-sized nylon-net filter (25 mm, Millipore), 3 μm pore-sized polycarbonate filter (25 mm, Millipore), and 0.7 μm pore-sized glass fiber filter (25 mm, Whatman GF/F) to collect micro- (>20 μm), nano- (3–20 μm), and pico cell-sized phytoplankton cells (<3 μm), respectively. The total Chl a was obtained by directly filtrating the 200 μm pore-sized mesh-prefiltered seawater onto a 0.7 μm pore-sized GF/F filter. All the filters with phytoplankton cells were stored at -20°C for later analysis. The Chl a concentration was measured fluorometrically using a Turner Designs 10 fluorometer (Parsons et al., 1984) after extracting in the dark for 24 h at 4°C in 10 ml acetone (90% v/v).
NCP, CR, and GPP
To measure NCP and CR, the collected seawater was gently siphoned into 6 narrow-mouth 250 ml borosilicate glass Winkler bottles. Approximately 3 of the 6 bottles were wrapped in aluminum foil for dark incubation, and the remaining 3 replicates were incubated with the neutral cloth covered to achieve an in situ light level. The in situ light conditions were measured with the Secci disk method (Song et al., 2012). After recording the initial DO level with a high-precision fluorescent oxygen probe (Microx 4; PreSens, Regensburg, Germany), all the Winkler bottles were incubated for 24 h in a water tanker with running surface seawater to control temperature. After the incubations, the final DO concentration in each bottle was measured again, and the NCP and CR were calculated as
where [O2]t24 and [O2]t0 indicate the DO concentration at time 24 h and 0 in the dark or light bottles. The GPP was calculated as
GPP, CR, and NCP were converted to carbon units, assuming a photosynthetic quotient (PQ) of 1.3 and a respiratory quotient (RQ) of 1 (Gazeau et al., 2005; Hopkinson & Smith, 2005).
Statistical Analysis
Before doing analysis, the data were tested for normality (Shapiro–Wilk test) and the homogeneity of variance (Levene’s test). Then, the independent t test or Mann–Whitney test was used to identify the seasonal differences of environmental and metabolic factors. Principal component analysis (PCA) for environmental and biological factors was performed with CANOCO 5.0 software (Lepš and Šmilauer, 2003). Data were standardized to minimize the influence of the variances of variables, and the individual transformed observations were named scores (Wu et al., 2016). The GGBA was divided into several zones according to the scores of the first two principal components of each station as well as their actual geographical locations (Song et al., 2011); then, the regional differences of environments and metabolisms were identified between different zones. Tukey’s honestly significant difference (HSD) test was used to verify the significance of regional differences of environmental and biological factors, and the results were visualized using “ggplot2” package in R v4.0.5 (Ginestet, 2011; Zhao et al., 2021). Spearman correlation was used to determine the correlation of environment factors to plankton community metabolisms, and the results were visualized using the “ggcor” package in R v4.0.5 (Huang et al., 2020). An optimal multiple regression model was used to evaluate the contribution of environmental factors to the variation of plankton community metabolisms in R v4.0.5 (Grömping, 2006; Field et al., 2012). To estimate the importance of the variables, percentage increases in the mean squared error (MSE) were used: higher MSE% values indicate more important variables, according to the result of Spearman correlation analysis and the optimal multiple regression model with variance decomposition analysis in R v4.0.5 (Jiao et al., 2020). The map of sampling stations, spatial distribution, and transection plots was drawn using Ocean Data View (Schlitzer, 2020).
Results
Environmental Characteristics
In summer, the seawater stratification generally existed in the GGBA according to vertical distributions of salinity and temperature, and all the investigated areas in the PRE, Daya Bay, and Huangmaohai Bay showed clear stratification, especially in nearshore waters (Figures 2A, B). The isohaline of 20 psu extended to the distances of over 100 and 40 km in transections A and C, respectively (Figure 2B-1, -5), and the salinity was higher than 29 psu in transection B (Figure 2B-3). High DO and Chl a concentration generally appeared in the surface water , as well as in the offshore areas of transections A and B. Hypoxia events occurred in bottom of all three transections, with the lowest DO level of less than 2.2 mg L-1 in transection A (Figure 2C-1), less than 3.3 mg L-1 in transection B (Figure 2C-3), and less than 1.7 mg L-1 in transection C, respectively (Figure 2C-5). Moreover, the surface water with DIN levels of over 25 μmol L-1 extended to the distances of over 100 and 50 km far from the land in transections A and C, respectively (Figure 2E-1, -5). In winter, the water column was well mixed than that in summer. The isohaline of 20 psu shrank to the distances of less than 20 and 10 km far from the land in transections A and C, respectively (Figure 2B-2, -6). Consistently, the DO concentration distributed evenly in vertical scale and no hypoxia happened in the bottom layer, and Chl a concentration was lower than summer (Mann–Whitney test, p < 0.05) especially in nearshore areas (Figure 2D). In addition, the surface water with higher DIN level (>25 μmol L-1) in winter just extended to ~50 and ~30 km far from the land in transections A and C, respectively (Figure 2E-2, -6).
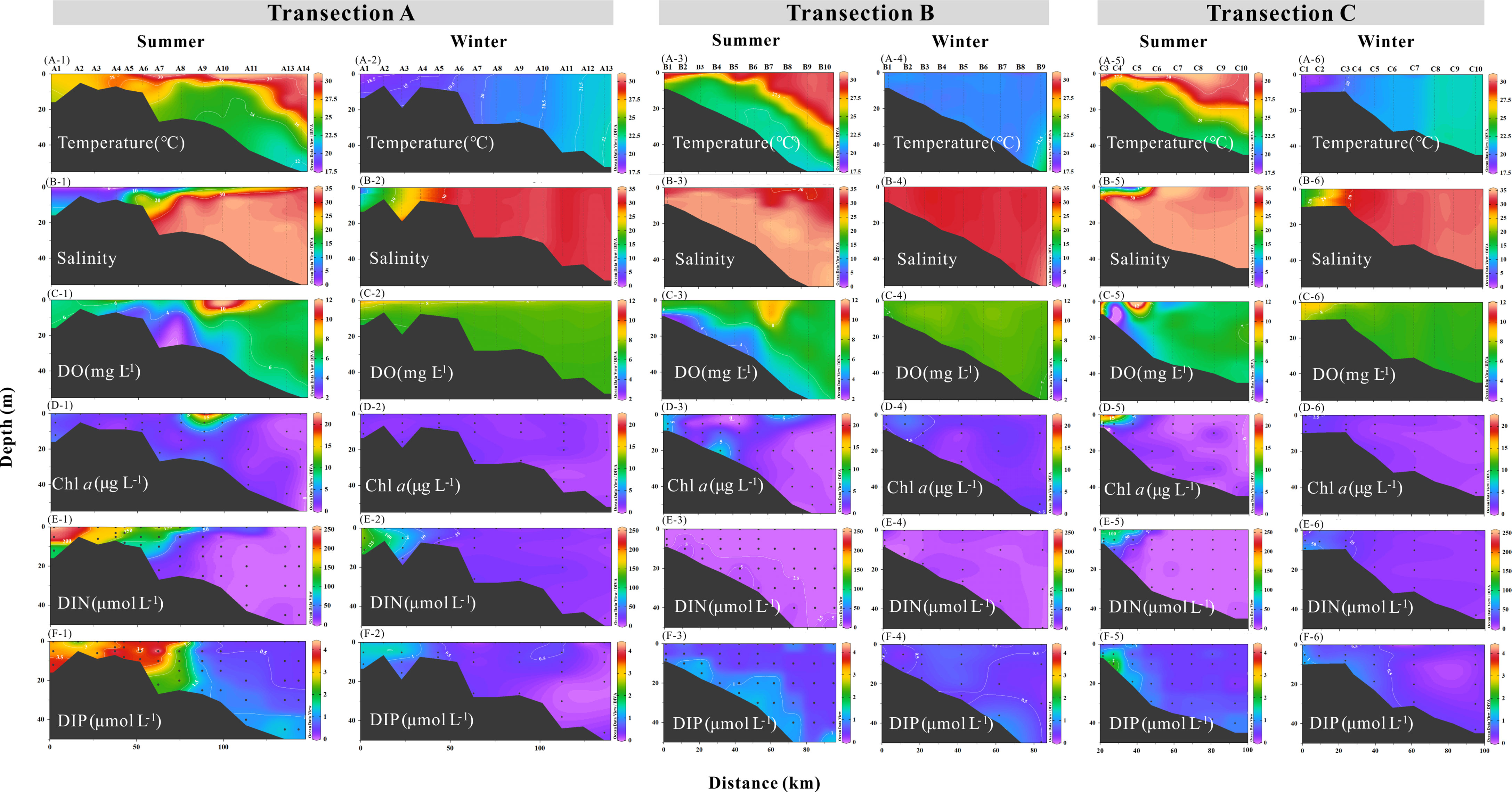
Figure 2 Vertical distributions of temperature (A, °C), salinity (B), DO (C, mg L-1), Chl a concentration (D, μg L-1), DIN (E, μmol L-1), and DIP (F, μmol L-1) along the transections A, B, and C in summer and winter periods.
In general, nano-phytoplankton (3–20 µm) dominated (~60%) both the surface and bottom layers of nearshore waters of all three transections during summer and winter periods, while the pico-phytoplankton (<3 µm) dominated (~50%) both layers of the offshore waters. Moreover, contribution of nano-Chl a to total Chl a decreased offshore-ward, while that of pico-Chl a increased. The contribution of micro-Chl a (>20 µm) to total Chl a (i.e., varying from 3.17% to 38%) showed no clear spatial change from nearshore to offshore waters (Figure 3).
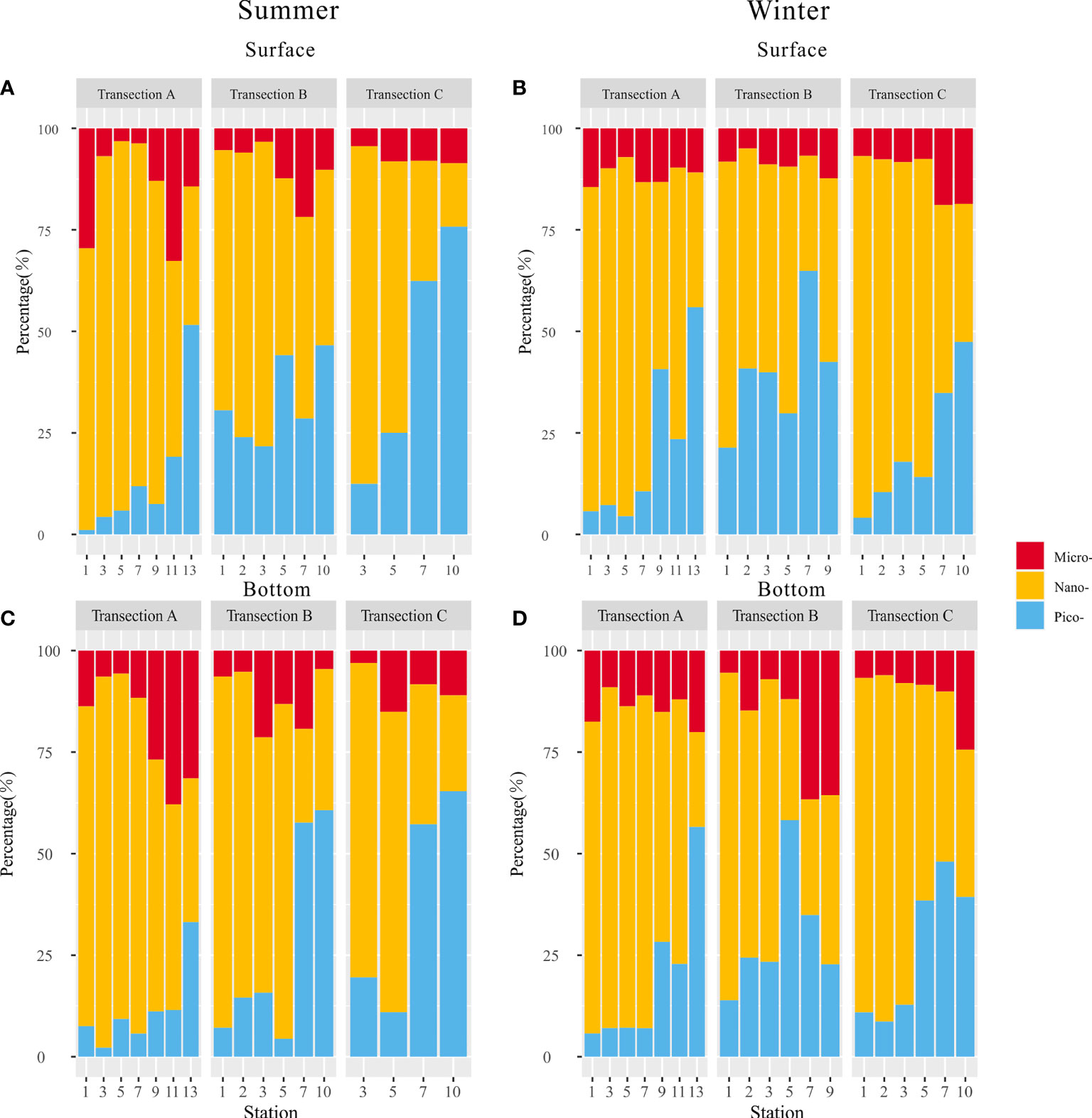
Figure 3 Contribution (%) of three cell size-fractioned Chl a in surface and bottom seawater along transections (A–C) of the GGBA in summer (A–C) and winter (B, D) periods.
Plankton Community Metabolisms
Coinciding with Chl a biomass, the GPP varied from 52.3 to 661 mg C m-3 d-1 in surface water during the summer period and decreased from nearshore to offshore waters (Figure 4A-1). The GPP reached as high as 661 mg C m-3 d-1 in estuarine–offshore waters, and such high GPP waters extended from southeast to northwest of the investigated area. In winter, however, the GPP ranged from 19.2 to 303 mg C m-3 d-1 and was significantly lower than that in summer (Mann–Whitney test, p < 0.05). The highest GPP (i.e., 303 mg C m-3 d-1) was observed in nearshore waters of the Daya Bay (Figure 4A-2). Moreover, the GPP in the bottom layer was very low in both summer and winter periods (i.e., summer, 19.5 ± 4.5 mg C m-3 d-1; winter, 8.9 ± 1.5 mg C m-3 d-1), and displayed less spatial variation than that in surface water (Figure 4A-3, -4).
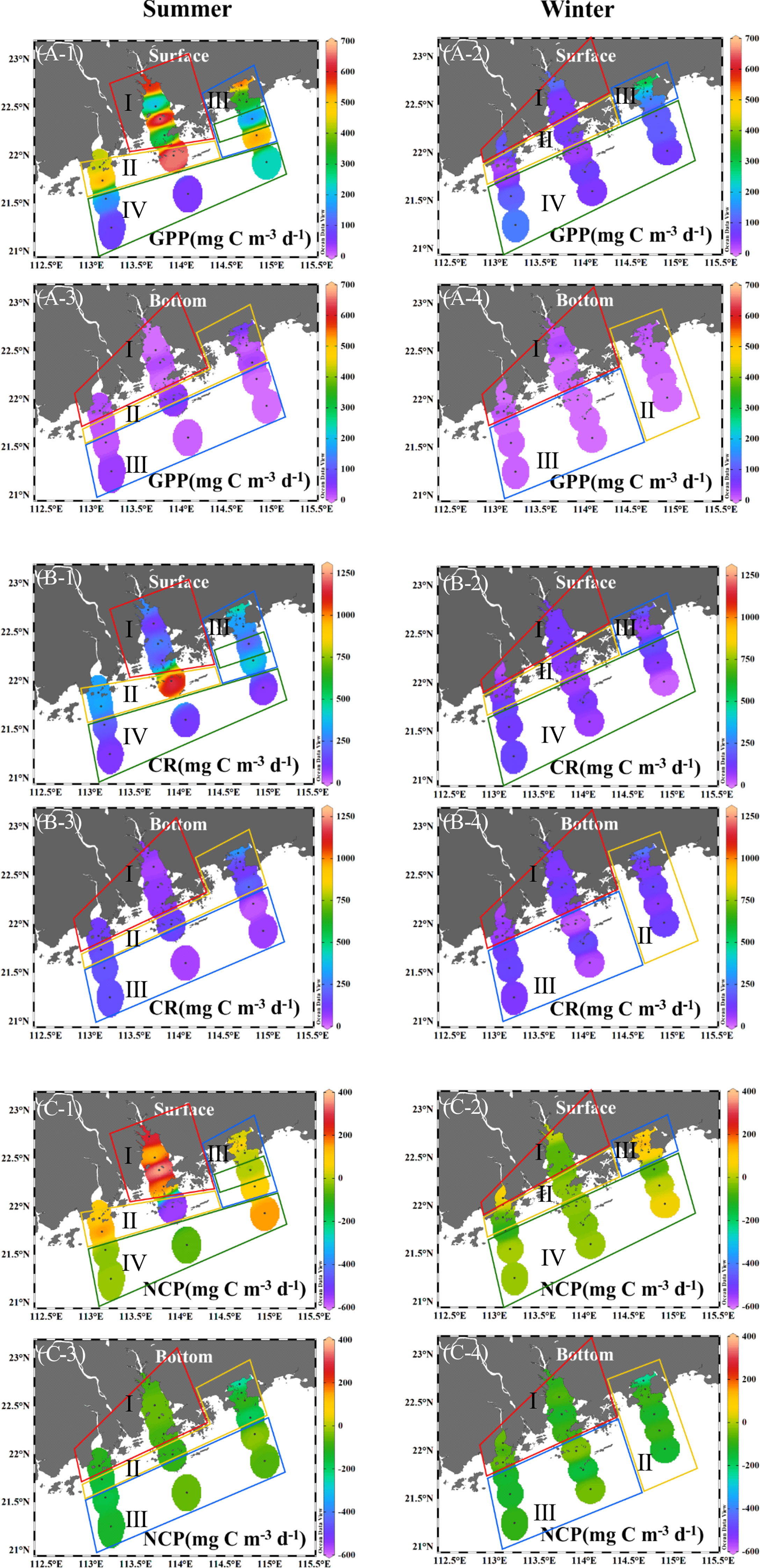
Figure 4 Distribution characteristics of GPP (A, mg C m-3 d-1), CR (B, mg C m-3 d-1), and NCP (C, mg C m-3 d-1) in surface and bottom seawater along transections (A-C) of the GGBA in summer and winter periods. The clusters divided here are consistent with the clusters shown in Figure 5.
Similarly, surface CR varied from 65.0 to 1,213 mg C m-3 d-1 in summer, and the active CR co-occurred with high GPP and Chl a, e.g., the CR reached up to 1,213 mg C m-3 d-1 in the interface of plume- and saline-waters, where the GPP and Chl a reached as high as 659 mg C m-3 d-1 and 21.7 μg L-1, respectively (Figure 4B-1). In winter, the surface CR varied from 20.0 to 210 mg C m-3 d-1 (Figure 4B-2). As compared to surface water, the CR in the bottom layer was markedly lower in summer (Mann–Whitney test, p < 0.05); however, such a difference did not occur in winter (Figure 4B-3, -4) and no significant difference occurred between summer and winter periods either (i.e., 131 ± 79.9 vs. 110 ± 51.4 mg C m-3 d-1).
The NCP in surface water ranged from -554 to 377 mg C m-3 d-1 in summer and showed a similar spatial change as the GPP and CR (Figure 4C-1). The highest NCP presented in inner PRE, while the lowest appeared in the interface of plume- and saline- water. In winter, surface NCP varied from -113 to 141 mg C m-3 d-1 and was lower than that in summer (Mann–Whitney test, p < 0.05) (Figure 4C-2). The NCP in the bottom layer appeared to be negative in both summer and winter periods (Figure 4C-3, -4), with the lowest values of -245 mg C m-3 d-1 in summer and of -241 mg C m-3 d-1 in winter presented in the Daya Bay. Moreover, the surface water of the GGBA was generally autotrophic in summer , and was close to the metabolic balance in winter; while the bottom water was heterotrophic in both investigated periods (Figure 4C).
Principal Component Analysis Results on Regional Variances
Based on the PCA results in surface water as well as the geographical locations, the investigated area of the GGBA was divided into four regional zones (Figures 5A, B). In summer, Zone I that is located in the PRE had lower salinity and higher DIP, DIN, and concentrations (Figures 6B, D, E; Table S1). Zone II had intermediate salinity between Zone I and Zone III or IV, as well as nutrient content. Moreover, Zone II had the highest DO and Chl a concentration among all four zones, wherein the GPP was higher as well (Figures 6C, F, J). Zones III and IV located in the coastal area of the Daya Bay and the far-offshore area of the GGBA respectively, and had higher salinity and lower nutrient loadings than Zones I and II (Figures 6B, D, E; Table S1). Zone III had lower temperature but higher DIN, DIP, and , as compared to Zone IV (Figures 6A, D, E; Table S1). Moreover, pico-Chl a allocation in Zone III was higher than Zones I and II but lower than Zone IV (Figure 6I-1). The proportion of nano-phytoplankton in Zone IV was significantly lower, as compared to other zones (Figure 6H-1). In addition, Chl a, GPP, and CR in Zone IV were lowest among all zones of the GGBA (Figures 6F, J, K; Table S1).
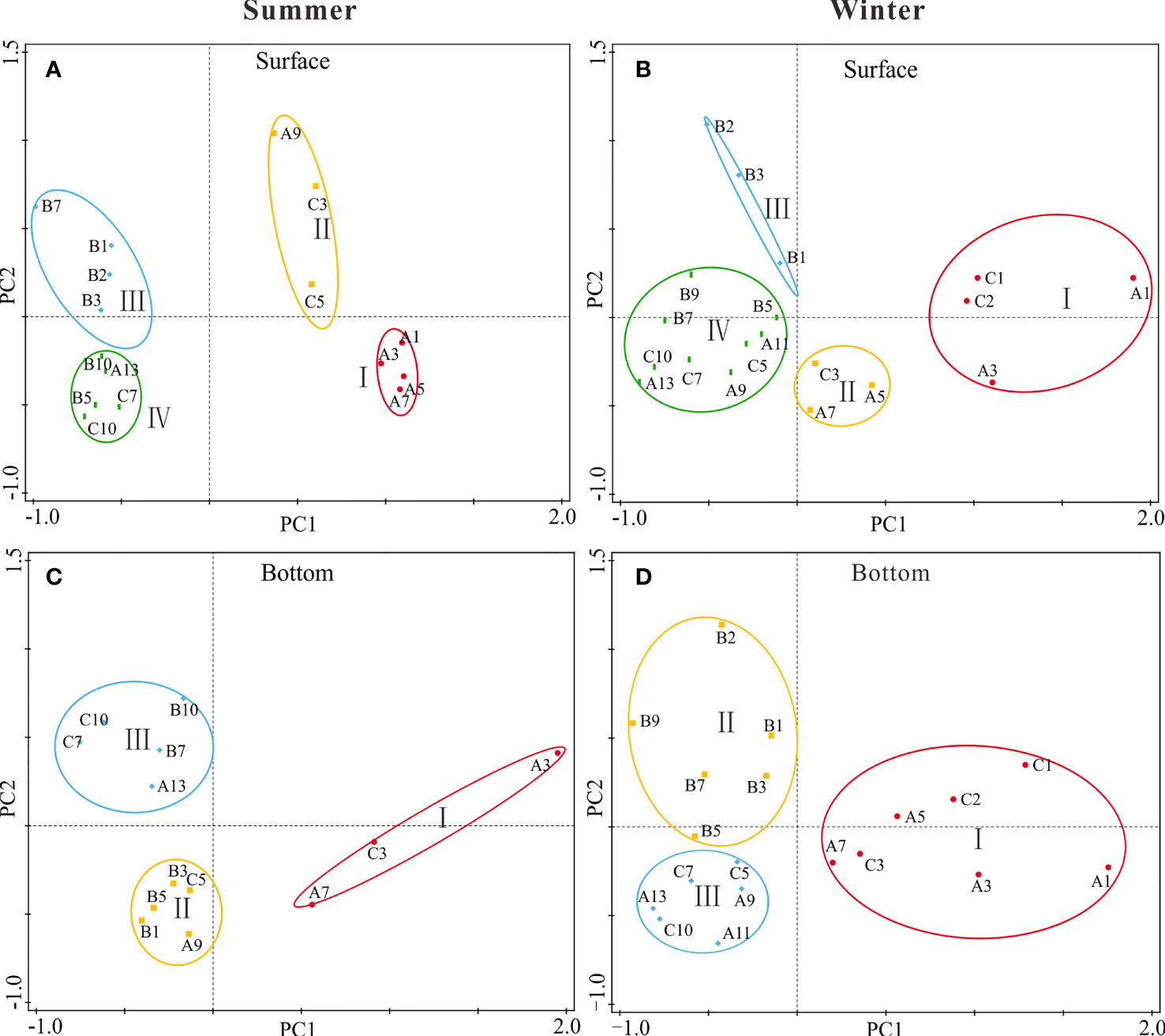
Figure 5 PCA results of environmental and biological characteristics in surface (A, B) and bottom (C, D) seawater along transections (A–C) of the GGBA in summer (A, C) and winter (B, D) periods.
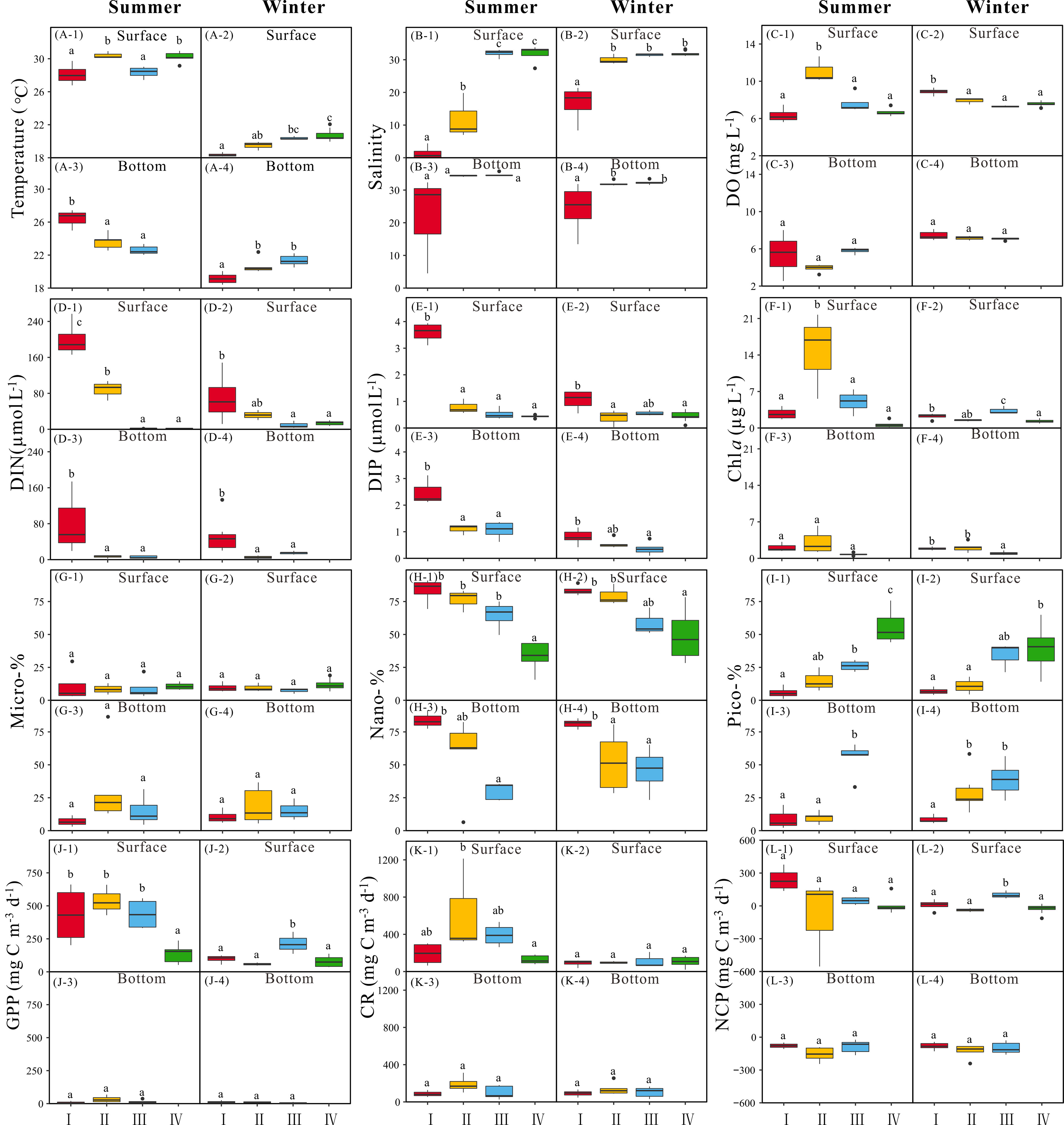
Figure 6 Regional variance of temperature (A, °C), salinity (B), DO (C, mg L-1), DIN (D, μmol L-1), DIP (E, μmol L-1), Chl a concentration (F, μg L-1), Micro-% (G), Nano-% (H), Pico-% (I), GPP (J, mg C m-3 d-1), CR (K, mg C m-3 d-1) and NCP (L, mg C m-3 d-1) in surface (-1, -2) and bottom (-3, -4) layer along transections of the GGBA in summer (-1, -3) and winter (-2, -4) periods. Different letters in each box plot indicate the significant differences among different groups (Turkey' HSD test, p < 0.05)
In winter, the surface water of Zone I is located near the PRE and Huangmaohai Bay and covered a narrower area than summer, and was closer to the upper reaches of the PRE. The salinity in Zone I was significantly lower than that in other zones (Figure 6B-2), while the DIP, DIN, and concentrations were significantly higher (Figures 6D, E; Table S1) but with moderate Chl a and lower GPP and NCP (Figures 6F, J, L). Zone II covered the interface area of fresh- and saline-water and was closer to the coast, as compared to summer. The salinity in Zone II was lower than that of Zone III or IV, and the DIN was higher than that of Zone III and IV; however, the DIP was lower, leading to the higher N:P ratio (~82) than that in Zone III (~13) or IV (~31) (Figures 6B, D, E; Table S1). Surface Chl a in Zone II appeared to be moderate, just higher than Zone IV but lower than Zone III (Figure 6F-2). The GPP and NCP in Zone II were lower either, while the CR was similar to the other zones (Figures 6J, K, L). As compared to summer, Zone III in winter just covered the nearshore area of the Daya Bay, wherein the salinity, temperature, and DIP were higher than Zone II or IV (Figures 6A, B, E). The Chl a, GPP, and NCP in Zone III were significantly higher than other zones (Figures 6F, J, L). Zone IV that covered a wider range of the offshore area in winter than summer had higher temperature and salinity than other zones but lower DIP, DIN and concentrations. Moreover, Zone IV had the lowest Chl a, GPP, and NCP among all zones in winter (Figure 6; Table S1).
In contrast to surface water, the bottom water that generally had higher salinity and lower temperature (Figures 6A, B), as well as lower Chl a, GPP, CR, and NCP (Figures 6F, J, K, L), was divided into three zones in both summer and winter periods (Figures 5C, D). In summer, Zone I that is located in the inner parts of the PRE and Huangmaohai Bay had higher temperature and nutrients, as well as lower salinity (Figures 6A, B, D, E; Table S1). Zone II covered the inner part of the Daya Bay and the interface area of fresh- and saline-water, wherein it had the lowest DO among all three zones (Figure 6C-3). Nano-phytoplankton dominated Zone II, wherein GPP and CR were highest and NCP was most negative (Figures 6H, J, K, L). Zone III covered the offshore waters off Zone II and was dominated by pico-phytoplankton and characterized with lower nutrients and Chl a (Figures 6I, D, E, F). Moreover, the GPP and CR in Zone III were low too, and the NCP was even closer to zero (Figures 6J, K, L).
In winter, the bottom water of Zone I covered similar area as in summer, and had the highest nutrients concentration and lowest temperature and salinity among all three zones (Figures 6A, B, D, E). Moreover, the Chl a and NCP in Zone I were higher than Zone II or III, and nano-phytoplankton dominated therein (Figures 6F, H, L). Zone II is located in the Daya Bay and covered its offshore area, but did not cover the coastal waters of the PRE and Huangmaohai Bay. Zone III covered the offshore waters of the investigated area except that off the Daya Bay. Both the Zones II and III were characterized with the higher temperature and salinity than Zone I, as well as lower nutrients (Figures 6A, B, D, E). The pico-Chl a allocation was higher in these two zones (Figure 6I-4). Finally, the Chl a biomass in Zone II was higher than that in Zone III, while the proportion of pico-Chl a was lower (Figure 6; Table S1).
Discussion
Regulation of Plankton Community Metabolisms
The environmental factors that influence the production and consumption of plankton community include temperature, light, nutrients, and turbidity (Rochelle-Newall et al., 2007; Kemp and Testa, 2011). Previous studies showed that temperature and nutrients are key factors to regulate the primary production and community respiration of the estuaries and their adjacent coastal areas (Caffrey, 2004; Caffrey et al., 2014; Hung et al., 2014). Consistently, in this study temperature and nutrients were identified as the key environmental factors for regulating the metabolisms of the plankton community of the GGBA, although the extent of these regulations on the GPP and CR differed greatly. According to the optimal regression model, field temperature explained more variance of the surface CR as compared to other variables, while the nutrients explained more of the GPP (Figure 7). Furthermore, the surface NCP was positively correlated to GPP (p < 0.01), but not to CR (p > 0.05) (Table S2), again indicating that the nutrients regulate the GPP and thus vary the NCP of the surface waters of GGBA. However, such differences in regulatory factors between primary production and respiratory metabolism were not obvious in the bottom waters, since the multiple regression analysis result suggests the similar key environmental factors explaining the variation of GPP and CR, both of which are significantly correlated with NCP (p < 0.01) (Figure 7; Table S2).
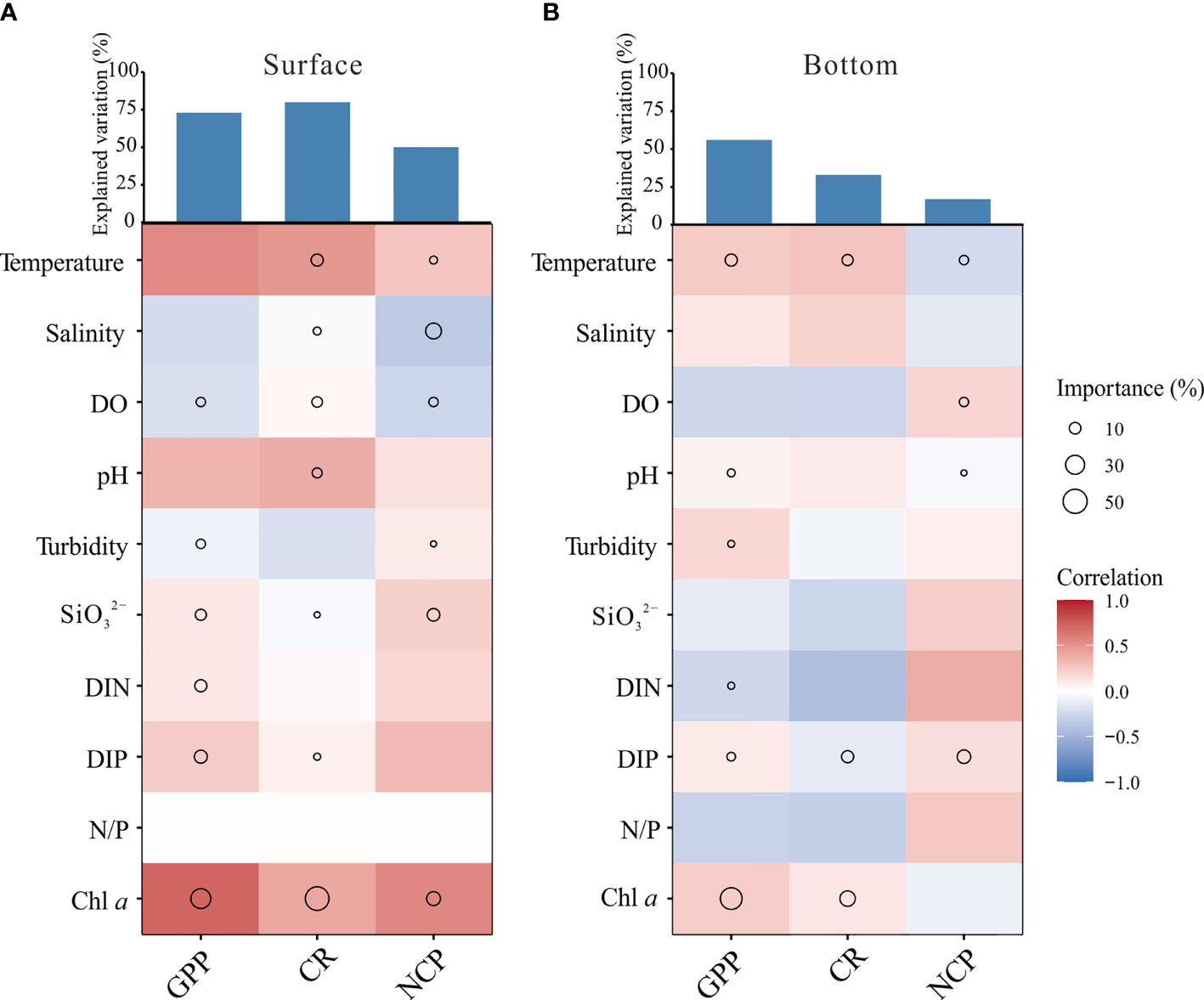
Figure 7 Contribution of environmental factors to variation of GPP, CR, and NCP based on optimal multiple regression model in (A) surface and (B) bottom layers. The above bar graph indicates the total contribution, and the heat plot indicates the Spearman correlation coefficients. The size of circles indicates the importance of environmental variables.
Coastal ecosystems of the GGBA are usually influenced by the Pearl River runoffs, Yuedong Coastal Current, and intruded oligotrophic oceanic water from the South China Sea (Yin et al., 2004a; Harrison et al., 2008; Song et al., 2011; Zu et al., 2014; Zu and Gan, 2015). Together with the influences of monsoon, tide, topography, and anthropogenic inputs, the metabolic processes of plankton community in this area varied greatly in spatial and seasonal scales (Figure 4). In this case, the regional analysis would be helpful to get a clear understanding about the complex relationship between community metabolism and driving forces in each regions. According to the PCA results, the GGBA was divided into different zones (Figure 5), corresponding to the different extent of the comprehensive effects of driving factors like the Pearl River runoffs, anthropogenic activities, and offshore water currents. These regional differences are more obvious in the upper water layer due to the generally higher and more various impacts of the driving factors therein (Figure 6; Table S1; Song et al., 2011; Dai et al., 2014; Zu et al., 2014).
The upper reach of the PRE (Zone I) was mainly influenced by the runoffs of Pearl River, with the influence range extending more seaward in summer. This region had the highest nutrient level but not highest Chl a and GPP (Figure 6; Table S1), probably due to the restriction of solar irradiation caused by the high turbidity and low transparency and disturbance therein (Lu and Gan, 2015). However, the metabolic state of this region was generally autotrophic in summer, inconsistent to other eutrophic estuaries where they were usually reported to be heterotrophic (Ducklow and McCallister, 2004; Caffrey et al., 2014), which may be explained by the greater negative effect of high disturbance upon the CR than the GPP (Alizadeh et al., 2018).
The river plume-dominated area (Zone II) often expands wider in summer due to the increased land-derived runoffs (Figure 2; Zu and Gan, 2015; Zhao et al., 2020) and was characterized with high Chl a and primary production (Figure 6; Table S1), consistent with previous studies (Yin et al., 2000; Yin et al., 2004b; Harrison et al., 2008; Song et al., 2011; Lu and Gan, 2015). Nutrients in this area are plentiful, and the restrictions of temperature, turbidity, and so on are less, probably leading to the higher primary production and plankton respiration (Figure 4A, B). In particular, the surface CR in this region is close to or even higher than the GPP, which may be attributed to the active metabolic decomposition during the post-algal bloom period (Figures 2, 4; Table S1; Buchan et al., 2014; Wemheuer et al., 2014), thus resulting in the low NCP and even a partially heterotrophic state.
The eastern nearshore area of the GGBA (Zone III) was less affected by the runoffs of Pearl River. So, the nutrient sources of this region are mainly taken from anthropogenic activities and terrigenous inputs, as well as the vertical upwelling in a certain period of rainy season (Song et al., 2015; Wu et al., 2017; Zhang et al., 2019), which may increase the GPP and CR to some extent. Moreover, the metabolic process in this zone was more active than the upper reaches of the PRE as well, possibly due to low turbidity. This coastal water showed an obvious autotrophic state in its surface layer in both summer and winter, being consistent with other coastal waters of e.g., the coastal waters of the Red Sea and the northern Australia (McKinnon et al., 2017; López-Sandoval et al., 2019). The far-offshore region (Zone IV), where had low GPP and CR, is understandable due to the offshore oligotrophic water with low nutrients and organic matters. In addition, a weak heterotrophy occurred in this region, indicating the primary production could not satisfy the requirements of community metabolism. It is consistent with the results from the similar oligotrophic oceanic waters (Duarte et al., 2013; Regaudie-de-gioux and Duarte, 2013).
The bottom water in the GGBA was less influenced by the Pearl River runoffs, and thus more stable than surface water, with the environmental factors varying less in both temporal and spatial scales (Figures 2, 6; Zu et al., 2014). Due to lacking solar light, the GPP therein was low; so, the regional variation of NCP could mainly be dependent on the CR (Table S2). Moreover, efficient transport of organic and inorganic nutrients via terrestrial inputs or downward depositions from the high-GPP upper water may make the community metabolism more active in nearshore areas (Ke et al., 2019; Dan et al., 2021), while in bottom of the offshore waters, the community metabolism was less active (Figure 6; Table S1), probably resulting from the lacking nutrient inputs.
The Greater Bay Area covers the coastal areas with spatially environmental diversity. The corresponding spatial variations of key environmental factors, such as nutrient supply, turbidity and temperature lead to the regional complexity of plankton metabolic processes. According to the water-column integrated data, the plankton community metabolisms in winter shifted from heterotrophy in upper reaches of the estuary to autotrophy in nearshore waters and back to heterotrophy again in offshore waters, consistent with other coasts (Gattuso et al., 1998; Ducklow and McCallister, 2004). In summer however, the expansion of river plume led to a large-scale heterotrophy in coastal waters off the PRE accompanying with an algal bloom, which has seldom been reported before. This is possibly because that the heterotrophic processes during the algal bloom are more active than primary production, thus leading to the negative state of metabolic balance (Song et al., 2021).
Plankton Metabolisms as Indicators of Ecosystem Health and Carbon Budget
The metabolic status of the plankton community is an important indicator for the health and carbon budget of an ecosystem. Liu et al. (2012) reported the close relationship between high primary production and algal blooms in nearshore waters of the Daya Bay and suggested the primary production as an index of coastal eutrophication. Similarly, here, an obviously high GPP was associated with an algal bloom [featured as maximum Chl a >20 μg L-1, more than twice of the criterion value described by He et al. (2013)] in the Pearl River plume during summer period (Figures 2, 4). Previous studies showed that this area is conducive to the primary production process and biomass accumulation due to the large amount of nutrient inputs from river runoffs, as well as the more stable water column in summer, resulting in the frequent records of algal blooms (Yin et al., 2000; Yin et al., 2004b; Harrison et al., 2008; Song et al., 2011; Lu and Gan, 2015; Alizadeh et al., 2018). In contrast, the upper reaches of the estuary are less likely to form algal blooms, since phytoplankton production therein are usually limited by turbidity and river-flow disturbance.
Hypoxia often occurs in the bottom water of the GGBA in summer, such as in the PRE and Daya Bay (Yin et al., 2004a; Song et al., 2015; Li et al., 2018; Lu et al., 2018; Qian et al., 2018). In summer, obvious stratification often forms in estuarine and coastal waters, which may block the transportation of oxygen from upper to bottom layers and thus hydrologically increase the probability of hypoxia (Figure 2; Lu and Gan, 2015; Zhao et al., 2020). On the other hand, high production in the upper layer usually enhances the downward transportation of organic carbon, which may have favored the community respiration in the bottom layer, and thus led to the negative NCP value and heterotrophy therein (Li et al., 2019). Consistently, the higher bottom CR, as compared to its adjacent waters, occurred around the high surface GPP area within the river plume (Figure 4). In addition, the benthic respiration (mainly microbial metabolism) may also benefit from the downward transportation of excess organic matters that are associated with high primary production in surface water (Aller et al., 2008; Zhu et al., 2011). Therefore, the high oxygen consumption in summer due to the active community metabolism and long-term stratification may result in the low DO in bottom water (Figure 2). It is also suggested that the formation of hypoxic zone is closely related to the massive growth and reproduction of surface phytoplankton cells (Qian et al., 2018). More interestingly, the bottom hypoxia zone in the estuary was not rightly located under the areas with high surface Chl a and primary production but under its adjacent area (Figure 2). This can be explained by the saltwater wedge effect that has transported the sinking organic matters to the nearby area through the current intrusion in lower or bottom layer (Hu and Li, 2009). However, this is not the case in the Daya Bay because there is no such wedge effect (Figure 2). The hypoxia seldom happened in winter either (Figure 2), possibly due to the strong vertical mixing caused by northeastern monsoon that has transported the high DO surface water to the bottom layer and thus enhanced the DO level therein. The lower temperature in winter may be another cause through enhancing oxygen solubility and lowering respiration metabolic rate.
A previous study suggested that annual sea–air CO2 exchange near the PRE was close to equilibrium, and the CO2-absorbing capacity was enhanced in summer (Zhai et al., 2013). In this study, the primary production and community respiration in the GGBA is close to be balanced in both winter and summer, and NCP is also higher in summer (Mann-Whitney test, p < 0.05), indicating the high similarity and potential correlation between the community metabolic carbon flux and carbon budgets. Moreover, the specific distribution of plankton community carbon budget varied greatly in spatial and temporal scales, making it difficult to exactly evaluate the detailed carbon flux. It is generally believed that the upstream of estuaries is the source of CO2, while the waters controlled by a river plume is the sink due to the high primary production (Guo et al., 2009; Dai et al., 2014). In this study however, the metabolic respiration appeared to be more active than primary production in river plume in summer, probably contributing to the carbon export of this area. In the eastern coastal part of the GGBA, the plankton community metabolisms were much less affected by the Pearl River runoffs, making this area appear to be a carbon source in both winter and summer (Figure 4). This heterotrophy also existed in the nearshore waters of the Daya Bay during a certain summer period (Li et al., 2021), suggesting the complication of ecosystem carbon flux even in a small-scaled area like the GGBA due to its temporal and spatial variations of the impacts of anthropogenic activities and other environmental factors.
In conclusion, we found that the field plankton community metabolisms in the GGBA showed a great regional difference in both summer and winter periods, interactively influenced by the physical–environmental–biological factors. The surface water of the GGBA exhibited autotrophic in summer, while such an autotrophic in summer status was weaker in winter; however, the bottom water exhibited heterotrophic in both summer and winter periods. Our results also showed that the high surface GPP and bottom CR can be considered as the important indicators for the algal blooms and hypoxia events that happened during that time in the GGBA. Further studies on the dynamic variations of plankton metabolism and its driving mechanisms are necessary for an in-depth understanding of the function of coastal ecosystem and better assessment and management of potential ecological risks.
Data Availability Statement
The original contributions presented in the study are included in the article/Supplementary Material. Further inquiries can be directed to the corresponding author.
Author Contributions
LZ, XS, and GL designed the experiments and analyzed the data. CX, YH, XF, and CZ conducted the shipboard sampling and environmental measurements. LZ and YH performed the in situ plankton metabolism measurements. ZW and ZO conducted nutrient measurements. LZ, XS, GL, and CX wrote, discussed, and edited the paper. All authors contributed to the article and approved the submitted version.
Funding
This study was supported by National Natural Science Foundation under contract No. 41890853; Southern Marine Science and Engineering Guangdong Laboratory (Guangzhou) under contract No. GML2019ZD0404; Science & Technology Basic Resources Investigation Program of China under contract No. 2018FY100105; Innovation Academy of South China Sea Ecology and Environmental Engineering, Chinese Academy of Sciences (NO. ISEE2018ZD02); the National Key Basic Research Program of China (973 program, No. 2015CB452904), Development Project of China under contract Nos 2017YFC0506302 and 2016YFC0502805.
Conflict of Interest
The authors declare that the research was conducted in the absence of any commercial or financial relationships that could be construed as a potential conflict of interest.
Publisher’s Note
All claims expressed in this article are solely those of the authors and do not necessarily represent those of their affiliated organizations, or those of the publisher, the editors and the reviewers. Any product that may be evaluated in this article, or claim that may be made by its manufacturer, is not guaranteed or endorsed by the publisher.
Supplementary Material
The Supplementary Material for this article can be found online at: https://www.frontiersin.org/articles/10.3389/fmars.2022.844970/full#supplementary-material
Supplementary Table 1 | Characteristics of environment and plankton community metabolism in different zones of the GGBA.
Supplementary Table 2 | Spearman correlation index of plankton community metabolic parameters and ecological environmental factors in the GGBA * indicates p < 0.05; ** indicates p < 0.01.
References
Agusti S., Vigoya L., Duarte C. M. (2018). Annual Plankton Community Metabolism in Estuarine and Coastal Waters in Perth (Western Australia). PeerJ 6, e5081. doi: 10.7717/peerj.5081
Alizadeh M. J., Kavianpour M. R., Danesh M., Adolf J., Shamshirband S., Chau K. W., et al. (2018). Effect of River Flow on the Quality of Estuarine and Coastal Waters Using Machine Learning Models. Eng. Appl. Comput. Fluid. Mech. 12, 810–823. doi: 10.1080/19942060.2018.1528480
Aller R. C., Blair N. E., Brunskill G. J. (2008). Early Diagenetic Cycling, Incineration, and Burial of Sedimentary Organic Carbon in the Central Gulf of Papua (Papua New Guinea). J. Geophys. Res. 113, F01S09. doi: 10.1029/2006jf000689
Bauer J. E., Cai W. J., Raymond P. A., Bianchi T. S., Hopkinson C. S., Regnier P. A. (2013). The Changing Carbon Cycle of the Coastal Ocean. Nature 504, 61–70. doi: 10.1038/nature12857
Borges A. V., Delille B., Frankignoulle M. (2005). Budgeting Sinks and Sources of CO2 in the Coastal Ocean: Diversity of Ecosystems Counts. Geophys. Res. Lett. 322019, 1–4. doi: 10.1029/2005gl023053
Buchan A., Lecleir G. R., Gulvik C. A., González J. M. (2014). Master Recyclers: Features and Functions of Bacteria Associated With Phytoplankton Blooms. Nat. Rev. Microbiol. 12, 686–698. doi: 10.1038/nrmicro3326
Caffrey J. M. (2004). Factors Controlling Net Ecosystem Metabolism in U.S. Estuaries. Estuaries 27, 90–101. doi: 10.1007/bf02803563
Caffrey J. M., Murrell M. C., Amacker K. S., Harper J. W., Phipps S., Woodrey M. S. (2014). Seasonal and Inter-Annual Patterns in Primary Production, Respiration, and Net Ecosystem Metabolism in Three Estuaries in the Northeast Gulf of Mexico. Estuar. Coast. 37, 222–241. doi: 10.1007/s12237-013-9701-5
Cai W.-J. (2011). Estuarine and Coastal Ocean Carbon Paradox: CO2 Sinks or Sites of Terrestrial Carbon Incineration? Ann. Rev. Mar. Sci. 3, 123–145. doi: 10.1146/annurev-marine-120709-142723
Cai W.-J., Dai M., Wang Y., Zhai W., Huang T., Chen S., et al. (2004). The Biogeochemistry of Inorganic Carbon and Nutrients in the Pearl River Estuary and the Adjacent Northern South China Sea. Cont. Shelf. Res. 24, 1301–1319. doi: 10.1016/j.csr.2004.04.005
Cai Y., Ning X., Liu Z. (2002). Studies on Primary Production and New Production of the Zhujiang Estuary, China. Acta Oceanol. Sin. 24, 101–111.
Chen C.-T., Huang T.-H., Chen Y.-C., Bai Y., He X., Kang Y. (2013). Air–sea Exchanges of CO2 in the World's Coastal Seas. Biogeosciences 10, 6509–6544. doi: 10.5194/bg-10-6509-2013
Cloern J. E., Foster S. Q., Kleckner A. E. (2014). Phytoplankton Primary Production in the World's Estuarine-Coastal Ecosystems. Biogeosciences 11, 2477–2501. doi: 10.5194/bg-11-2477-2014
Dai M., Gan J., Han A., Kung H., Yin Z. (2014). “Physical Dynamics and Biogeochemistry of the Pearl River Plume,” in Biogeochemical Dynamics at Large River–Coastal Interfaces. Eds. Bianchi T., Allison M., Cai W. J. (Cambridge University Press, Cambridge), 321–352. doi: 10.1017/CBO9781139136853
Dan S. F., Li S., Yang B., Cui D., Ning Z., Huang H., et al. (2021). Influence of Sedimentary Organic Matter Sources on the Distribution Characteristics and Preservation Status of Organic Carbon, Nitrogen, Phosphorus, and Biogenic Silica in the Daya Bay, Northern South China Sea. Sci. Tot. Environ. 783, 146899. doi: 10.1016/j.scitotenv.2021.146899
Duarte C. M., Prairie Y. T. (2005). Prevalence of Heterotrophy and Atmospheric CO2 Emissions From Aquatic Ecosystems. Ecosystems 8, 862–870. doi: 10.1007/s10021-005-0177-4
Duarte C. M., Regaudie-De-Gioux A. (2009). Thresholds of Gross Primary Production for the Metabolic Balance of Marine Planktonic Communities. Limnol. Oceanogr. 54, 1015–1022. doi: 10.4319/lo.2009.54.3.1015
Duarte C. M., Regaudie-De-Gioux A., Arrieta J. M., Delgado-Huertas A., Agustí S. (2013). The Oligotrophic Ocean Is Heterotrophic. Ann. Rev. Mar. Sci. 5, 551–569. doi: 10.1146/annurev-marine-121211-172337
Ducklow H. W., Doney S. C. (2013). What Is the Metabolic State of the Oligotrophic Ocean? A Debate. Ann. Rev. Mar. Sci. 5, 525–533. doi: 10.1146/annurev-marine-121211-172331
Ducklow H. W., McCallister S. L. (2004). “The Biogeochemistry of Carbon Dioxide in the Coastal Oceans,” in The Sea, Volume 13, The Global Coastal Ocean-Multiscale Interdisciplinary Processes. Eds. Robinson A. R., Brink K., Wiley J., Sons (New York: Harvard University Press).
Falkowski P. G. (1998). Biogeochemical Controls and Feedbacks on Ocean Primary Production. Science 281, 200–206. doi: 10.1126/science.281.5374.200
García Corral L. S., Agusti S., Duarte C. M. (2020 1142). Plankton Community Metabolism in Western Australia: Estuarine, Coastal and Oceanic Surface Waters. Front. Mar. Sci. 7. doi: 10.3389/fmars.2020.582136
Gattuso J. P., Frankignoulle M., Wollast R. (1998). Carbon and Carbonate Metabolism in Coastal Aquatic Ecosystems. Ann. Rev. Ecol. Syst. 29, 405–434. doi: 10.1146/annurev.ecolsys.29.1.405
Gazeau F., Borges A. V., Barrón C., Duarte C. M., Iversen N., Middelburg J. J., et al. (2005). Net Ecosystem Metabolism in a Micro-Tidal Estuary (Randers Fjord, Denmark): Evaluation of Methods. Mar. Ecol. Prog. Ser. 301, 23–41. doi: 10.3354/meps301023
Ginestet C. (2011). Ggplot2: Elegant Graphics for Data Analysis. J. R. Stat. Soc Ser. A174, 245–246. doi: 10.1111/j.1467-985x.2010.00676_9.x
Grömping U. (2006). Relative Importance for Linear Regression in R: The Package Relaimpo. J. Stat. Softw. 17, 1–27. doi: 10.18637/jss.v017.i01
Gruber N., Clement D., Carter B. R., Feely R. A., Van Heuven S., Hoppema M., et al. (2019). The Oceanic Sink for Anthropogenic CO2 From 1994 to 2007. Science 363, 1193–1199. doi: 10.1126/science.aau5153
Guo X., Dai M., Zhai W., Cai W.-J., Chen B. (2009). CO2 Flux and Seasonal Variability in a Large Subtropical Estuarine System, the Pearl River Estuary, China. J. Geophys. Res. 114, G03013. doi: 10.1029/2008jg000905
Hansen H. P., Koroleff F. (1999). “Determination of Nutrients,” in Methods of Seawater Analysis. Eds. Grasshoff K., Kremling K., Ehrhard M. (Wiley-VCH, Weinheim), 159–228.
Harrison P. J., Yin K., Lee J. H. W., Gan J., Liu H. (2008). Physical–biological Coupling in the Pearl River Estuary. Cont. Shelf. Res. 28, 1405–1415. doi: 10.1016/j.csr.2007.02.011
He X., Bai Y., Pan D., Chen C.-T. A., Cheng Q., Wang D., et al. (2013). Satellite Views of the Seasonal and Interannual Variability of Phytoplankton Blooms in the Eastern China Seas Over the Past 14 yr 1998–2011). Biogeosciences 10, 4721–4739. doi: 10.5194/bg-10-4721-2013
Heip C., Goosen N., Herman P., Kromkamp J., Middelburg J., Soetaert K. (1995). Production and Consumptionof Biological Particles in Temperate Tidal Estuaries, London. Oceanogr. Mar. Biol. 33, 1–150.
Hopkinson C., Smith E. M. (2005). Estuarine Respiration: An Overview of Benthic, Pelagic, and Whole System Respiration. Respirat. Aquat. Ecosyst. 8, 122–146.
Huang H., Zhou L., Chen J., Wei T. (2020) Ggcor: Extended Tools for Correlation Analysis and Visualization. R Package Version 0.9.8.1. Available at: https://cran.r-project.org/web/packages/ggcor/index.html (Accessed July 20, 2021).
Hu J., Li S. (2009). Modeling the Mass Fluxes and Transformations of Nutrients in the Pearl River Delta, China. J. Mar. Syst. 78, 146–167. doi: 10.1016/j.jmarsys.2009.05.001
Hung J.-J., Ho C.-M., Shiah F.-K. (2014). Effects of River Inputs on Nutrient and Organic-Carbon Conditions and Net Ecosystem Metabolism in the Kaoping (Taiwan) Coastal Sea. Mar. Freshw. Res. 65, 697–709. doi: 10.1071/MF13183
Jiao S., Yang Y., Xu Y., Zhang J., Lu Y. (2020). Balance Between Community Assembly Processes Mediates Species Coexistence in Agricultural Soil Microbiomes Across Eastern China. ISME. J. 14, 202–216. doi: 10.1038/s41396-019-0522-9
Karl D. M., Laws E. A., Morris P., Williams P. J. L., Emerson S. (2003). Metabolic Balance of the Open Sea. Nature 426, 32–32. doi: 10.1038/426032a
Kemp W., Testa J. (2011). Metabolic Balance Between Ecosystem Production and Consumption. Treat. Estuar. Coast. Sci. 7, 83–118. doi: 10.1016/B978-0-12-374711-2.00706-3
Ke Z., Tan Y., Huang L., Liu J., Xiang C., Zhao C., et al. (2019). Significantly Depleted 15N in Suspended Particulate Organic Matter Indicating a Strong Influence of Sewage Loading in Daya Bay, China. Sci. Tot. Environ. 650, 759–768. doi: 10.1016/j.scitotenv.2018.09.076
Lepš J., Šmilauer P. (2003). Multivariate Analysis of Ecological Data Using CANOCO (Cambridge: Cambridge University Press). doi: 10.1017/CBO9780511615146
Li G., Liu J., Diao Z., Jiang X., Li J., Ke Z., et al. (2018). Subsurface Low Dissolved Oxygen Occurred at Fresh- and Saline-Water Intersection of the Pearl River Estuary During the Summer Period. Mar. Pollut. Bull. 126, 585–591. doi: 10.1016/j.marpolbul.2017.09.061
Liu H., Song X., Huang L., Zhong Y. (2012). Using Primary Productivity as an Index of Coastal Eutrophication: A Case Study in Daya Bay. Water Environ. J. 26, 235–240. doi: 10.1111/j.1747-6593.2011.00281.x
Li Y., Xiang C., Jiang Z., Song X. (2021). Production and Metabolism Characteristics of Planktonic Community and Their Influencing Factors in Daya Bay During Summer. J. Trop. Oceanogr. 40, 83–92.
Li X., Xu J., Shi Z., Li R. (2019 1026). Response of Bacterial Metabolic Activity to the River Discharge in the Pearl River Estuary: Implication for CO2 Degassing Fluxes. Front. Microbiol. 10. doi: 10.3389/fmicb.2019.01026
López-Sandoval D. C., Rowe K., Carillo-de-Albonoz P., Duarte C. M., Agustí S. (2019). Rates and Drivers of Red Sea Plankton Community Metabolism. Biogeosciences 16 (15), 2983–2995. doi: 10.5194/bg-16-2983-2019
Lu Z., Gan J. (2015). Controls of Seasonal Variability of Phytoplankton Blooms in the Pearl River Estuary. Deep. Sea. Res. Part II Top. Stud. Oceanogr. 117, 86–96. doi: 10.1016/j.dsr2.2013.12.011
Lu Z., Gan J., Dai M., Liu H., Zhao X. (2018). Joint Effects of Extrinsic Biophysical Fluxes and Intrinsic Hydrodynamics on the Formation of Hypoxia West Off the Pearl River Estuary. J. Geophys. Res.: Ocean. 123, 6241–6259. doi: 10.1029/2018jc014199
McKinnon A. D., Duggan S., Logan M., Lønborg C. (2017). Plankton Respiration, Production, and Trophic State in Tropical Coastal and Shelf Waters Adjacent to Northern Australia. Front. Mar. Sci. 4. doi: 10.3389/fmars.2017.00346
Odum H. T. (1956). Primary Production in Flowing Waters1. Limnol. Oceanogr. 1, 102–117. doi: 10.4319/lo.1956.1.2.0102
Parsons T. R., Maita Y., Lalli C. M. (1984). A Manual of Chemical and Biological Methods for Seawater Analysis (Oxford: Pergamon Press).
Qian W., Gan J., Liu J., He B., Lu Z., Guo X., et al. (2018). Current Status of Emerging Hypoxia in a Eutrophic Estuary: The Lower Reach of the Pearl River Estuary, China. Estuar. Coast. Shelf. Sci. 205, 58–67. doi: 10.1016/j.ecss.2018.03.004
Regaudie-de-Gioux A., Duarte C. M. (2013). Global Patterns in Oceanic Planktonic Metabolism. Limnol. Oceanogr. 58, 977–986. doi: 10.4319/lo.2013.58.3.0977
Rochelle-Newall E. J., Winter C., Barrón C., Borges A. V., Duarte C. M., Elliott M., et al. (2007). Artificial Neural Network Analysis of Factors Controlling Ecosystem Metabolism in Coastal Systems. Ecol. Appl. 17, S185–S196. doi: 10.1890/05-1769.1
Schlitzer R. (2020) Ocean Data View. Available at: http://odv.awi.de (Accessed October 20, 2021).
Serret P., Robinson C., Aranguren-Gassis M., Garcia-Martin E. E., Gist N., Kitidis V., et al. (2015). Both Respiration and Photosynthesis Determine the Scaling of Plankton Metabolism in the Oligotrophic Ocean. Nat. Commun. 6, 1–10. doi: 10.1038/ncomms7961
Song X., Huang L., Zhang J., Huang H., Li T., Su Q. (2009). Harmful Algal Blooms (HABs) in Daya Bay, China: An in Situ Study of Primary Production and Environmental Impacts. Mar. Pollut. Bull. 58, 1310–1318. doi: 10.1016/j.marpolbul.2009.04.030
Song X., Huang L., Zhang J., Huang X., Zhang J., Yin J., et al. (2004). Variation of Phytoplankton Biomass and Primary Production in Daya Bay During Spring and Summer. Mar. Pollut. Bull. 49, 1036–1044. doi: 10.1016/j.marpolbul.2004.07.008
Song X., Huang L., Zhang J., Yin K., Liu S., Tan Y., et al. (2011). Chemometric Study of Spatial Variations of Environmental and Ecological Characteristics in the Zhujiang River (Pearl River) Estuary and Adjacent Waters. Acta Oceanol. Sin. 30, 60–74. doi: 10.1007/s13131-011-0137-0
Song X., Lai Z., Ji R., Chen C., Zhang J., Huang L., et al. (2012). Summertime Primary Production in Northwest South China Sea: Interaction of Coastal Eddy, Upwelling and Biological Processes. Cont Shelf. Res. 48, 110–121. doi: 10.1016/j.csr.2012.07.016
Song X., Liu H., Zhong Y., Tan Y., Qin G., Li K., et al. (2015). Bacterial Growth Efficiency in a Partly Eutrophicated Bay of South China Sea: Implication for Anthropogenic Impacts and Potential Hypoxia Events. Ecotoxicology 24, 1529–1539. doi: 10.1007/s10646-015-1497-6
Song X., Li Y., Xiang C., Su X., Xu G., Tan M., et al. (2021). Nitrogen and Phosphorus Enrichments Alter the Dynamics of the Plankton Community in Daya Bay, Northern South China Sea: Results of Mesocosm Studies. Mar. Freshw. Res. 72, 1632–1642. doi: 10.1071/mf21097
Wang Q., Wang X., Xiao K., Zhang Y., Luo M., Zheng C., et al. (2021). Submarine Groundwater Discharge and Associated Nutrient Fluxes in the Greater Bay Area, China Revealed by Radium and Stable Isotopes. Geosci. Front. 12, 101223. doi: 10.1016/j.gsf.2021.101223
Wemheuer B., Güllert S., Billerbeck S., Giebel H.-A., Voget S., Simon M., et al. (2014). Impact of a Phytoplankton Bloom on the Diversity of the Active Bacterial Community in the Southern North Sea as Revealed by Metatranscriptomic Approaches. FEMS Microbiol. Ecol. 87, 378–389. doi: 10.1111/1574-6941.12230
Williams P. J. L. B., Quay P. D., Westberry T. K., Behrenfeld M. J. (2013). The Oligotrophic Ocean Is Autotrophic. Ann. Rev. Mar. Sci. 5, 535–549. doi: 10.1146/annurev-marine-121211-172335
Wu M. L., Wang Y. S., Wang Y. T., Sun F. L., Dong J. D. (2016). Seasonal and Spatial Variations of Water Quality and Trophic Status in Daya Bay, South China Sea. Mar. Pollut. Bull. 112 (1), 341–348. doi: 10.1016/j.marpolbul.2016.07.042
Wu M. L., Wang Y. S., Wang Y. T., Yin J. P., Dong J. D., Jiang Z.-Y., et al. (2017). Scenarios of Nutrient Alterations and Responses of Phytoplankton in a Changing Daya Bay, South China Sea. J. Mar. Syst. 165, 1–12. doi: 10.1016/j.jmarsys.2016.09.004
Yang C., Li Q., Hu Z., Chen J., Shi T., Ding K., et al. (2019). Spatiotemporal Evolution of Urban Agglomerations in Four Major Bay Areas of US, China and Japan From 1987 to 2017: Evidence From Remote Sensing Images. Sci. Tot. Environ. 671, 232–247. doi: 10.1016/j.scitotenv.2019.03.154
Yin K., Lin Z., Ke Z. (2004a). Temporal and Spatial Distribution of Dissolved Oxygen in the Pearl River Estuary and Adjacent Coastal Waters. Cont. Shelf. Res. 24, 1935–1948. doi: 10.1016/j.csr.2004.06.017
Yin K., Qian P., Chen J., Hsieh D., Harrison P. (2000). Dynamics of Nutrients and Phytoplankton Biomass in the Pearl River Estuary and Adjacent Waters of Hong Kong During Summer: Preliminary Evidence for Phosphorus and Silicon Limitation. Mar. Ecol. Prog. Ser. 194, 295–305. doi: 10.3354/meps194295
Yin K., Zhang J., Qian P.-Y., Jian W., Huang L., Chen J., et al. (2004b). Effect of Wind Events on Phytoplankton Blooms in the Pearl River Estuary During Summer. Cont. Shelf. Res. 24, 1909–1923. doi: 10.1016/j.csr.2004.06.015
Yu H. (2019). The Guangdong-Hong Kong-Macau Greater Bay Area in the Making: Development Plan and Challenges. Camb. Rev. Int. Aff. 34, 1–29. doi: 10.1080/09557571.2019.1679719
Zhai W. D., Dai M. H., Chen B. S., Guo X. H., Li Q., Shang S. L., et al. (2013). Seasonal Variations of Sea–Air CO2 Fluxes in the Largest Tropical Marginal Sea (South China Sea) Based on Multiple-Year Underway Measurements. Biogeosciences 10, 7775–7791. doi: 10.5194/bg-10-7775-2013
Zhang L., Xiong L., Zhang J., Jiang Z., Zhao C., Wu Y., et al. (2019). The Benthic Fluxes of Nutrients and the Potential Influences of Sediment on the Eutrophication in Daya Bay, South China. Mar. Pollut. Bull. 149, 110540. doi: 10.1016/j.marpolbul.2019.110540
Zhao Z., Li H., Sun Y., Yang Q., Fan J. (2021). Contrasting the Assembly of Phytoplankton and Zooplankton Communities in a Polluted Semi-Closed Sea: Effects of Marine Compartments and Environmental Selection. Environ. Pollut. 285, 117256. doi: 10.1016/j.envpol.2021.117256
Zhao Y., Liu J., Uthaipan K., Song X., Xu Y., He B., et al. (2020). Dynamics of Inorganic Carbon and pH in a Large Subtropical Continental Shelf System: Interaction Between Eutrophication, Hypoxia, and Ocean Acidification. Limnol. Oceanogr. 65, 1359–1379. doi: 10.1002/lno.11393
Zhu Z., Zhang J., Wu Y., Zhang Y., Lin J., Liu S. (2011). Hypoxia Off the Changjiang (Yangtze River) Estuary: Oxygen Depletion and Organic Matter Decomposition. Mar. Chem. 125, 108–116. doi: 10.1016/j.marchem.2011.03.005
Zu T., Gan J. (2015). A Numerical Study of Coupled Estuary–Shelf Circulation Around the Pearl River Estuary During Summer: Responses to Variable Winds, Tides and River Discharge. Deep. Sea. Res. Part II Top. Stud. Oceanogr. 117, 53–64. doi: 10.1016/j.dsr2.2013.12.010
Keywords: primary production, community respiration, hypoxia, algal bloom, Pearl River Estuary
Citation: Zhang L, Li G, Xiang C, Huang Y, Fu X, Zheng C, Wang Z, Ouyang Z and Song X (2022) Plankton Metabolism in Coastal Waters of the Guangdong-Hong Kong-Macao Greater Bay: Regional Variance and Driving Factors. Front. Mar. Sci. 9:844970. doi: 10.3389/fmars.2022.844970
Received: 29 December 2021; Accepted: 19 April 2022;
Published: 27 May 2022.
Edited by:
Yu-Pin Lin, National Taiwan University, TaiwanReviewed by:
Yuyuan Xie, University of South Florida, United StatesPeng Jin, University of Guangzhou, China
Copyright © 2022 Zhang, Li, Xiang, Huang, Fu, Zheng, Wang, Ouyang and Song. This is an open-access article distributed under the terms of the Creative Commons Attribution License (CC BY). The use, distribution or reproduction in other forums is permitted, provided the original author(s) and the copyright owner(s) are credited and that the original publication in this journal is cited, in accordance with accepted academic practice. No use, distribution or reproduction is permitted which does not comply with these terms.
*Correspondence: Xingyu Song, songxy@scsio.ac.cn