- 1Southern Marine Science and Engineering Guangdong Laboratory (Zhuhai), School of Marine Sciences/Guangdong Key Laboratory of Marine Resources and Coastal Engineering, Sun Yat-sen University, Zhuhai, China
- 2National Marine Environmental Monitoring Center, Ministry of Ecology and Environment, Dalian, China
- 3Department of Ocean Sciences, Hong Kong University of Science and Technology, Hong Kong, Hong Kong SAR, China
Enrichment of nutrients is believed to lead to coastal hypoxia which have become a seasonal phenomenon over large river estuarine areas such as the Mississippi River-Northern Gulf of Mexico and Changjiang-East China Sea. A similar nutrient enrichment process exists in the Pearl River. However, hypoxia occurs only as episodic events over a relatively small area. We hypothesize that frequent wind events play the interruptive mechanism in preventing the seasonal formation of bottom hypoxia. We used 29 years’ time series data of dissolved oxygen (DO) and winds in the Hong Kong coastal waters to test the hypothesis. Our results show that bottom DO at 3 stations in southern waters of Hong Kong occasionally drops below the hypoxic level (2 mg/L), lasting only for less than one month in summer. Episodic hypoxia events appear to occur more frequently in recent years, but bottom DO does not show a significantly decreasing trend. The wind speed of 6 m/s appears to be a threshold, above which a wind event could destroy water column stratification and interrupt the formation of low-oxygen (DO <3 mg/L) water mass. The wind events above the threshold occur 14.3 times in June, 14.2 times in July and 10.0 times in August during 1990-2018. This explains why episodic events of hypoxia hardly occur in June and July, and only occasionally in August. The frequency of such the above-threshold events appears to show a decreasing trend during 1990-2018, which coincides with an increasing occurrences of episodic hypoxia events in recent years.
Introduction
Hypoxic environments are natural existence throughout geological time and distributed in many coastal ecosystems around the world (Diaz and Rosenberg, 2008). During the past half-century, however, the duration, intensity and extent of coastal hypoxia have been exacerbated by the increased nutrients input associated with human activities (Breitburg et al., 2018; Du et al., 2018). Typical examples of large dead zones in coastal zones around the world include Baltic Sea, northern Gulf of Mexico, northwestern shelf of the Black Sea and Changjiang-East China Sea (Bianchi et al., 2010; Capet et al., 2013; Väli et al., 2013; Zhu et al., 2016).
The Pearl River is the second largest river in China and the 13th largest in the world. The annual average river discharge is 10,524 m3s-1 with 20% occurring during the dry season and 80% during the wet season (Yin et al., 2004). The Pearl River estuary flows into the northern part of the South China Sea. Hong Kong is part of its eastern shores (Figure 1). This estuary has undergone intensive anthropogenic perturbations, such as domestic sewage, industry wastewater discharge, fertilizer usage and runoff. The total industrial and domestic wastewater discharge in Guangdong Province gradually increased from ~2.5 (1990) to ~9.4 (2016) billion tons (http://www.gdep.gov.cn), resulting in high N level and P limitation in the estuarine plume. (Yin et al., 2001, Yin et al., 2002). The region is subjected to the East Asian Monsoons with southwesterly wind dominates in summer and enables more eastward-directed plume propagation to affect the southern water of Hong Kong, resulting in two-layer circulation and intense stratification in the water column. The Pearl River estuarine coastal waters are very dynamic driven by factors such as river discharge, oceanic waters, coastal currents and monsoons (Yin et al., 2004).
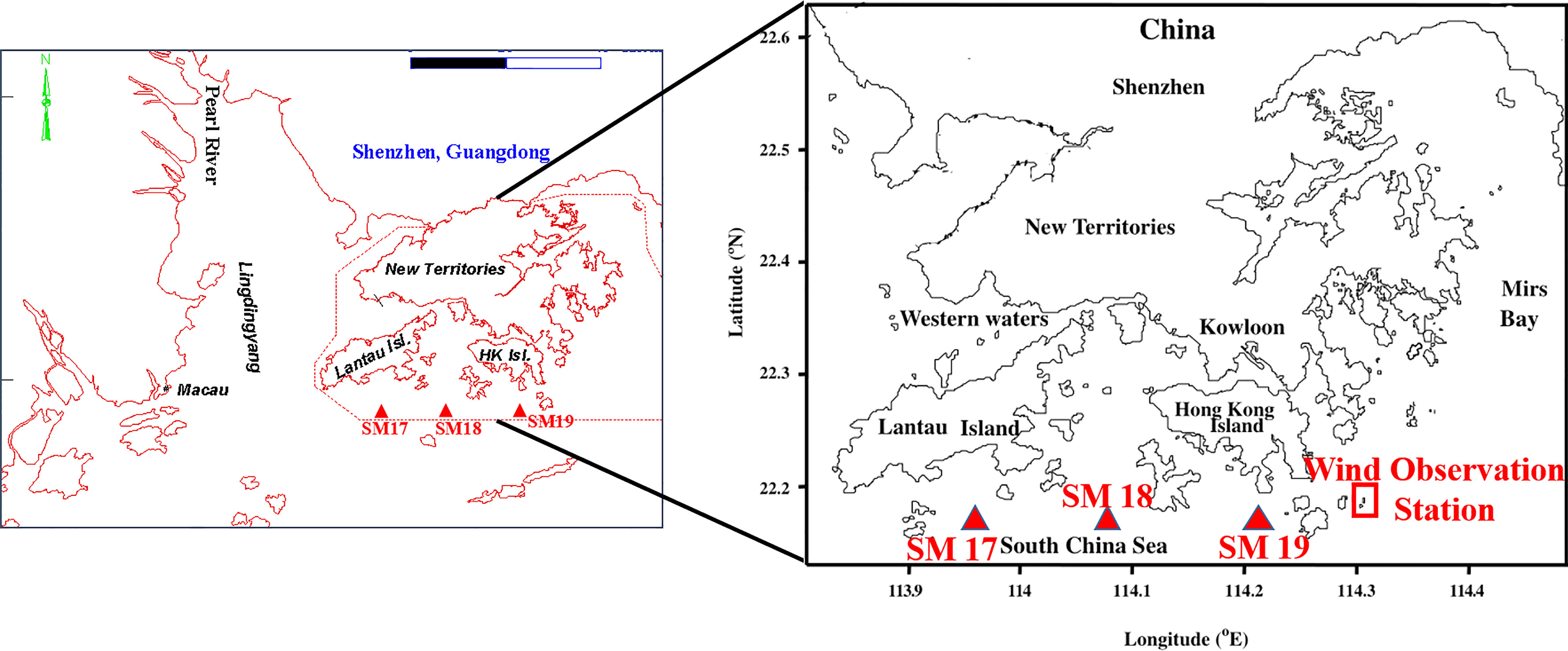
Figure 1 Map of the Pearl River estuary and Hong Kong waters showing the selected EPD water quality monitoring stations (SM17, SM18 and SM 19) and wind observation station in Waglan Island.
In recent years, the loading of anthropogenic nutrients has been increasing in the Pearl River (Hu and Li, 2009; Su et al., 2017), which is comparable to Mississippi and Yangtze where hypoxia has become a seasonal phenomenon (Rabalais et al., 1998; Li et al., 2002; Rabalais et al., 2002; Rabalais et al., 2010; Zhu et al., 2011; Wang et al., 2016). The increase in nutrients in the coastal waters is usually assumed to result in hypoxia in the estuary and coastal waters. However, over the coastal scale of the Pearl River estuarine influenced waters in the South China Sea, hypoxia has only occurred as episodic events over small areas (Yin et al., 2004; Xu et al., 2010; Li et al., 2019). Recent investigations reported a new occurrence of hypoxia in the coastal waters south of Macau (Ye et al., 2013; Su et al., 2017; Lu et al., 2018; Qian et al., 2018), but the spatial scale is only a small part of the Pearl River estuarine plume which influences the large part of the Northern South China Sea.
Water column stratification and decomposition/oxygen consumption of organic matter in the bottom water are two critical factors that lead to hypoxia, and both favorable conditions must occur simultaneously for hypoxia to develop and persist (Diaz, 2001). Since previous studies have shown lack of nutrient limitation to phytoplankton (Yin, 2003, EPD report 2017, http://www.epd.gov.hk/epd/english/environmentinhk/water/marine_quality/mwq_report.html), which indicate that lack of hypoxia is due to higher oxygen supplying rate by physical processes rather than due to shortage of organic matter. An event of episodic hypoxia in the Pearl River coastal waters was related to the wind mixing, short residence time and shallow (<15m) water depth (Zhou et al., 2012; Ye et al., 2013; Qian et al., 2018). In addition to the buoyancy flux induced by freshwater discharge, local wind forcing plays a regulating role in the stratification stability. Fisher et al. (2018) found that during periods of active wind-wave forcing, a three-layer turbulent response was detected by using numerical model and observational data, in which the wave transport layer transitioned to a surface log layer, which then merged with the tidal, bottom boundary layer in the Chesapeake Bay. Also, the estuarine stratification decreases with increasing magnitude of Wedderburn numbers for both down-estuary and up-estuary winds (Li and Li, 2011). Previous studies found that wind-driven vertical mixing accelerates the ventilation of water column and increases oxygen replenishment in the bottom layer in the Pearl River estuarine coastal waters (Zhou et al., 2012; Wang et al., 2015) and other regions (Wilson et al., 2008; Scully, 2010; Li and Li, 2011; Scully, 2013; Fisher et al., 2018). For example, bottom DO in hypoxic zone was observed to increase rapidly after the passage of a typhoon (Ni et al., 2016; Su et al., 2017). However, the mixing effect of typhoons is relatively short-lived in coastal ecosystems and the enhanced freshwater discharge, which can largely confine and dissipate the momentum input by winds within the plume water and prevent wind energy from penetrating to the subsurface water, will reduce the vertical mixing and re-establish stratification in only a few days (Zhou et al., 2012), to facilitate the re-formation of hypoxia (Su et al., 2017). Therefore, frequent strong wind events are the major force to interrupt the stratification and prevent the formation of hypoxia. Whether strong wind events relieve the tendency of hypoxia on a longer time scale depends partly on the frequency of wind events. Numerical modeling studies have illustrated the effects of wind stress/speed variations on coastal hypoxia (Chen et al., 2015; Wei et al., 2016; Lu et al., 2018). However, the role of frequency of wind events on the formation and maintenance of hypoxia is rarely studied, partly due to lack of long time series data. In order to explain the lack of seasonal phenomenon of hypoxia over the coastal scale in the Pearl River estuarine waters, we hypothesize that frequent wind events interrupt the formation of bottom hypoxia and prevent hypoxia from becoming a persistent seasonal phenomenon in the Pearl River estuarine coast. We used 29 years (1990-2018) time series data of dissolved oxygen and winds in Hong Kong to test the hypothesis. The approach is to examine the temporal trend of dissolved oxygen and frequency of wind speeds and to determine a threshold of wind speeds above which a wind event is strong enough to interrupt the formation of hypoxia.
Materials and Methods
The time series data of DO and other water quality variables at three stations SM17, SM18 and SM19 during 1990-2018 are obtained from the Environmental Protection Department (EPD) which has maintained a comprehensive marine water quality monitoring program since 1986 at 86 stations (Figure 1). The marine monitoring vessel “Dr. Catherine Lam” is equipped with a CTD profiler and a computer-controlled rosette water sampler to measure salinity, temperature and dissolved oxygen in situ and collect water samples for nutrients for later analysis in the laboratory. The surface DO is collected at 1m below the water surface and the bottom DO is collected at 1m above the seabed The water samples were analyzed in the EPD’s laboratory (EPD Report, 2017). This valuable dataset of salinity, temperature, chlorophyll a and DIN has been used in several publications over the last ten years (Yin et al., 2004; Xu et al., 2010; Lui and Chen, 2011; Lui and Chen, 2012; Qian et al., 2018).The three stations SM17, SM18 and SM19 are located in the southern waters of Hong Kong in coastal area of the Northern South China Sea. These stations are open to winds and least affected by tides with depths being 12 m, 21 m and 24 m, respectively (Figure 1). They are visited monthly for sampling. The monthly measurement would be enough to test the hypothesis because a hypoxic event, which can neither occur at the same location every year or last for 2 months, nor occur at 2 stations at the same time, will be considered as an episodic and local event, rather than a seasonal and coastal scale phenomenon. These stations are heavily influenced by the Pearl River estuarine plume during summer. Station SM18 is located in the southern end of Lamma Channel, the north end of which receives the sewage effluents from the outfalls of the Chemically Enhanced Primary Treatment (CEPT) of Stonecutter’s Island Works.
The daily averaged wind speeds and prevailing wind directions at Waglan Island (N 22°10′56″, E 114°18′12″, Figure 1) are obtained from the Hong Kong Observatory (HKO), and are assumed to represent the overall wind field over southern waters of Hong Kong including station SM17, SM18 and SM19. Southwesterly winds are dominant in June, July and August due to the Southwest Monsoon while easterly winds induced by typhoons occurred occasionally. Wind speeds above 6m/s occur less frequently in August which makes the water column in August most vulnerable to episodic events of hypoxia. However, the transition stage of monsoon and the frequent typhoons coming from the open sea generates dominated easterly winds in September (Figure 2). In general, wind direction, which is dominated by southwesterly monsoon winds during June, July and August, enable more eastward-directed plume propagation to the southern water of Hong Kong, which can enhance the water stratification and weaken the upwelling, favoring in the formation of hypoxia. Easterly winds due to the typhoons and transition stage of monsoon push the river plume westward away from Hong Kong waters and enhance the vertical mixing, preventing the formation of hypoxia (Kuang et al., 2011). However, wind speed is much more important in regulating the hypoxia formation as wind directions share the similar pattern from June to August in our study area. Also, a model study demonstrated that the hypoxic zone is much more sensitive to changes in the wind speed than the wind direction in the PRE (Wei et al., 2016). The summer period in our study includes June, July, August and September. Wind frequency is the number of days in each wind speed group (that is 1-2m/s, 2-3m/s and so on) for each month. The period of 29 years (from1990 to 2018) is divided into six groups with 5 years per group to illustrate the variations in wind speeds and the frequency of wind events in the long term.
Results
Time Series of DO at Surface and Bottom
The time series of DO during 1990-2018 at SM17, SM18 and SM19 showed that both the surface and bottom DO fluctuated, usually being high in winter and low in summer (Figure 3). In summer, the bottom DO was all low in June, July and August, and drops to the hypoxic levels occasionally in August. Hypoxic DO occurred more frequently at SM18 than SM17 and SM19, with 2, 4 and 2 times at SM17, SM18 and SM19, respectively, during 29 years. A hypoxic event has never lasted over 2 months in a year at one station and it does not usually occur at the 3 stations in the same month with the exception in August 2011 when the bottom DO was 0.4 mg/L and 0.9 mg/L at SM18 and SM19, respectively. These indicate that hypoxic events are episodic and have not developed a seasonal phenomenon over a coastal scale covering the 3 stations. Though there seems a declining trend in the annual minimum DO concentration, the bottom DO does not show any clear decreasing trend with seasonal variability included over the past decades as the linear regression over time (the red dashed line) is not significant (Figure 3). The P-value of SM17, SM18 and SM 19 were 0.6918, 0.7022 and 0.2598, respectively.
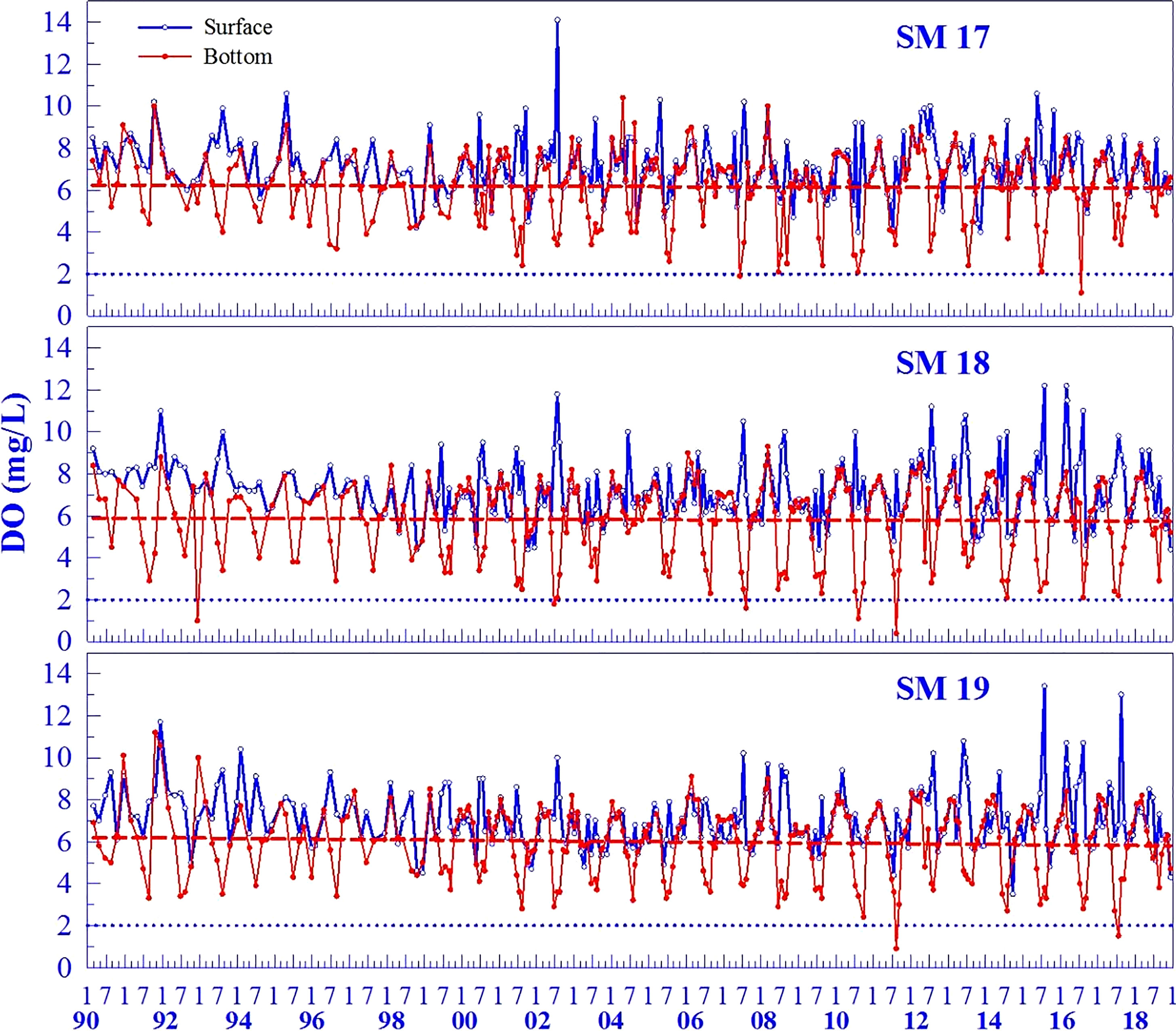
Figure 3 The time series of surface and bottom DO during 1990-2018 at SM17, SM18 and SM19 (the red dashed line indicates that the linear regression is not significant).
The Relationships Between Bottom DO, Stratification and Winds
In summer, the water column is usually stratified in estuarine and coastal areas due to river outflow and surface heating. In this study, we use the differences in sigma density between surface and bottom layers (△σ) to describe the strength of water column stratification. We use the differences in DO between surface and bottom layers (△DO) and Apparent Oxygen Utilization (AOU) to indicate DO consumption due to decomposition of organic matter in the bottom water mass. The correlation analysis (Table 1) shows that bottom DO is correlated to △σ at the 3 stations with correlation coefficient, r, being ~0.70 at p <0.01. The correlation coefficient r between △DO and △σ is all significant at 3 Stns, reaching 0.8 at SM19. Similarly, AOU is significantly correlated to △σ as AOU increases with △σ increasing. These relationships indicate that the strength of water column stratification plays a regulating role in the DO variability.
Since it takes some time for DO to be consumed to a low level from the surface DO, daily wind speeds of 7 days before the sampling versus bottom DO value are presented in summer during 1990-2018 at SM17, SM18 and SM19 (Figure 4) to inspect visually the wind effect on the low bottom DO. Generally, bottom DO is seen to decrease during a period of low wind speeds and is elevated obviously after each episodic high wind period. For example, the bottom DO increased, reaching 7.8 mg/L and 6.8 mg/L in June 1990 at SM17 and SM18, respectively, after the daily averaged wind speed blew for 7 days at 14.9, 15.2, 11.5, 8.2, 6.8, 3.8 and 9.6 m/s. When an event of hypoxia occurs, it is usually after a period of low winds. For example, bottom DO was 0.4 mg/L and 0.9 mg/L in August 2011 at SM18 and SM19, respectively, after the daily averaged wind speed blew for 7 days at 4.0, 3.8, 4.4, 3.4, 5.7, 4.5 and 3.6 m/s. This reflects the close connection between bottom DO and wind speed. It is worth noting that low wind speed event is a necessary condition for the occurrence of hypoxia, but not a sufficient condition. That is to say, when hypoxia occurs, there must be low wind speed event, while when low wind speed event occurs, hypoxia may not occur. The correlation analysis of bottom DO, AOU, △DO, △σ vs. wind speeds for 1-7 preceding days before a low DO event (Table 2) shows that correlation is better between these DO related parameters and 5-7 days averaged wind speed before sampling than 1-3 days averaged wind speed before sampling, which is related to the water depth and the water column stratification at stations. We choose V7 (7 days averaged wind speed before sampling) to represent the preceding wind speed. Bottom DO is positively correlated to wind speeds while AOU, △DO and △σ are negatively and significantly correlated to wind speeds. The differences in correlation coefficient across stations and variables are resulted from different hydrodynamics and organic matter conditions.
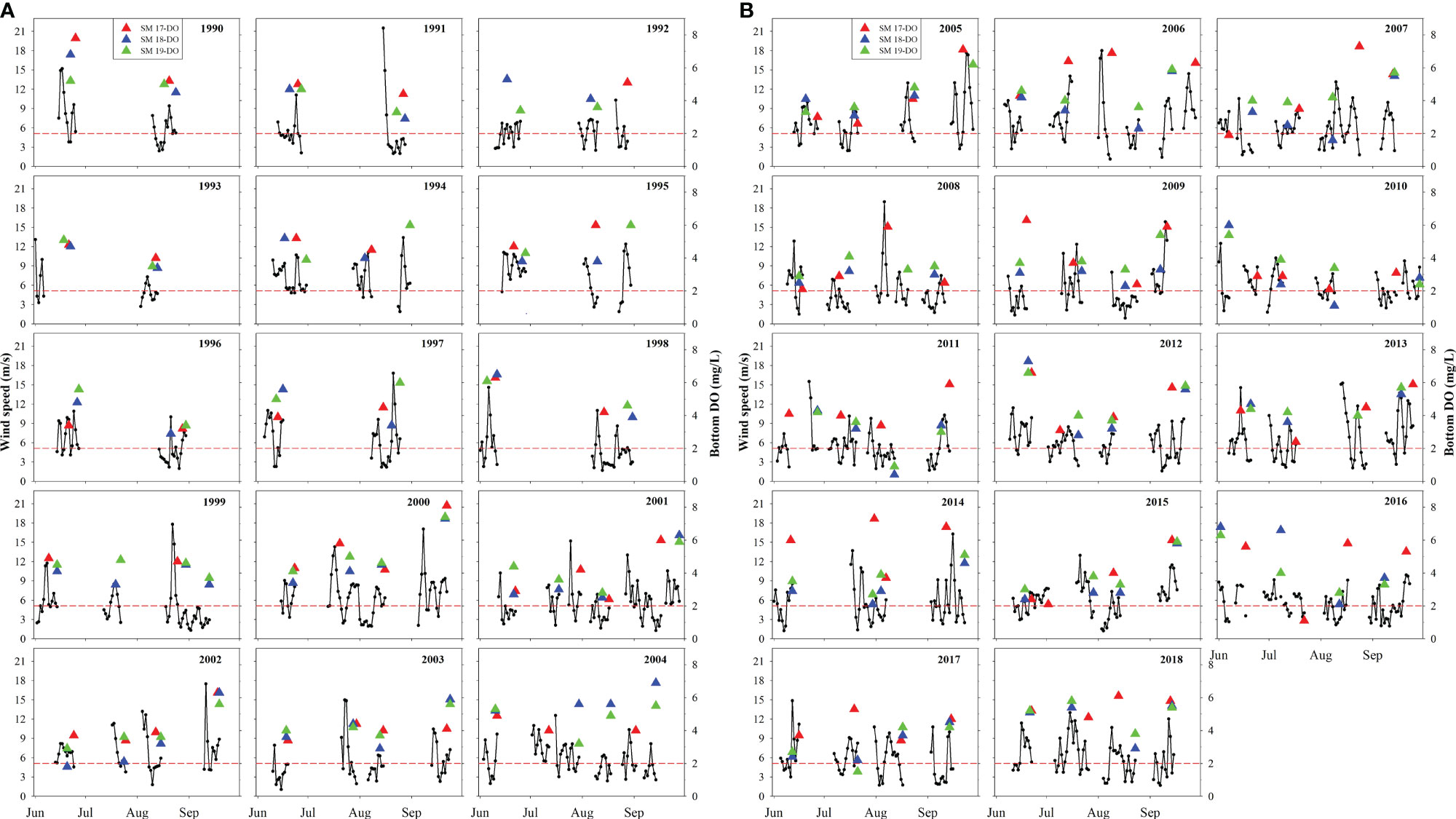
Figure 4 (A) Wind speed prior to the DO sampling and bottom DO during 1990-2004 in summer at SM17, SM18 and SM19 (the red dashed line denotes the level of DO=2 mg/L). (B) Wind speed prior to the DO sampling and bottom DO during 2005-2018 in summer at SM17, SM18 and SM19 (the red dashed line denotes the level of DO=2 mg/L).
The time series of V7 for DO in summer at SM17, SM18 and SM19 (Figure 5) does not show any decreasing trend during 1990-2018. The relationship between bottom DO, △σ and V7 during 1990-2018 at three stations (Figure 6) shows that when the wind speed is higher than 8 m/s, △σ is almost close to 0 and bottom DO is usually 5-6 mg/L. The frequency of wind speed V7 vs bottom DO during 1990-2018 in summer at SM17, SM18 and SM19 (Table 3A) shows that occurrences of bottom DO <3 mg/L is 0 when the averaged wind speed in preceding 7 days is >8 m/s.
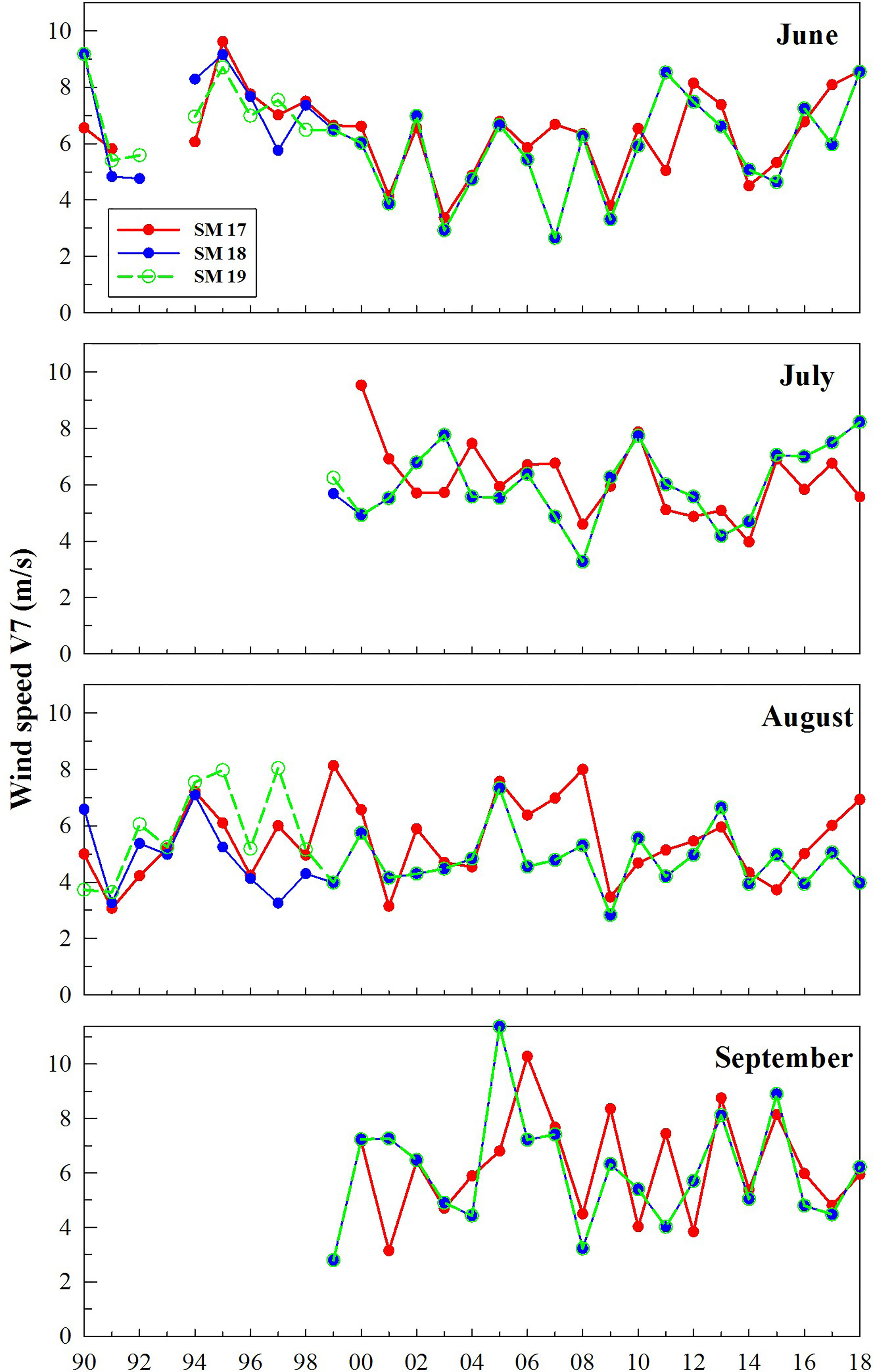
Figure 5 Time series of averaged wind speed 7 days before sampling (V7) during 1990-2018 in summer at SM17, SM18 and SM19. There is no significant trend by linear regression.
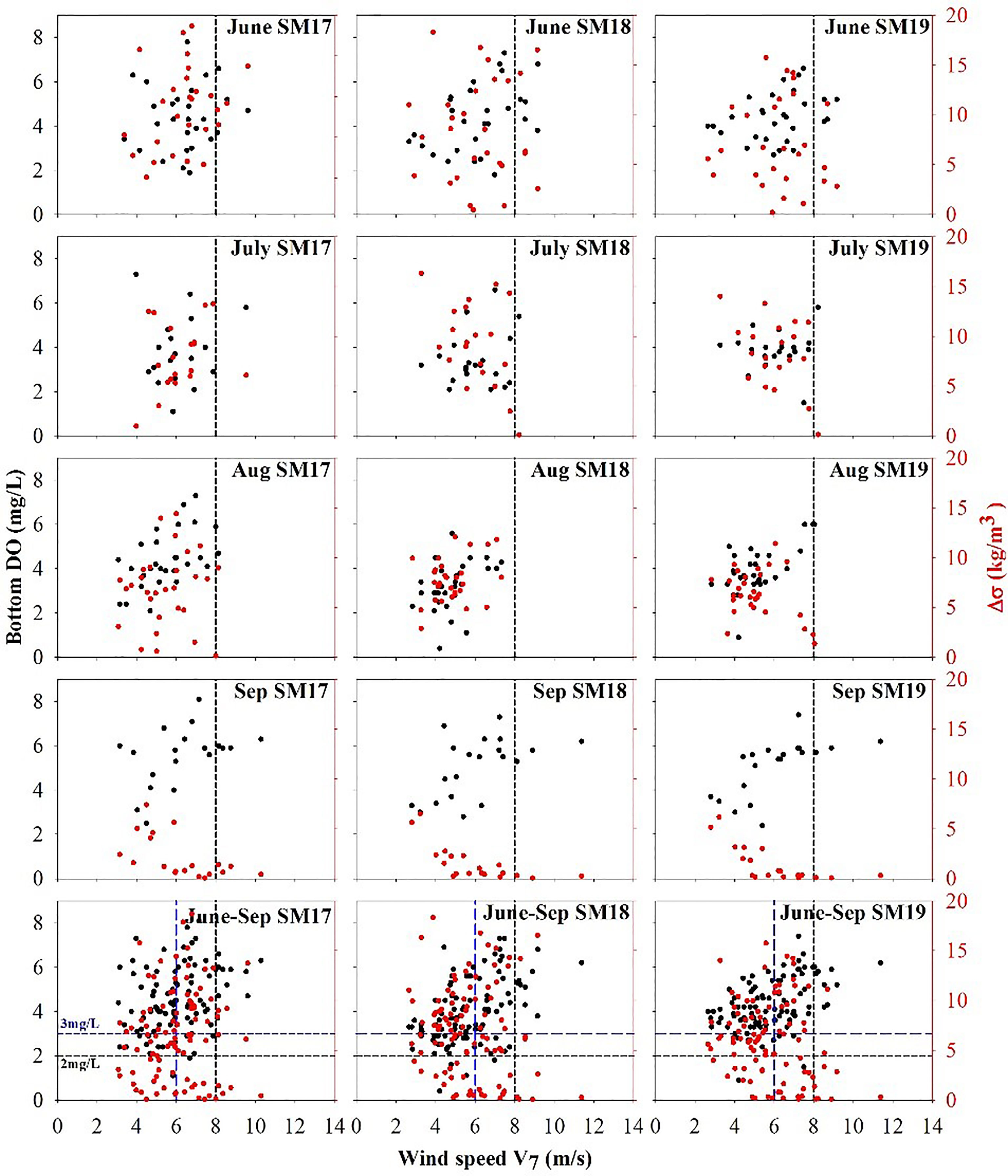
Figure 6 The relationship between bottom DO, △σ and wind speed V7 in summer during 1990-2018 at SM17, SM18 and SM19.
However, when wind speed is between 5 and 7 m/s, the bottom DO drops to the hypoxic level occasionally. Since hypoxic events (DO <2 mg/L) occurred rarely in the Pearl River estuarine coastal waters, we take 3 mg/L as the indicator or an event of low-oxygen water mass formation. The frequency of the low-oxygen events varies at different wind speeds, being 9.4%, 4.9%, 1.7% and 0 of bottom DO in summer of 29 years for >5, >6, >7 and >8 m/s in wind speed, respectively. We consider an event with a probability <5% as a small-probability event. When wind speed is >6 m/s, the water column can be largely mixed and the probability of bottom hypoxic events have a <5% chance to occur. Therefore, wind speeds >6 m/s are strong enough to interrupt the water column stratification. The accumulative frequency of the △σ-descending group vs the ascending wind speed V7 groups during 1990-2018 in summer (Table 3B) shows that occurrences of △σ >15 and the other 3 groups decrease when the wind speed increases. For example, occurrences of △σ >15 is 0% at SM19 and <5% at SM17 and SM18 when the averaged wind speed in preceding 7 days is >6 m/s.
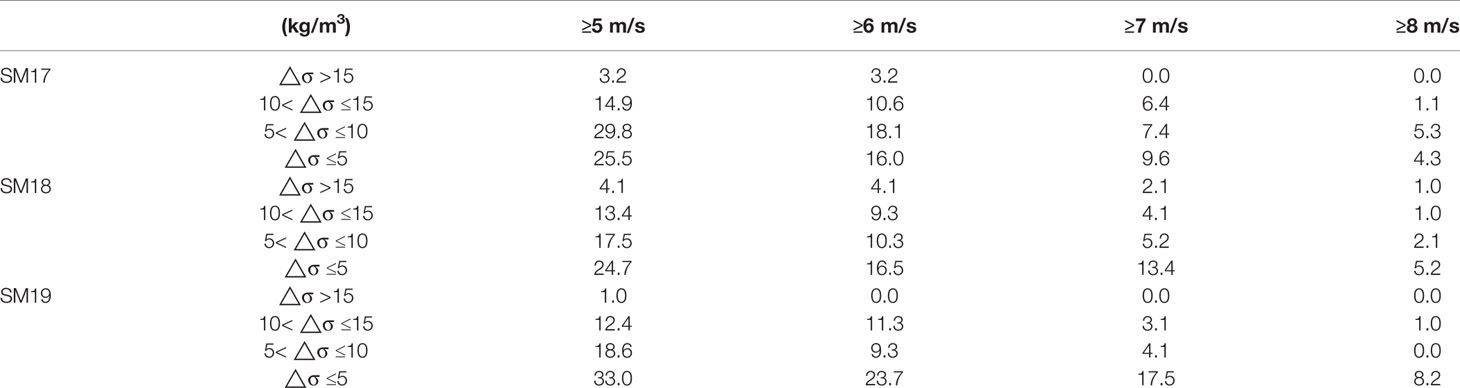
Table 3 (B) The Accumulative Frequency (%) of △σ at different V7 Wind Speeds during 1990-2018 in summer. Group ≥5 m/s includes the other 3 groups, group ≥6 m/s includes the other 2 groups and so on.
The Frequency of Wind Events
Winds >6 m/s are found to be a wind event that is strong enough to mix the water column (Figure 6). Wind events were very frequent over southern waters of Hong Kong (Figure 4). The frequency vs. wind speeds in June, July, August and September during 1990-2018 shows that wind speeds are usually between 2 m/s and 9 m/s and wind speeds <2 m/s or >9 m/s occur rarely (Figure 7). In June and July, wind speeds are mostly 4-7 m/s and are reduced to 2-5 m/s in August. In September, strong wind speeds occur more frequently than August. The accumulative frequency of wind speeds >6 m/s is 14.3, 14.2, 10.0 and 13.0 days per month in June, July, August and September, respectively. Compared to other months, the wind condition is much weaker in August. This means that August is most vulnerable to episodic events of hypoxia.
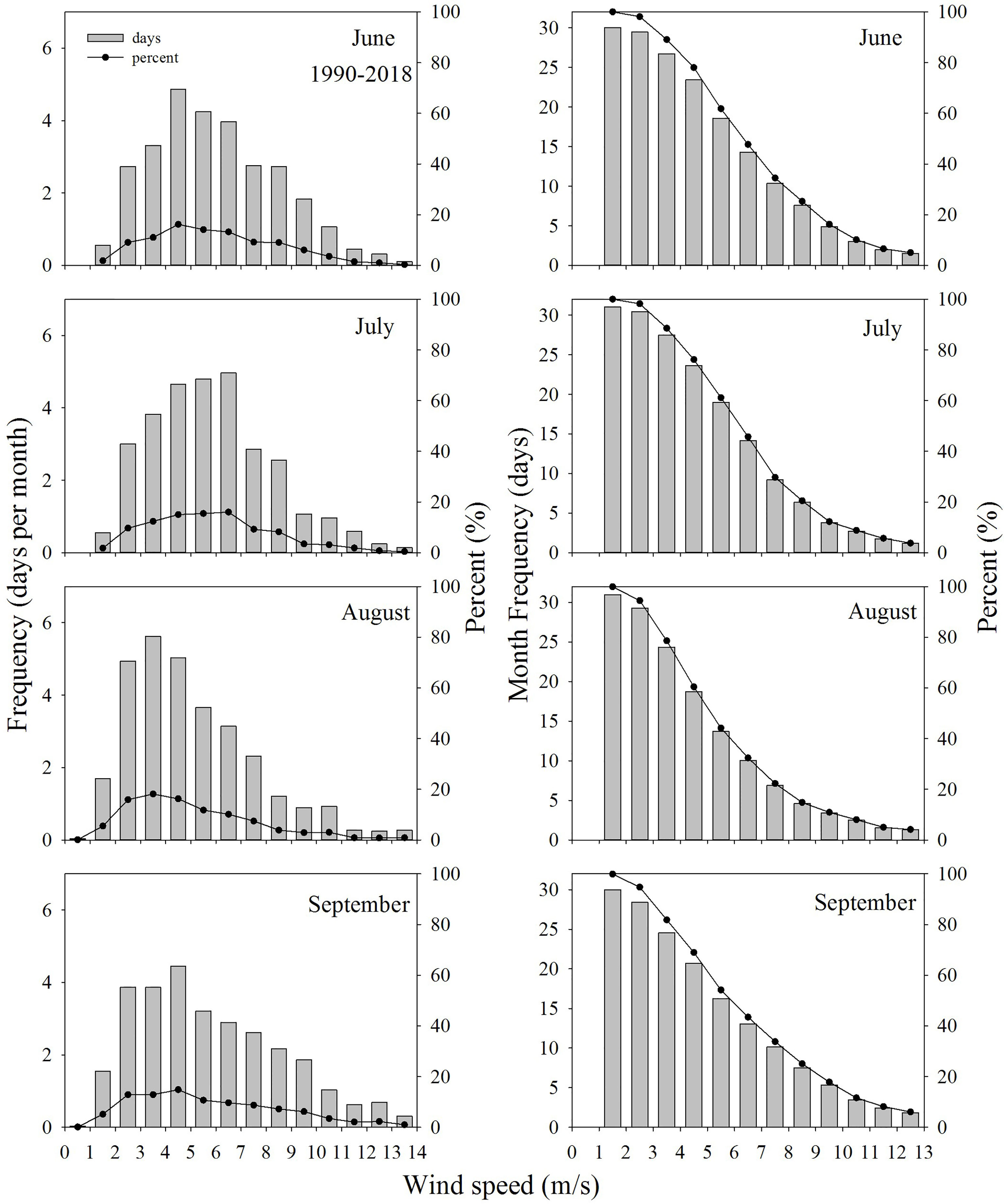
Figure 7 The frequency of grouped wind speeds during 1990-2018 in summer (left panel) and accumulative frequency of grouped wind speeds (accumulated from the largest wind speed group to the smallest one) (right panel).
The monthly averages of wind speeds for July and August decrease significantly during 29 years (Figure 8). Decrease can also be seen in June and September, but is not statically significant with the p-value of 0.0851 and 0.1412. It is more apparent that there appears to be a decrease in wind speeds >6 m/s and an increase in wind speeds ≤6 m/s in August based on 5 years grouping of wind speeds during 1990-2018 while no major changes in June, July and September (Figure 9). This suggests that the strong wind events occur less frequently in August than other months during the last 10 years (Figure 7). Among the five groups, the frequency of wind speeds >6 m/s appears to show a decreasing trend, especially in June, July and August. For example, in August, the frequency of wind speeds >6 m/s is 12.6, 12.4, 8.4, 10.6, 7 and 9 days per month during 1990-1994, 1995-1999, 2000-2004, 2005-2009, 2010-2014 and 2015-2018, respectively. In the long period of time, this decreasing trend means that low winds may be more frequent, which potentially results in an increase in the frequency of hypoxia in summer.
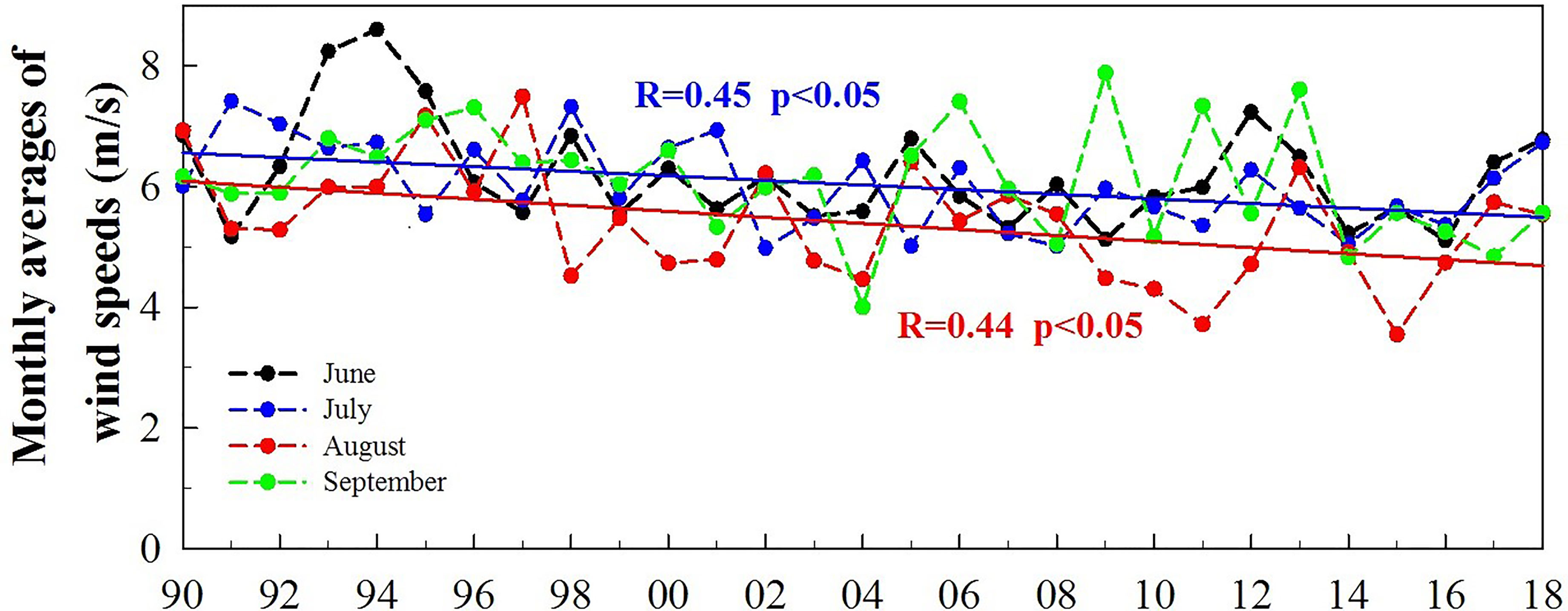
Figure 8 The monthly averages of wind speeds for June, July, August and September, respectively, over 29 years (the red and blue solid lines denote the significant regression).
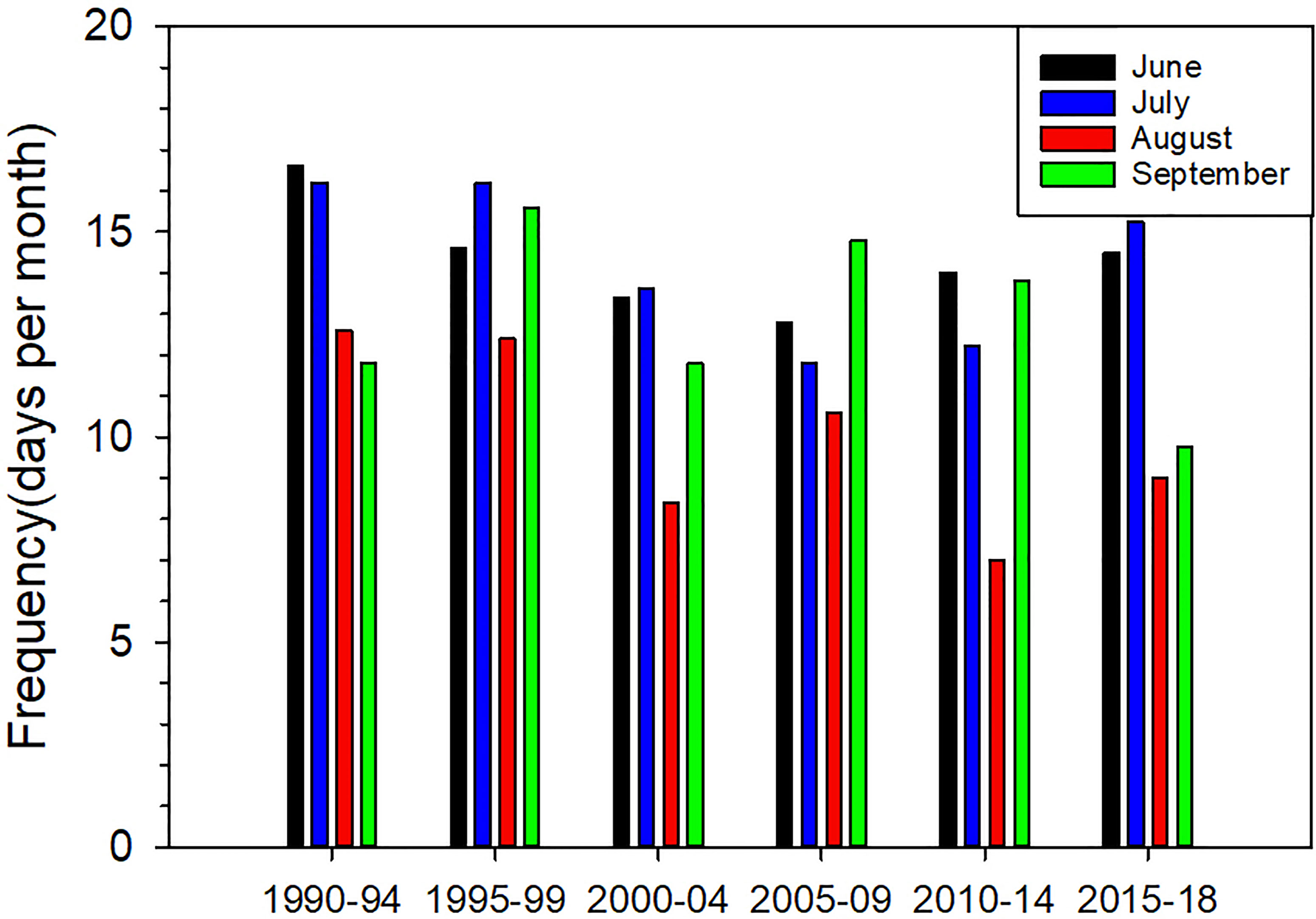
Figure 9 The monthly averaged frequency of wind speeds >6 m/s based on 5-year grouping in summer during 1990-2018 at Waglan Island.
Discussion
There is a lack of a significantly decreasing trend in DO in the southern water of Hong Kong during 1990-2018 with occasional drops below the hypoxic level a few times in summer. Nutrients in the southern waters are non-limiting (EPD Report, 2017). However, the drop has not lasted for two consecutive months at one station and has not happened at 2 stations in the same month. This demonstrates that the temporal scale of hypoxia occurring in southern waters of Hong Kong are episodic, not a seasonal phenomenon and the spatial scale is small, not even covering the two stations within 12 km. Yin et al. (2004) proposed the concept of ecosystem buffering capacity against hypoxia, which are determined by a number of drivers and processes (Yin and Harrison, 2007; Harrison et al., 2008; Yin and Harrison, 2008; Ho et al., 2008; Yin et al., 2010). Wind events of typhoons have been reported to mix the water column and subsequently increase bottom DO (Paerl et al., 1998; Paerl et al., 2001; Yin and Harrison, 2007; Zhou et al., 2012; Zhou et al., 2014; Ni et al., 2016). This study gives evidence to testify the hypothesis that frequent strong winds interrupt the stratification and slow down the formation of hypoxia.
The Formation of Low DO Water Mass
It is the residence time of the bottom layer and DO consumption rate that determine the formation of hypoxia in the bottom. The former depends on the physical processes of water advection, vertical mixing, and air-sea exchange and the latter photosynthesis, chemolithotrophic production, and respiration in the water column and sediment oxygen demand (Paerl, 2006; Chen et al., 2015). When the supply of oxygen is cut off to bottom waters, usually due to stratification of the water column, and consumption of DO through respiration exceeds resupply during a sufficiently long period of time, DO will decrease, reaching the level of hypoxia if organic matter is sufficient (Diaz, 2001). In many estuarine and coastal systems, excess nutrients lead to increased primary production, adding new organic matter to the coastal waters. Generally, a coastal water body receiving a large freshwater input with basic features of low physical energy (tidal, currents, or wind) is prone to hypoxia (Diaz, 2001).
The Interruptive Role of Wind Events on Hypoxia Formation
Many studies have demonstrated that physical processes such as estuarine circulation, tide and wind determine the residence time of bottom water and play a crucial role in the establishment, maintenance and termination of hypoxia (Simpson and Bowers, 1981; Simpson et al., 1990; Yin and Harrison, 2007; Rabouille et al., 2008; Wang et al., 2012). Whether an estuary is stratified or mixed depends on the transformation between kinetic energy and potential energy induced by these physical factors (Simpson and Bowers, 1981; Simpson et al., 1990). The freshwater from the river flows above the seawater, and hence exerts a buoyancy/stratifying influence in the estuary. A study found that although freshwater induced mixing may enhance the mixing in the upper layer of water column, but the whole water body is still stratified in the PRE (Kuang et al., 2011). The tides affect the water column in two ways: tidal straining and tidal stirring (Simpson et al., 2005). Winds can affect the turbulent mixing in several ways, including (1) direct mixing due to shear imposed at the surface by the wind stress, (2) generation of waves and wave breaking, and (3) modification of the plume velocity profile, and shear, through coastal set-up and/or straining of isopycnals (Li et al., 2007; Wilson et al., 2008; Wang et al., 2015; Pan and Gu, 2016). As tidal ranges are <2 m in Hong Kong and the tide-induced mixing is mainly confined in the bottom layer and can hardly reach the surface plume layer (Chen et al., 2016), which means tide cannot break down the stratification or ventilate the bottom water to increases oxygen replenishment. Therefore, winds may play the most important role in interrupting the hypoxia formation. In general, the intensity of hypoxia was inversely related to wind stress. Chen et al. (2015) pointed that wind speed and direction are the most important among the physical factors influencing oxygen dynamics in the Yangtze Estuary. A 10% increase in wind speed reduced the areal extent of hypoxia by 46.66%, and a 10% reduction increased the hypoxic area by 67.28% (Chen et al., 2015). In the Pearl River Estuary, the DO concentration and volume of the hypoxic zone changed dramatically at 50% of the standard wind stress forcing of 0.025 Pa in summer and quickly reached a non-hypoxic state when wind forcing approached 200% (Lu et al., 2018). In the Mississippi River-Northern Gulf of Mexico, the size of the ‘dead zone’ was found to be strongly correlated with high river discharges and strong stratification (Justić et al., 1996). The Baltic Sea with persistent stratification is prone to the occurrences of hypoxia (Conley et al., 2002; Diaz and Rosenberg, 2008; Lehmann et al., 2014). Weak tidal forcing and deep topography which lead to the formation of a stable pycnocline also result in seasonal hypoxia in the Chesapeake Bay. The formation of seasonal hypoxia in these large coastal systems are much related to the persistent intense stratification and the lack of wind events is a favorable condition for the formation of hypoxia when organic matter supply is sufficient. Our results show that the occurrences of hypoxia are usually after a long period of low winds (Figure 4). The wind speed of 6 m/s can be considered as the threshold of a wind event, above which the stratified water column can be mixed to interrupt the formation of the bottom hypoxia in coastal waters south of Hong Kong. A numerical model simulation study demonstrated that the water column is almost homogeneously mixed and the development of hypoxia in the bottom water is interrupted when the wind speed increased to 8m/s (Wei et al., 2016).The examination of the monthly frequency of such wind events (>6 m/s) reveals that wind events >6 m/s occur every two or three days on average during June, July and September, which appears to be frequent enough to raise the bottom DO in southern waters of Hong Kong. The wind speed is the lowest in August, which explains why most hypoxic events at SM17, SM18 and SM19 occurred in August (Figure 3). The formation process of hypoxia is interrupted, reset and starts over again after such a wind event. The consumption of bottom DO to the hypoxic level will take some time as the development of phytoplankton blooms and the bacteria degradation of dissolved organic matter require a period of time, saying 7 days at least to consume DO to a hypoxia condition, based on Table 2. Previous study found that in the bottled estuarine surface waters, phytoplankton took 3-4 days to reach the maximum during the incubation. Similarly, it took 3-4 days to consume DO from 5-7 mg/L to 2 mg/L during the dark closed incubation (Yin et al., 2000; Yin and Harrison, 2008). However, in the bottled bottom water, DO consumption to 2 mg/L took longer time with ~ 5 days (J. Yao unpubl). Considering that the real condition in the field is more dynamic than bottled water, the bottom DO consumption will take longer time (>5 days) in situ situation. Observation found that Hypoxia was completely destroyed by the typhoon passage but was quickly restored 8~12 days later in the PRE (Zhao et al., 2021). Each time when the bottom hypoxia is going to be developed, strong winds slow down its formation. A stronger episodic wind event will interrupt its formation completely. The low-oxygen reoccurrence is determined by the recovery of vertical stratification after strong wind events (Su et al., 2017) as blooms in a mixed water column did not develop hypoxia in the spring when stratification was weak at station SM18 (Qian et al., 2018). The resuming processes may not be a simple recovery. As estuarine coastal water are profoundly influenced by the complex interaction processes among three water masses: brackish plume water, offshore surface water and upwelled subsurface water, which influences phytoplankton growth and its organic matter sinking to the bottom water. In addition, the consumption of DO may also be variable. Apparently, each wind event supplies oxygen to bottom layer, which resets the bottom to a higher initial DO value for consumption and hence, leads to longer formation time for the next hypoxia event to occur. This explains why seasonal and coastal scale hypoxic events have rarely occurred in Hong Kong waters despite of the large nutrient inputs.
Due to the southwest monsoon, the Pearl River estuarine freshwater flows across the southern waters of Hong Kong. SM17 is most affected by the river outflow meanwhile the shallow depth of 12 m makes wind mixing more effective at SM17. SM18 is located in the southern end of Lamma Channel. In the northern end of it, the sewage effluent outfalls of the biggest sewage treatment plant (Stonecutter’s CEPT Plant) in Hong Kong are laid in the bottom. Part of the treated effluent flows through the Lamma Channel to the southern waters. Thus, SM18 is most influenced by the sewage effluent with proper depth of 21m, and hypoxia occurred most frequently at SM18. SM19 appears to be least influenced by the estuarine plume and sewage effluent, and by a wind event due to its deepest depth (24 m). This explains low occurrences of hypoxia at SM19 at wind speeds >5 m/s (Table 3A).
Ecosystem Buffering Capacity
Cloern (2001) pointed out that some coastal ecosystems can accommodate an excess nutrient enrichment without showing apparent eutrophication symptoms. Yin et al. (2010) proposed that it is the ecosystem buffering that makes the Pearl River estuary “robust” to N enrichment. It is determined by physical driving forces such as monsoons, river outflow, tidal cycles and rainfall, and some of them become dominant over different temporal and spatial scales, which induce circulation, stratification and turbulent mixing. As a result, the fields of light, salinity, temperature and nutrients vary, thus influencing algal growth and DO consumption. When anthropogenic nutrients enter coastal waters, there would be a series of physical and biological processes before nutrient enrichment causes any ecological impacts. If the ecosystem buffering capacity is large enough, the input may not lead to major symptoms of eutrophication. One is the long-term increase in chlorophyll-a (chl-a) in coastal regions due to accelerated phytoplankton growth in response to elevated concentrations of nutrients. The other is hypoxia due to the increased production or input of organic matter (i.e., phytoplankton, benthic microalgae and macro-algae, and sea grass biomass) in coastal waters. Inversely, algae bloom and hypoxia may occur.
Lacking of a seasonal hypoxia over the coastal scale in the Pearl River estuarine influenced waters suggests that the ecosystem buffering capacity plays a regulating role in controlling the production and accumulation of algal blooms and DO consumption and potential occurrence of hypoxia (Lee et al., 2006; Harrison et al., 2008). In addition to these physical controls on hypoxia, the lower PO4 concentrations relative to nitrogen (N:P~100:1), may limit the phytoplankton biomass production through P limitation, and hence the amount of organic matter sinking to depth (Yin et al., 2004). It will have more impact as the total phosphorus input in Guangdong Province gradually decreased from ~21400 (2011) to ~7400 (2018) tons. Zooplankton grazing pressure could also be an influencing factor in limiting the phytoplankton biomass via the top down control in HK waters in summer (Ho et al., 2008). Strong solar radiation can reach the shallow bottom layer of HK waters (although it might still be limiting) and support some growth of phytoplankton at depth that can release and partially replenish DO (Ho et al., 2008). In summary, hypoxia might therefore develop only when bottom DO consumption exceeds the buffering capacity maintained by all these physical and biochemical factors above.
The frequency of wind events (>6 m/s) appears to show a decreasing trend in summer in the long term, which may be well related to global climate change. Climate change is likely contributing to the increase in dead zones, by influencing factors such as winds, precipitation and temperature (Altieri and Gedan, 2015). For example, changes in the direction and strength of seasonal wind patterns can modify hypoxic conditions by affecting circulation patterns that determine nutrient delivery and water column stratification (Conley et al., 2007; Altieri and Gedan, 2015). Changes in rainfall patterns can increase discharges of freshwater and nutrients to coastal ecosystems (Diaz and Rosenberg, 2008). Recently, global warming is predicted to enhance stratification, decrease oxygen solubility and accelerate respiration, thus exacerbating the oxygen depletion in nutrient-enriched coastal systems (Breitburg et al., 2018). In the Chesapeake Bay, sea level rise and larger winter-spring runoff led to stronger stratification and large reductions in the vertical oxygen supply to the bottom water. The hypoxic and anoxic volumes would increase by 10-30% between the late 20th and mid-21st century (Ni et al., 2019). In the Gulf of Mexico, a 20% increase of the Mississippi River discharge, which may occur under some climate change scenarios, would produce an increase in the frequency of hypoxia by 37% (Justić et al., 2003). In the Baltic Sea, the impacts of warming and eustatic sea level rise were comparatively smaller than changing river-borne nutrient loads and atmospheric deposition, but still important (Meier et al., 2019). When it comes to the PRE, The weakening of the East Asia Summer Monsoon, induced by a reduced thermal contrast due to a greater increase of sea surface air temperature than the land, has been observed over the Northern South China Sea (Chang et al., 2000; Lei et al., 2011). If the weak wind condition or the tendency of decreasing wind speeds continues in the future, the occurrence of hypoxia in this system may become more frequent, and likely develops into large areas of seasonal hypoxia. This may contribute to a relatively large hypoxic zone in the south water of Macau reported recently (Su et al., 2017; Lu et al., 2018; Qian et al., 2018). This raises an alarming signal and an urgent need to fully understand the influence of climate change and how multiple factors interact to drive the dead zone dynamics.
Conclusions
Due to population growth and economic development in last 60 years, riverine nutrients have increased dramatically, which leads to increased organic matter production in estuarine and coastal waters. However, not all estuaries or coastal waters show eutrophication symptoms such as red tides or hypoxia (Cloern, 2001). Nutrients in the Pearl River have been steadily increasing in the last 4 decades, but hypoxic water mass has not developed into a seasonal phenomenon in a large scale over the plume influenced waters in the Northern South China Sea. Our study testified the hypothesis that frequent strong wind events destroy the water column stratification and interrupt the formation of hypoxia. The wind speed >6 m/s can be considered to be the threshold of an interruptive wind event in Hong Kong waters. Our finding demonstrates the role winds play in the ecosystem buffering capacity against enrichment of nutrients. The finding is significant because climate change may have resulted in the decreasing trend in the frequency of wind speeds >6 m/s in the recent years, which is an alarming signal for more occurrences of hypoxic events in the region. The water quality management needs to keep long-term monitoring and develop strategies for controlling and regulating the input of nutrients in coastal waters to the level that is below the threshold for triggering the hypoxia in the downstream of the estuary.
Data Availability Statement
The original contributions presented in the study are included in the article/supplementary material. Further inquiries can be directed to the corresponding author.
Author Contributions
The contributions made by each of the authors are as follows. KW and JY analyze the long time series data and write the original manuscript. JW and HL review the manuscript and give valuable and helpful comments. KY provides guidance on the conceptualization of the scientific story and makes revision of the manuscript. All authors contributed to the article and approved the submitted version.
Funding
This study is part of GDKJT 2020B1111350001, NSFC-GD Joint Scheme Project (U1701247), SML99147-42080013, GDNRC [2021]62, NSFC grant (91328203) and NMEMC Key Laboratory for Ecological Environment in Coastal Area (Grant 201819). HL acknowledge the support of Hong Kong Research Grants Council (T21/602/16 and N_HKUST609/15).
Conflict of Interest
The authors declare that the research was conducted in the absence of any commercial or financial relationships that could be construed as a potential conflict of interest.
Publisher’s Note
All claims expressed in this article are solely those of the authors and do not necessarily represent those of their affiliated organizations, or those of the publisher, the editors and the reviewers. Any product that may be evaluated in this article, or claim that may be made by its manufacturer, is not guaranteed or endorsed by the publisher.
Acknowledgments
We acknowledge the Hong Kong EPD and HKO for permitting us to use their water quality monitoring data and weather data for this study.
References
Altieri A. H., Gedan K. B. (2015). Climate Change and Dead Zones. GCB Bioenergy 21 (4), 1395–1406. doi: 10.1111/gcb.12754
Bianchi T. S., Dimarco S. F., Cowan J. H., Hetland R. D., Chapman P., Day J. W., et al. (2010). The Science of Hypoxia in the Northern Gulf of Mexico: A Review. Sci. Total Environ. 408 (7), 1471–1484. doi: 10.1016/j.scitotenv.2009.11.047
Breitburg D., Levin L. A., Oschlies A., Gregoire M., Chavez F. P., Conley D. J., et al. (2018). Declining Oxygen in the Global Ocean and Coastal Waters. Science 359 (6371), 46. doi: 10.1126/science.aam7240
Capet A., Beckers J. M., Grégoire M. (2013). Drivers, Mechanisms and Long-Term Variability of Seasonal Hypoxia on the Black Sea Northwestern Shelf & Ndash; is There Any Recovery After Eutrophication? Biogeosciences 10 (6), 3943–3962. doi: 10.5194/bg-10-3943-2013
Chang C., Zhang Y., Li T. (2000). Interannual and Interdecadal Variations of the East Asian Summer Monsoon and Tropical Pacific SSTs. Part I: Roles of the Subtropical Ridge. J. Climate. 13 (24), 4310–4325. doi: 10.1175/1520-0442(2000)013<4310:IAIVOT>2.0.CO;2
Chen Z., Pan J., Jiang Y. (2016). Role of Pulsed Winds on Detachment of Low Salinity Water From the Pearl River Plume: Upwelling and Mixing Processes. J. Geophs. Res-Oceans 121 (4), 2769–2788. doi: 10.1002/2015jc011337
Chen X., Shen Z., Li Y., Yang Y. (2015). Physical Controls of Hypoxia in Waters Adjacent to the Yangtze Estuary: A Numerical Modeling Study. Mar. Pollut. Bull. 97 (1-2), 349–364. doi: 10.1016/j.marpolbul.2015.05.067
Cloern J. E. (2001). Our Evolving Conceptual Model of the Coastal Eutrophication Problem. Mar. Ecol. Prog. Ser. 210, 223–253. doi: 10.3354/meps210223
Conley D. J., Carstensen J., Aertebjerg G., Christensen P. B., Dalsgaard T., Hansen J. L. S., et al. (2007). Long-Term Changes and Impacts of Hypoxia in Danish Coastal Waters. Ecol. Appl. 17 (sp5), S165–S184. doi: 10.1890/05-0766.1
Conley D. J., Humborg C., Rahm L., Savchuk O. P., Wulff F. (2002). Hypoxia in the Baltic Sea and Basin-Scale Changes in Phosphorus Biogeochemistry. Environ. Sci. Technol. 36 (24), 5315–5320. doi: 10.1021/es025763w
Diaz R. J. (2001). Overview of Hypoxia Around the World. J. Environ. Qual. 30 (2), 275–281. doi: 10.2134/jeq2001.302275x
Diaz R. J., Rosenberg R. (2008). Spreading Dead Zones and Consequences for Marine Ecosystems. Science 321 (5891), 926–929. doi: 10.1126/science.1156401
Du J., Shen J., Park K., Wang Y. P., Yu X. (2018). Worsened Physical Condition Due to Climate Change Contributes to the Increasing Hypoxia in Chesapeake Bay. Sci. Total Environ. 630, 707–717. doi: 10.1016/j.scitotenv.2018.02.265
Fisher A. W., Sanford L. P., Scully M. E. (2018). Wind-Wave Effects on Estuarine Turbulence: A Comparison of Observations and Second-Moment Closure Predictions. J. Phys. Oceanography 48, 905–923. doi: 10.1175/JPO-D-17-0133.1
Harrison P. J., Yin K., Lee J. H. W., Gan J., Liu H. (2008). Physical-Biological Coupling in the Pearl River Estuary. Cont. Shelf Res. 28 (12), 1405–1415. doi: 10.1016/j.csr.2007.02.011
Ho A. Y. T., Xu J., Yin K., Yuan X., He L., Jiang Y., et al. (2008). Seasonal and Spatial Dynamics of Nutrients and Phytoplankton Biomass in Victoria Harbour and its Vicinity Before and After Sewage Abatement. Mar. Pollut. Bull. 57 (6-12), 313–324. doi: 10.1016/j.marpolbul.2008.04.035
Hu J., Li S. (2009). Modeling the Mass Fluxes and Transformations of Nutrients in the Pearl River Delta, China. J. Marine Syst. 78 (1), 146–167. doi: 10.1016/j.jmarsys.2009.05.001
Justić D., Rabalais N. N., Turner R. E. (1996). Effects of Climate Change on Hypoxia in Coastal Waters: A Doubled CO2 Scenario for the Northern Gulf of Mexico. Limnol. Oceanogr. 41 (5), 992–1003. doi: 10.4319/lo.1996.41.5.0992
Justić D., Rabalais N. N., Turner R. E. (2003). Simulated Responses of the Gulf of Mexico Hypoxia to Variations in Climate and Anthropogenic Nutrient Loading. J. Marine Syst. 42, 115–126. doi: 10.1016/S0924-7963(03)00070-8
Kuang C., Lee J. H. W., Harrison P. J., Yin K. (2011). Effect of Wind Speed and Direction on Summer Tidal Circulation and Vertical Mixing in Hong Kong Waters. J. Coastal Res. 27 (6A, Suppl. S), 74–86. doi: 10.2112/JCOASTRES-D-11-00050.1
Lee J. H. W., Harrison P. J., Kuang C., Yin K. (2006). “Eutrophication Dynamics in Hong Kong Coastal Waters: Physical and Biological Interactions,” in The Environment in Asian Pacific Harbors (Netherlands: Springer), 187–206. doi: 10.1007/1-4020-3655-8_13
Lehmann A., Hinrichsen H. H., Getzlaff K., Myrberg K. (2014). Quantifying the Heterogeneity of Hypoxic and Anoxic Areas in the Baltic Sea by a Simplified Coupled Hydrodynamic-Oxygen Consumption Model Approach. J. Marine Syst. 134 (6), 20–28. doi: 10.1016/j.jmarsys.2014.02.012
Lei Y., Hoskins B., Slingo J. (2011). Exploring the Interplay Between Natural Decadal Variability and Anthropogenic Climate Change in Summer Rainfall Over China. Part I: Observational Evidence. J. Climate. 24 (17), 4584–4599. doi: 10.1175/2010JCLI3794.1
Li Y., Li M. (2011). Effects of Winds on Stratification and Circulation in a Partially Mixed Estuary. J. Geophys. Res.: Oceans 116 (C12012), 1–16. doi: 10.1029/2010JC006893
Li X., Lu C., Zhang Y., Zhao H., Wang J., Liu H., et al. (2019). Low Dissolved Oxygen in the Pearl River Estuary in Summer: Long-Term Spatio-Temporal Patterns, Trends, and Regulating Factors. Mar. Pollut. Bull 151, 110814.1-110814.13. doi: 10.1016/j.marpolbul.2019.110814
Li D., Zhang J., Huang D., Wu Y., Liang J. (2002). Oxygen Depletion Off the Changjiang (Yangtze River) Estuary. Sci. China 45 (12), 1137–1146. doi: 10.3969/j.issn.1674-7313.2002.12.008
Li M., Zhong L., Boicourt W. C., Zhang S., Zhang D. L. (2007). Hurricane-Induced Destratification and Restratification in a Partially-Mixed Estuary. J. Mar. Res. 65, 169–192(24). doi: 10.1357/002224007780882550
Lu Z., Gan J., Dai M., Liu H., Zhao X. (2018). Joint Effects of Extrinsic Biophysical Fluxes and Intrinsic Hydrodynamics on the Formation of Hypoxia West Off the Pearl River Estuary. J. Geophys. Res.: Oceans 123 (9), 6241–6259. doi: 10.1029/2018JC014199
Lui H., Chen C. (2011). Shifts in Limiting Nutrients in an Estuary Caused by Mixing and Biological Activity. Limnol. Oceanogr. 56 (3), 989–998. doi: 10.4319/lo.2011.56.3.0989
Lui H., Chen C. (2012). The Nonlinear Relationship Between Nutrient Ratios and Salinity in Estuarine Ecosystems: Implications for Management. Curr. Opin. Environ. Sustain. 4 (2), 227–232. doi: 10.1016/j.cosust.2012.03.002
Meier H., Eilola K., Almroth-rosell E., Schimanke S., Keniebusch M., Hoglund A., et al. (2019). Disentangling the Impact of Nutrient Load and Climate Changes on Baltic Sea Hypoxia and Eutrophication Since 1850. Climate Dynamics 53, 1145–1166. doi: 10.1007/s00382-018-4296-y
Ni X., Huang D., Zeng D., Zhang T., Li H., Chen J. (2016). The Impact of Wind Mixing on the Variation of Bottom Dissolved Oxygen Off the Changjiang Estuary During Summer. J. Marine Syst. 154, 122–130. doi: 10.1016/j.jmarsys.2014.11.010
Ni W., Li M., Ross A. C., Najjar R. G. (2019). Large Projected Decline in Dissolved Oxygen in a Eutrophic Estuary Due to Climate Change. J. Geophys. Res.: Oceans 124, 8271–8289. doi: 10.1029/2019JC015274
Paerl H. W. (2006). Assessing and Managing Nutrient-Enhanced Eutrophication in Estuarine and Coastal Waters: Interactive Effects of Human and Climatic Perturbations. Ecol. Eng. 26 (1), 40–54. doi: 10.1016/j.ecoleng.2005.09.006
Paerl H. W., Bales J. D., Ausley L. W. (2001). Ecosystem Impacts of Three Sequential Hurricanes (Dennis, Floyd, and Irene) on the United States' Largest Lagoonal Estuary, Pamlico Sound, Nc. Proc. Natl. Acad. Sci. U. S. A. 98 (10), 5655–5660. doi: 10.1073/pnas.101097398
Paerl H. W., Pinckney J. L., Fear J. M., Peierls B. L. (1998). Ecosystem Responses to Internal and Watershed Organic Matter Loading: Consequences for Hypoxia in the Eutrophying Neuse River Estuary, North Carolina, USA. Mar. Ecol. Prog. Ser. 166 (8), 17–25. doi: 10.3354/meps166017
Pan J., Gu Y. (2016). Cruise Observation and Numerical Modeling of Turbulent Mixing in the Pearl River Estuary in Summer. Cont. Shelf Res. 120, 122–138. doi: 10.1016/j.csr.2016.03.019
Qian W., Gan J., Liu J., He B., Dai M. (2018). Current Status of Emerging Hypoxia in a Eutrophic Estuary: The Lower Reach of the Pearl River Estuary, China. Estuarine Coastal Shelf Sci. 205, 58–67. doi: 10.1016/j.ecss.2018.03.004
Rabalais N. N., Díaz R. J., Levin L. A., Turner R. E., Zhang J. (2010). Dynamics and Distribution of Natural and Human-Caused Hypoxia. Biogeosciences 7 (2), 585–619. doi: 10.5194/bgd-6-9359-2009
Rabalais N. N., Turner R. E., Justić D. (1998). Charaterization of Hypoxia: Topic 1 Report for the Integrated Assessment of Hypoxia in the Gulf of Mexico. NOAA Coastal Ocean Program. Decision Analysis Series No.15. (Silver Spring: NOAA Coastal Ocean Program) 167.
Rabalais N. N., Turner R. E., Wiseman W. J. (2002). Gulf of Mexico Hypoxia, a.K.a. \"the Dead Zone\". Annu. Rev. Ecol. Syst. 33, 235–263. doi: 10.2307/3069262
Rabouille C., Conley D. J., Dai M., Cai W., Mckee B. (2008). Comparison of Hypoxia Among Four River-Dominated Ocean Margins: The Changjiang (Yangtze), Mississippi, Pearl, and Rhône Rivers. Cont. Shelf Res. 28 (12), 1527–1537. doi: 10.1016/j.csr.2008.01.020
Scully M. E. (2010). Wind Modulation of Dissolved Oxygen in Chesapeake Bay. Estuaries Coasts 33 (5), 1164–1175. doi: 10.1007/s12237-010-9319-9
Scully M. E. (2013). Physical Controls on Hypoxia in Chesapeake Bay: A Numerical Modeling Study. J. Geophys. Res.: Oceans 118 (3), 1239–1256. doi: 10.1002/jgrc.20138
Simpson J. H., Bowers D. B. (1981). Models of Stratification and Frontal Movement in Shelf Seas. Deep-Sea Res. Part A 28 (7), 727–738. doi: 10.1016/0198-0149(81)90132-1
Simpson J. H., Brown J., Matthews J., Allen G. (1990). Tidal Straining, Density Currents, and Stirring in the Control of Estuarine Stratification. Estuaries 13 (2), 125–132. doi: 10.2307/1351581
Simpson J. H., Williams E., Brasseur L. H., Brubaker J. M. (2005). The Impact of Tidal Straining on the Cycle of Turbulence in a Partially Stratified Estuary. Cont. Shelf Res. 25 (1), 51–64. doi: 10.1016/j.csr.2004.08.003
Su J., Dai M., He B., Wang L., Gan J., Guo X., et al. (2017). Tracing the Origin of the Oxygen-Consuming Organic Matter in the Hypoxic Zone in a Large Eutrophic Estuary: The Lower Reach of the Pearl River Estuary, China. Biogeosciences Discussions 14 (18), 1–24. doi: 10.5194/bg-2017-43
Väli G., Meier H. E. M., Elken J. (2013). Simulated Halocline Variability in the Baltic Sea and its Impact on Hypoxia During 1961-2007. J. Geophys. Res.: Oceans 118 (12), 6982–7000. doi: 10.1002/2013JC009192
Wang H., Dai M., Liu J., Kao S., Zhang C., Cai W., et al. (2016). Eutrophication-Driven Hypoxia in the East China Sea Off the Changjiang Estuary. Environ. Sci. Technol. 50, 2255–2263. doi: 10.1021/acs.est.5b06211
Wang J. F., Macdonald D. G., Orton P. M., Cole K., Lan J. (2015). The Effect of Discharge, Tides, and Wind on Lift-Off Turbulence. Estuaries Coasts 38 (6), 2117–2131. doi: 10.1007/s12237-015-9958-y
Wang B., Wei Q., Chen J., Xie L. (2012). Annual Cycle of Hypoxia Off the Changjiang (Yangtze River) Estuary. Mar. Environ. Res. 77, 1–5. doi: 10.1016/j.marenvres.2011.12.007
Wei X., Zhan H., Ni P., Cai S. (2016). A Model Study of the Effects of River Discharges and Winds on Hypoxia in Summer in the Pearl River Estuary. Mar. Pollut. Bull. 113 (1-2), 414–427. doi: 10.1016/j.marpolbul.2016.10.042
Wilson R. E., Swanson R. L., Crowley H. A. (2008). Perspectives on Long-Term Variations in Hypoxic Conditions in Western Long Island Sound. J. Geophys. Res. 113 (C12), C12011. doi: 10.1029/2007jc004693
Xu J., Yin K., Liu H., Lee J. H. W., Anderson D. M., Ho A. Y. T., et al. (2010). A Comparison of Eutrophication Impacts in Two Harbours in Hong Kong With Different Hydrodynamics. J. Marine Syst. 83 (3-4), 276–286. doi: 10.1016/j.jmarsys.2010.04.002
Ye F., Huang X., Shi Z., Liu Q. (2013). Distribution Characteristics of Dissolved Oxygen and Its Affecting Factors in the Pearl River Estuary During the Summer of the Extremely Drought Hydrological Year 2011. China Environ. Sci. 34 (5), 1707–1714.
Yin K. (2002). Monsoonal Influence on Seasonal Variations in Nutrients and Phytoplankton Biomass in Coastal Waters of Hong Kong in the Vicinity of the Pearl River Estuary. Mar. Ecol. Prog. 245, 111–122. doi: 10.3354/meps245111
Yin K. (2003). Influence of Monsoons and Oceanographic Processes on Red Tides in Hong Kong Waters. Mar. Ecol. Prog. 262 (1), 27–41. doi: 10.3354/meps262027
Yin K., Harrison P. J. (2007). Influence of the Pearl River Estuary and Vertical Mixing in Victoria Harbor on Water Quality in Relation to Eutrophication Impacts in Hong Kong Waters. Mar. Pollut. Bull. 54 (6), 646–656. doi: 10.1016/j.marpolbul.2007.03.001
Yin K., Harrison P. J. (2008). Nitrogen Over Enrichment in Subtropical Pearl River Estuarine Coastal Waters: Possible Causes and Consequences. Cont. Shelf Res. 28 (12), 1435–1442. doi: 10.1016/j.csr.2007.07.010
Yin K., Harrison P.J., Xu J. (2010). A Comparison of Eutrophication Processes in Three Chinese Subtropical Semi-Enclosed Embayments With Different Buffering Capacity, Chapter 15. In Kennish M., Paerl H. W. (eds), Coastal Lagoons: Critical Habitats of Environmental Change., (New York: CRC Press, Taylor and Francis Publisher) 367–398. doi: 10.1201/EBK1420088304-c15
Yin K., Lin Z., Ke Z. (2004). Temporal and Spatial Distribution of Dissolved Oxygen in the Pearl River Estuary and Adjacent Coastal Waters. Cont. Shelf Res. 24 (16), 1935–1948. doi: 10.1016/j.csr.2004.06.017
Yin K., Qian P., Chen J. C., Hsieh P. H. D., Harrison P. J. (2000). Dynamics of Nutrients and Phytoplankton Biomass in the Pearl River Estuary and Adjacent Waters of Hong Kong During Summer: Preliminary Evidence for Phosphorus and Silicon Limitation. Mar. Ecol. Prog. 194 (3), 295–305. doi: 10.3354/meps194295
Yin K., Qian P., Wu M., Chen J., Huang L., Song X., et al. (2001). Shift From P to N Limitation of Phytoplankton Growth Across the Pearl River Estuarine Plume During Summer. Mar. Ecol. Prog. 221 (4), 17–28. doi: 10.3354/meps221017
Zhao Y., Uthaipan K., Lu Z., Li Y., Liu J., Liu H., et al. (2021). Destruction and Reinstatement of Coastal Hypoxia in the South China Sea Off the Pearl River Estuary. Biogeosciences 18, 2755–2775. doi: 10.5194/bg-18-2755-2021
Zhou W., Yin K., Harrison P. J., Lee J. H. W. (2012). The Influence of Late Summer Typhoons and High River Discharge on Water Quality in Hong Kong Waters. Estuarine Coastal Shelf Sci. 111 (4), 35–47. doi: 10.1016/j.ecss.2012.06.004
Zhou W., Yuan X., Long A., Huang H., Yue W. (2014). Different Hydrodynamic Processes Regulated on Water Quality (Nutrients, Dissolved Oxygen, and Phytoplankton Biomass) in Three Contrasting Waters of Hong Kong. Environ. Monit. Assess. 186 (3), 1705–1718. doi: 10.1007/s10661-013-3487-6
Zhu Z., Zhang J., Wu Y., Zhang Y., Lin J., Liu S. M. (2011). Hypoxia Off the Changjiang (Yangtze River) Estuary: Oxygen Depletion and Organic Matter Decomposition. Mar. Chem. 125 (1-4), 108–116. doi: 10.1016/j.marchem.2011.03.005
Keywords: hypoxia, wind events, Hong Kong waters, ecosystem buffering, climate change
Citation: Wang K, Yao J, Wang J, Liu H and Yin K (2022) Role of Winds in Interrupting the Formation of Coastal Hypoxia. Front. Mar. Sci. 9:839812. doi: 10.3389/fmars.2022.839812
Received: 20 December 2021; Accepted: 12 April 2022;
Published: 19 May 2022.
Edited by:
Jianfang Chen, Ministry of Natural Resources, ChinaReviewed by:
Wenfei Ni, Pacific Northwest National Laboratory, United StatesTaiping Wang, Pacific Northwest National Laboratory (DOE), United States
Copyright © 2022 Wang, Yao, Wang, Liu and Yin. This is an open-access article distributed under the terms of the Creative Commons Attribution License (CC BY). The use, distribution or reproduction in other forums is permitted, provided the original author(s) and the copyright owner(s) are credited and that the original publication in this journal is cited, in accordance with accepted academic practice. No use, distribution or reproduction is permitted which does not comply with these terms.
*Correspondence: Kedong Yin, eWlua2RAbWFpbC5zeXN1LmVkdS5jbg==
†These authors have contributed equally to this work and share first authorship