- 1Harbor Branch Oceanographic Institute, Florida Atlantic University, Fort Pierce, FL, United States
- 2Unidad Multidisciplinaria de Docencia e Investigación–Sisal, Facultad de Ciencias, Universidad Nacional Autónoma de México, Puerto de Abrigo S/N, Sisal, Yucatán, Mexico
- 3International Chair for Coastal and Marine Studies, Harte Research Institute for Gulf of Mexico Studies, Texas A&M University-Corpus Christi, Corpus Christi, TX, United States
- 4Laboratorio Nacional de Resiliencia Costera (LANRESC), Laboratorios Nacionales, CONACYT, Sisal, Mexico
Depth-generalist coral holobionts inhabit shallow (0–30 m) and mesophotic (30–150 m) reef zones but may exhibit genetic specialization as a result of adaptations to environmental shifts and/or due to a lack of dispersal across depth. The level of depth-dependent genetic structuring varies across reef locations which may impact the roles mesophotic populations play in the persistence and recovery of metapopulations. A depth-generalist coral species, Montastraea cavernosa, was sampled across a shallow to mesophotic gradient at 10, 15, 25, and 35 m at Alacranes and Bajos del Norte reefs on Campeche Bank, Mexico. Both 2bRAD and ITS2 sequencing were used to quantify genetic differentiation of the coral hosts and community structure of their algal endosymbionts (Family Symbiodiniaceae) across depth zones and between these reefs. Significant levels of M. cavernosa genetic differentiation were identified across depth at both reefs, especially between the shallow (10, 15, and 25 m) and mesophotic (35 m) zones. While Symbiodiniaceae hosted by these corals were predominantly Cladocopium species, both depth and reef location were significant factors driving ITS2 type profiles found within each population. The magnitude of depth-dependent genetic structuring of both the coral and Symbiodiniaceae was much greater at Alacranes, relative to Bajos del Norte, suggesting that the refugia potential of mesophotic communities at Alacranes is limited. However, relatively high levels of horizontal coral connectivity between the two reefs, and Bajos del Norte’s location at the entrance to the Gulf of Mexico suggests that it may play an important role in the maintenance of the coral’s regional metapopulation and should be considered in future assessments of this species’ connectivity dynamics and management.
Introduction
Mesophotic coral ecosystems (MCEs, 30–150 m) are relatively light-limited, as compared to their shallow-water counterparts, but still function as important reef habitats, supporting diverse communities of photosynthetically obligate invertebrates, including scleractinian corals (Lesser et al., 2009; Kahng et al., 2017; Lesser et al., 2018). As much as 25–40% of coral species in the Caribbean are considered “depth-generalists” and can be found across shallow depths and into the mesophotic zone (Bongaerts et al., 2010a). These depth-generalist coral holobionts tend to exhibit high phenotypic variation and employ multiple mechanisms to optimize light-capture and adapt to these mesophotic habitats, sometimes even hosting depth-specialist Symbiodiniaceae communities distinct from their shallow counterparts (Bongaerts et al., 2013; Bongaerts et al., 2015; Studivan et al., 2019; Eckert et al., 2020).
As shallow coral reefs continue to decline and technological advances allow for easier and safer access to deeper depths, increased research focus has been placed on exploring and characterizing MCEs (Armstrong et al., 2019; Pyle, 2019). Due to their depth, and oftentimes, distance from human development, researchers have hypothesized that MCEs may be buffered from anthropogenic disturbances relative to their shallow counterparts (Glynn, 1996; Bak et al., 2005; Slattery et al., 2011). Therefore, MCEs may act as coral refugia and mesophotic populations of depth-generalist coral species may have the capacity to re-seed shallow populations following disturbance-driven decline (Bongaerts et al., 2010a; Laverick et al., 2018). The combination of these tenets is termed the “Deep Reef Refugia Hypothesis” (DRRH) but so far our understanding of its real-world validity is limited by the lack of studies and long-term monitoring involving MCEs (Glynn, 1996; Bongaerts et al., 2010a; Bongaerts and Smith, 2019). The few available studies suggest mixed support of the tenets of the DRRH, as some report relatively low levels of bleaching and disease incidence on MCEs while others have identified MCE mortality events associated with these threats (Bak et al., 2005; Smith et al., 2014; Holstein et al., 2015; Smith et al., 2016; Reed et al., 2018; Rocha et al., 2018). In addition, evidence of connectivity between shallow and mesophotic populations also varies among coral species, location, and even among depth zones within the traditional “shallow” and “mesophotic” definitions (e.g. upper mesophotic: 30–60 m, or lower mesophotic 60–150 m, Bongaerts et al., 2017).
Applying molecular techniques to the depth-stratified collection of corals can characterize potential ecological differences between shallow and mesophotic populations of depth-generalist coral species and begin to evaluate the refugia potential of mesophotic populations (Bongaerts et al., 2015; Studivan and Voss, 2018b; Eckert et al., 2019; Drury et al., 2020; Eckert et al., 2020; Sturm et al., 2021). Restriction-site associated DNA sequencing (RADseq) methods have become an increasingly popular tool in the field of coral population genetics, as they allow for relatively inexpensive but high-resolution genotyping (on the order of thousands of single nucleotide polymorphisms, SNPs; Puritz et al., 2014). RADseq methods require fewer replicates per population than more traditional molecular markers and can be used to study non-model species without genomic resources (Wang et al., 2012; Willing et al., 2012). Genotyping individual coral colonies across many SNP loci allows for quantification of even low levels of genetic differentiation among populations which can serve as a proxy measure for larval connectivity among them (Selkoe and Toonen, 2011; Bradbury et al., 2015). High levels of genetic connectivity between shallow and mesophotic coral populations would suggest historic or contemporary levels of larval connectivity between these populations (Holstein et al., 2016; Garavelli et al., 2018; Studivan and Voss, 2018a).
Genetic characterization of the coral’s in hospite Symbiodiniaceae communities is most commonly accomplished through sequencing of the internal transcribed spacer-2 (ITS2) region of the ribosomal RNA gene (Hume et al., 2019). Despite its widespread use, this marker has limitations associated with its multi-copy nature which has reduced the ability to resolve intra- vs. intergenomic diversity (Thornhill et al., 2007). However, novel analytical methods like SymPortal have been developed to improve the resolution of putative Symbiodiniaceae taxa (Hume et al., 2019). ITS2 sequencing can be employed in addition to coral genetics to investigate how both the coral host and Symbiodiniaceae communities may differ across a shallow to mesophotic depth gradient. If the structure of the Symbiodiniaceae communities hosted by these colonies is similar regardless of depth zone, it would suggest that the host-symbiont partnership is phenotypically flexible enough to be advantageous regardless of depth-associated environmental shifts (Bongaerts et al., 2011).
This is particularly important for coral species that acquire their algal symbiont assemblages via vertical transmission (from parent to offspring) as larvae may acquire symbionts that were well-suited to their natal environment but may be unfavorable if the larvae settle in a distinct habitat (Hartmann et al., 2017). For a vertically transmitting species, if the larvae acquire depth-specialist algal symbionts from a mesophotic parent colony there may be reduced recruitment success to shallow populations, functioning as a post-zygotic barrier to connectivity between shallow and mesophotic coral populations and reducing mesophotic populations’ refugia potential (Shlesinger and Loya, 2021). Coral species that acquire algal symbionts via horizontal transmission (acquiring symbionts from their surrounding environment as larvae or juveniles) may exhibit high levels of genetic similarity of the coral host across depth despite strong depth-dependent structuring of their algal symbiont communities (Fujiwara et al., 2021). In this case, it would appear that depth-dependent genetic structuring of algal symbiont communities would not function as a barrier to vertical connectivity or reduce mesophotic refugia potential. However, species that acquire algal symbionts via horizontal transmission tend to exhibit depth-dependent genetic structuring of either both or neither of the partners which may be a result of co-evolutionary pressures on the holobiont as a whole to adapt to a particular environment or be phenotypically flexible (Serrano et al., 2014; Polinski and Voss, 2018; Studivan and Voss, 2018a; Eckert et al., 2019; Eckert et al., 2020). Overall, high levels of genetic similarity in both the coral host and the Symbiodiniaceae communities across depth would offer evidential support of the DRRH (Bongaerts et al., 2010b). In contrast, depth-dependent genetic structuring of the coral host and/or Symbiodiniaceae communities suggests that there may be pre or post-zygotic barriers to connectivity between shallow and mesophotic coral populations that could potentially limit the refugia capacity of the mesophotic population (Levitan et al., 2004; Bongaerts et al., 2011; Eckert et al., 2019; Eckert et al., 2020).
Montastraea cavernosa is a depth-generalist coral species that has been widely used to estimate levels of vertical genetic connectivity and quantify depth-dependent structuring of its Symbiodiniaceae communities (Serrano et al., 2014; Polinski and Voss, 2018; Studivan and Voss, 2018b; Eckert et al., 2019; Dodge et al., 2020; Eckert et al., 2020; Drury et al., 2020; Sturm et al., 2020). This species is dominant across the majority of reefs throughout the Tropical Western Atlantic, occurs across depths ranging from 3–113 m, and is a broadcast spawning species which may support potentially high levels of genetic connectivity across wide geographic and depth ranges (Reed, 1985; Szmant, 1991; Favoretto et al., 2020). Like most broadcast spawning species, M. cavernosa acquires its algal symbionts from the surrounding environment during early ontogeny (Abrego et al., 2009; Baird et al., 2009). In general, M. cavernosa’s Symbiodiniaceae communities are dominated by Cladocopium species, usually type C3, but background levels of Breviolum and Durusdinium species have been identified across some M. cavernosa populations (Lesser et al., 2010; Klepac et al., 2015; Polinski and Voss, 2018; Eckert et al., 2020).
The levels of vertical connectivity of M. cavernosa across depth gradients tend to be location-specific and depth-dependent “genetic breaks” do not always coincide with traditional definitions of shallow and mesophotic depth zones. For example, on the Belize Barrier Reef, significant levels of genetic differentiation were observed between samples collected at relatively shallow (10 and 16 m) and relatively deep zones (25 and 35 m; Eckert et al., 2019). In contrast, in the Northwest Gulf of Mexico, genetic connectivity among shallow and mesophotic M. cavernosa populations was higher (Studivan and Voss, 2018a). In a study of vertical connectivity of M. cavernosa in the Florida Keys between paired shallow and mesophotic sites, genetic connectivity in the Northern Dry Tortugas and the Upper Keys was relatively high but much lower between shallow and mesophotic Southern Dry Tortugas and Lower Keys’ populations (Sturm et al., 2021). Even across a relatively shallow sampling gradient in the Florida Keys, significant genetic differentiation was identified between M. cavernosa sampled from 0–10 m and 25–30 m depth zones in the Lower Keys but not in the Upper Keys or Northern Dry Tortugas where genetic connectivity was relatively high (Serrano et al., 2014).
Similar to regional variation in depth-dependent genetic structuring of the M. cavernosa coral host, the level of depth-dependent structuring of the Symbiodiniaceae communities hosted by these corals also varies among reef locations. In the Northwest Gulf of Mexico, Symbiodiniaceae assemblages among M. cavernosa were similar across all sampled banks, and between shallow and mesophotic depth zones (Polinski and Voss, 2018). On the Belize Barrier Reef, the majority of samples harbored relatively homogeneous Symbiodiniaceae communities, however, some shallow M. cavernosa hosted Symbiodiniaceae type profiles that were not found in the deeper populations (Eckert et al., 2020). These shallow-only Symbiodiniaceae types might represent shallow-specialist communities and offer some type of physiological advantage for the holobiont in the shallow environment. Additionally, in the Bahamas, Symbiodiniaceae community structure was relatively homogeneous across M. cavernosa samples from 0–60 m but exhibited a significant shift to putative deep-water specialist communities in the lower mesophotic zone (>60 m; Lesser et al., 2010). Taken together, these previous results highlight the wide variation observed in the level of depth-dependent host and algal symbiont genetic structuring across different locations. These studies also found that if depth-dependent structuring is present, it may not coincide with the traditionally defined shallow-mesophotic boundary, underscoring the importance of developing a sampling design across a finer scale depth gradient.
Coral reef ecosystems across Campeche Bank in the southeastern Gulf of Mexico represent a novel opportunity to expand our understanding of the regional variation of depth-dependent differentiation of M. cavernosa host and symbiont populations. Campeche Bank reefs are heavily influenced by the Yucatan/Loop Current System, potentially making these coral populations an important stepping stone in the regional connectivity of this species (Veron and Stafford-Smith, 2000; Sanvicente-Añorve et al., 2014). The Campeche Bank reef system consists of a series of coral platforms which include Alacranes reef, the largest bank-atoll system in the Gulf of Mexico. Alacranes is located ~135 km off the coast of the Yucatan peninsula and ranges in depth from ~1–55 m (Chávez et al., 2007; Favoretto et al., 2020). To the northeast of Alacranes reef is the relatively less characterized Bajos del Norte reef system, which consists of a series of submarine coral ridges which are located ~160 km to the north of the Yucatan peninsula and range from ~5–70 m depth (Tuz-Sulub and Brulé, 2015; Favoretto et al., 2020). Montastraea cavernosa is the dominant scleractinian species across both Alacranes and Bajos del Norte reefs which represent important, although still understudied, shallow and mesophotic habitat for the species (Favoretto et al., 2020). These reefs provide an opportunity to investigate patterns of genetic differentiation of both coral hosts and their Symbiodiniaceae communities across a shallow to mesophotic depth gradient.
Materials and Methods
Sample Collection
Montastraea cavernosa were sampled across four target depth zones (shallow: 10 m, 15 m, 25 m, and mesophotic: 35 m) at Alacranes and Bajos del Norte reefs by SCUBA divers on air during a July 2019 expedition onboard the M/V Caribbean Kraken (Figure 1 and Supplementary Table S1). Approximately fifteen samples per depth zone per reef were collected. Due to limitations associated with the dive tour itinerary of the Caribbean Kraken, limited bathymetry data available for some of these locations, and lack of available technical diving platforms in the region which led to restrictive dive profiles, it was not possible to collect samples from deeper than 35 m nor from every depth zone at a singular location for each reef. However, horizontal distances between sampling locations within a reef relative to the distances between reefs were minimized. The distance between two sites within Alacranes reef did not exceed 22 km, while the shortest pairwise distance between Alacranes and Bajos del Norte reefs was 125 km.
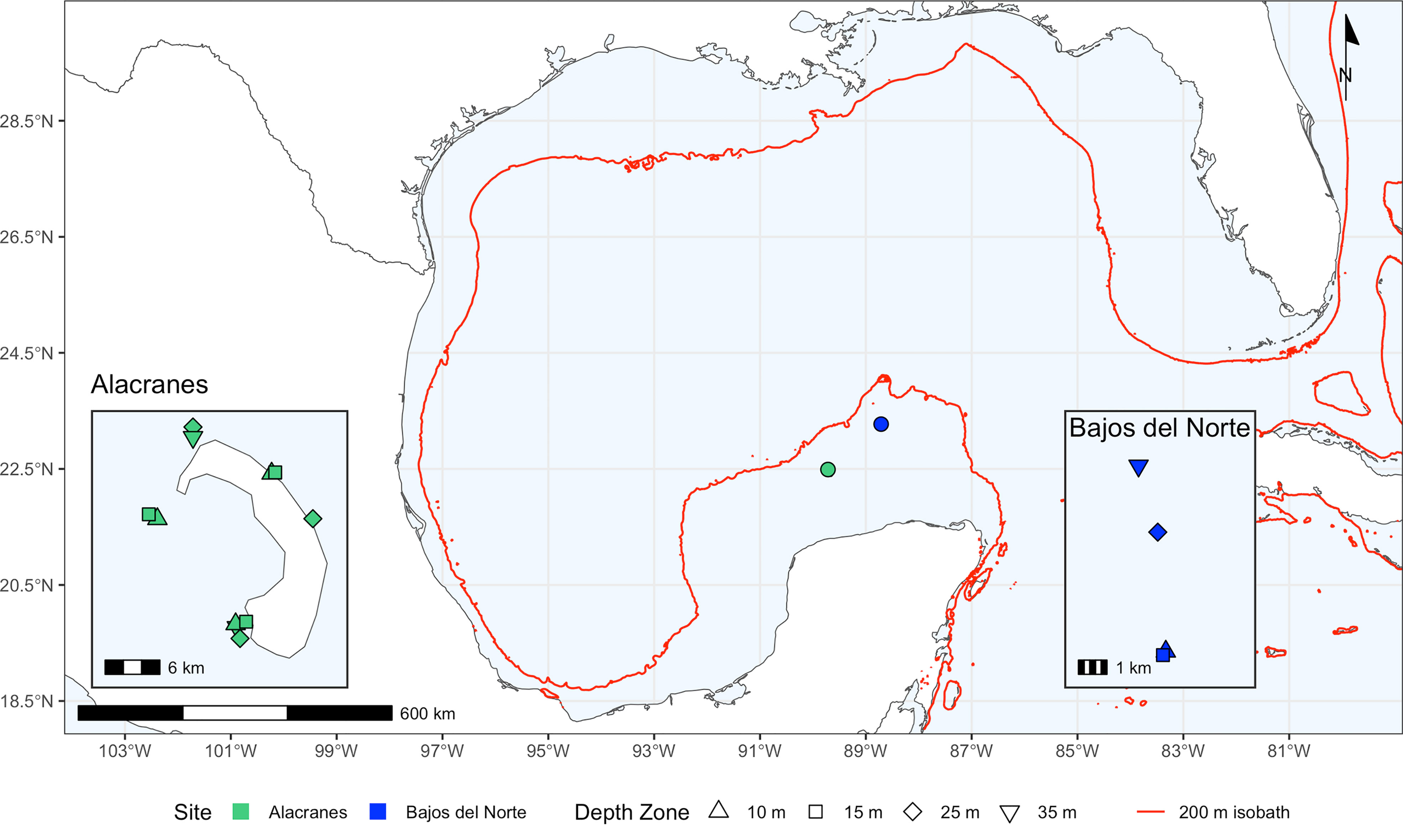
Figure 1 Coral sampling sites at Alacranes and Bajos del Norte with shape indicating the depth zone of samples collected at that location. The red line on the main map indicates the 200 m isobath which approximates the continental shelf margin.
Small tissue and skeletal fragment biopsies were collected from the edge of target colonies using a hammer and chisel and then placed in individually labeled plastic bags. Scaled photographs were taken of each colony pre- and post-sampling and colony depth was recorded. Following each SCUBA dive, samples were split and immediately preserved in duplicate 2 mL tubes pre-filled with 1 mL of 100% molecular-grade ethanol. Samples were maintained at -20°C for the remainder of the expedition and were shipped overnight back to FAU Harbor Branch on ice. Once in the laboratory, the ethanol was replaced with fresh 100% molecular-grade ethanol and the samples were stored at -80°C until DNA extraction.
Genomic DNA Extraction
Prior to DNA extraction, the tissue was transferred into a 2 mL tube with 500 μL of TRIzol reagent. The samples were stored in TRIzol at 4°C for a minimum of 1 hour and up to multiple days. Tubes were then centrifuged at 20,000 x g for two min to pellet the tissue, which was transferred to a new 2 mL fastprep tube with ~0.075 grams of 0.5 mm glass beads to macerate the tissue and promote cell lysis. Genomic DNA was extracted using a modified dispersion buffer/phenol-chloroform-isoamyl alcohol extraction protocol (Sturm, 2020). The extracted DNA was cleaned using a Zymo DNA Clean and Concentrator Kit following manufacturer’s protocols and then eluted in 15 μL of nuclease-free water. DNA quality was checked on a NanoDrop 2000 (Thermo Fisher) and dsDNA quantity was measured using a broad-range assay kit on a Qubit 4.0 fluorometer (Thermo Fisher). Samples were then diluted and equalized to 25 ng μL-1 concentrations.
2bRAD Library Preparation and Sequencing
A 2bRAD SNP approach was employed to genotype M. cavernosa, preparing DNA libraries following Wang et al. (2012) including modifications described in the protocol’s GitHub repository (https://github.com/z0on/2bRAD_denovo). Notably, 100 ng of DNA was added to the initial digestion reaction with BcgI enzyme. In addition, fully degenerate 5’ adapters and twelve uniquely indexed 3’ adapters were ligated onto the sticky ends of the digested sequences, allowing for 12 sample ligations to be pooled prior to amplification and for filtering of PCR duplicates in downstream analyses. Three samples had 2bRAD libraries prepared and sequenced in triplicate which were used downstream as a sequencing quality check and to identify natural clones (Sturm et al., 2020). The final pooled library underwent automated size selection with Pippin Prep (Sage Science) before conducting 100 bp single-end sequencing on an Illumina NovaSeq S1 flow cell with 20% phiX at the University of Texas at Austin’s Genome Sequencing and Analysis Facility.
Symbiodiniaceae ITS2 Library Preparation and Sequencing
To describe putative Symbiodiniaceae taxa associated with these M. cavernosa colonies, the ITS2 region of the ribosomal RNA gene was targeted for library preparation and sequencing. The same genomic DNA extracts that were used in the 2bRAD library preparations were also amplified with the ITS2 specific primers SYM_VAR_5.8S2 and SYM_VAR_REV which were modified to include forward and reverse indexed Illumina adaptors for downstream pooling and sequencing (Hume et al., 2018; Eckert et al., 2020). The 30 µL PCR reactions included the following components: 1U Takara HS Taq, 1X Takara Taq Buffer, 0.15 µM each forward and reverse primer, 0.25 mM dNTP mixture, and 25 ng of the template DNA. The PCRs were run with an initial melt of 95°C for 5 min, followed by 28 cycles of 95°C for 30 s, 56°C for 30 s, and 72°C for 30 s, and a final extension of 72°C for 5 min. After 28 cycles, 5 µL of the PCR product was run on a 2% agarose gel to visually verify amplification.
The remaining 25 µL of PCR product was cleaned using the Zymo DNA Clean and Concentrator Kit following manufacturer’s protocols and then eluted in 12 μL of nuclease-free water. dsDNA quantity in the PCR product was measured again using a broad-range assay kit on a Qubit 4.0 fluorometer (Thermo Fisher) and re-diluted to 5 ng μL-1 concentrations. These purified PCR products were also sent out to the University of Texas at Austin’s Genome Sequencing and Analysis Facility. 50 ng of ITS2 product was incorporated into an indexing PCR (7 cycles) to incorporate a unique combination of indexed forward and reverse Illumina adapters. The libraries were then equalized and pooled prior to 250 bp paired-end sequencing on an Illumina MiSeq (V2 chemistry) spiked with 20% phiX.
2bRAD Population Genetic Structure Analyses
2bRAD reads were demultiplexed and the adapters trimmed using custom Perl scripts (https://github.com/z0on/2bRAD_denovo) and further quality-filtered using the fastq_quality_filter in the FASTX-Toolkit v0.0.14 (≥90% of bases with Phred quality scores ≥20; Gordon & Hannon, 2010). Trimmed and quality-filtered reads were aligned to a algal symbiont metagenome assembled by concatenating available genomes for species belonging to four Symbiodiniaceae genera (formerly clades A–D), Symbiodinium microadriacticum (Aranda et al., 2016), Breviolum minutum (Shoguchi et al., 2013), Cladocopium goreaui (Liu et al., 2018), and Durusdinium trenchii (Shoguchi et al., 2021) using the sequence aligner Bowtie2 (Langmead and Salzberg, 2012). These reads were then aligned to the M. cavernosa genome and any reads that aligned to both the Symbiodiniaceae and the coral host genomes were removed from further analysis. All high-quality reads that did not align to the algal symbiont metagenomic reference were aligned to the M. cavernosa genome. This iterative alignment approach divided reads into those that aligned solely to the Symbiodiniaceae genomes and those that aligned solely to the M. cavernosa genome. The latter were retained for downstream analyses of coral population genetic structure.
The program ANGSD v0.933 (Korneliussen et al., 2014) was used to generate genotype likelihoods for the dataset and was run with the following filters: a minimum mapping quality score of 20, minimum base quality score of 25, maximum p-value of 10-5 that a locus is variable, at least 75% of non-missing genotypes across samples, minimum p-value for deviation from Hardy-Weinberg equilibrium of 10-5, minimum p-value for strand bias of 10-5, minimum allele frequency of 0.05, and a filter that removed any tri-allelic SNPs. An identity-by-state (IBS) matrix was generated for the full dataset and used to create a cluster dendrogram using the function hclust in R v3.6.2. Pairs of samples that exhibited levels of genetic similarity to one another near to the level of the technical triplicate groups were identified as naturally occurring genetic clones and omitted from further analyses.
ANGSD was re-run on the clones-removed dataset with the same filters as described above to generate an IBS genetic distance matrix. The IBS matrices were used to conduct a Principal Coordinates Analysis (PCoA) using the cmdscale function in the package vegan v2.5.6 in R (Oksanen et al., 2019). In addition, the BCF file generated by ANGSD was converted to a genlight file format suitable for analysis using the program poppr v2.8.3 in R (Kamvar et al., 2014). poppr was used to conduct an analysis of molecular variance (AMOVA, 999 permutations), and the package StAMPP v1.5.1 was used to calculate pairwise fixation indices (FST) between the populations and corresponding p-values (999 permutations; Pembleton et al., 2013). ANGSD was also run on the clones-removed dataset including both variant and invariant sites and without filters that distort allelic frequencies. Heterozygosity was calculated from ANGSD-generated genotype likelihoods across all sites (variant and invariant) and SNPs using a custom R script (https://github.com/z0on/2bRAD_denovo).
Population structure models for clusters K = 1–11 were generated using the program NGSAdmix (Skotte et al., 2013). The most likely number of genetic clusters (the best value of K) was estimated using multiple methods. First, the likelihood values from each NGSAdmix run (10 iterations for each value of K) were imported into the program CLUMPAK which uses the Evanno method to estimate the best value of K (Evanno et al., 2005; Kopelman et al., 2015). Then, the same NGSAdmix runs were imported into the program StructureSelector, which employs the Puechmaille method to generate four different estimators for the optimal value of K (Puechmaille, 2016; Li and Liu, 2018). ADMIXTURE v.1.3.0 and Discriminant Analysis of Principal Components procedures were also used to generate population structure models from the hard-called genotypes for clusters K = 1–11 as additional methods to estimate the optimal value of K (Alexander et al., 2009; Jombart et al., 2010; Jombart and Ahmed, 2011).
The program BAYESCAN v2.1 was employed to identify putative outlier SNP loci (50,000 burn-in, 5000 iterations; Foll and Gaggiotti, 2008). BAYESCAN employs Bayesian models to conduct genome scans and identify loci putatively under natural selection pressures using differences in allele frequencies among sample populations. Outlier SNP loci were compared against annotated gene regions (within ± 2 kb) of the M. cavernosa genome to identify putative functional effects (Rippe et al., 2021).
Symbiodiniaceae Typing With ITS2 Amplicon Analyses
Demultiplexed, paired forward and reverse ITS2 fastq.gz files were submitted to SymPortal and run remotely through the analytical framework and database (Hume et al., 2019). Within this framework, standardized quality control functions were employed to reduce sequencing artifacts and non-Symbiodiniaceae sequences (Camacho et al., 2009; Schloss et al., 2009; Eren et al., 2015; Hume et al., 2019). Each sample’s ITS2 sequences were separated by genera (formerly clade) and all genera-sequence collections with fewer than 200 sequences were excluded from further analysis to reduce sequencing depth artifacts (Hume et al., 2019). The retained ITS2 sequences were compared to ITS2 sequences in other samples from this dataset and across the SymPortal database which hosts sequencing information from all previously analyzed datasets submitted to SymPortal. Sets of sequences found across a sufficient number of samples are characterized as defining intragenomic variants (DIVs). The sample’s characterizing DIVs and their relative abundances are used to determine their ITS2 type profiles which may function as putative Symbiodiniaceae genotypes. These ITS2 type profiles are named based on the DIVs that make up that profile listed from highest to lowest abundance (separated by hyphens). If some of the samples sharing a type profile have differing DIVs that are most abundant those are indicated in the profile’s nomenclature by a slash.
Absolute count tables of ITS2 sequences and ITS2 type profiles produced by SymPortal were used as data inputs in downstream statistical analysis pipelines in R to examine potential differences in Symbiodiniaceae communities across depth zone or site (Hume et al., 2019; Eckert, 2020). A PCoA was conducted on a Bray-Curtis distance matrix generated from samples ITS2 type profiles using the package vegan (Oksanen et al., 2019). Differences in beta diversity between reef locations (Alacranes vs. Bajos del Norte) and across depth zones were analyzed using the betadisper function in the package vegan. A permutational multivariate analysis of variance (PERMANOVA; 9,999 permutations) was conducted on the Bray-Curtis distance matrix generated from samples ITS2 type profiles. PERMANOVA was conducted using the adonis function in the program vegan to compare Symbiodiniaceae communities between reef locations and across depth zones, further pairwise comparisons between depth zones were analyzed with the pairwise.adonis v0.0.1 package (Arbizu, 2020).
Results
Of the 126 M. cavernosa samples collected in total, 124 samples were utilized in the ITS2 analysis pipeline and 98 were utilized in the 2bRAD analysis pipeline (Table 1). Some samples were excluded from analyses due to complete sample consumption, failed library preparations, or poor sequence quality. 2bRAD sequencing generated a total of 539 million reads, averaging 5.1 million reads per sample library. Following PCR de-duplication and quality filtering steps a total of 224 million reads were retained, or an average of 2.1 million reads per sample library. On average, 13.1% of all high-quality reads for a sample aligned to the algal symbiont metagenome reference. Of the reads that did align to the Symbiodiniaceae genomes, an overwhelming majority (on average 98.8%) aligned to the Cladocopium genome. Following removal of these algal symbiont aligned reads, an IBS genetic distance matrix calculated by ANGSD from the M. cavernosa genotype likelihoods revealed a singular pair of putative natural clones from the 25 m depth zone at Bajos del Norte (Table 1).
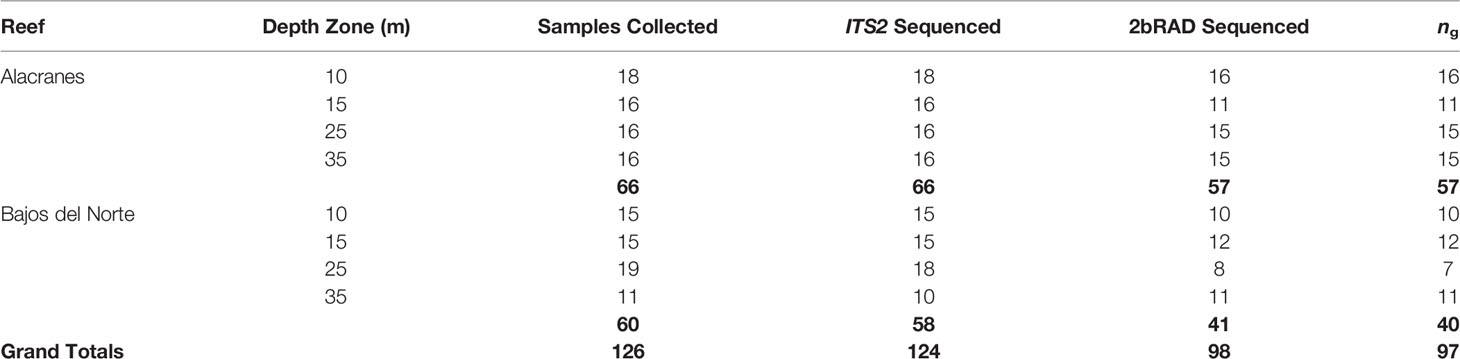
Table 1 The number of samples collected at each depth zone from each reef; the number of samples with successfully sequenced ITS2 libraries that were included in the Symbiodiniaceae community analysis; the number of samples with successfully sequenced 2bRAD libraries; and the number of samples that were identified as unique multilocus genotypes (i.e. clones removed, ng) that were retained in the coral host population genetics analysis. Bolded values indicate totals for each reef location and grand totals.
With all but one sample removed from the pair of natural clones and the technical triplicate libraries, ANGSD generated a total of 10,321 M. cavernosa SNP loci that passed the previously described filters. Heterozygosity did not vary widely across sample population, ranging from 0.00279–0.00317 across all sites (variant and invariant) and 0.250–0.276 across variant sites (Supplementary Table S2). An IBS genetic distance matrix calculated from the clones-removed M. cavernosa dataset was used to conduct a PCoA (Figure 2). Samples tended to cluster primarily based on depth zone and not reef location. Samples from the mesophotic (35 m) depth zones were relatively distanced from the majority of samples across the shallow 10, 15, and 25 m depth zones. Mesophotic, 35 m, samples from Alacranes were more distanced than mesophotic, 35 m, samples from Bajos del Norte were from shallow samples.
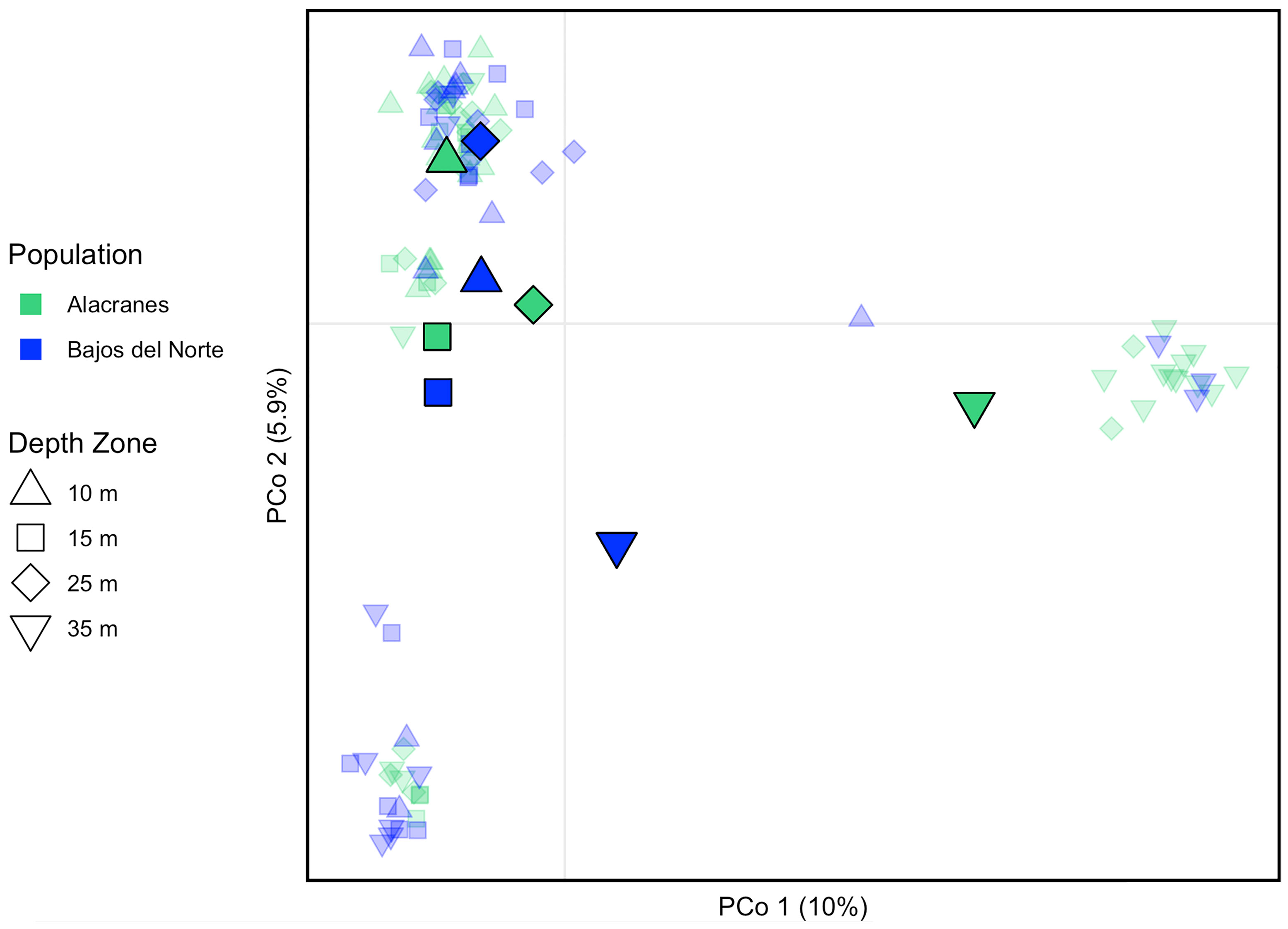
Figure 2 Principal coordinates analysis based on identity-by-state (IBS) distance matrix. Individual samples are represented by small, transparent symbols whereas population centroids are indicated by larger, solid symbols. Color indicates reef and shape indicates depth zone of each sample and population centroid. The percent variation explained by each axis is indicated.
Analysis of molecular variance (AMOVA) indicated that a significant portion of genetic variation is attributed to differences among sample populations (2.41%, SS7,193 = 14,224.94, p < 0.001). Pairwise FST values identified significant pairwise population differentiation (FDR-corrected p < 0.05) for the majority of comparisons between depth zones and reef locations (Figure 3). However, non-significant comparisons were identified between both of the 15 m populations at Alacranes and Bajos del Norte, the 15 m and 25 m Alacranes populations, and the Bajos del Norte-15 m and the Alacranes-25 m populations. Pairwise FST values between the mesophotic, Alacranes-35 m and all other shallow populations were the highest (FST = 0.054–0.074) but were much lower when compared to the Bajos del Norte-35 m population (FST = 0.021). In contrast, pairwise FST comparisons between the mesophotic Bajos del Norte-35 m population and all other populations were much lower, with FST values ranging between 0.006–0.026. Within depth zone FST comparisons between the two reefs ranged from 0–0.021, with the highest within depth zone pairwise comparison between the two mesophotic populations, as mentioned previously (Figure 3).
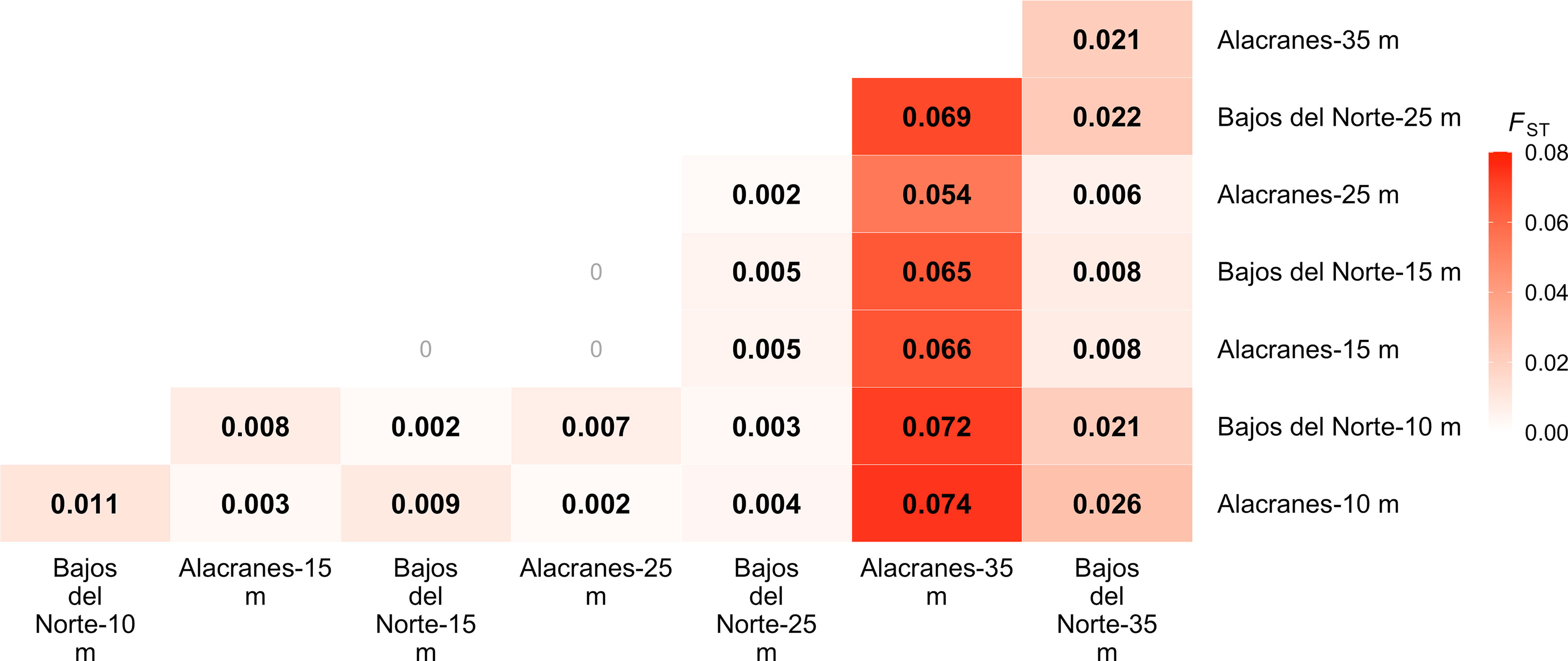
Figure 3 Heat map representations of pairwise population differentiation as estimated by fixation index (FST). Values within cells are estimated FST with increasing intensity of the color red corresponding to increasing FST values. Bolded FST values denote significant differentiation between populations (post FDR-correction, p < 0.05).
Depending on the method and estimator, K = 2 or K = 3 were selected as the best values of K, therefore admixture plots were generated for both (Figure 4). In the K = 2 plot, the majority of samples, especially those from the 10, 15, and 25 m depth zones at both Alacranes and Bajos del Norte, are dominated by the turquoise genetic cluster while the mesophotic Alacranes-35 m population is dominated by the yellow cluster. Individual samples dominated by this yellow cluster were also present in the Alacranes-25 m population and the Bajos del Norte-35 m population, suggesting that this is a “depth-specialist” genetic cluster. In the K = 3 plot, there is also an addition of a pink genetic cluster. Members of this pink genetic cluster occur at both reefs but are more prevalent at Bajos del Norte across every depth zone except at 25 m, although this population had fewer sample replicates overall. Altogether, based on this NGSAdmix analysis, Alacranes demonstrates a stronger pattern of depth-dependent genetic structuring (increased proportion of membership to the yellow genetic cluster with depth) than Bajos del Norte.
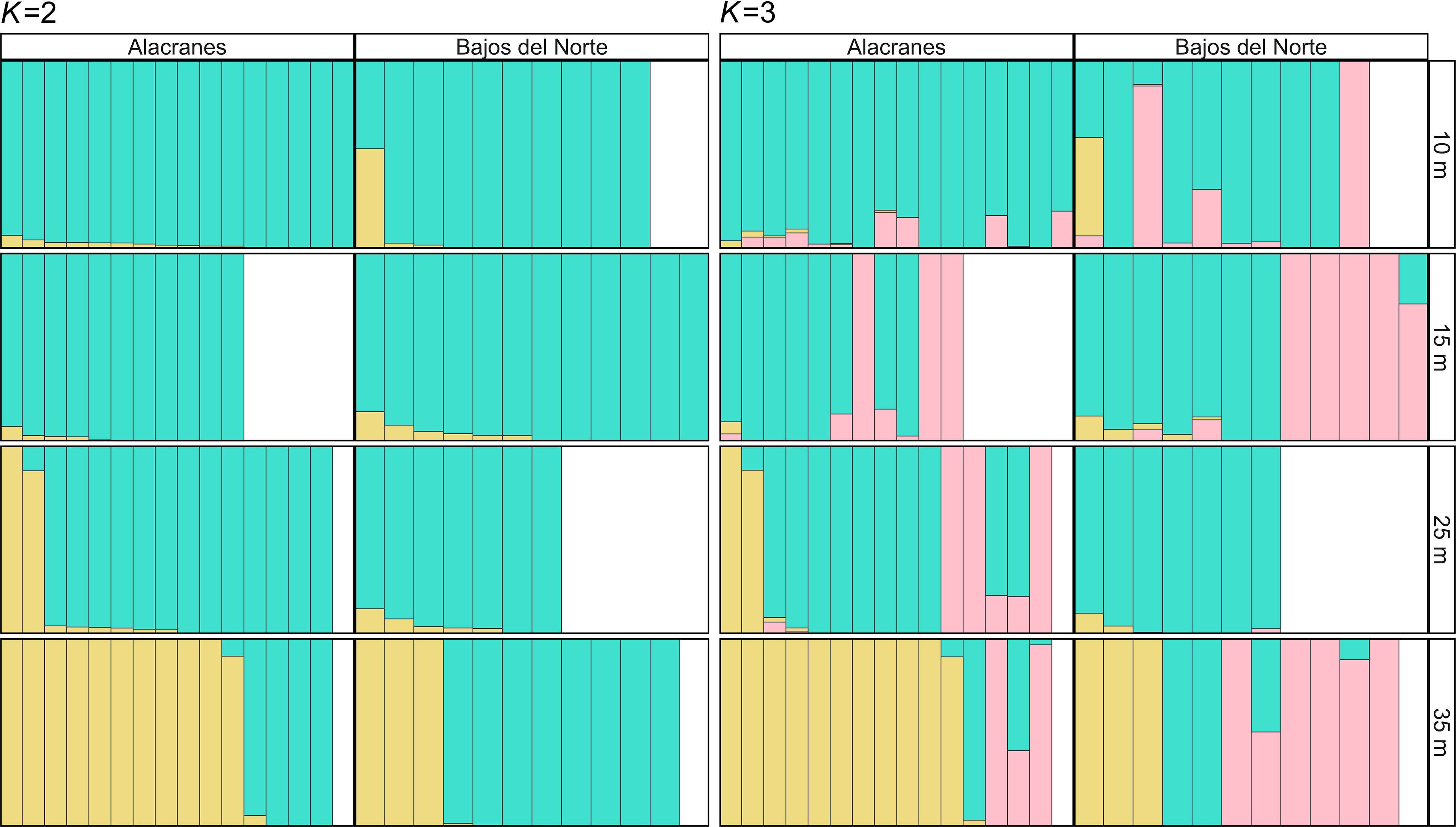
Figure 4 Population structure models generated for Montastraea cavernosa coral host populations across the four depth zones and two reefs. The Evanno and Puechmaille methods were used to estimate the optimal values of K, and the models K = 2 and K = 3 were selected as the most likely number of genetic clusters, represented by the colors turquoise, yellow, and pink. Each bar indicates individual M. cavernosa samples and the relative proportion of the three colors represent the relative likelihood of membership to each of the two or three proposed genetic clusters.
The program BAYESCAN identified 35 outlier SNP loci (FDR-corrected p-value < 0.1) potentially under diversifying selection. Of these 35 putative outlier SNP loci, only 8 were identified within ± 2 kb of annotated M. cavernosa gene regions (Supplementary Table S3). These genes were classified as functioning in carbohydrate and lipid metabolism and transport; transcription; signal transduction; cytoskeletal structure; as well as those with unknown function.
ITS2 sequencing generated a total of 4.05 million reads, averaging 32,688 reads per sample library. Following SymPortal’s quality filtering steps, a total of 1.78 million reads were retained, for an average of 14,428 reads per sample library. 99.8% of all filtered sequences were assigned to Cladocopium. A total of 12 unique ITS2 type profiles were identified. All sample type profiles were dominated by Cladocopium, especially C3 type algal symbionts (Figure 5). Only a few samples exhibited background levels of Symbiodiniaceae from a different genus, Gerakladium, type G3.
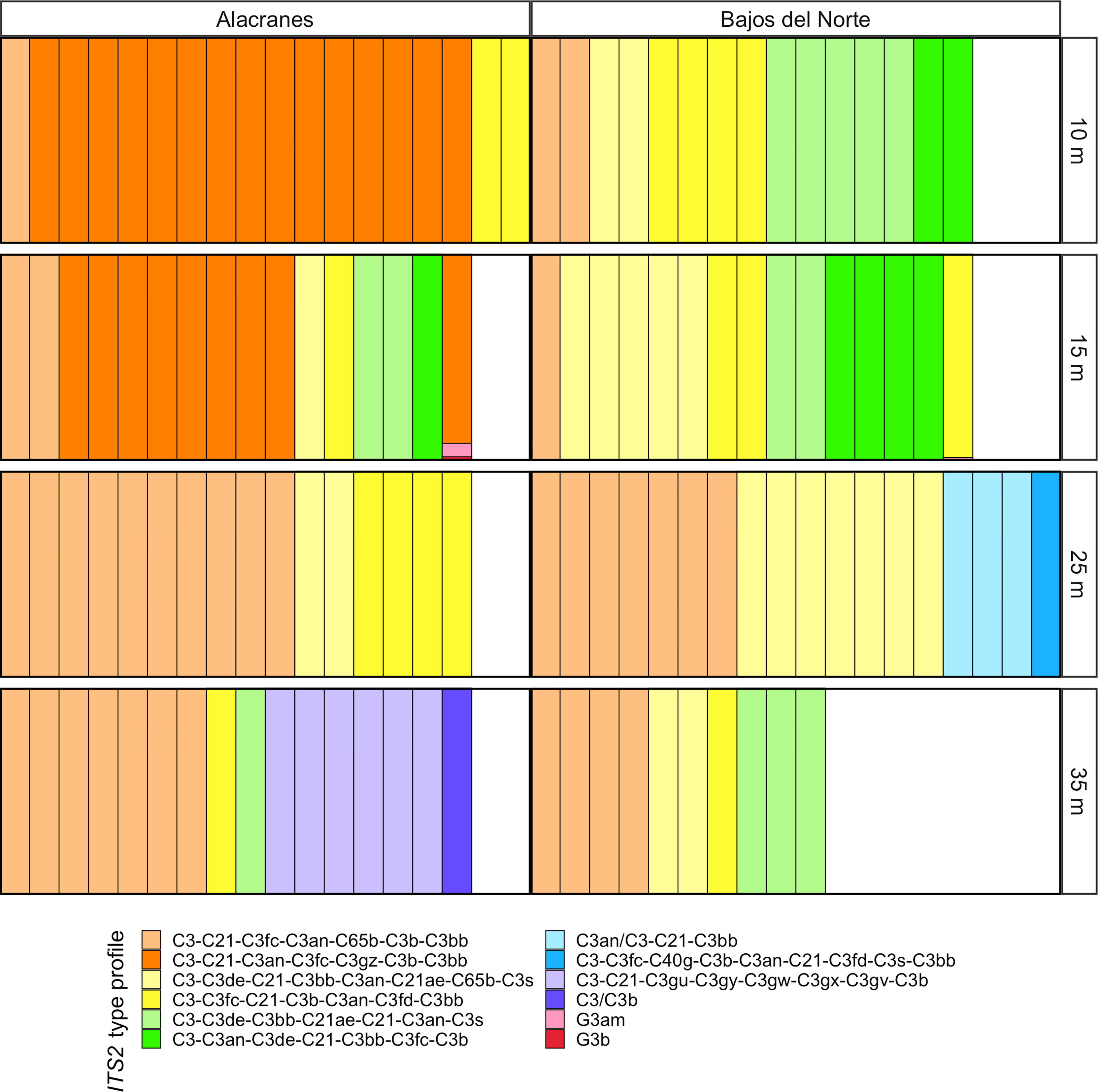
Figure 5 The relative proportion of ITS2 type profiles of the Symbiodiniaceae communities hosted by the Montastraea cavernosa coral samples across the four depth zones and two reefs. ITS2 type profiles are listed in order of overall abundance. ITS2 type profiles are named for the defining intragenomic variants (DIVs) used to characterize them. DIVs in a type profile name are listed in order of abundance with slashes separating DIVs that are co-dominant across samples.
In general, Alacranes demonstrated stronger patterns in depth-dependent structuring of Symbiodiniaceae communities and more type profiles that were endemic to that reef. For example, the C3-C21-C3an-C3fc-C3gz-C3b-C3bb type profile (represented by the dark orange color, Figure 5) dominated the 10 and 15 m zones at Alacranes but was not identified in any other population. The mesophotic 35 m Alacranes population also had samples that hosted two endemic type profiles (light purple: C3-C21-C3gu-C3gy-C3gw-C3gx-C3gv-C3b and dark purple: C3/C3b). In contrast, only four samples at the 25 m depth zone hosted symbiont type profiles endemic to a Bajos del Norte population (light blue: C3an/C3-C21-C3bb and dark blue: C3-C3fc-C40g-C3b-C3an-C21-C3fd-C3s-C3bb). Bajos del Norte populations were dominated by more depth and reef-generalist Symbiodiniaceae type profiles but while certain profile types like light yellow: C3-C3de-C21-C3bb-C3an-C21ae-C65b-C3s, light green: C3-C3de-C3bb-C21ae-C21-C3an-C3s, and neon green: C3-C3an-C3de-C21-C3bb-C3fc-C3b were found across both reefs, they were more prevalent at Bajos del Norte. The most abundant profile type, light orange: C3-C21-C3fc-C3an-C65b-C3b-C3bb, was identified in samples across all depth zones and at both reefs but was most prevalent at the 25 and 35 m depth zones.
PCoA visualization of the pairwise Bray-Curtis distance between ITS2 type profiles generally indicates separation of the samples’ symbiont communities based on reef location along PCo2 (Figure 6). Within a reef, the relatively shallow 10 and 15 m samples tend to be split from the relatively deep 25 and 35 m samples. However, the samples from the four depth zones across Bajos del Norte are generally more closely clustered to one another than the Alacranes samples. In addition, the 10 and 15 m Alacranes samples are more highly differentiated from the 10 and 15 m Bajos del Norte samples when compared to the differentiation among 25 and 35 m samples from these two reefs, suggesting that deeper Symbiodiniaceae communities appear to be more uniform across geographic location than the shallow communities.
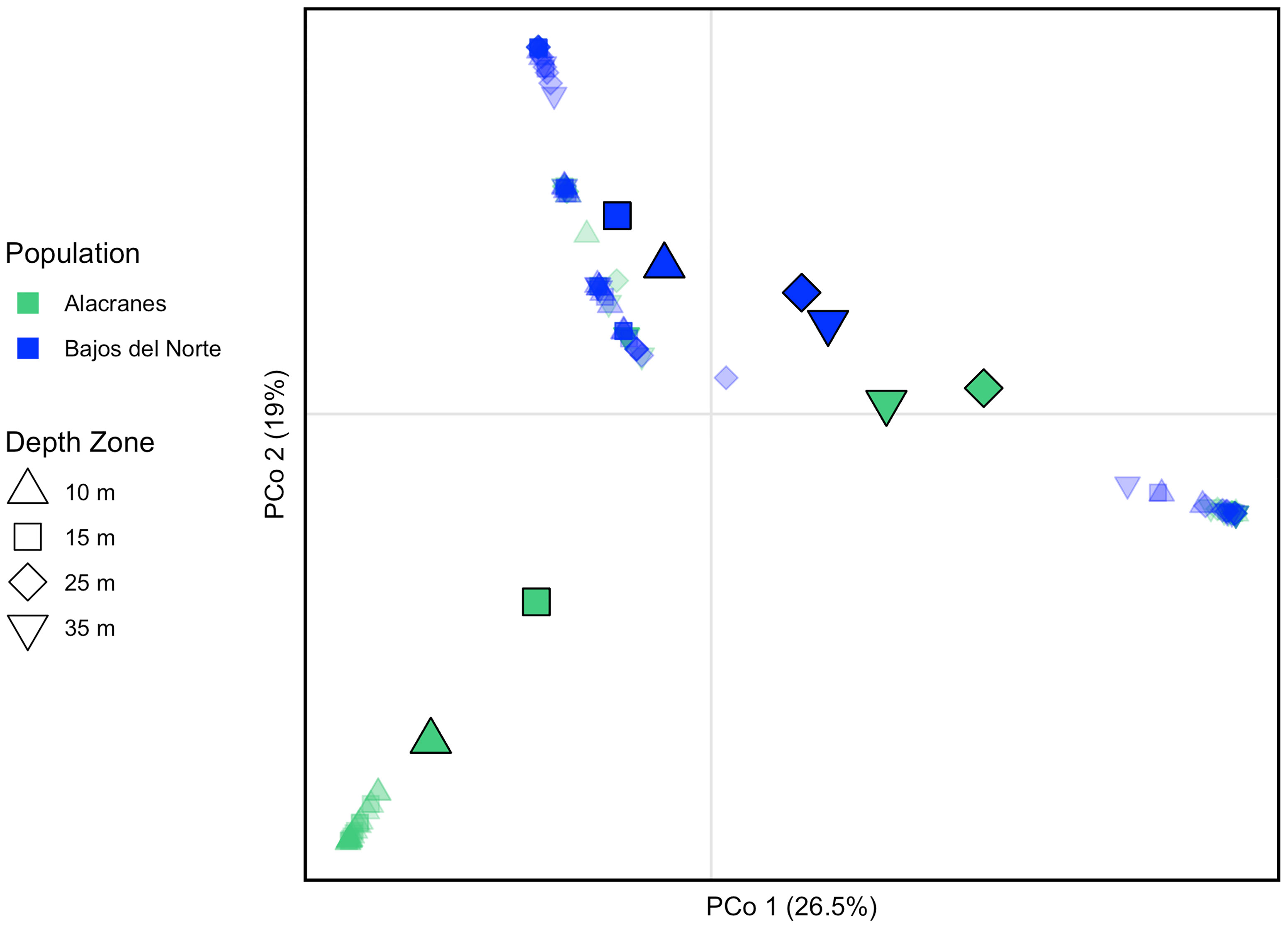
Figure 6 Principal coordinates analysis based on Bray-Curtis distance matrix among samples’ Symbiodiniaceae ITS2 type profiles. Individual samples are represented by small, transparent symbols and are jittered for visual clarity. Population centroids are indicated by larger, solid symbols. Color indicates reef and shape indicates depth zone of each sample and population centroid. The percent variation explained by each axis is indicated.
The beta diversity of ITS2 type profiles was not significantly differentiated between reefs or across depth zones. However, PERMANOVA analysis confirmed that Symbiodiniaceae communities varied significantly by reef (Pseudo-F = 3.3081, 123, p = 0.0001), depth zone (Pseudo-F = 5.8433, 123, p = 0.0001), and the interaction between reef and depth zone (Pseudo-F = 3.6273, 123, p = 0.0001). Pairwise PERMANOVA analysis indicated that there was significant differentiation between all pairwise depth zone comparisons except for between the shallowest, 10 and 15 m, depth zones (Supplementary Table S4).
Discussion
Cladocopium-Dominated Symbiodiniaceae Communities
Both the 2bRAD and the ITS2 sequencing data demonstrated that the Symbiodiniaceae communities hosted by M. cavernosa collected from Alacranes and Bajos del Norte reefs, Campeche Bank, Mexico, were dominated by the genus Cladocopium. Specifically, the sample profiles were dominated by the Cladocopium DIV C3 which is consistent with previous studies of M. cavernosa Symbiodiniaceae communities across the Northwest Gulf of Mexico, Belize, Florida Keys, Bermuda, U.S. Virgin Islands, and the Bahamas (Lesser et al., 2010; Serrano et al., 2014; Polinski and Voss, 2018; Eckert et al., 2020). While in the past the C3 ITS2 type was considered to be a generalist taxon of symbiont, it is now considered to be a highly genetically and ecologically diverse group of species lineages (LaJeunesse, 2005; Thornhill et al., 2014; Hume et al., 2015). This suggests that even though all ten of the dominant ITS2 type profiles listed C3 as their most abundant DIV, these profiles are still likely to represent unique taxa or genotypes. Surprisingly, background levels of algal symbionts from the genus Gerakladium were also identified in two samples. In general, this genus is not known to associate with scleractinian corals, but rather with excavating sponge species (Mote et al., 2021). It is possible that excavating sponge species were colonizing the M. cavernosa skeleton and perhaps small amounts may have been unintentionally collected in addition to the coral tissue. However, other studies have also identified Gerakladium at low densities in some corals and it may also occur in a free-living form in the environment (LaJeunesse et al., 2018).
Depth-Dependent Coral Genetic and Symbiodiniaceae Community Structuring Is Stronger at Alacranes Than Bajos del Norte Reef
Both the M. cavernosa corals and the in hospite Symbiodiniaceae communities demonstrated significant genetic structuring across a shallow to mesophotic depth gradient at Alacranes and Bajos del Norte reefs. However, there was location-based variation in the magnitude of this depth-dependent genetic structuring. Montastraea cavernosa from the mesophotic, 35 m population, at Alacranes were highly distanced from the rest of the samples on the IBS PCoA (Figure 2), exhibited the highest within-reef pairwise FST values (0.054–0.074, Figure 3), and tended to be dominated by the yellow, depth-specialist genetic cluster in the admixture plot (Figure 4). In addition, high levels of depth-dependent genetic structuring were observed across the Symbiodiniaceae communities at Alacranes, with the presence of a putative shallow-specialist ITS2 type profile at the 10 and 15 m depth zones (dark orange profile, Figure 5) and putative mesophotic-specialist ITS2 type profiles at the 35 m depth zone (light and dark purple profiles, Figure 5). Depth-dependent structuring across coral host populations at Bajos del Norte reef, was present but much weaker when compared to Alacranes reef. The Bajos del Norte-35 m population clustered much more closely to the shallow samples in the IBS PCoA than the Alacranes-35 m population (Figure 2), the within reef pairwise FST values were much lower (0.008–0.022, Figure 3), and only a few samples were dominated by the putative depth-specialist genetic cluster in the admixture plots (Figure 4). There were also fewer ITS2 type profiles endemic to different depth zones across Bajos del Norte but certain profile types (e.g. neon green and dark orange, Figure 5) were more prevalent at either the 10 and 15 m or 25 and 35 m populations.
The difference in the level of depth-dependent genetic structuring of the coral host and algal symbionts between Alacranes and Bajos del Norte may be associated with the distinct geomorphological features of each of these reefs. Alacranes is an emergent, semi-circular bank-atoll reef, forming a semi-enclosed lagoon and a series of small islands (Chávez et al., 2007). The northern and western sides of the reef are characterized by steeply sloping wall structures that rise quickly from their bases at 40 m to about 15 m depth (Bello-Pineda et al., 2005; Quesada et al., 2006). Alacranes’ eastern side exhibits a barrier reef formation with patch reefs along the reef flat and crest between 8–15 m and then a steep, wall-like forereef slope extending past 20 m. In contrast, Bajos del Norte consists of a series of submerged, carbonate capped mounts and ridges that range, at their shallowest, between 5–20 m but slope down to depths of 70 m in between coral peaks (Tuz-Sulub et al., 2003; Tuz-Sulub and Brulé, 2015).
Other studies have also hypothesized a link between reef geomorphology and observed patterns of depth-dependent coral and symbiont genetic structuring, or lack thereof, and represent interesting comparisons to reefs on Campeche Bank. For example, the Belize Barrier Reef and nearby Glover’s reef atoll exhibit similar geomorphological characteristics to Alacranes, notably a steeply sloped forereef. Significant levels of genetic differentiation were identified between samples above the fore-reef slope at 10 and 16 m and samples on the fore-reef slope at 25 and 35 m (Eckert et al., 2019). In addition, multiple samples on the reef flat/crest (10 and 16 m depth zones) hosted putative shallow-specialist ITS2 type profiles not hosted by samples deeper on the fore-reef slope (25 and 35 m; Eckert et al., 2020). These results are similar to the coral and Symbiodiniaceae patterns of differentiation across Alacranes reef. Abiotic factors such as light levels and temperature may shift rapidly across this steeply sloping geomorphological gradient, and the depth-dependent structuring we observe could be potentially driven by holobiont specialization to possibly distinct environmental regimes (Lesser et al., 2010; Bongaerts et al., 2013; González et al., 2018). In this study, relatively few outlier SNP loci within annotated gene regions were identified, however, 2bRAD sequencing interrogates only a relatively small proportion of the coral’s genome limiting our ability to identify functional effects of population genetic structuring. Further whole-genome sequencing, gene expression, and physiological approaches are needed to assess if these genetic differences coincide with biological or ecological characteristics that confer potential adaptive advantages to the coral’s habitat (Studivan et al., 2019; Fuller et al., 2020; Studivan and Voss, 2020; Rippe et al., 2021). In addition, across other barrier reef systems, small-scale oceanographic currents that vary in their strength and direction across depth have been hypothesized to function as a pre-zygotic barrier to larval mixing between shallow and mesophotic coral populations further driving their genetic differentiation, this is also potentially a factor driving differentiation at Alacranes (Eckert et al., 2019).
Bajos del Norte exhibits geomorphological similarities to the coral-capped salt diapir domes of the Flower Garden Banks in the Northwest Gulf of Mexico (Schmahl et al., 2008; Tuz-Sulub and Brulé, 2015). Interestingly, the Flower Garden Banks also demonstrate a lack of strong depth-dependent differentiation between shallow and mesophotic populations of M. cavernosa and their Symbiodiniaceae communities (Polinski and Voss, 2018; Studivan and Voss, 2018a). Both Bajos del Norte and the Flower Garden Banks are also located in areas that are heavily influenced by loop current associated eddies. In the Flower Gardens, it was hypothesized that these loop current eddies promote gene flow across banks and depth zones, and similar hydrodynamics may also be driving similar mixing across depth at Bajos del Norte (Garavelli et al., 2018; Studivan and Voss, 2018a; Studivan and Voss, 2018b; Limer et al., 2020). While bathymetry data for Bajos del Norte is limited, the ridges are reported to extend to depths of 70 m (Tuz-Sulub and Brulé, 2015). However, to the best of our knowledge, there is currently no mesophotic benthic community data presently available for Bajos del Norte. If significant coral communities, including M. cavernosa populations, persist deeper into the mesophotic zone it is possible that a depth-dependent genetic “break-point” occurs at Bajos del Norte deeper than the 35 m depth zone sampled in this study (Favoretto et al., 2020). Further characterization of the geomorphology, light, temperature, and oceanographic regimes across wider depth gradients at Alacranes and Bajos del Norte is needed to assess the validity of potential drivers of depth-dependent genetic differentiation of corals and their associated Symbiodiniaceae communities, to characterize why the magnitude of the depth-dependent differentiation varies between these two reefs, and to verify if the patterns hold true deeper into the mesophotic zone.
Montastraea cavernosa Coral Exhibit Higher Levels of Horizontal Connectivity Between Reefs Than Their Symbiodiniaceae
While both M. cavernosa and their in hospite Symbiodiniaceae exhibited significant genetic structuring across depth zones (albeit to varying degrees at Alacranes vs. Bajos del Norte) there are relatively high levels of horizontal coral gene flow between Alacranes and Bajos del Norte reefs, especially at shallow depths. The majority of shallow coral samples were shown to cluster together in the PCoA regardless of reef (Figure 2), and multiple Alacranes to Bajos del Norte pairwise FST comparisons were low or non-significant values (Figure 3). Relatively high levels of horizontal connectivity across similar spatial scales have been commonly observed for this broadcast spawning species with a high pelagic larval duration (Studivan and Voss, 2018b; Eckert et al., 2019; Sturm et al., 2020).
In contrast, Symbiodiniaceae communities varied between Alacranes and Bajos del Norte even at the same depth zone (Figure 5). In the Symbiodiniaceae ITS2 type profile PCoA, samples primarily cluster together with those from the same reef along PCo2 (Figure 6), and reef was a significant factor in the structuring of Symbiodiniaceae communities. Montastraea cavernosa acquire their algal symbionts from their surrounding environment during early ontogeny (Abrego et al., 2009; Baird et al., 2009). These results suggest that the community makeup of the available environmental pools of Symbiodiniaceae may be distinct between the two reefs (Cunning et al., 2015; Quigley et al., 2017). The limited level of dispersal of these algal symbionts relative to their coral hosts may be a factor of their life history, as many Symbiodiniaceae live primarily within their sessile hosts, with a relatively short free-living stage restricted mainly to the sediment which limits potential dispersal via oceanic currents (Quigley et al., 2017; Thornhill et al., 2017; Ali et al., 2019; Davies et al., 2020). Similar studies of algal symbiont communities in both Caribbean and Pacific Acropora species also found that the coral’s Symbiodiniaceae exhibit strong patterns of genetic structuring despite a lack of genetic differentiation among the coral hosts across the same spatial scales (Baums et al., 2014; Davies et al., 2020). Some exceptions to this pattern include the light orange ITS2 type profile which was commonly identified at both Alacranes and Bajos del Norte (Figure 5). Similarly, asexual lineages of both Cladocopium and Symbiodinium species have been identified across large, multi-island/reef spatial scales on the order of thousands of kilometers, suggesting that long-range dispersal of Symbiodiniaceae may be improbable but not impossible (Baums et al., 2014; Davies et al., 2020).
Conclusions and Implications for Management
In summary, both Alacranes and Bajos del Norte demonstrated significant genetic structuring of M. cavernosa and their associated Symbiodiniaceae across a shallow to mesophotic depth gradient, but the magnitude of differentiation was much greater at Alacranes. In the case of M. cavernosa at Alacranes, the depth-dependent “genetic break” does appear to occur between 25 and 35 m, coinciding with the traditional definition of the shallow/mesophotic boundary. It is unknown if this genetic breakpoint occurs at a deeper depth at Bajos del Norte or if the pattern of relatively high levels of gene flow among shallow and mesophotic populations holds across deeper mesophotic depths at this location. Across both reefs, however, algal symbiont communities tend to shift significantly between 15 and 25 m, although this shift is less dramatic at Bajos del Norte. While we were not able to collect light profiles at depth intervals for the study locations, significant declines in light availability or shifts in spectral quality at this 15–25 m depth range may be driving the observed shifts in the algal symbiont community structure. Light penetration is one of the most significant factors in structuring photic communities including algal symbiont assemblages and local differences in light penetration may lead to genetic breakpoints that do not exactly align with the traditionally defined ~30 m shallow to mesophotic border (Tamir et al., 2019). These results have important implications for the management of Alacranes reef. The high levels of genetic differentiation between shallow and mesophotic M. cavernosa populations suggest that there may be relatively limited capacity for the mesophotic populations to re-seed shallow reefs within this region. The associated Symbiodiniaceae communities appear to be highly depth-specialized as well, and if certain coral genotypes co-evolve to establish symbiosis with certain algal symbiont types then this may further drive depth-dependent differentiation (Prada et al., 2014; Thornhill et al., 2014). Overall, shallow and mesophotic M. cavernosa populations at Alacranes may need to be managed independently, and further efforts should focus on understanding if this pattern holds across other ecologically important depth-generalist coral species. Relative to Alacranes reef, Bajos del Norte and other reef systems on Campeche Bank, receive limited management and have had little ecological characterization. The results of this study suggest that there are relatively high levels of connectivity of M. cavernosa both across depth zones within Bajos del Norte as well as to Alacranes downstream. Currents on Campeche Bank predominantly flow towards the west, highlighting Bajos del Norte as a potential source of coral larvae to not only Alacranes but other reefs on Campeche Bank and, on a regional scale, as a potential stepping stone between the Caribbean and Gulf of Mexico reef systems (Kornicker et al., 1959). Future efforts should focus on assessing shallow and mesophotic reef habitat health and their coral holobiont connectivity dynamics across Campeche Bank. A combination of increasing anthropogenic pressures on Campeche Bank including overfishing and offshore oil exploration would make Bajos del Norte and potentially other Campeche Bank reefs excellent candidates for incorporation into regional marine protected area networks in the Gulf of Mexico (Favoretto et al., 2020; Gil-Agudelo et al., 2020).
Data Availability Statement
The data has been uploaded to NCBI SRA under the following project accession: PRJNA789549 https://www.ncbi.nlm.nih.gov/bioproject/PRJNA789549/. The most up-to-date data processing, analyses, and figures are available at the following github repository: https://github.com/lexiebsturm/mxMcavSnp.
Author Contributions
AS, NS, and JV conceived the study. AS and NS organized and planned the field expedition. AS and RE conducted the fieldwork. AS, RE, and AC conducted the lab work. AS and RE conducted the data analysis and generated figures. AS and JV secured funding for this research. All authors reviewed and contributed to the final manuscript drafted by AS.
Funding
Funding for this research was awarded to JV by the NOAA Office of Ocean Exploration and Research under award NA14OAR4320260 through the Cooperative Institute for Ocean Exploration, Research, and Technology and by the NOAA National Center for Coastal Ocean Science under award NA18NOS4780166 through the Connectivity of Coral Ecosystems in the Northwest Gulf of Mexico project. Additional funding was provided to AS through a National Science Foundation Graduate Research Fellowship and scholarships from Florida Sea Grant and the Women Divers Hall of Fame.
Conflict of Interest
The authors declare that the research was conducted in the absence of any commercial or financial relationships that could be construed as a potential conflict of interest.
Publisher’s Note
All claims expressed in this article are solely those of the authors and do not necessarily represent those of their affiliated organizations, or those of the publisher, the editors and the reviewers. Any product that may be evaluated in this article, or claim that may be made by its manufacturer, is not guaranteed or endorsed by the publisher.
Acknowledgments
We thank Diana Ugalde and members of the Biodiversidad Marina de Yucatán research group for assisting in the organization and execution of this field expedition. We thank Manuel Victoria Muguira and the crew of the M/V Caribbean Kraken for scuba diving and field support. All samples were collected under Comisión Nacional de Acuacultura y Pesca (CONAPESCA) permit PPF/DGOPA-064/21. Sequencing was conducted at the University of Texas at Austin’s Genome and Sequencing Analysis Facility, B. Hume conducted SymPortal analysis remotely, and FAU High Performance Computing provided computational support for bioinformatic analyses. This is contribution 2310 from Harbor Branch Oceanographic Institute.
Supplementary Material
The Supplementary Material for this article can be found online at: https://www.frontiersin.org/articles/10.3389/fmars.2022.835789/full#supplementary-material
References
Abrego D., Van Oppen M. J. H., Willis B. L. (2009). Highly Infectious Symbiont Dominates Initial Uptake in Coral Juveniles. Mol. Ecol. 18, 3518–3531. doi: 10.1111/j.1365-294X.2009.04275.x
Alexander D. H. D., Novembre J., Lange K. (2009). Fast Model-Based Estimation of Ancestry in Unrelated Individuals. Genome Res. 19, 1655–1664. doi: 10.1101/gr.094052.109
Ali A., Kriefall N. G., Emery L. E., Kenkel C. D., Matz M. V., and Davies S. W. (2019). Recruit Symbiosis Establishment and Symbiodiniaceae Composition Influenced by Adult Corals and Reef Sediment. Coral Reefs 38, 405–415.doi: 10.1007/s00338-019-01790-z
Aranda M., Li Y., Liew Y. J., Baumgarten S., Simakov O., Wilson M. C., et al. (2016). Genomes of Coral Dinoflagellate Symbionts Highlight Evolutionary Adaptations Conducive to a Symbiotic Lifestyle. Sci. Rep. 6, 1–15. doi: 10.1038/srep39734
Arbizu M. P. (2020). Pairwiseadonis: Pairwise Multilevel Comparison Using Adonis. R package version 0.4.
Armstrong R. A., Pizarro O., Roman C. (2019). “Underwater Robotic Technology for Imaging Mesophotic Coral Ecosystems,” in Mesophotic Coral Ecosystems. Eds. Loya Y., Puglise K. A., Bridge T. C. L. (Cham: Springer), 973–988. doi: 10.1007/978-3-319-92735-0_51
Baird A. H., Guest J. R., Willis B. L. (2009). Systematic and Biogeographical Patterns in the Reproductive Biology of Scleractinian Corals. Annu. Rev. Ecol. Evol. Syst. 40, 551–571. doi: 10.1146/annurev.ecolsys.110308.120220
Bak R. P. M., Nieuwland G., Meesters E. H. (2005). Coral Reef Crisis in Deep and Shallow Reefs: 30 Years of Constancy and Change in Reefs of Curacao and Bonaire. Coral Reefs. 24, 475–479. doi: 10.1007/s00338-005-0009-1
Baums I. B., Devlin-Durante M. K., Lajeunesse T. C. (2014). New Insights Into the Dynamics Between Reef Corals and Their Associated Dinoflagellate Endosymbionts From Population Genetic Studies. Mol. Ecol. 23, 4203–4215. doi: 10.1111/mec.12788
Bello-Pineda J., Liceaga-Correa M. A., Hernández-Núñez H., Ponce-Hernández R. (2005). Using Aerial Video to Train the Supervised Classification of Landsat TM Imagery for Coral Reef Habitats Mapping. Environ. Monit. Assess. 105, 145–164. doi: 10.1007/s10661-005-3499-y
Bongaerts P., Frade P. R., Hay K. B., Englebert N., Latijnhouwers K. R. W., Bak R. P. M., et al. (2015). Deep Down on a Caribbean Reef: Lower Mesophotic Depths Harbor a Specialized Coral-Endosymbiont Community. Sci. Rep. 5, 10.1038/srep07652. doi: 10.1038/srep07652
Bongaerts P., Frade P. R., Ogier J. J., Hay K. B., Van Bleijswijk J., Englebert N., et al. (2013). Sharing the Slope: Depth Partitioning of Agariciid Corals and Associated Symbiodinium Across Shallow and Mesophotic Habitats (2-60 M) on a Caribbean Reef. BMC Evol. Biol. 13, 205. doi: 10.1186/1471-2148-13-205
Bongaerts P., Ridgway T., Sampayo E. M., Hoegh-Guldberg O. (2010a). Assessing the ‘Deep Reef Refugia’ Hypothesis: Focus on Caribbean Reefs. Coral Reefs. 29, 309–327. doi: 10.1007/s00338-009-0581-x
Bongaerts P., Riginos C., Brunner R., Englebert N., Smith S. R., Hoegh-Guldberg O. (2017). Deep Reefs are Not Universal Refuges: Reseeding Potential Varies Among Coral Species. Sci. Adv. 3, e1602373. doi: 10.1126/sciadv.1602373
Bongaerts P., Riginos C., Ridgway T., Sampayo E. M., van Oppen M. J. H., Englebert N., et al. (2010b). Genetic Divergence Across Habitats in the Widespread Coral Seriatopora Hystrix and its Associated Symbiodinium. PloS One 5, e10871. doi: 10.1371/journal.pone.0010871
Bongaerts P., Sampayo E. M., Bridge T. C. L., Ridgway T., Vermeulen F., Englebert N., et al. (2011). Symbiodinium Diversity in Mesophotic Coral Communities on the Great Barrier Reef: A First Assessment. Mar. Ecol. Prog. Ser. 439, 117–126. doi: 10.3354/meps09315
Bongaerts P., Smith T. B. (2019). “Beyond the ‘Deep Reef Refuge’ Hypothesis: A Conceptual Framework to Characterize Persistence at Depth,” in Mesophotic Coral Ecosystems. Eds. Loya Y., Puglise K. A., Bridge T. C. L. (Cham: Springer International Publishing), 881–895. doi: 10.1007/978-3-319-92735-0_45
Bradbury I. R., Hamilton L. C., Dempson B., Robertson M. J., Bourret V., Bernatchez L., et al. (2015). Transatlantic Secondary Contact in Atlantic Salmon, Comparing Microsatellites, a Single Nucleotide Polymorphism Array and Restriction-Site Associated DNA Sequencing for the Resolution of Complex Spatial Structure. Mol. Ecol. 24, 5130–5144. doi: 10.1111/mec.13395
Camacho C., Coulouris G., Avagyan V., Ma N., Papadopoulos J., Bealer K., et al. (2009). BLAST+: Architecture and Applications. BMC Bioinf. 10, 421. doi: 10.1186/1471-2105-10-421
Chávez E. A., Tunnell J. W. Jr., Withers K. (2007). “Coral Reef Zonation and Ecology: Veracruz Shelf and Campeche Bank,” in Coral Reefs of the Southern Gulf of Mexico, 88–129. Published by Texas A&M University Press, College Station, Texas.
Cunning R., Yost D. M., Guarinello M. L., Putnam H. M., Gates R. D. (2015). Variability of Symbiodinium Communities in Waters, Sediments, and Corals of Thermally Distinct Reef Pools in American Samoa. PloS One 10, e0145099. doi: 10.1371/journal.pone.0145099
Davies S. W., Moreland K. N., Wham D. C., Kanke M. R., Matz M. V. (2020). Cladocopium Community Divergence in Two Acropora Coral Hosts Across Multiple Spatial Scales. Mol. Ecol. 29, 4559–4572. doi: 10.1111/mec.15668
Dodge D. L., Studivan M. S., Eckert R. J., Chei E., Beal J., Voss J. D. (2020). Population Structure of the Scleractinian Coral Montastraea cavernosa in Southeast Florida. Bull. Mar. Sci. 96, 767–782. doi: 10.5343/bms.2019.0074
Drury C., Pérez Portela R., Serrano X. M., Oleksiak M., Baker A. C. (2020). Fine-Scale Structure Among Mesophotic Populations of the Great Star Coral Montastraea cavernosa Revealed by SNP Genotyping. Ecol. Evol. 10, 6009–6019. doi: 10.1002/ece3.6340
Eckert R. J. (2020). RyanEckert/Belize_Mcav_Symbiodiniaceae_ITS2: Depth Influences Symbiodiniaceae Associations Among Montastraea cavernosa Corals on the Belize Barrier Reef. doi: 10.5281/zenodo.3738970
Eckert R. J., Reaume A. M., Sturm A. B., Studivan M. S., Voss J. D. (2020). Depth Influences Symbiodiniaceae Associations Among Montastraea cavernosa Corals on the Belize Barrier Reef. Front. Microbiol. 11. doi: 10.3389/fmicb.2020.00518
Eckert R. J., Studivan M. S., Voss J. D. (2019). Populations of the Coral Species Montastraea cavernosa on the Belize Barrier Reef Lack Vertical Connectivity. Sci. Rep. 9, 7200. doi: 10.1038/s41598-019-43479-x
Eren A. M., Morrison H. G., Lescault P. J., Reveillaud J., Vineis J. H., Sogin M. L. (2015). Minimum Entropy Decomposition: Unsupervised Oligotyping for Sensitive Partitioning of High-Throughput Marker Gene Sequences. ISME J. 9, 968–979. doi: 10.1038/ismej.2014.195
Evanno G., Regnaut S., Goudet J. (2005). Detecting the Number of Clusters of Individuals Using the Software STRUCTURE: A Simulation Study. Mol. Ecol. 14, 2611–2620. doi: 10.1111/j.1365-294X.2005.02553.x
Favoretto F., Mascareñas-Osorio I., León-Deniz L., González-Salas C., Pérez-España H., Rivera-Higueras M., et al. (2020). Being Isolated and Protected is Better Than Just Being Isolated: A Case Study From the Alacranes Reef, Mexico. Front. Mar. Sci. 7. doi: 10.3389/fmars.2020.583056
Foll M., Gaggiotti O. (2008). A Genome-Scan Method to Identify Selected Loci Appropriate for Both Dominant and Codominant Markers: A Bayesian Perspective. Genetics 180, 977–993. doi: 10.1534/genetics.108.092221
Fujiwara Y., Kawamura I., Reimer J. D., Parkinson J. E. (2021). Zoantharian Endosymbiont Community Dynamics During a Stress Event. Front. Microbiol. 12. doi: 10.3389/fmicb.2021.674026
Fuller Z. L., Mocellin V. J. L., Morris L. A., Cantin N., Shepherd J., Sarre L., et al. (2020). Population Genetics of the Coral Acropora millepora: Toward Genomic Prediction of Bleaching. Science 369. doi: 10.1126/science.aba4674
Garavelli L., Studivan M. S., Voss J. D., Kuba A., Figueiredo J., Chérubin L. M. (2018). Assessment of Mesophotic Coral Ecosystem Connectivity for Proposed Expansion of a Marine Sanctuary in the Northwest Gulf of Mexico: Larval Dynamics. Front. Mar. Sci. 5. doi: 10.3389/fmars.2018.00174
Gil-Agudelo D. L., Cintra-Buenrostro C. E., Brenner J., González-Díaz P., Kiene W., Lustic C., et al. (2020). Coral Reefs in the Gulf of Mexico Large Marine Ecosystem: Conservation Status, Challenges, and Opportunities. Front. Mar. Sci. 6. doi: 10.3389/fmars.2019.00807
Glynn P. W. (1996). Coral Reef Bleaching: Facts, Hypotheses and Implications. Glob. Change Biol. 2, 495–509. doi: 10.1111/j.1365-2486.1996.tb00063.x
González A. M., Prada C. A., Ávila V., Medina M. (2018). “Ecological Speciation in Corals,” in Population Genomics: Marine Organisms. Eds. Oleksiak M. F., Rajora O. P. (Springer International Publishing), 303–324. doi: 10.1007/13836_2018_35
Gordon A., Hannon G. (2010) FASTX-Toolkit. FASTQ/A Short-Reads Pre-Processing Tools. Available at: http://hannonlab.cshl.edu/fastx_toolkit/.
Hartmann A. C., Baird A. H., Knowlton N., Huang D. (2017). The Paradox of Environmental Symbiont Acquisition in Obligate Mutualisms. Curr. Biol. 27, 3711–3716.e3. doi: 10.1016/j.cub.2017.10.036
Holstein D. M., Paris C. B., Vaz A. C., Smith T. B. (2016). Modeling Vertical Coral Connectivity and Mesophotic Refugia. Coral Reefs. 35, 23–37. doi: 10.1007/s00338-015-1339-2
Holstein D. M., Smith T. B., Gyory J., Paris C. B. (2015). Fertile Fathoms: Deep Reproductive Refugia for Threatened Shallow Corals. Sci. Rep. 5, 1–12. doi: 10.1038/srep12407
Hume B. C. C., D’Angelo C., Smith E. G., Stevens J. R., Burt J., Wiedenmann J. (2015). Symbiodinium thermophilum Sp. Nov., a Thermotolerant Symbiotic Alga Prevalent in Corals of the World’s Hottest Sea, the Persian/Arabian Gulf. Sci. Rep. 5, 1–8. doi: 10.1038/srep08562
Hume B. C. C., Smith E. G., Ziegler M., Warrington H. J. M., Burt J. A., LaJeunesse T. C., et al. (2019). SymPortal: A Novel Analytical Framework and Platform for Coral Algal Symbiont Next-Generation Sequencing ITS2 Profiling. Mol. Ecol. Resour. 19, 1063–1080. doi: 10.1111/1755-0998.13004
Hume B. C. C., Ziegler M., Poulain J., Pochon X., Romac S., Boissin E., et al. (2018). An Improved Primer Set and Amplification Protocol With Increased Specificity and Sensitivity Targeting the Symbiodinium ITS2 Region. PeerJ. doi: 10.7717/peerj.4816
Jombart T., Ahmed I. (2011). Adegenet 1.3-1: New Tools for the Analysis of Genome-Wide SNP Data. Bioinformatics 27, 3070–3071. doi: 10.1093/bioinformatics/btr521
Jombart T., Devillard S., Balloux F., Falush D., Stephens M., Pritchard J., et al. (2010). Discriminant Analysis of Principal Components: A New Method for the Analysis of Genetically Structured Populations. BMC Genet. 11, 94. doi: 10.1186/1471-2156-11-94
Kamvar Z. N., Tabima J. F., Gr̈unwald N. J. (2014). Poppr: An R Package for Genetic Analysis of Populations With Clonal, Partially Clonal, and/or Sexual Reproduction. PeerJ 2014, 1–14. doi: 10.7717/peerj.281
Kahng S., Copus J. M., Wagner D. (2017). Mesophotic Coral Ecosystems. Marine Animal Forests Springer International Publishing, Cham, 185–206. doi: 10.1007/978-3-319-21012-4_4
Klepac C. N., Beal J., Kenkel C. D., Sproles A., Polinski J. M., Williams M. A., et al. (2015). Seasonal Stability of Coral-Symbiodinium Associations in the Subtropical Coral Habitat of St. Lucie Reef, Florida. Mar. Ecol. Prog. Ser. 532, 137–151. doi: 10.3354/meps11369
Kopelman N. M., Mayzel J., Jakobsson M., Rosenberg N. A., Mayrose I. (2015). Clumpak: A Program for Identifying Clustering Modes and Packaging Population Structure Inferences Across K. Mol. Ecol. Resour. 15, 1179–1191. doi: 10.1111/1755-0998.12387
Korneliussen T. S., Albrechtsen A., Nielsen R. (2014). ANGSD: Analysis of Next Generation Sequencing Data. BMC Bioinf. 15, 356. doi: 10.1186/s12859-014-0356-4
Kornicker L. S., Bonet F., Cann R., Hoskin C. M. (1959). Alacran Reef, Campeche Bank, Mexico. Publ. Inst. Mar. Sci. 6, 1–22.
LaJeunesse T. C. (2005). “Species” Radiations of Symbiotic Dinoflagellates in the Atlantic and Indo-Pacific Since the Miocene-Pliocene Transition. Mol. Biol. Evol. 22, 570–581. doi: 10.1093/molbev/msi042
LaJeunesse T. C., Parkinson J. E., Gabrielson P. W., Jeong H. J., Reimer J. D., Voolstra C. R., et al. (2018). Systematic Revision of Symbiodiniaceae Highlights the Antiquity and Diversity of Coral Endosymbionts. Curr. Biol. 28, 1–11. doi: 10.1016/j.cub.2018.07.008
Langmead B., Salzberg S. L. (2012). Fast Gapped-Read Alignment With Bowtie 2. Nat. Methods 9, 357–359. doi: 10.1038/nmeth.1923
Laverick J. H., Piango S., Andradi-Brown D. A., Exton D. A., Bongaerts P., Bridge T. C. L., et al. (2018). To What Extent do Mesophotic Coral Ecosystems and Shallow Reefs Share Species of Conservation Interest? A Systematic Review. Environ. Evid. 7, 1–8. doi: 10.1186/s13750-018-0127-1
Lesser M. P., Marc S., Michael S., Michiko O., Gates R. D., Andrea G. (2010). Photoacclimatization by the Coral Montastraea cavernosa in the Mesophotic Zone: Light, Food, and Genetics. Ecology 91, 990–1003. doi: 10.1890/09-0313.1
Lesser M. P., Slattery M., Leichter J. J. (2009). Ecology of Mesophotic Coral Reefs. J. Exp. Mar. Bio. Ecol. 375, 1–8. doi: 10.1016/j.jembe.2009.05.009
Lesser M. P., Slattery M., Mobley C. D. (2018). Biodiversity and Functional Ecology of Mesophotic Coral Reefs. Annu. Rev. Ecol. Evol. Syst. 49, 49–71. doi: 10.1146/annurev-ecolsys-110617-062423
Levitan D. R., Fukami H., Jara J., Kline D., McGovern T. M., McGhee K. E., et al. (2004). Mechanisms of Reproductive Isolation Among Sympatric Broadcast-Spawning Corals of the Montastraea annularis Species Complex. Evol. (N. Y). 58, 308–323. doi: 10.1111/j.0014-3820.2004.tb01647.x
Li Y.-L., Liu J.-X. (2018). StructureSelector: A Web-Based Software to Select and Visualize the Optimal Number of Clusters Using Multiple Methods. Mol. Ecol. Resour. 18, 176–177. doi: 10.1111/1755-0998.12719
Limer B. D., Bloomberg J., Holstein D. M. (2020). The Influence of Eddies on Coral Larval Retention in the Flower Garden Banks. Front. Mar. Sci. 7. doi: 10.3389/fmars.2020.00372
Liu H., Stephens T. G., González-Pech R. A., Beltran V. H., Lapeyre B., Bongaerts P., et al. (2018). Symbiodinium Genomes Reveal Adaptive Evolution of Functions Related to Coral-Dinoflagellate Symbiosis. Commun. Biol. 1, 95. doi: 10.1038/s42003-018-0098-3
Mote S., Gupta V., De K., Hussain A., More K., Nanajkar M., et al. (2021). Differential Symbiodiniaceae Association With Coral and Coral-Eroding Sponge in a Bleaching Impacted Marginal Coral Reef Environment. Front. Mar. Sci. 8. doi: 10.3389/fmars.2021.666825
Oksanen J., F. Guillaume Blanchet R. K., Legendre P., Minchin P. R., O’Hara R. B., Simpson G. L., et al. (2019). Vegan: Community Ecology Package R package version 2.5-6. https://CRAN.R-project.org/package=vegan.
Pembleton L. W., Cogan N. O. I., Forster J. W. (2013). StAMPP: An R Package for Calculation of Genetic Differentiation and Structure of Mixed-Ploidy Level Populations. Mol. Ecol. Resour. 13, 946–952. doi: 10.1111/1755-0998.12129
Polinski J. M., Voss J. D. (2018). Evidence of Photoacclimatization at Mesophotic Depths in the Coral-Symbiodinium Symbiosis at Flower Garden Banks National Marine Sanctuary and McGrail Bank. Coral Reefs. 37, 779–789. doi: 10.1007/s00338-018-1701-2
Prada C., McIlroy S. E., Beltrán D. M., Valint D. J., Ford S. A., Hellberg M. E., et al. (2014). Cryptic Diversity Hides Host and Habitat Specialization in a Gorgonian-Algal Symbiosis. Mol. Ecol. 23, 3330–3340. doi: 10.1111/mec.12808
Puechmaille S. J. (2016). The Program Structure Does Not Reliably Recover the Correct Population Structure When Sampling is Uneven: Subsampling and New Estimators Alleviate the Problem. Mol. Ecol. Resour. 16, 608–627. doi: 10.1111/1755-0998.12512
Puritz J. B., Matz M. V., Toonen R. J., Weber J. N., Bolnick D. I., Bird C. E. (2014). Demystifying the RAD Fad. Mol. Ecol. 23, 5937–5942. doi: 10.1111/mec.12965
Pyle R. L. (2019). “Advanced Technical Diving,” in Mesophotic Coral Ecosystems. Eds. Loya Y., Puglise K. A., Bridge T. C. L. (Cham: Springer), 959–972. doi: 10.1007/978-3-319-92735-0_50
Quesada V. F., Luege Tamargo J. L., Patrón Laviada P. J., Enkerlin Hoeflich E., Gutiérrez Carbonell D., Arellano Guillermo A., et al. (2006). Programa De Conservación Y Manejo Parque Nacional Arrecife Alacranes.
Quigley K. M., Bay L. K., Willis B. L. (2017). Temperature and Water Quality-Related Patterns in Sediment-Associated Symbiodinium Communities Impact Symbiont Uptake and Fitness of Juveniles in the Genus Acropora. Front. Mar. Sci. 4. doi: 10.3389/fmars.2017.00401
Reed J. K. (1985). Deepest Distribution of Atlantic Hermaptypic Corals Discovered in the Bahamas. Proc. Fifth Int. Coral Reef. Congr. 6, 249–254.
Reed J. K., González-Díaz P., Busutil L., Farrington S., Martínez-Daranas B., Cobián Rojas D., et al. (2018). Cuba’s Mesophotic Coral Reefs and Associated Fish Communities. Rev. Investig. Mar. 38, 56–125.
Rippe J. P., Dixon G., Fuller Z. L., Liao Y., Matz M. (2021). Environmental Specialization and Cryptic Genetic Divergence in Two Massive Coral Species From the Florida Keys Reef Tract. Mol. Ecol. 30, mec.15931. doi: 10.1111/mec.15931
Rocha L. A., Pinheiro H. T., Shepherd B., Papastamatiou Y. P., Luiz O. J., Pyle R. L., et al. (2018). Mesophotic Coral Ecosystems are Threatened and Ecologically Distinct From Shallow Water Reefs. Sci. 361, 281–284. doi: 10.1126/science.aaq1614
Sanvicente-Añorve L., Zavala-Hidalgo J., Allende-Arandía M. E., Hermoso-Salazar M. (2014). Connectivity Patterns Among Coral Reef Systems in the Southern Gulf of Mexico. Mar. Ecol. Prog. Ser. 498, 27–41. doi: 10.3354/meps10631
Schloss P. D., Westcott S. L., Ryabin T., Hall J. R., Hartmann M., Hollister E. B., et al. (2009). Introducing Mothur: Open-Source, Platform-Independent, Community-Supported Software for Describing and Comparing Microbial Communities. Appl. Environ. Microbiol. 75, 7537–7541. doi: 10.1128/AEM.01541-09
Schmahl G. P., Hickerson E. L., Precht W. F. (2008). “Biology and Ecology of Coral Reefs and Coral Communities in the Flower Garden Banks Region, Northwestern Gulf of Mexico,” in Coral Reefs of the USA. Eds. Riegl B. M., Dodge R. E. (Dordrecht: Springer Netherlands), 221–261. doi: 10.1007/978-1-4020-6847-8_6
Selkoe K. A., Toonen R. J. (2011). Marine Connectivity: A New Look at Pelagic Larval Duration and Genetic Metrics of Dispersal. Mar. Ecol. Prog. Ser. 436, 291–305. doi: 10.3354/meps09238
Serrano X., Baums I. B., O’Reilly K., Smith T. B., Jones R. J., Shearer T. L., et al. (2014). Geographic Differences in Vertical Connectivity in the Caribbean Coral Montastraea cavernosa Despite High Levels of Horizontal Connectivity at Shallow Depths. Mol. Ecol. 23, 4226–4240. doi: 10.1111/mec.12861
Shlesinger T., Loya Y. (2021). Depth-Dependent Parental Effects Create Invisible Barriers to Coral Dispersal. Commun. Biol. 4, 1–10. doi: 10.1038/s42003-021-01727-9
Shoguchi E., Beedessee G., Hisata K., Tada I., Narisoko H., Satoh N., et al. (2021). A New Dinoflagellate Genome Illuminates a Conserved Gene Cluster Involved in Sunscreen Biosynthesis. Genome Biol. Evol. 13, 1–7. doi: 10.1093/gbe/evaa235
Shoguchi E., Shinzato C., Kawashima T., Gyoja F., Mungpakdee S., Koyanagi R., et al. (2013). Draft Assembly of the Symbiodinium minutum Nuclear Genome Reveals Dinoflagellate Gene Structure. Curr. Biol. 23, 1399–1408. doi: 10.1016/j.cub.2013.05.062
Skotte L., Korneliussen T. S., Albrechtsen A. (2013). Estimating Individual Admixture Proportions From Next Generation Sequencing Data. Genetics 195, 693–702. doi: 10.1534/genetics.113.154138
Slattery M., Lesser M. P., Brazeau D., Stokes M. D., Leichter J. J. (2011). Connectivity and Stability of Mesophotic Coral Reefs. J. Exp. Mar. Bio. Ecol. 408, 32–41. doi: 10.1016/j.jembe.2011.07.024
Smith T. B., Glynn P. W., Maté J. L., Toth L. T., Gyory J. (2014). A Depth Refugium From Catastrophic Coral Bleaching Prevents Regional Extinction. Ecology 95, 1663–1673. doi: 10.1890/13-0468.1
Smith T. B., Gyory J., Brandt M. E., Miller W. J., Jossart J., Nemeth R. S. (2016). Caribbean Mesophotic Coral Ecosystems are Unlikely Climate Change Refugia. Glob. Change Biol. 22, 2756–2765. doi: 10.1111/gcb.13175
Studivan M. S., Milstein G., Voss J. D. (2019). Montastraea cavernosa Corallite Structure Demonstrates Distinct Morphotypes Across Shallow and Mesophotic Depth Zones in the Gulf of Mexico. PloS One 14, 1–21. doi: 10.1371/journal.pone.0203732
Studivan M. S., Voss J. D. (2018a). Assessment of Mesophotic Coral Ecosystem Connectivity for Proposed Expansion of a Marine Sanctuary in the Northwest Gulf of Mexico: Population Genetics. Front. Mar. Sci. doi. doi: 10.3389/fmars.2018.00152
Studivan M. S., Voss J. D. (2018b). Population Connectivity Among Shallow and Mesophotic Montastraea cavernosa Corals in the Gulf of Mexico Identifies Potential for Refugia. Coral Reefs. 37, 1183–1196. doi: 10.1007/s00338-018-1733-7
Studivan M. S., Voss J. D. (2020). Transcriptomic Plasticity of Mesophotic Corals Among Natural Populations and Transplants of Montastraea cavernosa in the Gulf of Mexico and Belize. Mol. Ecol. 29, 2399–2415. doi: 10.1111/mec.15495
Sturm A. B. (2020). Lexiebsturm/Mcavdispersionbufferextraction: Montastraea cavernosa DNA Extraction With Dispersion Buffer. doi: 10.5281/zenodo.3836665
Sturm A. B., Eckert R. J., Carreiro A. M., Voss J. D. (2021). Population Genetic Structure of the Broadcast Spawning Coral, Montastraea cavernosa, Demonstrates Refugia Potential of Upper Mesophotic Populations in the Florida Keys. Coral Reefs. doi: 10.1007/s00338-021-02112-y
Sturm A. B., Eckert R. J., González Méndez, Juliett González-Díaz P., Voss J. D. (2020). Population Genetic Structure of the Great Star Coral Montastraea cavernosa, Across the Cuban Archipelago With Comparisons Between Microsatellite and SNP Markers. Sci. Rep. 10. doi: 10.1038/s41598-020-72112-5
Szmant A. M. (1991). Sexual Reproduction by the Caribbean Reef Corals Montastrea annularis and M. cavernosa. Mar. Ecol. Prog. Ser. 74, 13–25. doi: 10.3354/meps074013
Tamir R., Eyal G., Kramer N., Laverick J. H., Loya Y. (2019). Light Environment Drives the Shallow-to-Mesophotic Coral Community Transition. Ecosphere 10. doi: 10.1002/ecs2.2839
Thornhill D. J., Howells E. J., Wham D. C., Steury T. D., Santos S. R. (2017). Population Genetics of Reef Coral Endosymbionts (Symbiodinium, Dinophyceae). Mol. Ecol. 26, 2640–2659. doi: 10.1111/mec.14055
Thornhill D. J., LaJeunesse T. C., Santos S. R. (2007). Measuring rDNA Diversity in Eukaryotic Microbial Systems: How Intragenomic Variation, Pseudogenes, and PCR Artifacts Confound Biodiversity Estimates. Mol. Ecol. 16, 5326–5340. doi: 10.1111/j.1365-294X.2007.03576.x
Thornhill D. J., Lewis A. M., Wham D. C., Lajeunesse T. C. (2014). Host-Specialist Lineages Dominate the Adaptive Radiation of Reef Coral Endosymbionts. Evol. (N. Y). 68, 352–367. doi: 10.1111/evo.12270
Tuz-Sulub A., Brulé T. (2015). Spawning Aggregations of Three Protogynous Groupers in the Southern Gulf of Mexico. J. Fish Biol. 86, 162–185. doi: 10.1111/jfb.12555
Tuz-Sulub A., Cervera-Cervera K., Colas-Marrufo T., Brule T. (2003). Primeros Indicios Sobre La Formacion De Agregaciones De Reproduccion De Meros En El Banco De Campeche, Mexico, in Gulf and Caribbean Fisheries Institute. 54th 652–667.
Wang S., Meyer E., McKay J. K., Matz M. V. (2012). 2b-RAD: A Simple and Flexible Method for Genome-Wide Genotyping. Nat. Methods 9, 808–810. doi: 10.1038/nmeth.2023
Keywords: mesophotic coral ecosystems, Symbiodiniaceae, 2bRAD, ITS2, Gulf of Mexico, Alacranes, Bajos del Norte, depth-dependent structuring
Citation: Sturm AB, Eckert RJ, Carreiro AM, Simões N and Voss JD (2022) Depth-Dependent Genetic Structuring of a Depth-Generalist Coral and Its Symbiodiniaceae Algal Communities at Campeche Bank, Mexico. Front. Mar. Sci. 9:835789. doi: 10.3389/fmars.2022.835789
Received: 14 December 2021; Accepted: 29 March 2022;
Published: 17 May 2022.
Edited by:
Juan Armando Sanchez, University of Los Andes, ColombiaReviewed by:
John Everett Parkinson, University of South Florida, United StatesNikolaos V. Schizas, University of Puerto Rico at Mayagüez, Puerto Rico
Copyright © 2022 Sturm, Eckert, Carreiro, Simões and Voss. This is an open-access article distributed under the terms of the Creative Commons Attribution License (CC BY). The use, distribution or reproduction in other forums is permitted, provided the original author(s) and the copyright owner(s) are credited and that the original publication in this journal is cited, in accordance with accepted academic practice. No use, distribution or reproduction is permitted which does not comply with these terms.
*Correspondence: Alexis B. Sturm, YXN0dXJtMjAxN0BmYXUuZWR1; Joshua D. Voss, anZvc3MyQGZhdS5lZHU=