- 1School of Biological Sciences, University of Auckland, Auckland, New Zealand
- 2Institute of Environmental Science and Research, Christchurch, New Zealand
- 3Manufacturing and Bioproducts, Scion, Te Papa Tipu Innovation Park, Rotorua, New Zealand
- 4Coastal and Freshwater Group, Cawthron Institute, Nelson, New Zealand
- 5Forest Genetics and Biotechnology, Scion, Te Papa Tipu Innovation Park, Rotorua, New Zealand
- 6Chemistry and Physics Group, Scion, Te Papa Tipu Innovation Park, Rotorua, New Zealand
- 7Materials, Engineering and Manufacturing, Scion, Te Papa Tipu Innovation Park, Rotorua, New Zealand
The ubiquity of plastic debris in marine environments raises the question, what impacts do plastics have on our marine microbiota? To investigate this, we applied bacterial 16S rRNA gene and fungal ITS2 region sequencing to identify changes in microbial biofilm community compositions on marine plastic, over time. We sampled biofilm on virgin linear low-density polyethylene (LLDPE), nylon-6 (PA) and glass after 2, 6 and 12 weeks of constant immersion in Te Whakaraupō-Lyttelton Harbour, Aotearoa-New Zealand. Of the prokaryotes, Proteobacteria and Bacteroidetes were predominant in all samples and Verrucomicrobiota were most abundant in mature biofilms. Microbial communities on the three substrate types were significantly distinct from those in the surrounding seawater, regardless of age, but not between attachment substrates. Bacterial communities occurring two weeks after immersion and fungal communities at six weeks were found to vary more among substrate types than at other times; however, no significant substrate-specific communities were identified overall. Taxa closely related to previously reported plastic-biodegrading species were found in very low abundance across all substrates, including on the glass slides. Our findings suggest that microorganisms do not selectively persist on the LLDPE or PA surfaces to gain significant direct metabolic benefit, instead using these plastics primarily as an attachment surface on which they form generalist biofilm communities.
Introduction
Since industrial production began in the 1950s, plastic debris has become ubiquitously distributed in our environment; it is even found in remote locations, from isolated mountain catchments in the French Alps (Allen et al., 2019) to the depths of the Mariana Trench in the western Pacific Ocean (Jamieson et al., 2019). An estimated 19 to 23 million metric tons of plastic waste amassed in our oceans in 2016 alone (Borrelle et al., 2020), increasing societal concerns regarding the accumulation and impact of plastics on the marine environment (Schnurr et al., 2018). As a result, the harm inflicted on macrobiota such as birds, fish and mammals, by ingestion or entanglement has been well documented (Laist, 1997; Wilcox et al., 2013; Besseling et al., 2015). However, the ecological impacts of plastic polymers and their additives on the ecology of marine microbiota are less explored and therefore not well understood (Lear et al., 2021).
Following preliminary observations of bacterial and diatom colonisation on plastic debris from the Sargasso Sea in the 1970s (Carpenter and Smith, 1972), it has become clear that complex, diverse microbial and metazoan communities rapidly colonise marine plastic (Zettler et al., 2013). Many studies have since characterised the bacterial and eukaryotic communities formed on environmental plastics, often referred to as the ‘plastisphere’, providing evidence that taxonomically distinct plastisphere communities develop as compared to communities in the surrounding environment (Lear et al., 2021). Whilst it has been shown that microbes such as the fungal phylum Chytridiomycota (Kettner et al., 2017) and the bacterial phyla Bacteriodetes, Proteobacteria (Oberbeckmann et al., 2014) and Cyanobacteria (Bryant et al., 2016) are highly abundant in the plastisphere, the specificity of plastisphere taxa remains enigmatic as none are known to colonise specific plastics alone. It has yet to be concluded whether different communities associate with, and develop on different types of plastic, which could impact the broader ecosystem’s functionality, with unknown and unforeseen consequences (Oberbeckmann et al., 2017; Pinto et al., 2019).
When studying the plastisphere microbiome, the focus has predominantly been to assess mature biofilm, or biofilm of undeterminable age. Whilst there have been limited investigations into the pioneer species during the early formation of plastisphere biofilms, a recent study investigated the microbiomes of plastic pre-production pellets in flowing seawater, revealing compositionally distinct communities forming on plastic between 8 and 70 days (Ward et al., 2022). Erni-Cassola et al. (2020) suggested that obligate hydrocarbon-degrading bacteria may dominate the preliminary stages of colonisation if they possess the ability to utilise hydrocarbons which can leach from weathered plastics, as a carbon source. As surface hydrophobicity influences microbial biofilm formation (Teughels et al., 2006; Rummel et al., 2017), early colonisers may be better adapted to colonising plastic surfaces, before succession results in domination by more ‘generic’ biofilm community members (Wright et al., 2020b). Due to the extensive variability in the physicochemical dynamics of plastic surfaces and particularly the presence of different additives and contaminants, microbial communities are likely to be impacted both positively and negatively by plastics, depending on their combined responses to these substrates as either sources or carbon and nutrients, or as sources of toxicants. The paucity of studies investigating microbial colonisation of marine plastics in situ leaves a significant knowledge gap in understanding which taxa are involved in the early stages of plastic colonisation and at different stages of biofilm succession. This is of particular concern since the composition of even the most commonly used plastics is highly variable (Roosen et al., 2020; Wiesinger et al., 2021), meaning microbial responses to marine plastics are likely to be very specific to the particular substrates and environments under study. Currently, we understand relatively little with regard to whether generalist biofilm communities form on plastics, or if different taxa colonise different plastics, as well as how these communities vary over time and the implications this may have on ecosystem health and function.
Previous studies have shown that plastisphere communities are significantly influenced by their geographic location (Hoellein et al., 2014; Oberbeckmann et al., 2014), highlighting the need for investigations to take place over a more extensive range of spatial scales to determine any geographic factors shaping plastisphere assembly and to identify a core microbiome within plastisphere communities, should one exist. Our study is the first to characterise marine plastisphere communities in Aotearoa-New Zealand (A-NZ), to determine the presence of taxa previously identified as putative plastic biodegraders in global studies and conversely, to determine whether there are taxa in our ocean that might be sensitive to plastics, with potentially unintended consequences. To assess the identities and abundance of plastic-associated bacteria and fungi, we deployed a structure containing two different plastic types along with an inert glass control into the marine environment at Te Whakaraupō-Lyttelton Harbour, A-NZ, for a short period of time (sampling from 2 – 12 weeks), allowing assessment of the early stages of microbial plastic colonisation. The polymers in our study – nylon-6 (also called polyamide-6, abbreviated in our study as PA) and linear low-density polyethylene (LLDPE), were selected based on their high abundance in marine plastic debris (Guzzetti et al., 2018; Erni-Cassola et al., 2019) and different chemical compositions as PA contains heteroatoms in the polymer backbone, LLDPE does not. They also contained additives typically used in commercial plastics (e.g., tris(2,4-di-tert-butylphenyl)phosphite, or Irgafos 168®) which have been previously reported as hazardous or toxic (Hammond et al., 2013; Li et al., 2016; Groh et al., 2019). First, we hypothesised that microbial communities associating with these substrates differ taxonomically from microbes present in ambient seawater. Second, since the diversity of microbial communities is thought to be influenced by the degree of hydrophobicity of the substrate (PA is hydrophilic (Huang and McCutcheon, 2014) whereas LLDPE is hydrophobic (Lu et al., 2004)), we predicted to observe significant differences in the composition, evenness and diversity of bacterial and fungal communities between the biofilms that formed on these plastics and also on glass. Further, we predicted to see greater differences between the communities of younger plastic-associating biofilms compared to communities growing on the glass, indicating the presence of pioneer substrate-specific taxa capable of biodegrading plastics. We hypothesised that the community differences among substrates would reduce as the biofilm aged since we expect more ‘generalist’ marine taxa would dominate the biofilm over time.
Materials and methods
Sampling Site and Collection
The location of the marine plastics deployment was at the Lyttelton Port Company (LPC) floating pontoon in Te Whakaraupō-Lyttelton Harbour, Aotearoa-New Zealand (A-NZ) (43°36’27.3”S; 172°43’24.2”E). The site is a major commercial port, with no public access to the deployment site.
The polymer types used in the study, PA and LLDPE, contained additives typically included in these products for UV light stability and degradation prevention; PA comprised the polyamide base polymer Ultramid B3S with 0.5% Nylostab S-EED additive; LLDPE comprised base polymer Innopulus LL7410D1 with additive 0.25% Irganox B215 (33% Irganox 1010 and 67% Irgafos 168). Any other contaminants associated with these were at very low levels, i.e. inorganic contaminants were below the limits of detection of thermogravimetric analysis ( ± 0.1% of the original mass). Plastics were injection-moulded into rectangular paddles (75 mm x 50 mm x 3 mm) with an integrated arm (Figure S1A) using a bespoke die. The plastic paddles were distributed across three marine-grade stainless-steel (316) poles using a stainless-steel (316) frame. Plastic paddles were installed at three levels due to space constraints, starting 20 cm beneath the water surface and spaced approximately every 20 cm (top, middle and bottom). As the experimental structure was deployed on a floating pontoon, the depth of samples remained consistent throughout the study and was not impacted by tides. Sterile glass slides (76 mm x 51 mm x 1 mm made from extra white low iron soda-lime float-glass, ProSciTech, Australia) were clamped within laser-cut marine grade stainless-steel frames and attached to the rods with the plastic paddles, at each depth. All slides and paddles were oriented horizontally to the water surface (Figure S1B) and data loggers (HOBO Pendant MX2202; Onset Computer Corp, Bourne, MA, USA) were attached at each depth to monitor temperature and light intensity within the water column, every 15 minutes, throughout the experiment (Figure S2). No significant difference in temperature exposure was found between the three depths in our structure (average temperature was 9.1 °C at each depth). However, light intensity declined significantly with sample depth (1213, 883 and 553 average lux for loggers fixed to the top, middle and bottom sampling levels, respectively; two-factor ANOVA, P < 0.01).
The structure was deployed on 11 June 2019 (austral winter) and sampled on three occasions (14, 44 and 80 days after deployment, hereafter referred to as weeks 2, 6 and 12). At each time, samples were collected in triplicate for each substrate type (one per depth); a total of six plastic paddles and three glass slide samples were collected per sampling date, resulting in 27 paddles and glass slides overall. On each sampling occasion, the deployment structure was removed from the water and, using gloved hands, individual sterile Fisherbrand sample bags (Cat No. 14955189; Thermo Fisher Scientific, Waltham, MA, USA) were carefully placed over paddles which were then excised from the structure using pipe cutters by cutting the integrated arm. Three 2 L seawater samples were also collected from the same location and approximate depth as the deployment structure at each timepoint to determine the ambient microbial community composition of the seawater. Before use, a stainless-steel water collection bucket and 2 L Schott Duran® glass bottles were acid-washed and sterilised by autoclaving. Before sampling, the bucket and bottles were rinsed thrice with seawater. All samples were placed on ice for transportation back to the laboratory for processing (i.e. three timepoints, three replicates and four substrates [two plastics, glass and seawater], for a total of 36 samples).
Sample Processing
Biomass was extracted within three hours of collection. All procedures were performed on ice. The bulk of the biomass was removed from both sides of the plastic paddles and glass slides, using sterile flat-edged razor blades. Biomass was placed into a separate sterile 50 ml tube per sample (Cat No. 227-261; Greiner Cellstar®, Sigma-Aldrich, St. Louis, MO, USA). The scraped paddle or slide was placed back in the original sampling bag along with 30 ml ice-cold, sterile Tris-EDTA buffer (TE; Tris 10 M, EDTA 1 mM, pH 8.0). Samples were then sonicated in an ice-cold ultrasonic water bath (Bandelin Sonorex RK 100H; Sigma-Aldrich, St. Louis, MO, USA) for 2 min at 35 kHz to recover additional biomass. The sonicate solution was added, by pouring, to the recovered biomass from the same sample and centrifugated at 4,500 xg for 10 min at 4°C. Supernatants were gently decanted and discarded, followed by an additional 1 min centrifugation and removal of the remaining supernatant by pipette. Seawater was filtered (one litre per filter) through 0.2 µm filter membranes (Supor® 200; Whatman, Maidstone, UK) using a vacuum pump. Membranes were placed in individual, sterile, 15 ml centrifuge tubes and all samples were stored at -80°C until required.
DNA Extraction
Microbial DNA was manually extracted individually from each pelleted biofilm and seawater filter sample using a DNeasy PowerSoil kit (Qiagen, Hilden, Germany) according to the manufacturer’s instructions, except for the mechanical lysis step, which was performed using a TissueLyser II (Cat No. 85300; Qiagen, Hilden, Germany) for 2 min at 30 Hz. DNA was extracted from up to 250 mg biofilm biomass. When sample biomass was <100 mg, 100 µl UltraPure Dnase/Rnase-Free Distilled Water (Cat No. 10977015; Invitrogen, Thermo Fisher Scientific, Waltham, MA, USA) was used to resuspend the pellet, and the entire volume was then used for extraction. Filtered seawater samples were placed directly into the PowerSoil bead tubes using sterile tweezers. DNA was extracted from a total of 36 samples (i.e. 27 biofilm samples, plus nine seawater samples), alongside an extraction kit control blank, following the manufacturers’ instructions and each eluted in 100 µl elution buffer. DNA concentration was assessed using a Nanodrop photometer (Implen Nanophotometer, Munich, Germany) and DNA samples were stored at -80°C until required.
PCR and DNA Sequencing
Polymerase chain reactions (PCRs) were performed to amplify: (i) the hypervariable V3/V4 region of the bacterial small-subunit of ribosomal RNA (16S) genes, using the universal amplicon primer pair 341F and 785R (see Table S1), and (ii) the fungal internal transcribed spacer 2 (ITS2) region of the nuclear ribosomal gene using the primer pair: fITS7 and ITS4 (Table S1). All primers were modified to contain the Illumina adapter sequences required for amplicon sequencing (Kozich et al., 2013). All PCRs, including sampling blanks from a parallel study at the same location, extraction kit blanks and PCR controls, were conducted in total reaction volumes of 25 µl containing 12.5 µl KAPA HiFi HotStart ReadyMix (KAPA Biosystems Roche, Millennium Science, NZ), 0.75 µl of each primer (10 µM), 1 µl template DNA and 10 µl UltraPure distilled water. Following PCR (Table S1), gel electrophoresis was performed to verify amplicon sizes; 5 µl of PCR products were run on a 2% (w/v) agarose gel, stained using SYBR SAFE DNA Gel Stain (Invitrogen, Thermo Fisher Scientific, Waltham, MA, USA) and visualised under UV light using a GelDoc imaging system. PCR products were quantified using a Qubit double-stranded DNA (dsDNA) High Sensitivity Assay kit (Invitrogen, Thermo Fisher Scientific, Waltham, MA, USA), purified using a DNA Clean and Concentrator-5 kit (Zymo Research, Irvine, CA, USA) and eluted in 12 µl DNA elution buffer, according to the manufacturer’s instructions. DNA extraction controls (and sampling controls collected at the same site in a parallel study) yielded no sequencing product and therefore were not sent for sequencing. The Auckland Genomics Facility (The University of Auckland, New Zealand) conducted DNA sequencing on an Illumina MiSeq instrument using 2‐by‐300‐bp V3 chemistry. Before DNA sequencing, the sequencing provider attached a unique combination of Nextera XT dual indices (Illumina Inc., San Diego, CA, USA) to the DNA from each sample to allow sample multiplexing.
Processing and Analysis of DNA Sequence Data
Auckland Genomics Facility demultiplexed raw sequence reads. Following this, we removed sequence adapters from reads with Trimmomatic (version 0.39; Bolger et al., 2014) using the parameters: ILLUMINACLIP:2:40:15. Primers were then removed from reads using Cutadapt (Martin, 2011). Successfully trimmed reads were processed using the ‘DADA2’ package (Callahan et al., 2016) in R (version 4.0.1; R Core Team, 2020) following the package instructions. Quality plots of sequence reads were inspected, and reads were then filtered by allowing a maximum estimated error (“maxEE”) of 2 per 100 bp. Pooling of samples to achieve convergence of the parametric error model was performed to estimate sequence error rates. To reduce low sampling depth bias, all samples were dereplicated and pooled together for amplicon sequence variant (ASV) inference. Following this, paired-end reads were merged, chimeric sequences removed and an ASV table constructed. Taxonomic assignment was performed against the SILVA reference database for bacterial 16S rRNA sequence reads (version 138; McLaren, 2020) and against the UNITE reference database for fungal ITS sequence reads (general FASTA release version 10.05.2021; Abarenkov et al., 2021), using the native implementation of the naïve Bayesian classifier method. ASVs were defined as clusters sharing DNA sequence identity of 100%.
Accession Numbers
Raw reads are available from the NCBI Sequence Read Archive under the BioProject ID PRJNA764817.
Bioinformatics and Statistical Analysis
Data Preparation
Bacterial and fungal ASV tables were filtered to only include read data assigned to the kingdoms of ‘Bacteria/Archaea’ or ‘Fungi’, respectively. ASVs that remained unclassified to the level of kingdom were removed, as were ASVs classified at the order level as chloroplasts. This represented less than 10% of all sequences.
All statistical analyses and visualisations were performed using R (version 4.0.2; R Core Team, 2020). To evaluate if sufficient DNA sequencing depth had been achieved, rarefaction curves of the ASV data were plotted. To achieve standard sequencing depth and ensure comparability of diversity, samples were then rarefied using the ‘vegan’ R package (version 2.5-7; Oksanen et al., 2020) before downstream analysis. ASV tables were randomly subsampled to the minimum library size (rarefying; set.seed(123), bacteria n = 6810, fungi n = 577) to ensure comparable sequencing depth.
Quantitative Analyses
To investigate species richness and evenness within the samples, alpha diversity analyses were carried out by plotting the rank abundance of ASVs. Chao1, Shannon and inverse Simpson statistics were calculated using the phyloseq ‘estimate_richness’ function to compare bacterial and fungal richness and diversity. Permutational multivariate analysis of variance (PERMANOVA; Anderson et al., 2008) with 999 permutations was carried out on Bray-Curtis dissimilarity matrices using the vegan ‘adonis’ function to statistically evaluate whether substrate type or biofilm age had a significant effect (P < 0.05) on the composition of bacterial and fungal communities. Pairwise PERMANOVA was also conducted to assess the statistical significance (P < 0.05) between the composition of communities on different substrates or biofilm ages, using the vegan ‘pairwise.adonis’ function. Homogeneity of multivariate variance was quantified using the vegan ‘betadisper’ function. Two-dimensional non-metric multi-dimensional scaling (NMDS) based on Bray-Curtis dissimilarity measures was plotted using ‘ggplot2’ for visualisation. Ellipses were drawn onto NMDS plots using the stat_ellipse function of ggplot2 assuming a multivariate t-distribution. Rarefied ASV tables were used to calculate relative abundances and the top five genera per substrate were determined per sample. Stacked bar charts were created at the genus level to show biofilm composition at different ages (2 weeks, 6 weeks and 12 weeks) and on different substrates (LLDPE, PA, glass and seawater). To investigate whether bacterial and fungal ASVs are shared or unique for each substrate and sample time, Venn diagrams were created using R. Indicative value (IndVal) analyses (with 999 permutations and P > 0.05) were performed to evaluate which ASVs contributed most to the observed differences in bacterial and fungal community compositions (Dufrêne and Legendre, 1997) among substrates. To determine the presence of any previously reported plastic biodegraders within our samples, taxonomy tables were searched against a database of putative plastic-biodegrading organisms (Gambarini et al., 2022); potential biodegraders were identified to genus and species level.
Results
A total of 36 marine samples were collected, comprising three replicates of each substrate, sampled 2, 6 and 12 weeks after deployment, and triplicate water samples taken at each timepoint. After quality filtering, a total of 5142 and 1773 unique taxa were obtained for bacterial and fungal comparative analyses, respectively. Rarefaction curves based on the ASVs of the bacterial communities (Figure S3A) indicated adequate sequencing depth was achieved to ensure the majority of species were represented. However, many fungal rarefaction curves did not reach saturation (Figure S3C); further sequencing would therefore give a better indication of diversity. Following rarefaction, 4431 bacterial and 688 fungal unique taxa were retained for further analysis.
Comparison of Bacterial and Fungal Community Richness, Evenness and Diversity Across Substrates and Ages
Rank abundance curves were generated to assess the alpha diversity of the bacterial (Figure S3B) and fungal (Figure S3D) communities. In both cases, steep slopes indicate uneven distributions of ASV abundance, demonstrating the communities in the majority of samples were highly dominated by a small number of taxa. ANOVA indicated significant differences in bacterial community richness comparing substrate types when seawater samples were included (Chao1; substrate P = 0.001). Community evenness was more impacted by the length of sample deployment (Shannon and inverse Simpson; age P = 0.001, interaction factor between substrate and age P = 0.025 and 0.019 respectively). Differences in fungal community richness and evenness were also significantly related to substrate type (Chao1; P = 0.021, Shannon; P = 0.035, inverse Simpson; P = 0.002) and a significant interaction term was detected between substrate type and timepoint (Chao1; P = 0.003, Shannon; P = 0.091, inverse Simpson; P = 0.009). When removing seawater data to compare only the communities on solid substrates (i.e., including on glass slides), significant differences in bacterial community richness and evenness were only detected due to sampling date (ANOVA; P all < 0.05), rather than substrate type (ANOVA; P = 0.716, 0.744 and 0.832, for Chao1, Shannon and Inverse Simpson values, respectively). Similarly, no differences in fungal community indices were determined between the glass, PA and LLDPE substrates (ANOVA; P = 0.272, 0.197 and 0.659, for Chao1, Shannon and Inverse Simpson values, respectively). Bacterial biofilm communities had a higher Chao1 richness than seawater communities across all ages (Figure S4A), whereas seawater fungal communities had a higher Chao1 richness only at weeks 2 and 6 (Figure S4B). Shannon and inverse Simpson diversity indices indicated no change in fungal biofilm diversity, but higher bacterial diversity at 2 weeks compared to 6 and 12 weeks (Figure S4).
Variation in Bacterial and Fungal Community Composition Across Substrates and Ages
Non-metric multidimensional scaling (NMDS) analysis suggested data from ambient seawater clustered separately from biofilm microbial community data (Figures 1A, C). This indicates that the composition of both bacterial and fungal communities present in the seawater are distinct from those in the biofilms (glass, PA and LLDPE), which was supported by pairwise PERMANOVA (bacterial and fungal; seawater vs PA P = 0.001, seawater vs LLDPE P = 0.001, seawater vs glass P = 0.002; all other pairwise comparisons were not significant). After confirming that the seawater communities differed from the biofilm communities, we repeated these analyses using only the glass, PA, and LLDPE biofilm data. Both biofilm age and substrate type were significantly correlated with the observed variation in both bacterial and fungal biofilm community composition (PERMANOVA P < 0.05; Figures 1B, D). For the bacteria, the biofilm communities after 2 weeks of incubation were most different to those at 6 and 12 weeks (as determined by average Bray-Curtis distance; pairwise PERMANOVA; 2 weeks vs 6 weeks P = 0.001, 2 weeks vs 12 weeks P = 0.001, 6 weeks vs 12 weeks P = 0.024). In contrast, the fungal biofilm communities differed most after 6 weeks of incubation (pairwise PERMANOVA; 2 vs 6 weeks P = 0.06, 2 vs 12 weeks P = 0.001, 6 vs 12 weeks P = 0.001). A significant interaction term was detected (PERMANOVA P = 0.004; Figure 1D) between substrate and age for the fungal data, with the greatest variation among communities on different substrates occurring at 6 weeks. No significant interaction term was identified for the bacterial community data (PERMANOVA P = 0.161; Figure 1B), indicating that the extent of variation among the three solid substrate types did not differ with biofilm age. A permutational test for homogeneity of multivariate dispersions was not significant for either factor (P all > 0.05). We found no evidence that sample depth, and thus related declines in light intensity with depth, had any significant impact on bacterial or fungal community compositions (Figure S5), suggesting the observed variation in composition is dominated by factors other than sample depth.
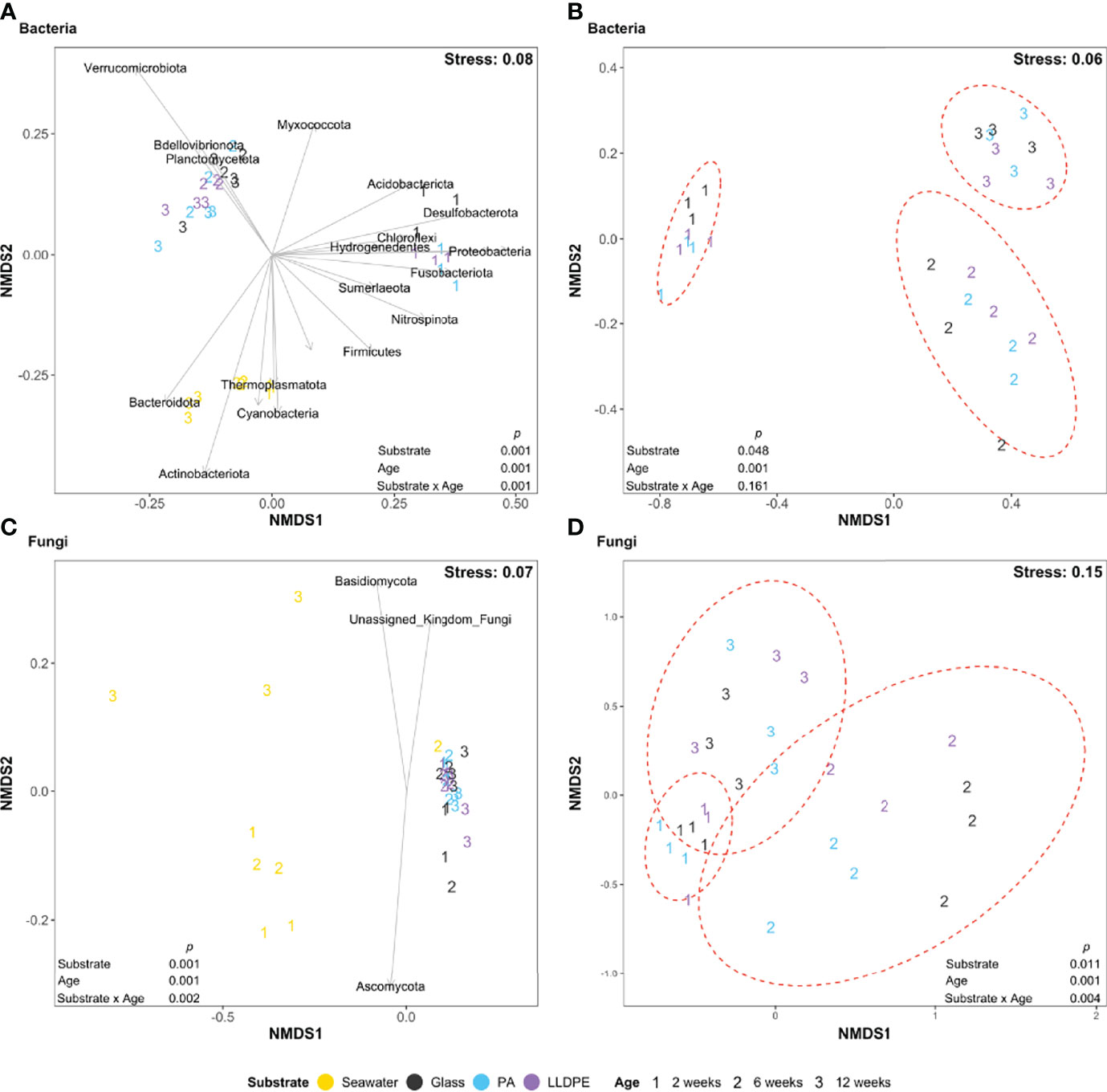
Figure 1 Non-metric multidimensional scaling (NMDS) ordination for the visualisation of Bray-Curtis similarities of (A, B) bacterial (C, D) fungal communities in the surrounding seawater and on glass, nylon-6 (PA) or linear low-density polyethylene (LLDPE) in the marine environment of Te Whakaraupō-Lyttelton Harbour, Aotearoa-New Zealand, labelled according to substrate type and biofilm age. Closer proximity of points indicates increasing compositional similarity among those data. PERMANOVA results are shown as p values, assessing the impact of the substrate type, biofilm age and their interaction. Black arrows represent significant (P < 0.05) phyla correlating with ordination of samples. Ellipses were drawn using the stat_ellipse function of ggplot2 assuming a multivariate t-distribution and represent variance observed among each different sample age with 95% confidence intervals.
Taxonomic Variation in Bacterial and Fungal Community Composition Across Substrates and Ages
To understand which phyla were dominating within each sample, total relative abundances were plotted, showing that Proteobacteria and Bacteroidetes dominated microbial communities across all samples (Figure S6A). Verrucomicrobiota were present at low abundances in all samples at 2 weeks, increasing in abundance in biofilm communities at 6 and 12 weeks. Actinobacteriota were also present at low levels across all samples, with a higher abundance in seawater. Very low abundances of Campilobacterota, Planctomycetota and Bdellovibrionota were detectable in all samples, as were low levels of Cyanobacteria and Desulfobacterota at 12 weeks except for no detection in one PA 12-week sample and one LLDPE 6-week sample, respectively. Patescibacteria were present at low levels in all PA and LLDPE samples and most seawater and glass samples. Marinimicrobia (SAR406 clade) were mainly present in seawater at low levels. Most fungal reads remained unassigned at the phylum level (Figure S6B) due to the lack of identification of fungi in the marine environment. Within the fungal sequences assigned, low levels of Ascomycota were present across all samples, as were low abundances of Basidiomycota and Chytridiomycota, which increased in abundance in biofilm samples after 12 weeks. Biofilm phyla were therefore not observed to be specific to the three solid substrates deployed in this study.
Communities in ambient seawater were dominated by the bacterial genera N5S marine group and Planktomarina across all timepoints (Figure 2A). At 2 weeks, SAR11 Clade-Ia and unassigned genera were among the five most abundant genera across all seawater samples, along with high abundances of either Candidatus Actinomarina or Amylibacter. At 6 weeks, Formosa and NS4 marine groups were among the five most abundant, with the abundance of Polaribacter and Candidatus Actinomarina increasing at 12 weeks in seawater samples. Although Thalassotalea and Colwellia dominated all 2-week biofilm samples, a greater diversity of highly abundant bacteria were detected within the 2 week biofilm. Sulfitobacter were also abundant in all samples, particularly in bacterial communities present on PA. At 2 weeks, Thiomicrorhabdus and Yoonia-Loktanella were highly abundant on glass. In contrast, Oleiphilus, Fluviicola, Polaribacter and Dasania were highly abundant in PA bacterial communities and Fluviicola, Yoonia-Loktanella, Oleibacter and Arenicella in bacterial assemblages of LLDPE. At 6 and 12 weeks, the relative abundances of Yoonia-Loktanella, Rubritalea and Pibocella increased across all biofilm communities. Overall, the most dominant genera in seawater microbial communities appear to have a more consistent relative abundance over time compared with glass, PA and LLDPE biofilm communities. Early 2-week communities showed sample-level variation among the five most abundant genera associated with glass, PA and LLDPE; however, this is reduced as the biofilms matured and the composition of the most abundant taxa varied less over time.
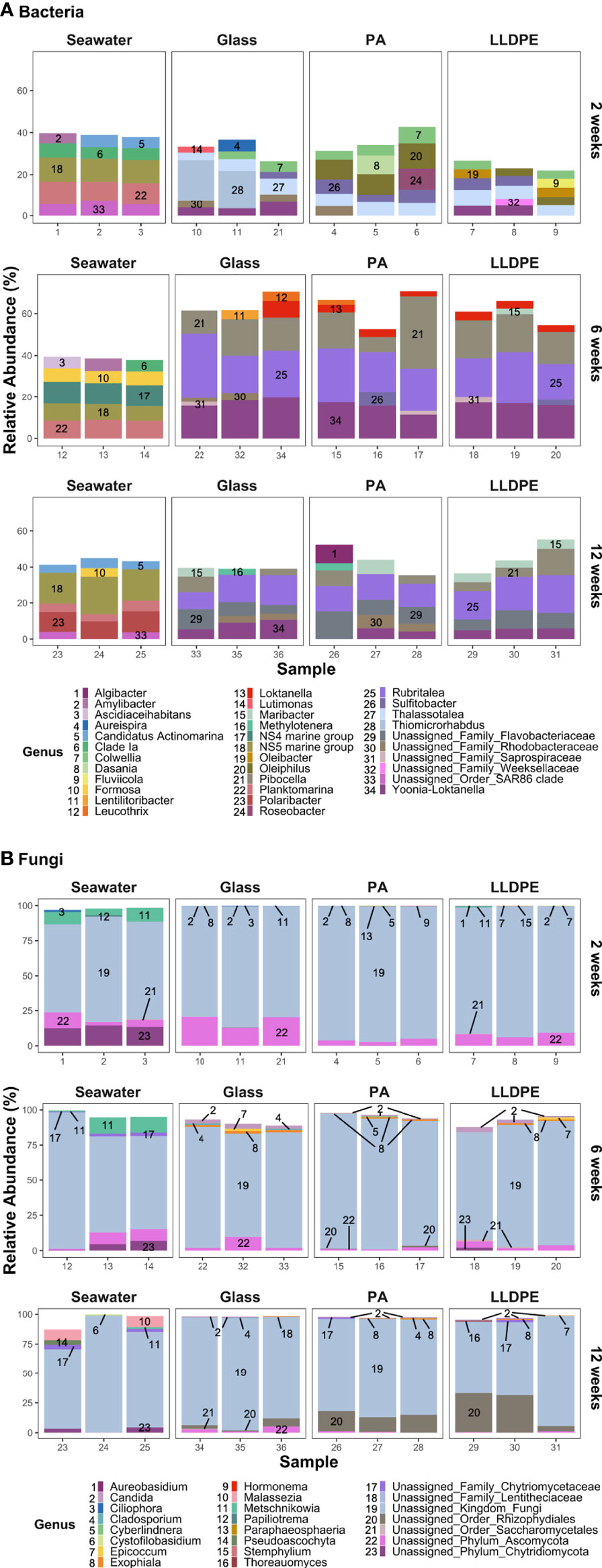
Figure 2 Relative abundances (%) of the five most dominant (A) bacterial and (B) fungal genera within communities in each sample on glass, nylon-6 (PA) and linear low-density polyethylene (LLDPE) and in seawater from the marine environment of Te Whakaraupō-Lyttelton Harbour, Aotearoa-New Zealand. Each bar represents the relative abundance of the five most dominant taxa within each sample from various substrates, collected at different biofilm ages. Each colour and corresponding number represents a particular genus.
In terms of the fungal communities, a common issue faced in this field is an underrepresentation of marine fungal species in databases used for taxonomic assignment, meaning that unassigned genera represent a majority of the five most dominant fungi detected. Other than unassigned fungi, Ciliophora and Metschnikowia were present at relatively high abundances within fungal communities of seawater at 2 weeks (Figure 2B) and 6 weeks. Candida was observed at low abundances after 6 weeks, whereas the abundances of Malassezia and Pseudoascochyta increased in some seawater communities at 12 weeks.
Core Community Analysis
Bacterial ASVs unique to the plastisphere equated to 567, with 177 and 161 ASVs being unique to PA and LLDPE respectively, and 229 shared among both plastics. Glass specific bacterial ASVs totalled 231, with 261 and 276 being shared with PA and LLDPE respectively, showing a similar richness of ASVs were shared among the three solid substrates. Most ASVs were present on more than one substrate. A total of 741 bacterial ASVs were shared across all substrates, 2341 bacterial ASVs appeared to be shared among at least two biofilm substrates/communities, with 1575 ASVs shared among LLDPE, PA and glass communities (Figure 3A). A majority of fungal ASVs appeared to be unique to each substrate, with only 32 fungal ASVs being shared across all substrates and 85 ASVs appeared to be shared between at least two biofilm substrates/communities (Figure 3B). At 2 weeks, 659 bacterial ASVs and 28 fungal ASVs were unique to the plastisphere; however 1313 bacterial ASVs and 34 ASVs fungi were shared across at least three substrates (Figures S7A, H). At 6 weeks, as 788 bacterial ASVs and 114 fungal ASVs unique to the plastisphere were observed (Figures S7B, I). At 12 weeks, microbial communities become more similar as 633 bacterial and 87 fungal ASVs were determined to be unique to the plastisphere (Figures S7C, J). However, at both 6 and 12 weeks more ASVs were unique to the communities associated with the glass slides than with communities associated with either the LLDPE or PA plastics. Few bacterial, and no fungal ASVs unique to each plastic or glass surface were shared across the biofilm ages (Figure S7).
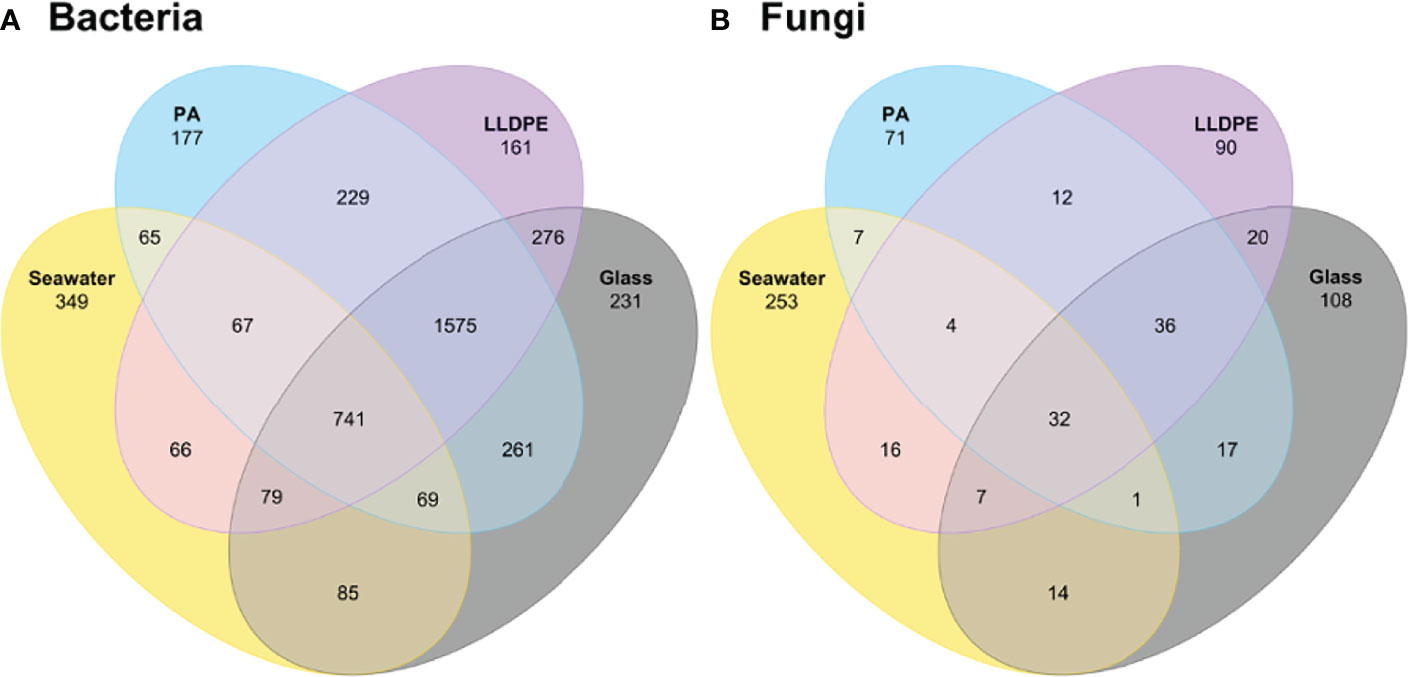
Figure 3 Venn diagrams comparing the observed number of shared (A) bacterial and (B) fungal ASVs present in the surrounding seawater and on glass, nylon-6 (PA) or linear low-density polyethylene (LLDPE) in the marine environment of Te Whakaraupō-Lyttelton Harbour, Aotearoa-New Zealand. Colours represent substrate type and numbers inside ellipses represent the number of shared ASVs.
Bacterial and Fungal ASVs Associated With Biofilms From Specific Substrates or Ages
Indicator species (IndVal) analyses revealed that certain bacterial and fungal ASVs are significantly associated with specific substrates. A total of 590 out of 4431 bacterial ASVs and 41 out of 688 fungal ASVs were suggested to be potential indicators of substrate type, regardless of age. A majority of the fungal ASV indicators were associated with ambient seawater, with only 7 ASVs indicating glass, 1 ASV associated with LLDPE and no PA indicator species were detected; further, the majority of these ASVs were unassigned beyond kingdom. Out of the bacterial indicators of substrate type, 346 ASVs were associated with ambient seawater, 131 ASVs were associated with glass biofilms, 46 ASVs were indicative of PA and 79 ASVs were indicators of LLDPE. Some phyla appear to be more indicative of certain substrates than others. For example, two thirds of the phylum Bacteroidota and all Bdellovibrionota ASVs are indicative of plastic substrates (i.e. rather than glass). All Actinobacteriota, Acidobacteriota and Desulfobacterota appear to be indicative of glass-associated biofilm communities, no obvious clustering based on substrate type can be seen by bacterial phylogenetic analyses (Figure 4). Three ASV indicators of LLDPE were found to be related to taxa previously reported as potential plastic biodegraders, all of which are from the phylum Proteobacteria; two belonging to the genus Alcanivorax and one belonging to the genus Shewanella.
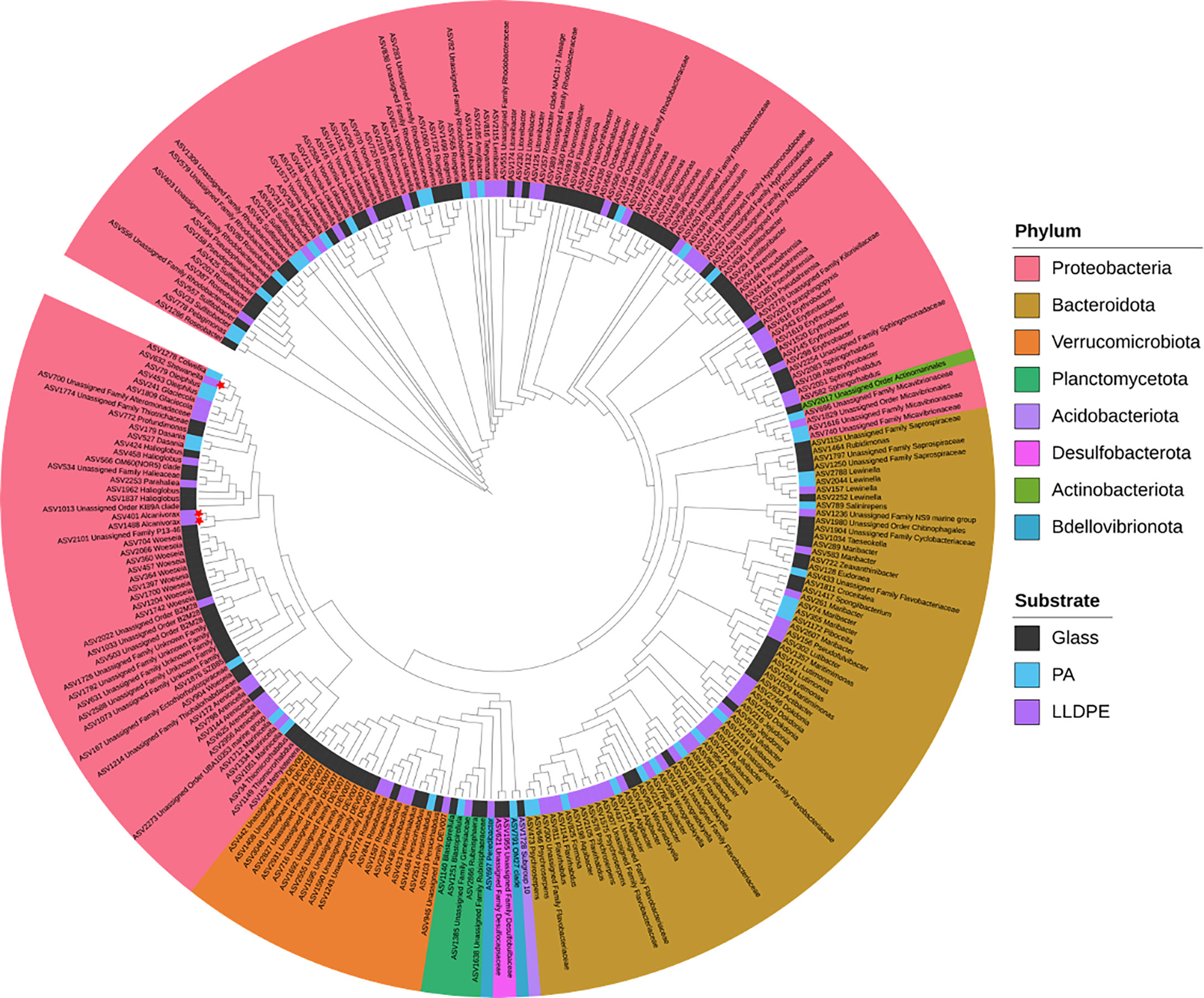
Figure 4 Phylogenetic tree showing bacterial ASVs from biofilms in the marine environment of Te Whakaraupō-Lyttelton Harbour, Aotearoa-New Zealand, identified as indicative of substrate type by indicator species (IndVal) analyses assessed Monte Carlo tests using 999 permutations (P < 0.05). Substrate type is shown by the colours between branches and leaves (i.e. the inner coloured ring), leaves are coloured according to their phylum and red stars represent previously reported plastic biodegraders at genus level. An interactive version of this tree is available at: https://itol.embl.de/tree/13021612675209791628467510.
Screening for Reported Potential Plastic Biodegraders Within Microbial Communities
At the genus level, 24 bacteria and 20 fungi (Figure 5) identified as indicator taxa belonged to the same genus as previously reported plastic biodegraders. At the species level, Stenotrophomonas maltophilia was the only bacterial species identified (Figure S8A) as belonging to a species previously reported to biodegrade plastic, along with five fungal species (Figure S8B). The relatively low abundance of these potential bacterial and fungal plastic biodegraders varied across all sample types and ages, being present on glass as well as LLDPE or PA plastics.
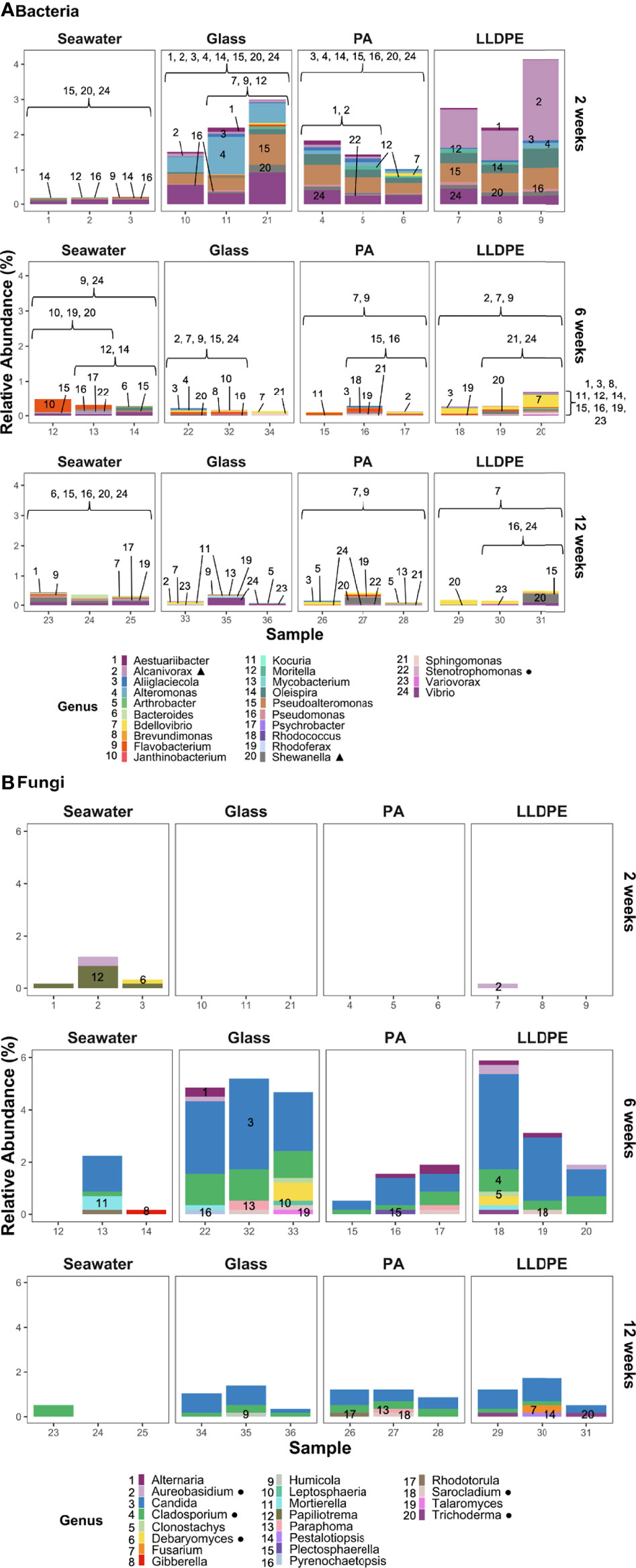
Figure 5 Relative abundance of reported potential plastic-biodegrading genera present within (A) bacterial and (B) fungal communities on glass, nylon-6 (PA) and linear low-density polyethylene (LLDPE) and in seawater from the marine environment of Te Whakaraupō-Lyttelton Harbour, Aotearoa-New Zealand. Each bar represents the stacked relative abundance for each sample from various substrates of different biofilm ages. Each colour and corresponding number represents a particular genus. Triangles (▲) represent genera containing ASVs determined in this study as potential indicators of LLDPE. Circles (●) represent genera that contain microbes identified as a reported plastic biodegrader at the species level.
Vibrio spp. were abundant across all samples at 2 weeks, along with Pseudoalteromonas, Alteromonas, Alcanivorax and Aestuariibacter spp. (Figure 5A). Oleispira and Shewanella spp. were also abundant, mainly in PA and LLDPE samples at 2 weeks. Flavobacterium spp. became more abundant across all samples at 6 weeks, with Bdellovibrio spp. observed in glass, PA and LLDPE samples at 6 weeks, remaining in some PA and LLDPE samples up to 12 weeks. Although Shewanella and Vibrio spp. were also detected at 12 weeks, fewer bacterial genera previously reported to be plastic biodegraders were identified at 12 weeks, especially on plastics. While Papiliotrema spp. were abundant in seawater fungal communities, fewer fungal genera were identified as reported plastic biodegraders at 2 and 12 weeks (Figure 5B). A greater diversity of fungi were detected at 6 weeks, with a high relative abundance of Candida observed across all substrates. Cladosporium spp. were also abundant in the fungal biofilm communities at 6 weeks; Mortierella spp. were present in low abundance. At 6 weeks, Sarocladium spp. appears in biofilms, Plectosphaerella spp. was unique to PA and Trichoderma appeared to be present in LLDPE alone. A smaller relative abundance of putative plastic-degrading taxa were detected at 12 weeks. Paraphoma and Sarocladium spp. were unique to PA, and Fusarium and Pestalotiopsis spp. were unique to LLDPE. Candida spp. and Cladosporium spp. were associated with all three solid substrate types (i.e., including glass).
Species-level findings revealed extremely low abundances of the bacterium Stenotrophomonas maltophilia in PA samples at 2 and 12 weeks and at 6 weeks in seawater samples (Figure S8A). This species has been reported to be a putative plastic-biodegrading bacterium. Fungal species reported as plastic biodegraders were more abundant than bacterial plastic biodegraders, although most were associated with glass samples at 6 weeks, rather than plastic substrates (Figure S8B). Of the five identified plastic-biodegrading species, only Trichoderma viride appeared to be specific to the plastisphere, present in three LLDPE samples, one at 6 weeks and two at 12 weeks. Low abundances of potential plastic biodegraders across the plastic and glass substrates suggest these microorganisms are predominantly utilising the surface of these substrates to form biofilm communities, more so than to gain metabolic benefit from the plastics.
Discussion
The microbial colonisation of plastics established in the marine environment has been well-documented (Carpenter and Smith, 1972) but there is a lack of study identifying the community establishment over time. To address this knowledge gap, we explored temporal changes in bacterial and fungal communities following the deployment of two plastic polymer substrates (as well as glass slides) into the marine environment of Te Whakaraupo-Lyttelton Harbour, Aotearoa-New Zealand. We found distinct differences between communities in the water column and surface colonisers forming a biofilm at all timepoints, supporting the findings of Zettler et al. (2013) and Dussud et al. (2018). No significant difference in diversity was observed between communities on glass and the two plastic types tested at any timepoint. We did however, establish that surface associated microbial communities changed over time across all surfaces, as previously found by Oberbeckmann et al. (2016) and Xu et al. (2019). More taxa related to putative-plastic biodegraders were found on glass, LLDPE and PA at weeks two and six, for bacteria and fungi, respectively. However, we found no evidence that putative plastic degrading taxa were more abundant on any one substrate.
Biodegradation of polymers by microbial communities can include biodeterioration, bio-fragmentation, mineralisation and assimilation, as defined by Zeenat et al. (2021). We identified the presence of certain species and genera within our marine samples that might be capable of biodegrading these plastics via, noting that mineralisation and assimilation have yet to be directly determined in the marine environment. These ASVs were shared amongst glass, LLDPE and PA-associated communities, with the most abundant putative biodegraders being identified on multiple substrates (e.g., Vibrio spp.). Biofilm age appeared to have a significant impact on the diversity of proposed plastic-degraders present, rather than substrate type. Once immersed in the marine environment, organic matter builds up rapidly forming a conditioning film on any solid substrate, promoting microbial colonisation (Marshall, 1985; Galloway et al., 2017). This has been shown to occur within a matter of minutes following immersion (Harrison et al., 2014, Wright et al., 2020b) and provides a nutrient-enriched environment for biofilm-forming taxa. Factors including surface roughness and hydrophilicity may impact rates of surface conditioning and also subsequent rates of colonisation on different substrates (Bhagwat et al., 2021). However, the results of our study suggest immersion time, rather than substrate composition, was the more important determinant of biofilm community composition.
Although the plastics used in our study were not artificially aged by exposure to elevated UV light or temperature, it is likely that some carbon present on, and within, the virgin plastic surface is biologically accessible to colonising microbes in the form of short chain oligomers and monomers (Gewert et al., 2018; Romera-Castillo et al., 2018; Erni-Cassola et al., 2020). Monomers and short-chain polymers may leach out of plastic quickly (Kwon et al., 2015). Further, organic additives to plastic substrates added during manufacturing are not bound to the polymer and may be leached into the environment and used as a carbon or nutrient source by the plastisphere community (Wright et al., 2020a). The additives present in the PA and LLDPE used in this study (Nylostab S-EED and Irganox B215 (Irganox 1010 and Irgafos 168)), which are typically included to promote UV light stability and prevent physicochemical degradation, are not readily biodegradable according to their MSDS data. However, such additives may impact microbes trying to establish on the plastics, influencing the communities that are able to survive. An enormous diversity of chemical additives are currently incorporated into plastics such as LDPE (Thomas et al., 2012) including, in the case of ‘oxy-degradable’ plastics, metal salts of iron or manganese (Thomas et al., 2012) intended to catalyse abiotic plastic degradation. The sheer diversity of plastic additives used means that very different microbial community responses may be witnessed, even in reference to the same base polymer in the same environment. In addition, diverse contaminants within the ambient seawater may sorb to plastics, impacting community composition and functioning variably depending on their concentrations in the environment under investigation. Unfortunately, the diversity of environmental contaminants present was not assessed in the present study and so any related impacts caused by variation across substrate type and sampling time could not be quantified.
Research has previously indicated that plastic degradation, where observed, is likely to slow after the early stages of colonisation or immersion (Datta et al., 2016; Enke et al., 2018; Wright et al., 2019, Wright et al., 2020b), which could explain the greater relative abundance of reported bacterial plastic-biodegraders in the first weeks after immersion of plastics into seawater. Some studies demonstrate biofilm maturation after days to over a week (Erni-Cassola et al., 2020, Wright et al., 2020b), with the fast rates of colonisation and succession perhaps explaining the low relative abundances of putative plastic-biodegrading taxa in our samples as generalist taxa out-compete pioneer species for space and resources. Further, as succession occurred, the biofilms increased in thickness due to an increased abundance of microalgae (represented in Figure S9, not all data shown) as is commonly observed (Eich et al., 2015). The increased biomass of taxa such as algae, that are not known to mineralise and assimilate plastics, would provide an alternative attachment substrate for the bacteria. This means a decreased proportion of the bacterial community would be in direct contact with the plastic surface over time, and they would also be less exposed to any toxic substances associated with the plastic polymer, as proposed by Ward et al. (2022). It is likely that a combination of these explanations is responsible for shaping the communities of putative plastic-degrading taxa in the marine environment. Temporal abundance patterns of putative plastic-degrading microbes were similar on plastic substrates as to those observed on inert glass slides. Thus, it remains unlikely that observed increases of presumed plastic-degrading taxa on the submerged plastic substrates were driven by community changes in response to the metabolism of the plastic polymers or their additives.
While providing a useful avenue for further exploration, we did not seek to confirm evidence of plastic biodegradation in the present study. At least 286 bacteria and 150 fungi have been reported as plastic biodegraders (Gambarini et al., 2021) yet it is often unclear whether or not these microbes are responsible for the biodegradation of the plastic polymer itself, monomers and short-chain polymers that are quickly leached from the plastic, or any additives present. To assess the putative plastic biodegradation potential of these communities would require additional physicochemical assessment of the plastics, as outlined in Lear et al. (2021). However, the identification of putative plastic biodegrading taxa, or closely related species and strains by taxonomic analysis, as used in this study, nevertheless provides a useful mechanism to identify environments, and timescales, for more targeted analyses of plastic biodegrading organisms and enzymes. PCR biases prevent the amplification of DNA from certain taxa, including putative plastic-biodegrading strains but the inclusion of mock-community extraction and PCR controls as recommended by Lear et al. (2018) might help to quantify the extent of such biases. Omitting the need for PCR and its associated biases, future investigations into the metagenomes or metatranscriptomes of these microbes and communities may reveal additional taxonomic details. Using genomics and proteomic profiling, Oberbeckmann et al. (2021) reported that the properties of polyethylene and polystyrene pellets had little influence on their associated microbial communities, they were similar on both substrate types. Future community-based assessments, including from metagenomics and proteomics data, as used by Oberbeckmann et al. (2021), may further help to identify appropriate sites for the later enrichment and isolation of potential plastic biodegrading organisms. In particular, confirmation of the presence of complete plastic biodegradation pathways, along with their corresponding regulatory mechanisms could help to identify environments and sites for the mining of novel plastic degrading genes and taxa.
We were not able to identify any fungal ASVs indicative of plastic-specific biofilms (i.e. none were exclusively or reliably found on any one plastic substrate). However, the presence of the previously reported plastic biodegrading bacterial genera Alcanivorax and Shewanella suggests that they attach more readily to LLDPE or grow at a faster rate on this substrate. Whilst Delacuvellerie et al. (2019) propose Alcanivorax borkumensis as a biodegrader of low-density polyethylene (LDPE), many other studies were unable to determine the identity of putative bacterial or fungal biodegraders of plastic past the genus level (Sekiguchi et al., 2011). Alcanivorax, unidentified at the species level, have been reported to biodegrade polycaprolactone (PCL) (Sekiguchi et al., 2011) and poly-β-hydroxybutyric acid (PHB) (Suzuki et al., 2017). Sekiguchi et al. (2011) also reported an unidentified Shewanella with the ability to biodegrade PCL in the marine environment of Japan. Shewanella isolated from soil has also been reported to biodegrade PHB by producing clear zones in agar infused with a PHB emulsion (Sung et al., 2016; Suzuki et al., 2017). Without clear taxonomic classification to the species level in both the samples from this study and reported putative biodegraders, it remains challenging to verify that the species we detected are the same as those reported in other studies. This limits our ability to elucidate that species present in our samples have the potential to be plastic biodegraders. Further, since the abundance of putative plastic-degraders was equally high in glass-associated biofilms it remains possible that none of the taxa identified have the capability to metabolise PA or LLDPE. Hundreds of publications identify putative PA and LDPE-degrading taxa, yet Lear et al. (2022) recently postulated that, in fact, there remains no strong evidence for their microbial degradation.
Gangoiti et al. (2012); Jeon and Kim (2013) and Montazer et al. (2018) reported the mesophilic bacterium Stenotrophomonas maltophilia to be a potential biodegrader of poly(3-hydroxydecanoate) (P3HO), PLA and LDPE, respectively, after isolating this bacterium from soil or landfill. S. maltophilia was identified in PA biofilm samples from this study, suggesting that this bacterium may form specific associations with PA in the marine environment. The fungus Aureobasidium pullulans was also present in seawater, glass and LLDPE biofilm samples from this study, and has previously been reported to biodegrade five different plastics: polyurethane (PU) (Crabbe et al., 1994), PCL (Fields et al., 1974), PHB, PLA (Jeszeová et al., 2018) and a polyvinyl chloride (PVC) blend (Webb et al., 2000). The pathogenic fungus Cladosporium cladosporioides, present in our samples across all substrates at 6 and 12 weeks, is reported to biodegrade polyethylene (PE) (Bonhomme et al., 2003), polyethylene terephthalate (PET) (Breuker et al., 2003) and PU (Brunner et al., 2018). Additionally, Gonda et al. (2000) reported that the fungus Debaryomyces hansenii, found in the North Sea, biodegraded PHB, as did Matavulj and Molitoris (2009), who reported the biodegradation of poly-3-hydroxyalkanoate (PHA) by D. hansenii. Sarocladium kiliense is another fungus identified within our samples, which has been reported to biodegrade PE (Karlsson et al., 1988), whilst Trichoderma viride, also found in soil and landfill material, has been reported to biodegrade PLA (Lipsa et al., 2016) and LDPE (Munir et al., 2018). In general, we found organisms previously reported to degrade plastics to only be present in extremely low relative abundances and they were shared throughout the glass, LLDPE and PA communities at each timepoint, indicating their presence and abundance were not substrate specific. Despite identifying numerous taxa related to proposed plastic degrading bacteria and fungi among our samples, it is important to note that many presumed plastic degraders were previously exposed to plastics subjected to quite specific laboratory conditions, including exposure to elevated temperature (e.g., Koutny et al., 2006) and UV light (e.g., Karlsson et al., 1988). These conditions are chosen to purposefully alter the physicochemical structure of the polymer. Significant polymer degradation is rarely or never demonstrated under ambient environmental conditions (Lear et al., 2022) and might not be possible under the natural conditions to which our plastics were exposed.
Studies with a much shorter range of incubation lengths [i.e. sampling from days to weeks, rather than over seasons or months as conducted by Xu et al. (2019) and De Tender et al. (2017)] are desirable to determine if microbial substrate specificity for different plastics could occur during the very early stages of colonisation and succession. Indeed, Ward et al. (2022) suggest that some early- to mid-stage colonizers associated with hydrocarbon degradation may contribute to the degradation of plastic leachate before early colonisers are replaced by ‘cheaters’ (Datta et al., 2016) which may not produce plastic- (or additive) degrading enzymes, but scavenge the products of their degradation. In addition to conducting studies over a broader range of incubation times, future investigations may benefit from including a wider range of plastic substrates, with additive combinations to control for their effects. This would help to determine whether plastisphere communities are unique to each plastic or are largely consistent across all substrates, as indicated here. Including different plastic conditions such as ‘aged’ plastics may also be useful since plastic weathering causes carbon availability to increase, as aged plastics contain a greater abundance of short chain polymers, compared to that of non-aged plastics (Gewert et al., 2018; Romera-Castillo et al., 2018; Erni-Cassola et al., 2020).
There remains a limited number of studies regarding the functional potential of the plastisphere (Lear et al., 2021). Oberbeckmann et al. (2021) determined proteomic and genomic profiles from microbial communities were not specific to PE and PS polymers, suggesting plastic type has a limited influence on plastisphere functional potentials. However, variability in the chemical composition of plastics, including additives and contaminants, may be expected to elicit different functional profiles in the communities colonising plastic surfaces. As we propose that immature plastispheres are more likely to contain plastic-biodegrading microbes, it is important to investigate the expression of known genes conferring plastic-biodegradation potential within these earlier communities. via Metatranscriptomic studies focussing on earlier timepoints would allow for the investigation of gene expression during colonisation, such as those relating to biofilm formation, adherence/surface attachment, type IV pilus expression, quorum sensing, flagella production and motility (Vu et al., 2009; Belas, 2014; Rampadarath et al., 2017). Analysis of differential expression to first determine whether the expression of certain genes is being up/down-regulated in the presence of plastics, as well as between plastic types, followed by establishing at which biofilm age(s) are these patterns being observed, would provide further evidence as to if taxa previously identified as putative plastic biodegraders do have the potential to biodegrade plastic. The isolation and testing of individual taxa identified for their plastic-biodegrading attributes would then be essential for characterisation of novel plastic-biodegrading organisms. Even if plastic biodegraders with limited degradation capacity are identified, prior studies indicate that enzymatic degradative capabilities might be enhanced following genetic modification (Yoshida et al., 2016; Austin et al., 2018). Such investigations would widen our understanding of the plastisphere and bring us closer to identifying the impact of these communities on the microbial ecology and functioning of our oceans, as well as identifying and characterising further microbes with plastic-biodegradation potentials.
Conclusion
We investigated the influence of two plastic polymers on the diversity and composition of surface-associated microbial communities in the marine environment using 16S rRNA gene and ITS2 region amplicon sequencing. Biofilm-associated communities (glass and plastic) significantly differed from communities found in ambient seawater, and age of the biofilm appeared to be a significant factor in determining community composition, as also recently reported by Ward et al. (2022). In particular, within our plastisphere communities, bacteria and fungi appeared to be more unique at two and six weeks of immersion, respectively, with these communities containing microbes previously reported as having plastic biodegradation potential. To our knowledge, this is the first study to investigate the plastisphere in the marine environment of Aotearoa-New Zealand. Our results suggest that communities attaching to plastic in the marine environment include species reported to have the potential for plastic biodegradation, yet these were no more abundant than in glass-associated biofilm communities. Rather, our research indicates that microbial degradation is not likely to be a dominant process in marine environments. To gain a more comprehensive understanding of the potential of the marine plastisphere, analysis of communities from shorter immersion durations and incorporating more plastic substrates and conditions is necessary, alongside analysis of metagenomic data, to confirm the presence of genes known or suspected to confer plastic biodegradation traits.
Data Availability Statement
The datasets presented in this study can be found in the Sequence Read Archive (SRA) of National Centre for Biotechnology Information (NCBI) under the accession number PRJNA764817 and ID 764817.
Author Contributions
All authors contributed to the research, writing and editing of this manuscript and provided their full consent for publication.
Funding
This work was conducted as part of the Aotearoa Impacts and Mitigation of Microplastics (AIM2) project, in receipt of funds from a New Zealand Ministry of Business, Innovation and Employment (MBIE) Endeavour Research Programme grant (C03X1802).
Conflict of Interest
The authors declare that the research was conducted in the absence of any commercial or financial relationships that could be construed as a potential conflict of interest.
Publisher’s Note
All claims expressed in this article are solely those of the authors and do not necessarily represent those of their affiliated organizations, or those of the publisher, the editors and the reviewers. Any product that may be evaluated in this article, or claim that may be made by its manufacturer, is not guaranteed or endorsed by the publisher.
Acknowledgments
We wish to acknowledge our colleague Pierre-Yves Dupont who passed away suddenly during the writing of this article and thank him for his involvement in the initial community analysis of these samples. We acknowledge the Lyttelton Port Company (LPC) for allowing the deployment of and access to the structure to collect our environmental data. New Zealand eScience Infrastructure (NeSI) provided computing resources for our bioinformatic analyses. This study was supported by the Aotearoa Impacts and Mitigation of Microplastics (AIM2) project (Ministry of Business, Innovation, and Employment, New Zealand, Endeavour Fund C03X1802).
Supplementary Material
The Supplementary Material for this article can be found online at: https://www.frontiersin.org/articles/10.3389/fmars.2022.841142/full#supplementary-material
References
Abarenkov K., Zirk A., Piirmann T., Pöhönen R., Ivanov F., Nilsson H. R., et al (2021). UNITE General FASTA Release for Fungi (UNITE Community). Ed. Community U.. doi: 10.15156/BIO/1280049
Allen S., Allen D., Phoenix V. R., Le Roux G., Durántez Jiménez P., Simonneau A., et al (2019). Atmospheric Transport and Deposition of Microplastics in a Remote Mountain Catchment. Nat. Geosci. 12, 339–344. doi: 10.1038/s41561-019-0335-5
Anderson M. J., Gorley R. N., Clarke K. R. (2008). PERMANOVA+ for PRIMER: Guide to Software and Statistical Methods (UK: PRIMER-E, Plymouth).
Austin H. P., Allen M. D., Donohoe B. S., Rorrer N. A., Kearns F. L., Silveira R. L., et al (2018). Characterization and Engineering of a Plastic-Degrading Aromatic Polyesterase. Proc. Natl. Acad. Sci. 115, E4350. doi: 10.1073/pnas.1718804115
Belas R. (2014). Biofilms, Flagella, and Mechanosensing of Surfaces by Bacteria. Trends Microbiol. 22, 517–527. doi: 10.1016/j.tim.2014.05.002
Besseling E., Foekema E. M., Van Franeker J. A., Leopold M. F., Kuhn S., Bravo Rebolledo E. L., et al (2015). Microplastic in a Macro Filter Feeder: Humpback Whale Megaptera Novaeangliae. Mar. Poll. Bull. 95, 248–252. doi: 10.1016/j.marpolbul.2015.04.007
Bhagwat G., O’Connor W., Grainge I., Palanisami T. (2021). Understanding the Fundamental Basis for Biofilm Formation on Plastic Surfaces: Role of Conditioning Films. Front. Microbiol. 12. doi: 10.3389/fmicb.2021.687118
Bolger A. M., Lohse M., Usadel B. (2014). Trimmomatic: A Flexible Trimmer for Illumina Sequence Data. Bioinformatics 30, 2114–2120. doi: 10.1093/bioinformatics/btu170
Bonhomme S., Cuer A., Delort A. M., Lemaire J., Sancelme M., Scott G. (2003). Environmental Biodegradation of Polyethylene. Polym. Degrad. Stab. 81, 441–452. doi: 10.1016/S0141-3910(03)00129-0
Borrelle S. B., Ringma J., Law K. L., Monnahan C. C., Lebreton L., McGivern A., et al (2020). Predicted Growth in Plastic Waste Exceeds Efforts to Mitigate Plastic Pollution. Science 369, 1515–1518. doi: 10.1126/science.aba3656
Breuker M., McNamara C., Young L., Perry T., Young A., Mitchell R. (2003). Fungal Growth on Synthetic Cloth From Apollo Spacesuits. Ann. Microbiol. 53, 47–54.
Brunner I., Fischer M., Rüthi J., Stierli B., Frey B. (2018). Ability of Fungi Isolated From Plastic Debris Floating in the Shoreline of a Lake to Degrade Plastics. PloS One 13, e0202047. doi: 10.1371/journal.pone.0202047
Bryant J. A., Clemente T. M., Viviani D. A., Fong A. A., Thomas K. A., Kemp P., et al (2016). Diversity and Activity of Communities Inhabiting Plastic Debris in the North Pacific Gyre. mSystems 1, e00024–16. doi: 10.1128/mSystems.00024-16
Callahan B. J., McMurdie P. J., Rosen M. J., Han A. W., Johnson A. J., Holmes S. P. (2016). DADA2: High-Resolution Sample Inference From Illumina Amplicon Data. Nat. Methods 13, 581–583. doi: 10.1038/nmeth.3869
Carpenter E. J., Smith K. L. Jr. (1972). Plastics on the Sargasso Sea Surface. Science 175, 1240–1241. doi: 10.1126/science.175.4027.1240
Crabbe J. R., Campbell J. R., Thompson L., Walz S. L., Schultz W. W. (1994). Biodegradation of a Colloidal Ester-Based Polyurethane by Soil Fungi. Int. Biodeterior. Biodegradation 33, 103–113. doi: 10.1016/0964-8305(94)90030-2
Datta M. S., Sliwerska E., Gore J., Polz M. F., Cordero O. X. (2016). Microbial Interactions Lead to Rapid Micro-Scale Successions on Model Marine Particles. Nat. Commun. 7, 1–7. doi: 10.1038/ncomms11965
Delacuvellerie A., Cyriaque V., Gobert S., Benali S., Wattiez R. (2019). The Plastisphere in Marine Ecosystem Hosts Potential Specific Microbial Degraders Including Alcanivorax Borkumensis as a Key Player for the Low-Density Polyethylene Degradation. J. Hazard. Mater. 380, 120899. doi: 10.1016/j.jhazmat.2019.120899
De Tender C., Devriese L. I., Haegeman A., Maes S., Vangeyte J., Cattrijsse A., et al (2017). Temporal Dynamics of Bacterial and Fungal Colonization on Plastic Debris in the North Sea. Environ. Sci. Technol. 51, 7350–7360. doi: 10.1021/acs.est.7b00697
Dufrêne M., Legendre P. (1997). Species Assemblages and Indicator Species: The Need for a Flexible Asymmetrical Approach. Ecol. Monogr. 67, 345–366. doi: 10.2307/2963459
Dussud C., Meistertzheim A. L., Conan P., Pujo-Pay M., George M., Fabre P., et al (2018). Evidence of Niche Partitioning Among Bacteria Living on Plastics, Organic Particles and Surrounding Seawaters. Environ. Poll. 236, 807–816. doi: 10.1016/j.envpol.2017.12.027
Eich A., Mildenberger T., Laforsch C., Weber M. (2015). Biofilm and Diatom Succession on Polyethylene (PE) and Biodegradable Plastic Bags in Two Marine Habitats: Early Signs of Degradation in the Pelagic and Benthic Zone? PloS One 10, e0137201–e0137201. doi: 10.1371/journal.pone.0137201
Enke T. N., Leventhal G. E., Metzger M., Saavedra J. T., Cordero O. X. (2018). Microscale Ecology Regulates Particulate Organic Matter Turnover in Model Marine Microbial Communities. Nat. Commun. 9, 1–8. doi: 10.1038/s41467-018-05159-8
Erni-Cassola G., Wright R. J., Gibson M. I., Christie-Oleza J. A. (2020). Early Colonization of Weathered Polyethylene by Distinct Bacteria in Marine Coastal Seawater. Microb. Ecol. 79, 517–526. doi: 10.1007/s00248-019-01424-5
Erni-Cassola G., Zadjelovic V., Gibson M. I., Christie-Oleza J. A. (2019). Distribution of Plastic Polymer Types in the Marine Environment; A Meta-Analysis. J. Hazard. Mater. 369, 691–698. doi: 10.1016/j.jhazmat.2019.02.067
Fields R. D., Rodriguez F., Finn R. K. (1974). Microbial Degradation of Polyesters: Polycaprolactone Degraded by P. Pullulans. J. Appl. Polym. Sci. 18, 3571–3579. doi: 10.1002/app.1974.070181207
Galloway T. S., Cole M., Lewis C. (2017). Interactions of Microplastic Debris Throughout the Marine Ecosystem. Nat. Ecol. Evol. 1, 0116. doi: 10.1038/s41559-017-0116
Gambarini V., Pantos O., Kingsbury J. M., Weaver L., Handley K. M., Lear G. (2021). Phylogenetic Distribution of Plastic-Degrading Microorganisms. mSystems 6, e01112–20. doi: 10.1128/mSystems.01112-20
Gambarini V., Pantos O., Kingsbury J. M., Weaver L., Handley K. M., Lear G. (2022). PlasticDB: A Database of Microorganisms and Proteins Linked to Plastic Biodegradation. Database 2022. doi: 10.1093/database/baac008
Gangoiti J., Santos M., Prieto M. A., de la Mata I., Serra J. L., Llama M. J. (2012). Characterization of a Novel Subgroup of Extracellular Medium-Chain-Length Polyhydroxyalkanoate Depolymerases From Actinobacteria. Appl. Environ. Microbiol. 78, 7229–7237. doi: 10.1128/AEM.01707-12
Gewert B., Plassmann M., Sandblom O., MacLeod M. (2018). Identification of Chain Scission Products Released to Water by Plastic Exposed to Ultraviolet Light. Environ. Sci. Technol. Lett. 5, 272–276. doi: 10.1021/acs.estlett.8b00119
Gonda K. E., Jendrossek D., Molitoris H. P. (2000). Fungal Degradation of the Thermoplastic Polymer Poly-ß-Hydroxybutyric Acid (PHB) Under Simulated Deep Sea Pressure (Dordrecht: Springer Netherlands), 173–183.
Groh K. J., Backhaus T., Carney-Almroth B., Geueke B., Inostroza P. A., Lennquist A., et al (2019). Overview of Known Plastic Packaging-Associated Chemicals and Their Hazards. Sci. Total Environ. 651, 3253–3268. doi: 10.1016/j.scitotenv.2018.10.015
Guzzetti E., Sureda A., Tejada S., Faggio C. (2018). Microplastic in Marine Organism: Environmental and Toxicological Effects. Environ. Toxicol. Pharmacol. 64, 164–171. doi: 10.1016/j.etap.2018.10.009
Hahladakis J. N., Velis C. A., Weber R., Iacovidou E., Purnell P. (2018). An Overview of Chemical Additives Present in Plastics: Migration, Release, Fate and Environmental Impact During Their Use, Disposal and Recycling. J. Hazard. Mater. 344, 179–199. doi: 10.1016/j.jhazmat.2017.10.014
Hammond M., Nunn H., Rogers G., Lee H., Marghitoiu A.-L., Perez L., et al (2013). Identification of a Leachable Compound Detrimental to Cell Growth in Single-Use Bioprocess Containers. PDA J. Pharm. Sci. Technol. 67, 123. doi: 10.5731/pdajpst.2013.00905
Harrison J. P., Schratzberger M., Sapp M., Osborn A. M. (2014). Rapid Bacterial Colonization of Low-Density Polyethylene Microplastics in Coastal Sediment Microcosms. BMC Microbiol. 14, 232. doi: 10.1186/s12866-014-0232-4
Hoellein T., Rojas M., Pink A., Gasior J., Kelly J. (2014). Anthropogenic Litter in Urban Freshwater Ecosystems: Distribution and Microbial Interactions. PloS One 9, e98485. doi: 10.1371/journal.pone.0098485
Huang L., McCutcheon J. R. (2014). Hydrophilic Nylon 6,6 Nanofibers Supported Thin Film Composite Membranes for Engineered Osmosis. J. Membr. Sci. 457, 162–169. doi: 10.1016/j.memsci.2014.01.040
Jamieson A. J., Brooks L. S. R., Reid W. D. K., Piertney S. B., Narayanaswamy B. E., Linley T. D. (2019). Microplastics and Synthetic Particles Ingested by Deep-Sea Amphipods in Six of the Deepest Marine Ecosystems on Earth. R. Soc. Open Sci. 6, 180667. doi: 10.1098/rsos.180667
Jeon H. J., Kim M. N. (2013). Biodegradation of Poly(L-Lactide) (PLA) Exposed to UV Irradiation by a Mesophilic Bacterium. Int. Biodeterior. Biodegradation 85, 289–293. doi: 10.1016/j.ibiod.2013.08.013
Jeszeová L., Puškárová A., Bučková M., Kraková L., Grivalský T., Danko M., et al (2018). Microbial Communities Responsible for the Degradation of Poly(Lactic Acid)/Poly(3-Hydroxybutyrate) Blend Mulches in Soil Burial Respirometric Tests. World J. Microbiol. Biotechnol. 34, 101. doi: 10.1007/s11274-018-2483-y
Karlsson S., Ljungquist O., Albertsson A. C. (1988). Biodegradation of Polyethylene and the Influence of Surfactants. Polym. Degrad. Stab. 21, 237–250. doi: 10.1016/0141-3910(88)90030-4
Kettner M. T., Rojas-Jimenez K., Oberbeckmann S., Labrenz M., Grossart H. P. (2017). Microplastics Alter Composition of Fungal Communities in Aquatic Ecosystems. Environ. Microbiol. 19, 4447–4459. doi: 10.1111/1462-2920.13891
Koutny M., Sancelme M., Dabin C., Pichon N., Delort A. M., Lemaire J. (2006). Acquired Biodegradability of Polyethylenes Containing Pro-Oxidant Additives. Polym. Degrad. Stab. 91, 1495–1503. doi: 10.1016/j.polymdegradstab.2005.10.007
Kozich J. J., Westcott S. L., Baxter N. T., Highlander S. K., Schloss P. D. (2013). Development of a Dual-Index Sequencing Strategy and Curation Pipeline for Analyzing Amplicon Sequence Data on the MiSeq Illumina Sequencing Platform. Appl. Environ. Microbiol. 79, 5112–5120. doi: 10.1128/AEM.01043-13
Kwon B. G., Koizumi K., Chung S. Y., Kodera Y., Kim J. O., Saido K. (2015). Global Styrene Oligomers Monitoring as New Chemical Contamination From Polystyrene Plastic Marine Pollution. J. Hazard. Mater. 300, 359–367. doi: 10.1016/j.jhazmat.2015.07.039
Laist D. (1997). Impacts of Marine Debris: Entanglement of Marine Life in Marine Debris Including a Comprehensive Ist of Species With Entanglement and Ingestion Records in Marine Debris- Sources, Impacts and Solutions. eds. Coe J. M., Rogers B. (New York, NY: Springer). doi: 10.1007/978-1-4613-8486-1_10
Lear G., Dickie I., Banks J. C., Boyer S., Buckley H. L., Buckley T. R., et al (2018). Methods for the Extraction, Storage, Amplification and Sequencing of DNA From Environmental Samples. N. Z. J. Ecol. 42, 10–50A. doi: 10.20417/nzjecol.42.9
Lear G., Kingsbury J. M., Franchini S., Gambarini V., Maday S. D. M., Wallbank J. A., et al (2021). Plastics and the Microbiome: Impacts and Solutions. Environ. Microbiome 16, 2. doi: 10.1186/s40793-020-00371-w
Lear G., Maday S. D. M., Gambarini V., Northcott G., Abbel R., Kingsbury J., et al (2022). Microbial Abilities to Degrade Our Global Environmental Plastic Polymer Waste are Overstated. Environ. Res. Lett. 17, 043002. doi: 10.1088/1748-9326/ac59a7
Li H.-X., Getzinger G. J., Ferguson P. L., Orihuela B., Zhu M., Rittschof D. (2016). Effects of Toxic Leachate From Commercial Plastics on Larval Survival and Settlement of the Barnacle Amphibalanus Amphitrite. Environ. Sci. Technol. 50, 924–931. doi: 10.1021/acs.est.5b02781
Lipsa R., Tudorachi N., Darie-Nita R. N., Oprică L., Vasile C., Chiriac A. (2016). Biodegradation of Poly(Lactic Acid) and Some of its Based Systems With Trichoderma Viride. Int. J. Biol. Macromol. 88, 515–526. doi: 10.1016/j.ijbiomac.2016.04.017
Lu X., Zhang C., Han Y. (2004). Low-Density Polyethylene Superhydrophobic Surface by Control of its Crystallization Behavior. Macromol. Rapid Commun. 25, 1606–1610. doi: 10.1002/marc.200400256
Marshall K. C. (1985). “Mechanisms of Bacterial Adhesion at Solid-Water Interfaces,” in Bacterial Adhesion: Mechanisms and Physiological Significance. Eds. Savage D. C., Fletcher M. (Boston, MA: Springer US), 133–161.
Martin M. (2011). Cutadapt Removes Adapter Sequences From High-Throughput Sequencing Reads. EMBnetjournal 17, 3. doi: 10.14806/ej.17.1.200
Matavulj M., Molitoris H. P. (2009). Marine Fungi: Degraders of Poly-3-Hydroxyalkanoate Based Plastic Materials. Zbornik Matice srpske za prirodne nauke. 115, 253–265. doi: 10.2298/ZMSPN0916253M
McLaren M. R. (2020). Silva SSU Taxonomic Training Data Formatted for DADA2 (Silva Version 138) (Zenodo). doi: 10.5281/zenodo.4587955
Montazer Z., Habibi-Najafi M. B., Mohebbi M., Oromiehei A. (2018). Microbial Degradation of UV-Pretreated Low-Density Polyethylene Films by Novel Polyethylene-Degrading Bacteria Isolated From Plastic-Dump Soil. J. Polym. Environ. 26, 3613–3625. doi: 10.1007/s10924-018-1245-0
Munir E., Harefa R. S. M., Priyani N., Suryanto D. (2018). Plastic Degrading Fungi Trichoderma Viride and Aspergillus Nomius Isolated From Local Landfill Soil in Medan IOP Publishing Ltd Vol. 126 012145 (IOP Publishing). doi: 10.1088/1755-1315/126/1/012145
Oberbeckmann S., Bartosik D., Huang S., Werner J., Hirschfeld C., Wibberg D., et al (2021). Genomic and Proteomic Profiles of Biofilms on Microplastics are Decoupled From Artificial Surface Properties. Environ. Microbiol. 23, 3099–3115. doi: 10.1111/1462-2920.15531
Oberbeckmann S., Kreikemeyer B., Labrenz M. (2017). Environmental Factors Support the Formation of Specific Bacterial Assemblages on Microplastics. Front. Microbiol. 8, 2709. doi: 10.3389/fmicb.2017.02709
Oberbeckmann S., Loeder M. G., Gerdts G., Osborn A. M. (2014). Spatial and Seasonal Variation in Diversity and Structure of Microbial Biofilms on Marine Plastics in Northern European Waters. FEMS Microbiol. Ecol. 90, 478–492. doi: 10.1111/1574-6941.12409
Oberbeckmann S., Osborn A. M., Duhaime M. B. (2016). Microbes on a Bottle: Substrate, Season and Geography Influence Community Composition of Microbes Colonizing Marine Plastic Debris. PloS One 11, e0159289. doi: 10.1371/journal.pone.0159289
Oksanen J., Blanchet F. G., Friendly M., et al (2020) Vegan: Community Ecology Package. Available at: https://CRAN.R-project.org/package=vegan.
Pinto M., Langer T. M., Huffer T., Hofmann T., Herndl G. J. (2019). The Composition of Bacterial Communities Associated With Plastic Biofilms Differs Between Different Polymers and Stages of Biofilm Succession. PloS One 14, e0217165. doi: 10.1371/journal.pone.0217165
Rampadarath S., Bandhoa K., Puchooa D., Jeewon R., Bal S. (2017). Early Bacterial Biofilm Colonizers in the Coastal Waters of Mauritius. Electron. J. Biotechnol. 29, 13–21. doi: 10.1016/j.ejbt.2017.06.006
R Core Team (2020). R: A Language and Environment for Statistical Computing (Vienna, Austria: R Foundation for Statistical Computing).
Romera-Castillo C., Pinto M., Langer T. M., Álvarez-Salgado X. A., Herndl G. J. (2018). Dissolved Organic Carbon Leaching From Plastics Stimulates Microbial Activity in the Ocean. Nat. Commun. 9, 1430. doi: 10.1038/s41467-018-03798-5
Roosen M., Mys N., Kusenberg M., Billen P., Dumoulin A., Dewulf J., et al (2020). Detailed Analysis of the Composition of Selected Plastic Packaging Waste Products and its Implications for Mechanical and Thermochemical Recycling. Environ. Sci. Technol. 54, 13282–13293. doi: 10.1021/acs.est.0c03371
Rummel C. D., Jahnke A., Gorokhova E., Kühnel D., Schmitt-Jansen M. (2017). Impacts of Biofilm Formation on the Fate and Potential Effects of Microplastic in the Aquatic Environment. Environ. Sci. Technol. Lett. 4, 258–267. doi: 10.1021/acs.estlett.7b00164
Schnurr R. E. J., Alboiu V., Chaudhary M., Corbett R. A., Quanz M. E., Sankar K., et al (2018). Reducing Marine Pollution From Single-Use Plastics (SUPs): A Review. Mar. Poll. Bull. 137, 157–171. doi: 10.1016/j.marpolbul.2018.10.001
Sekiguchi T., Saika A., Nomura K., Watanabe T., Watanabe T., Fujimoto Y., et al (2011). Biodegradation of Aliphatic Polyesters Soaked in Deep Seawaters and Isolation of Poly(Ɛ-Caprolactone)-Degrading Bacteria. Polym. Degrad. Stab. 96, 1397–1403. doi: 10.1016/j.polymdegradstab.2011.03.004
Sung C. C., Tachibana Y., Suzuki M., Hsieh W. C., Kasuya K. (2016). Identification of a Poly(3-Hydroxybutyrate)-Degrading Bacterium Isolated From Coastal Seawater in Japan as Shewanella Sp. Polym. Degrad. Stab. 129, 268–274. doi: 10.1016/j.polymdegradstab.2016.05.008
Suzuki M., Tachibana Y., Kazahaya J., Takizawa R., Muroi F., Kasuya K. (2017). Difference in Environmental Degradability Between Poly(Ethylene Succinate) and Poly(3-Hydroxybutyrate). J. Polym. Res. 24, 217. doi: 10.1007/s10965-017-1383-4
Teughels W., Van Assche N., Sliepen I., Quirynen M. (2006). Effect of Material Characteristics and/or Surface Topography on Biofilm Development. Clin. Oral. Implants Res. 17 Suppl 2, 68–81. doi: 10.1111/j.1600-0501.2006.01353.x
Thomas N. L., Clarke J., McLauchlin A. R., Patrick S. G. (2012). Oxo-Degradable Plastics: Degradation, Environmental Impact and Recycling. Proc. ICE - Waste Resource Manage. 165, 133–140. doi: 10.1680/warm.11.00014
Vu B., Chen M., Crawford R. J., Ivanova E. P. (2009). Bacterial Extracellular Polysaccharides Involved in Biofilm Formation. Molecules 14, 2535–2554. doi: 10.3390/molecules14072535
Ward C. S., Diana Z., Ke K. M., Orihuela B., Schultz T. P., Rittschof D. (2022). Microbiome Development of Seawater-Incubated Pre-Production Plastic Pellets Reveals Distinct and Predictive Community Compositions. Front. Mar. Sci. 8. doi: 10.3389/fmars.2021.807327
Webb J. S., Nixon M., Eastwood I. M., Greenhalgh M., Robson G. D., Handley P. S. (2000). Fungal Colonization and Biodeterioration of Plasticized Polyvinyl Chloride. Appl. Environ. Microbiol. 66, 3194–3200. doi: 10.1128/AEM.66.8.3194-3200.2000
Wiesinger H., Wang Z., Hellweg S. (2021). Deep Dive Into Plastic Monomers, Additives, and Processing Aids. Environ. Sci. Technol. 55, 9339–9351. doi: 10.1021/acs.est.1c00976
Wilcox C., Hardesty B. D., Sharples R., Griffin D. A., Lawson T. J., Gunn R. (2013). Ghostnet Impacts on Globally Threatened Turtles, a Spatial Risk Analysis for Northern Australia. Conserv. Lett. 6, 247–254. doi: 10.1111/conl.12001
Wright R. J., Bosch R., Gibson M. I., Christie-Oleza J. A. (2020a). Plasticizer Degradation by Marine Bacterial Isolates: A Proteogenomic and Metabolomic Characterization. Environ. Sci. Technol. 54, 2244–2256. doi: 10.1021/acs.est.9b05228
Wright R. J., Erni-Cassola G., Zadjelovic V., Latva M., Christie-Oleza J. A. (2020b). Marine Plastic Debris: A New Surface for Microbial Colonization. Environ. Sci. Technol. 54, 11657–11672. doi: 10.1021/acs.est.0c02305
Wright R. J., Gibson M. I., Christie-Oleza J. A. (2019). Understanding Microbial Community Dynamics to Improve Optimal Microbiome Selection. Microbiome 7, 1–14. doi: 10.1186/s40168-019-0702-x
Xu X., Wang S., Gao F., Li J., Zheng L., Sun C., et al (2019). Marine Microplastic-Associated Bacterial Community Succession in Response to Geography, Exposure Time, and Plastic Type in China's Coastal Seawaters. Mar. Poll. Bull. 145, 278–286. doi: 10.1016/j.marpolbul.2019.05.036
Yoshida S., Hiraga K., Takehana T., Taniguchi I., Yamaji H., Maeda Y., et al (2016). A Bacterium That Degrades and Assimilates Poly(Ethylene Terephthalate). Science 351, 1196–1199. doi: 10.1126/science.aad6359
Zeenat, Elahi A., Bukhari D. A., Shamim S., Rehman A. (2021). Plastics Degradation by Microbes: A Sustainable Approach. J. King Saud Univ. - Sci. 33, 101538. doi: 10.1016/j.jksus.2021.101538
Keywords: microbial community, 16S rRNA gene, ITS2 region, plastisphere, LLDPE (linear low density polyethylene), PA (nylon-6), biofilm, metabarcoding
Citation: Wallbank JA, Lear G, Kingsbury JM, Weaver L, Doake F, Smith DA, Audrézet F, Maday SDM, Gambarini V, Donaldson L, Theobald B, Barbier M and Pantos O (2022) Into the Plastisphere, Where Only the Generalists Thrive: Early Insights in Plastisphere Microbial Community Succession. Front. Mar. Sci. 9:841142. doi: 10.3389/fmars.2022.841142
Received: 22 December 2021; Accepted: 04 April 2022;
Published: 10 May 2022.
Edited by:
Michaela M. Salcher, Academy of Sciences of the Czech Republic (ASCR), CzechiaReviewed by:
Daniel Rittschof, Duke University, United StatesPandeng Wang, Sun Yat-sen University, China
Copyright © 2022 Wallbank, Lear, Kingsbury, Weaver, Doake, Smith, Audrézet, Maday, Gambarini, Donaldson, Theobald, Barbier and Pantos. This is an open-access article distributed under the terms of the Creative Commons Attribution License (CC BY). The use, distribution or reproduction in other forums is permitted, provided the original author(s) and the copyright owner(s) are credited and that the original publication in this journal is cited, in accordance with accepted academic practice. No use, distribution or reproduction is permitted which does not comply with these terms.
*Correspondence: Gavin Lear, Zy5sZWFyQGF1Y2tsYW5kLmFjLm56