- 1State Key Laboratory of Marine Environmental Science, College of Ocean and Earth Sciences, Xiamen University, Xiamen, China
- 2College of Life Science, Ludong University, Yantai, China
- 3Co-innovation Center of Jiangsu Marine Bio-Industry Technology, Jiangsu Ocean University, Lianyungang, China
Phytoplankton are exposed to different concentrations of nutrients in different waters along with changing light levels during diurnal and seasonal cycles. We grew the coccolithophorid Gephyrocapsa oceanica semi-continuously at different nitrate levels under indoor low and outdoor high light conditions, and found that reduced nitrate availability significantly increased its production of particulate inorganic carbon (PIC), with its growth being reduced. High light treatment suppressed the growth of nitrate-limited cells and their efficiency of N assimilation by up to 63% compared to low light treatment. The combination of high light and nitrate limitation increased contents of PIC per cell due to sustained photochemical energy transfer, resulting in faster sinking rates by up to 82% in comparison with nitrate-repleted cells. Additionally, the sinking rates were positively correlated with ratios of PIC to particulate organic carbon (POC). These results imply that coccolithophores distributed in oligotrophic waters could be more effective as the ballast in aggregates, facilitating particulate organic carbon flux to deeper waters.
Introduction
Particulate organic carbon (POC) produced by phytoplankton and their grazers is sunk out the euphotic layer with particles and partly sequestered in the sediments, efficiently facilitating the absorption of atmospheric CO2 by the oceans (Falkowski et al., 1998; Boyd and Trull, 2007). Recent findings highlight the ballast effect in particles sinking, e.g., opal and CaCO3 produced by diatoms and coccolithophores can accelerate the sinking rate by increasing the specific gravity of particles during the sinking process (Armstrong et al., 2002; Klaas and Archer, 2002). For instance, when copepods were fed on coccolithophores or high-silica diatoms, the fecal pellets sank much faster than when they were fed on dinoflagellates or low-silica diatoms (Ploug et al., 2008; Liu and Wu, 2016). In addition, evidence on sediment traps has discovered that most POC fluxes are strongly correlated with amounts of CaCO3 (Klaas and Archer, 2002; Lam et al., 2011). As the main calcifying phytoplankton, coccolithophores have contributed to 20–80% of biogenic carbonate exported from the photic zone (Ziveri et al., 2007). Since the production of particulate inorganic carbon (PIC) by coccolithophores is susceptible to changes in pH, temperature, and nutrients (Feng et al., 2017; Zhang and Gao, 2021), the ballast effect is likely to be sensitive to ocean climate changes. It is known that the calcification of coccolithophores decreases under influence of ocean acidification, leading to a reduction of the CaCO3 precipitation (Riebesell et al., 2000; Raven and Crawfurd, 2012), which decreased the sinking rates of particles (Riebesell et al., 2016).
Nitrogen (N) is one of the major limiting nutrients in contemporary pelagic oceans (Moore et al., 2013). Such limitation is suggested to be exacerbated with progressive stratification of the upper mixed layer caused by ocean warming since the upward transport of nutrients from deeper layers is hindered (Boyd et al., 2008). It is well-known that N-limitation restricts phytoplankton photosynthesis and growth (Behrenfeld et al., 2006) and pressures phytoplankton cells to be more susceptive to other environmental stresses (Beardall et al., 2014; Eberlein et al., 2016; Marañón et al., 2018). In coccolithophores grown under constant light conditions, some studies showed that N deficiency decreased POC but increased PIC production (Kaffes et al., 2010; Zhang and Gao, 2021), but other works indicated that it decreased or resulted in insignificant changes in PIC content per cell (Langer et al., 2012; Feng et al., 2017). Such discrepancy demands further studies to explore the relationship of their physiology and sinking rates with environmental changes.
It has been documented that phytoplankton species grown under natural fluctuating sunlight exhibited different physiological traits compared to their growth under artificial constant light (Litchman et al., 2004; Floder and Burns, 2005; Jin et al., 2013). Consequently, influences of nutrients limitation on the cells may differ between indoor low constant and outdoor high fluctuating light conditions. Therefore, we hypothesize that coccolithophores exhibit different photo-physiological responses under constant and fluctuating light conditions to nitrate limitation, influencing the PIC production and sinking rates of the cells. To test this, we grew Gephyrocapsa oceanica, one of the major coccolith-carbonate flux contributors in the sediment-trap survey (Ziveri et al., 2007), under a range of nitrate concentrations and different light conditions to determine how its growth would be affected by the interaction of nitrate availability and light. Furthermore, by directly measuring the sinking rates of the cells grown under different nitrate conditions, we established a relationship between PIC/POC and sinking rates.
Materials and Methods
The coccolithophore G. oceanica (NIES-1318) was isolated from the East China Sea. Two types of experiments were carried out using PC bottles, and all cultures were in triplicate. Cells were grown semi-continuously by diluting the cultures every 24 or 48 h to maintain their exponential growth phase (Gao, 2021). The culture media were based on sterile seawater enriched with Aquil nutrients (Sunda et al., 2005) except that nitrate was supplied differently to obtain different concentrations.
The Indoor Cultures
Cells were grown in a plant growth chamber (Ruihua, Wuhan, China) at the photon flux densities [photosynthetically active radiation (PAR)] of 260 (HL), 115 (ML), or 50 μmol photons m–2 s–1 (LL), respectively, supplied by cool-white fluorescent tubes with a 12 light:12 dark cycle at 20°C. They were cultured under five levels of nitrate concentrations with initial values of 6, 12, 25, 50, and 100 μM in artificial seawater and diluted before the dark period every day down to 4.5 × 104 cells mL–1. This experiment was designed to (1) investigate the responses of G. oceanica to different levels of light and nitrate, (2) determine the specific nitrate concentrations for the following outdoor experiment, and (3) investigate the sinking rates of the cells grown with different nitrate availabilities (6, 12, 25, and 50 μM).
The Outdoor Cultures Under Incident Sunlight
We conducted the outdoor experiment for 16 days from 18th December 2018 to 2nd January 2019. Cultures in PC bottles were incubated in a water bath under natural sunlight at 20°C, with temperature controlled by a cooling circulator (CAP-3000, Rikakikai, Japan). Two nitrate treatments (10 and 50 μM) with the addition of nitrate in the natural seawater (4.8 μM nitrate) were set up according to the previous indoor culture test. Cells were pre-cultured under corresponding nitrate concentrations for 3 days in the plant growth chamber (115 μmol photons m–2 s–1, 20°C) before being transferred to and grown outdoor. Subsequently, they were acclimated to the solar PAR gradually according to Guan and Gao (2010). Briefly, the culture bottles were covered with three layers of neutral density screens for 2 days, then two layers for 4 days, and the bottles were subsequently exposed to full solar radiation. Since the PC bottles do not allow UV radiation (UVR) to penetrate and allow about 89% PAR to go through, the cells were exposed to about 89% incident sunlight. For the first 4 days, the cultures were diluted every 48 h. After that, dilutions were carried out every 24 h at about 17:30 with the renewed cell concentrations of about 4.5 × 104 cells mL–1.
Measurements of Cell Size, Growth Rate, and Chlorophyll a
Cell size and concentrations were measured by using a Coulter Particle Count and Size Analyser (Z2, Beckman Coulter, Indianapolis, IN, United States). The growth rate (μ) was determined as follows: μ = (lnC2 –lnC1)/(T2 –T1), where C2 and C1 represent the cell concentrations at T2 and T1 time, respectively. For Chlorophyll a (Chl a) measurements, at least 50 mL cultures were filtered on Whatman GF/F filters and extracted in absolute methanol at 4°C in the dark for 24 h. Then the absorption spectrum (400–800 nm) of the supernatant was measured using a scanning spectrophotometer (DU 800, Beckman Coulter, Indianapolis, IN, United States) after centrifuged at 6,000 × g for 10 min. Subsequently, the Chl a concentration was estimated according to Porra (Porra, 2002).
Determination of Coccosphere Size, Protoplast Size, and Coccosphere Thickness
Coccosphere and protoplast diameters were measured using the particle counter mentioned above. Protoplast size was obtained by dissolving the coccoliths with 0.1 mM HCl (Gerecht et al., 2015). In addition, the coccosphere thickness was calculated by subtracting protoplast diameters from coccosphere diameters and dividing by two.
Determination of Photochemical Parameters
All fluorescence parameters were measured using a pulse-amplitude-modulated fluorometer (Multi-color PAM, Walz, Germany). The indoor HL-cells were acclimated in the dark for 15 min to measure the maximal quantum yield of photosystem II (PSII) (Fv/Fm). The effective quantum yield of PSII (Yield) was measured at the actinic light of 250 μmol photons m–2 s–1, which was similar to the growth high light level. The maximum relative electron transport rate (rETRmax) and apparent electron transfer efficiency (α) were obtained by analyzing the rapid light curves (RLCs) according to Ralph et al. (2005).
To test the responses of LN- and HN-cells grown under fluctuating sunlight to short-time high light, their effective quantum yields were measured at 0, 2, 5, 8, 13, 18, 28, 45, and 60 min using the Multi-color PAM after exposures to a PAR level of 600 μmol photons m–2 s–1 under a solar simulator. Samples were cultured in quartz tubes covered with 395-nm cutoff film (Ultraphan UV Opak, Digefra) which allows transmission of irradiances above 395 nm. The yield was monitored for 50 min after the cells were transferred to dim light (15 μmol photons m–2 s–1) for photochemical recovery.
C and N Analysis
Particulate organic carbon (POC) and particulate organic nitrogen (PON) were determined using Elementar Vario EL cube (Vario EL cube, Elementar, Langenselbold, Germany). At least 50 mL cultures were filtered onto pre-combusted (450°C for 5 h) Whatman GF/F filters (25 mm) and frozen at −20°C until analysis. The filters for POC analysis were fumed with HCl to remove particulate inorganic carbon. And for total particulate carbon (TPC) analysis, samples were not treated with HCl. All samples were analyzed after drying. Cellular PIC content was calculated as PIC (pg cell–1) = TPC (pg cell–1)–POC (pg cell–1). The production rates of POC, PON and PIC (pg cell–1 d–1) were calculated by multiplying cellular contents by the specific growth rates μ.
Determination of Sinking Rate and Cell Density
The sinking rate of G. oceanica cells was measured according to the SETCOL method (Bienfang, 1981) in an incubator at the constant temperature of 20°C. Briefly, settling tubes (about 385 mL, 0.59 m) were vertically fixed, and samples of all treatments were filled into settling tubes after shaking, which were sealed and placed in the chamber. Meanwhile, an equal volume of tested cultures was placed in the same chamber as the control to monitor changes in cell concentrations. The sinking rates were determined after the G. oceanica cells had acclimated to the treatments for at least 9 days under indoor and for 16 days under outdoor conditions. Cell concentrations were regarded as the biomass index for the sinking rate calculations.
Cell density was calculated according to Stokes’ law: v = 2gr2 (ρ’-ρ)/9, where v the sinking rate (m s–1), g the gravitational acceleration (m s–2), r the radius of the coccosphere (m), ρ’ the cell density (kg m–3), ρ seawater’s density (kg m–3) and
is the dynamic viscosity of seawater (kg m–1 s–1).
Data Analysis
The specific growth rates at different light levels were plotted against nitrate concentrations, and were fitted to the Michaelis-Menten function (Michaelis and Menten, 1913) to analyze the relationship of growth to nitrate availability. The relationships of sinking rate vs. coccosphere diameter, cell density and PIC/POC ratio were obtained by linear fitting.
Results were presented as means ± SD for triplicate cultures for each treatment, and the data were analyzed with the software GraphPad Prism 8.0.2. The One-way ANOVA and Tukey Post hoc (Tukey HSD) test were used to test the differences between different treatments (p < 0.05).
Results
The specific growth rate (μ) of the exponentially grown G. oceanica increased with increasing nitrate concentrations, regardless of light levels (Figure 1). The μmax values of HL-cells were higher by 12 and 35% than that of ML- and LL-cells, respectively (Table 1, p < 0.01). Under the N-limited conditions, the specific growth rates were inhibited at the HL (260 μmol photons m–2 s–1) by 28% at 6 and by 3% at 12 μM nitrate compared to that grown at LL, respectively.
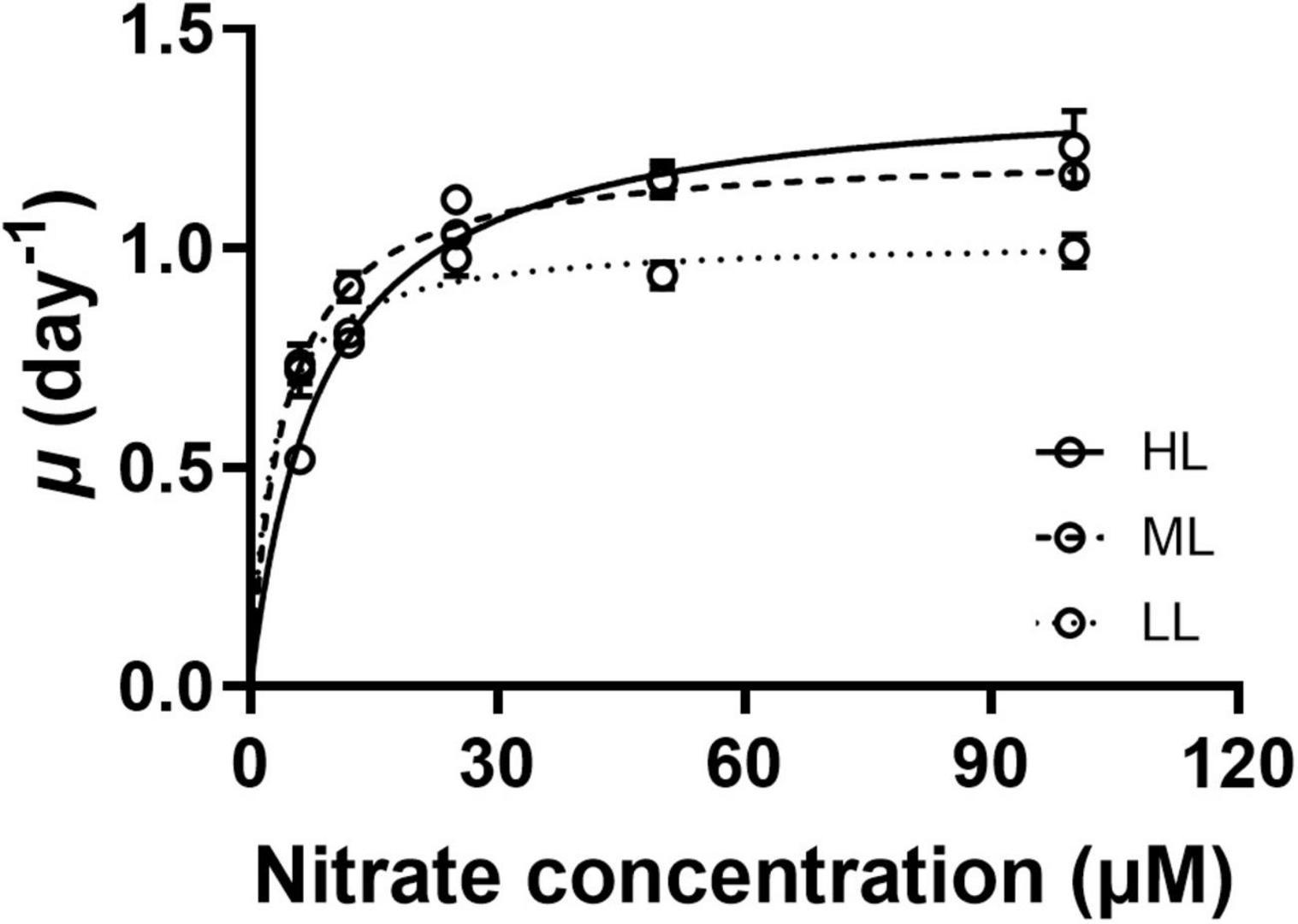
Figure 1. The specific growth rates of Gephyrocapsa oceanica as a function of nitrate concentrations (6, 12, 25, 50, and 100 μM) grown under constant light levels of 260 (HL, solid line), 115 (ML, broken line), and 50 μmol photons m– 2 s– 1 (LL, dotted line), respectively. The values are the means ± SD of triplicate cultures.

Table 1. The μmax, Km, and efficiency of N assimilation in Gephyrocapsa oceanica cells grown under different levels of photosynthetically active radiation (PAR) (LL: 50 μmol photons m–2 s–1, ML: 115 μmol photons m–2 s–1, and HL: 260 μmol photons m–2 s–1), which were derived from the relationship of growth vs. nitrate concentrations (Figure 1).
In the outdoor experiment, μ of HN-grown cells was higher than LN-cells (Figure 2B). When the growth rates were normalized to daytime mean PAR intensity, μ values increased by 75% (p < 0.01) in HN-cells and by 25% (p = 0.02) in LN-cells with daytime mean PAR increased till 270 μmol photons m–2 s–1 (Figure 2C). When the average daytime sunlight levels ranged between 270 and 500 μmol photons m–2 s–1, μ of HN-cells still increased with increased light levels (p = 0.01). However, no significant changes in μ were observed in LN-cells (p > 0.05). By fitting, the μmax and Km for light of LN-cells was 51 and 84% lower than that of HN-cells, respectively (Supplementary Figure 1). In addition, by comparing growth rates at similar nitrate concentrations between indoor and outdoor cultures, we found that μ values under constant (indoor) light regimes were higher than that under fluctuating (outdoor) sunlight.
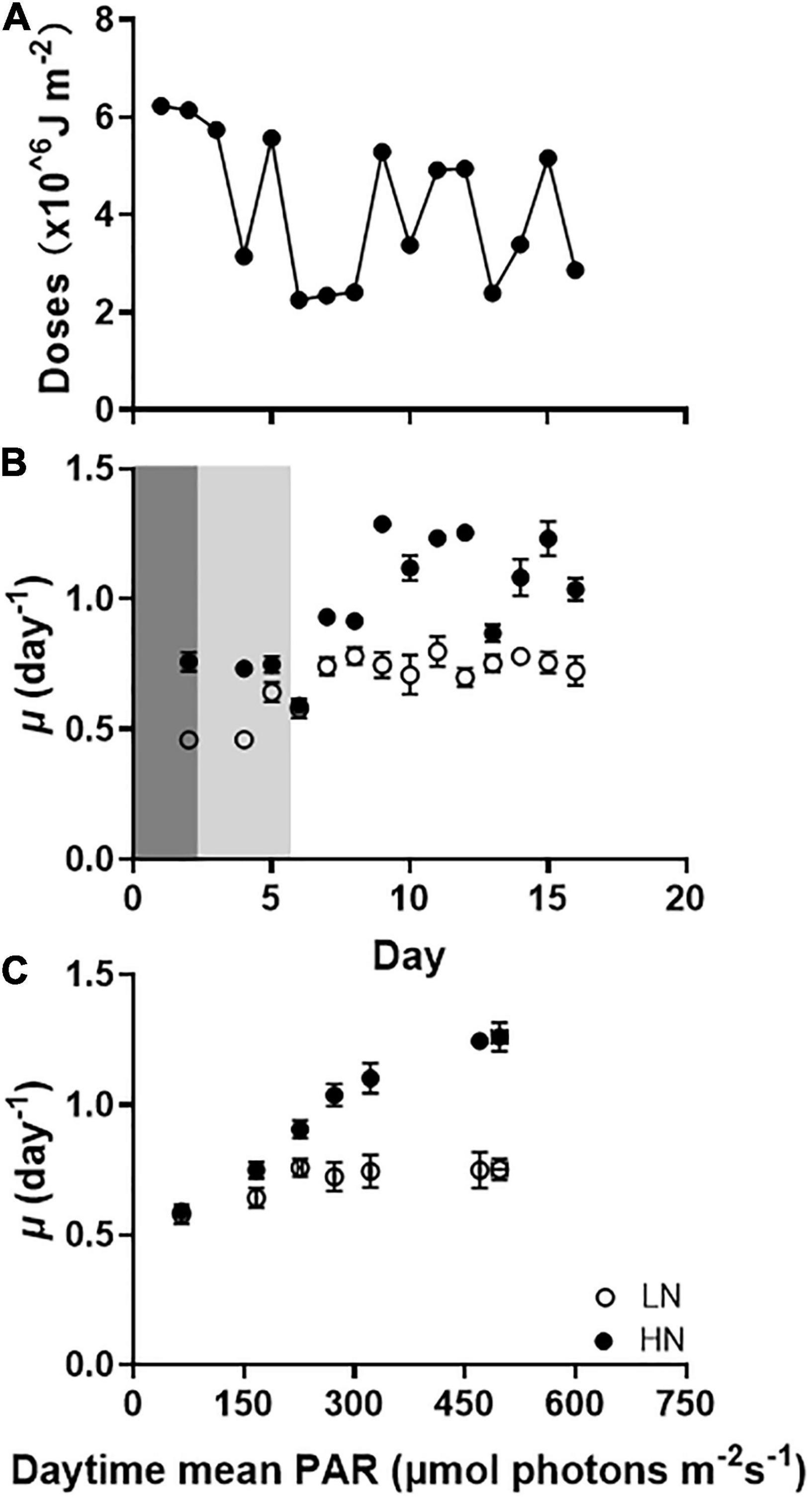
Figure 2. (A) Daily doses of photosynthetically active radiation (PAR) during the outdoor culture periods (from 18th December 2018 to 2nd January 2019). (B) The specific growth rates of Gephyrocapsa oceanica cells grown under fluctuating light regimes and replete (HN) and limited (LN) conditions. Values are the means ± SD of triplicate cultures. The dark gray and light gray darkened areas indicate the periods of reduced levels of solar radiation for the cells to acclimate from 17 to 30% and then to 100% incident sunlight. (C) The specific growth rates of the cells grown at HN and LN as a function of daytime mean light levels. The values of daytime mean PAR exposed to the algal cells was 89% incident sunlight.
The photochemical yield in HN- and LN-cells, decreased within 28 min, then leveled off (Supplementary Figure 2). After 60 min-exposure, the yield decreased by 48 and 33% for LN- and HN-cells, respectively. In addition, the yield of HN-cells recovered to their initial values under the dim light in 50 min, while that of LN-cells did not, indicating less tolerance of high-light stress in LN-grown cells.
Higher nitrate concentrations resulted in larger coccosphere and protoplast size in G. oceanica (Figure 3A and Supplementary Figure 4). However, the coccosphere thickness increased with reduced nitrate availability (Supplementary Figure 4). The coccosphere thickness here referred to all the coccoliths outside the cell, including any possible stacked ones. This was consistent with cellular PIC quotas, which increased, respectively, by 24% under indoor light and by 35% under outdoor sunlight in the N-limited cells (p > 0.05, Supplementary Table 1). The PON, PIC, and POC production rates exhibited the same trend as cellular PON and POC quotas, with values significantly lower in the low nitrate treatments (p < 0.01). The PIC production rates were significantly reduced by lowered N availability under constant light conditions (p < 0.01). However, the production rates of PIC were relatively stable for LN- and HN-cells exposed to fluctuating sunlight. Additionally, the less decrease in calcification rates than organic carbon fixation rates in the low nitrate treatments resulted in higher PIC/POC ratios (Supplementary Table 1 and Figure 3C).
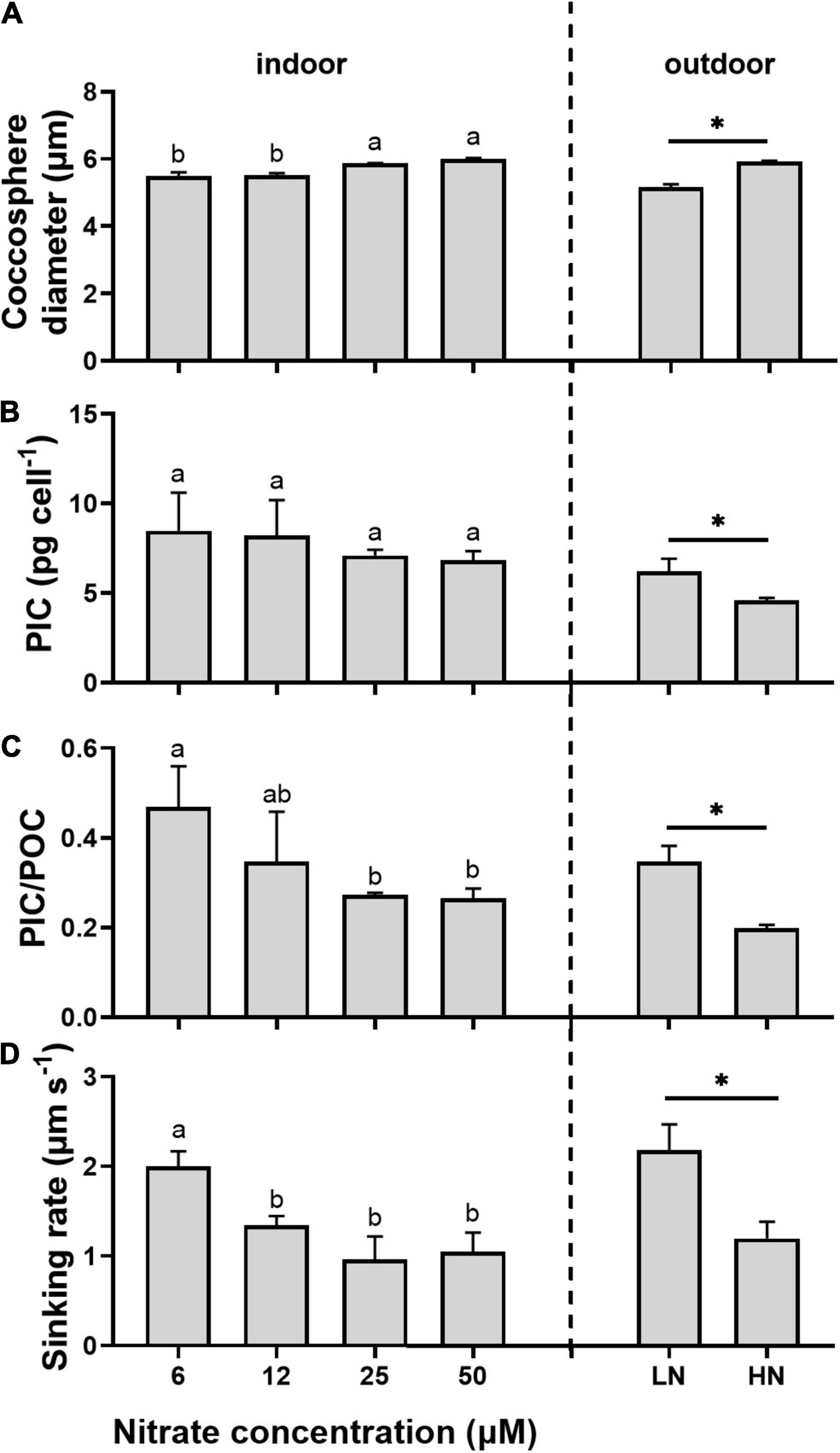
Figure 3. The coccosphere diameter (A), cellular particulate inorganic carbon (PIC) quotas (B), PIC/particulate organic carbon (POC) ratio (C), and sinking rate (D) of Gephyrocapsa oceanica cells grown under different levels of nitrate under indoor and outdoor light conditions. Values are the means ± SD of triplicate cultures. Symbols with different lowercase letters and * indicate significant differences among different treatments (p < 0.05). The difference is not significant if there the letters above the columns are the same or their combinations, completely different letters indicate significant changes.
The sinking rates of 6 μM nitrate-grown cells were significantly higher than that of the cells grown under other nitrate concentrations (p < 0.01, Figure. 3D). Outdoor LN-grown cells showed sinking rates of 2.19 ± 0.29 μm s–1, which were significantly faster by 82% than HN-cells (1.20 ± 0.19 μm s–1, p < 0.01). By plotting the relationships of sinking rates vs. coccosphere size and PIC/POC ratios irrespective of all the treatments, it revealed that the sinking rate was positively correlated with cell density and the ratio of PIC to POC and negatively correlated with coccosphere size in G. oceanica (Figure 4).
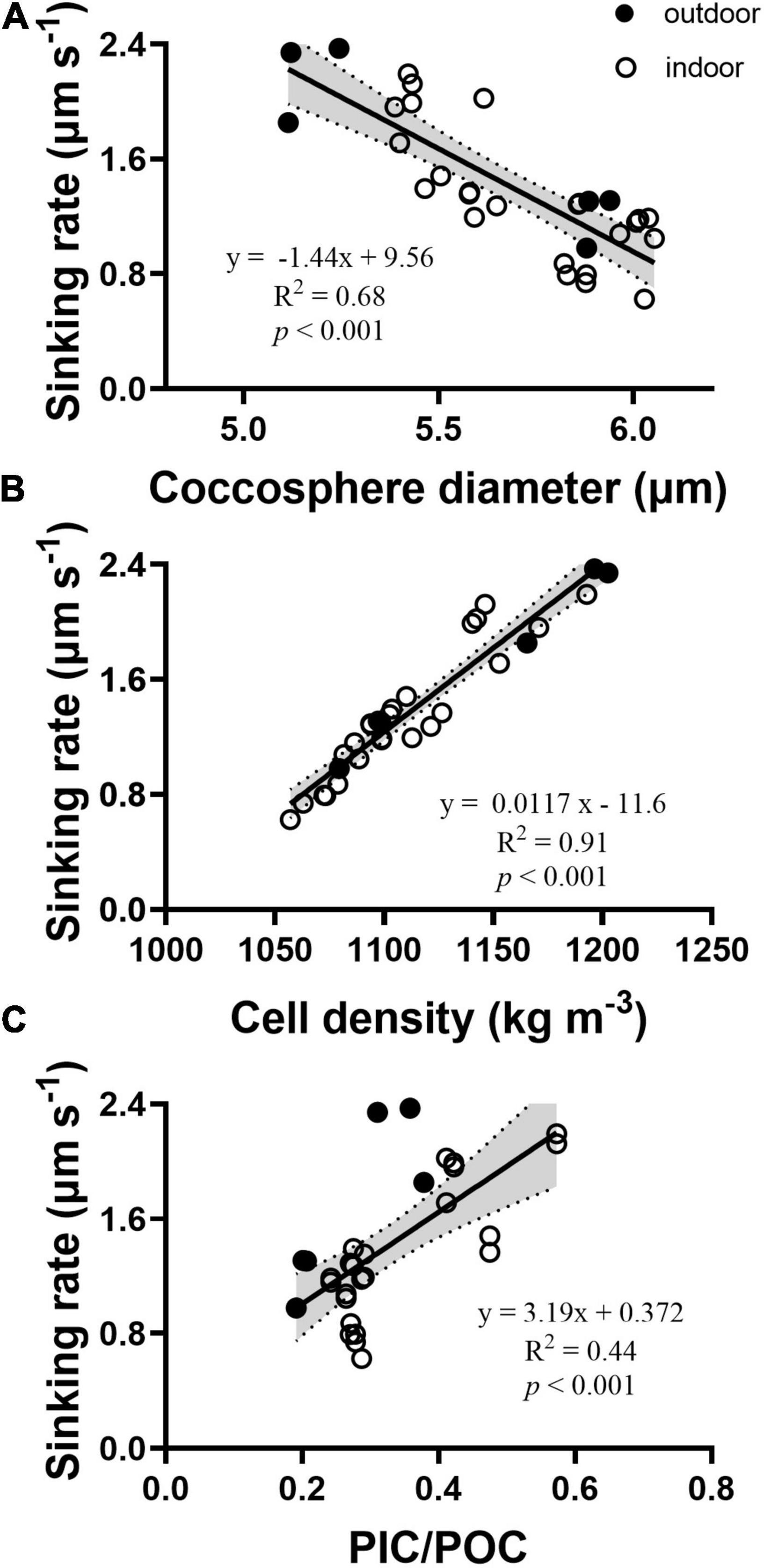
Figure 4. The relationship of sinking rates vs. coccosphere diameter (A), cell density (B), and PIC/POC ratios (C) of Gephyrocapsa oceanica. The solid line was obtained by linear fitting. Data represent sinking rates of the indoor and outdoor grown cells.
Discussion
Our results indicated that G. oceanica cells grown under N-limited conditions increased their PIC quotas with thicker coccoliths, resulting in faster sinking rates, which were positively correlated with PIC/POC ratios regardless of indoor constant light and outdoor fluctuating sunlight conditions. The microalgal cells sustained their photochemical performances with non-affected light use efficiency for photosynthetic electron transport even under nitrate deficient conditions (Table 2), so that energy required for PIC production was sufficiently provided.
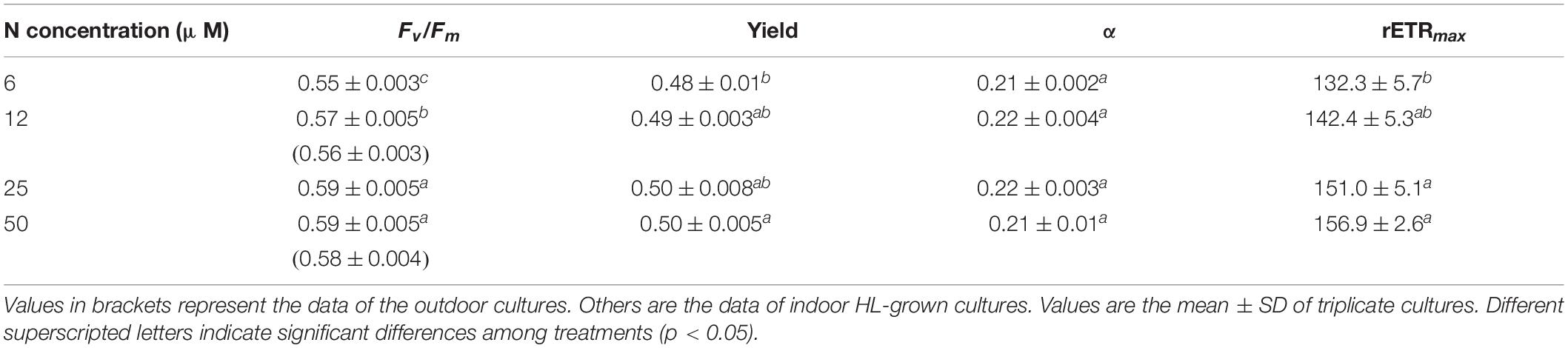
Table 2. The maximum quantum yield (Fv/Fm), effective quantum yield (Yield), apparent electron transfer efficiency (α), and maximum relative electron transfer rate (rETRmax) of Gephyrocapsa oceanica cells grown under different nitrate concentrations.
With reduced availability of nitrate, the decrease in Chl a content (Supplementary Figure 3), and maximal relative electron transfer rate should hinder light harvesting and photosynthetic energy transfer (Kolber et al., 1988). However, our results showed that photochemical performances that are essential for energy transfer sustained even under the nitrate limitation (Table 2), though the growth rates of G. oceanica were reduced (Figures 1, 2). Excess light energy is suggested to harm the PSII of microalgae and lead to photoinactivation (Loebl et al., 2010; Campbell and Serôdio, 2020), which can be exacerbated due to reduced availability of macro-nutrients (Talmy et al., 2013; Li et al., 2015). However, proteins associated with mitigation of photo-oxidative stress as shown in Emiliania huxleyi could be prominently enhanced under N-limitation, allowing the cells to maintain relatively high Fv/Fm values (Rokitta et al., 2014). In this study, although N-limitation made the G. oceanica cells more susceptible to high-light stress (Supplementary Figure 2), the cells acclimated to LN sustained their light use efficiency (Table 2), supporting the energy supply for calcification and assimilation processes. Calcification of coccolithophores has been discussed to play roles as a sunshade or in photoprotection in coccolithophores against solar radiation or high light (Guan and Gao, 2010; Xu and Gao, 2012; Monteiro et al., 2016). Although the reduced levels of transmission of UVR and PAR by the coccosphere in E. huxleyi was quantified (Gao et al., 2009) and calcification increased with increased artificial or solar radiation levels in E. huxleyi (Guan and Gao, 2010; Zhang et al., 2019), enhanced PIC quotas with reduced nitrate availability in the present study (Supplementary Table 1) can only infer a photoprotective strategy associated with PIC-sheltering (Gao, 2017). From the energetic point of view, G. oceanica cells grown under LN could allocate relatively more energy to produce PIC in the sacrifice of growth and POC production. This was reflected in the model established by Rokitta and Rost (2012), showing that the light-depend energetics of the cell was further altered by secondary treatments. Moreover, the decreased cell size of LN-grown cells might be beneficial for coccoliths to be expelled to the outside of cells due to increased ratios of cell surface to volume. This is because coccoliths are formed in the intracellular coccolith vesicle (Paasche, 2001), and the smaller cell diameter, the shorter distance for coccolith transportation from the coccolith vesicle to the cell surface (Müller et al., 2012). Comparing the results of indoor and outdoor experiments, the effects of lowered nitrate availability on the physiological performance of G. oceanica did not appear to be altered by fluctuating sunlight (Table 2, Figure 3, and Supplementary Figure 3). Interestingly, the growth rates of HN- and LN-cells under constant light conditions were higher than that under fluctuating sunlight at low to mid daytime mean light levels, at which the growth of LN-cells was no longer stimulated with increased light availability. This implies that the cells demand more energy to cope with fluctuation of light in sacrifice of growth.
While the Stokes’ law shows that the sinking rate of spherical particles is mainly dependent on size and density, here, we demonstrated that combination of N-limitation and high light gave rise to faster sinking rates that positively correlated with PIC content and PIC/POC ratios (Figures 3, 4). In E. huxleyi, cell size was considered as the major determinant of sinking rate, though a significant increase of lipid in N-limited cells was detected (Pantorno et al., 2013). Contrastingly, our results point to the “ballast effect” of coccoliths on sinking, which was negatively correlated with cell size in G. oceanica (Figure 4). Additionally, the positive correlation of sinking rate with PIC/POC ratios has been demonstrated in Coccolithus pelagicus cells grown with different availabilities of phosphate (Gerecht et al., 2015). And it has also been reported in E. huxleyi under increased temperature (Milner et al., 2016). Although N-limitation is known to facilitate the production of fatty acids and regulate the expression of related enzymes in coccolithophores (Rokitta et al., 2014; Bakku et al., 2017) and other microalgae (Kumar et al., 2019), specific gravities of microalgal species could be complicated by bio-mineralization, as reflected in G. oceanica in the present work.
Most POC exported to the deep ocean is in the form of aggregates such as fecal pellets and marine snow (Fowler and Knauer, 1986), and is partially re-mineralized to dissolved inorganic carbon (DIC) during sinking (Hedges, 1992; Iversen and Ploug, 2013). Faster sinking rates of aggregates can alleviate the POC loss caused by remineralization. Coccoliths in the form of calcium carbonate have been reported to be beneficial for aggregate formation and to protect the aggregates against disaggregation (Engel et al., 2009). Taken together with our results, we speculate that in the oligotrophic oceans, although the POC production is relatively slow, increased PIC content of coccolithophores as ballast in aggregates can enhance POC export efficiency. While, nutrient co-limitation matters more than nitrogen limitation alone in terms of phytoplankton growth in pelagic surface waters (Browning et al., 2021), coccolithophores in surface oceans under nutrient-co-limited conditions with high solar exposures may produce more PIC per POC. Consequently, the ballasting effect could be enhanced with progressive ocean climate changes due to less upward transport of nutrients associated with enhanced stratification of upper mixing layer (Gao et al., 2019). Nevertheless, the calcification-related genes for E. huxleyi were shown to be down-regulated under multiple stressors, including nutrients, warming, and CO2 (Feng et al., 2020). Although omics-responses to multiple stressors have not yet well-documented in coccolithophores, future ocean climate changes can inevitably impact their photosynthesis and calcification (Bolton et al., 2016; Tong et al., 2019). This emphasizes the need to understand mechanistic responses of phytoplankton physiology and sinking to multiple environmental changes (Feng et al., 2021).
Data Availability Statement
The raw data supporting the conclusions of this article will be made available by the authors, without undue reservation.
Author Contributions
XJ and KG designed the experiment and wrote the manuscript. XJ carried out the experiment with the assistance of HL and ST. All authors reviewed and gave their approval for the final manuscript.
Funding
This study was supported by National Natural Science Foundation of China (41721005, 41720104005, 41890803).
Conflict of Interest
The authors declare that the research was conducted in the absence of any commercial or financial relationships that could be construed as a potential conflict of interest.
Publisher’s Note
All claims expressed in this article are solely those of the authors and do not necessarily represent those of their affiliated organizations, or those of the publisher, the editors and the reviewers. Any product that may be evaluated in this article, or claim that may be made by its manufacturer, is not guaranteed or endorsed by the publisher.
Acknowledgments
We are grateful to the laboratory engineers Xianglan Zeng and Wenyan Zhao, and to the former Ph.D. student Liming Qu, for their logistical and technical support.
Supplementary Material
The Supplementary Material for this article can be found online at: https://www.frontiersin.org/articles/10.3389/fmars.2022.834358/full#supplementary-material
References
Armstrong, R. A., Lee, C., Hedges, J. I., Honjo, S., and Wakeham, S. G. (2002). A new, mechanistic model for organic carbon fluxes in the ocean based on the quantitative association of POC with ballast minerals. Deep Sea Res. Part II 49, 219–236. doi: 10.1016/S0967-0645(01)00101-1
Bakku, R. K., Araie, H., Hanawa, Y., Shiraiwa, Y., and Suzuki, I. (2017). Changes in the accumulation of alkenones and lipids under nitrogen limitation and its relation to other energy storage metabolites in the haptophyte alga Emiliania huxleyi CCMP 2090. J. Appl. Phycol. 30, 23–36. doi: 10.1007/s10811-017-1163-x
Beardall, J., Stojkovic, S., and Gao, K. (2014). Interactive effects of nutrient supply and other environmental factors on the sensitivity of marine primary producers to ultraviolet radiation: implications for the impacts of global change. Aquat. Biol. 22, 5–23. doi: 10.3354/ab00582
Behrenfeld, M. J. O., Malley, R. T., Siegel, D. A., McClain, C. R., Sarmiento, J. L., Feldman, G. C., et al. (2006). Climate-driven trends in contemporary ocean productivity. Nature 444, 752–755. doi: 10.1038/nature05317
Bienfang, P. K. (1981). SETCOL - A technologically simple and reliable method for measuring phytoplankton sinking rates. Can. J. Fish. Aquat. Sci. 38, 1289–1294. doi: 10.1139/f81-173
Bolton, C. T., Hernández-Sánchez, M. T., Fuertes, M.-Á., González-Lemos, S., Abrevaya, L., Mendez-Vicente, A., et al. (2016). Decrease in coccolithophore calcification and CO2 since the middle Miocene. Nat. Commun. 7:10284. doi: 10.1038/ncomms10284
Boyd, P. W., Doney, S. C., Strzepek, R., Dusenberry, J., Lindsay, K., and Fung, I. (2008). Climate-mediated changes to mixed-layer properties in the Southern Ocean: assessing the phytoplankton response. Biogeosciences 5, 847–864. doi: 10.5194/bg-5-847-2008
Boyd, P. W., and Trull, T. W. (2007). Understanding the export of biogenic particles in oceanic waters: Is there consensus? Prog. Oceanogr. 72, 276–312. doi: 10.1016/j.pocean.2006.10.007
Browning, T. J., Liu, X., Zhang, R., Wen, Z., Liu, J., Zhou, Y., et al. (2021). Nutrient co-limitation in the subtropical Northwest Pacific. Limnol. Oceanogr. Lett. 7, 52–61. doi: 10.1002/lol2.10205
Campbell, D. A., and Serôdio, J. (2020). “Photoinhibition of Photosystem II in Phytoplankton: Processes and Patterns,” in Photosynthesis in Algae: Biochemical and Physiological Mechanisms, eds A. W. D. Larkum, A. R. Grossman, and J. A. Raven (Cham: Springer), 329–365. doi: 10.1007/978-3-030-33397-3_13
Eberlein, T., Van de Waal, D. B., Brandenburg, K. M., John, U., Voss, M., Achterberg, E. P., et al. (2016). Interactive effects of ocean acidification and nitrogen limitation on two bloom-forming dinoflagellate species. Mar. Ecol. Prog. Ser. 543, 127–140. doi: 10.3354/meps11568
Engel, A., Szlosek, J., Abramson, L., Liu, Z., and Lee, C. (2009). Investigating the effect of ballasting by CaCO3 in Emiliania huxleyi: I. Formation, settling velocities and physical properties of aggregates. Deep Sea Res. Part II 56, 1396–1407. doi: 10.1016/j.dsr2.2008.11.027
Falkowski, P. G., Barber, R. T., and Smetacek, V. (1998). Biogeochemical controls and feedbacks on ccean primary production. Science 281, 200–207. doi: 10.1126/science.281.5374.200
Feng, Y., Chai, F., Wells, M. L., Liao, Y., Li, P., Cai, T., et al. (2021). The combined effects of increased pCO2 and warming on a coastal phytoplankton assemblage: from species composition to sinking rate. Front. Mar. Sci. 8:622319. doi: 10.3389/fmars.2021.622319
Feng, Y., Roleda, M. Y., Armstrong, E., Boyd, P. W., and Hurd, C. L. (2017). Environmental controls on the growth, photosynthetic and calcification rates of a Southern Hemisphere strain of the coccolithophore Emiliania huxleyi. Limnol. Oceanogr. 62, 519–540. doi: 10.1002/lno.10442
Feng, Y., Roleda, M. Y., Armstrong, E., Summerfield, T. C., Law, C. S., Hurd, C. L., et al. (2020). Effects of multiple drivers of ocean global change on the physiology and functional gene expression of the coccolithophore Emiliania huxleyi. Glob. Chang. Biol. 26, 5630–5645. doi: 10.1111/gcb.15259
Floder, S., and Burns, C. W. (2005). The influence of fluctuating light on diversity and species number of nutrient-limited phytoplankton. J. Phycol. 41, 950–956. doi: 10.1111/j.1529-8817.2005.00124.x
Fowler, S. W., and Knauer, G. A. (1986). Role of large particles in the transport of elements and organic compounds through the oceanic water column. Prog. Oceanogr. 16, 147–194. doi: 10.1016/0079-6611(86)90032-7
Gao, K. (2017). Changes in bioenergetics associated with ocean acidification and climate changes. Bioenergetics 6:147. doi: 10.4172/2167-7662.1000147
Gao, K. (2021). Approaches and involved principles to control pH/pCO2 stability in algal cultures. J. Appl. Phycol. 33, 3497–3505. doi: 10.1007/s10811-021-02585-y
Gao, K., Beardall, J., Häder, D.-P., Hall-Spencer, J. M., Gao, G., and Hutchins, D. A. (2019). Effects of ocean acidification on marine photosynthetic organisms under the concurrent influences of warming, UV radiation and deoxygenation. Front. Mar. Sci. 16:322. doi: 10.3389/fmars.2019.00322
Gao, K., Ruan, Z., Villafañe, V. E., Gattuso, J.-P., and Helbling, E. W. (2009). Ocean acidification exacerbates the effect of UV radiation on the calcifying phytoplankter Emiliania huxleyi. Limnol. Oceanogr. 54, 1855–1862. doi: 10.4319/lo.2009.54.6.1855
Gerecht, A. C., Šupraha, L., Edvardsen, B., Langer, G., and Henderiks, J. (2015). Phosphorus availability modifies carbon production in Coccolithus pelagicus (Haptophyta). J. Exp. Mar. Biol. Ecol. 472, 24–31. doi: 10.1016/j.jembe.2015.06.019
Guan, W., and Gao, K. (2010). Impacts of UV radiation on photosynthesis and growth of the coccolithophore Emiliania huxleyi (Haptophyceae). Environ. Exp. Bot. 67, 502–508. doi: 10.1016/j.envexpbot.2009.08.003
Hedges, I. J. (1992). Global biogeochemical cycles: progress and problems. Mar. Chem. 39, 67–93. doi: 10.1016/0304-4203(92)90096-S
Iversen, M. H., and Ploug, H. (2013). Temperature effects on carbon-specific respiration rate and sinking velocity of diatom aggregates - potential implications for deep ocean export processes. Biogeosciences 10, 4073–4085. doi: 10.5194/bg-10-4073-2013
Jin, P., Gao, K., Villafañe, V. E., Campbell, D. A., and Helbling, E. W. (2013). Ocean acidification alters the photosynthetic responses of a coccolithophorid to fluctuating ultraviolet and visible radiation. Plant Physiol. 162, 2084–2094. doi: 10.1104/pp.113.219543
Kaffes, A., Thoma, S., Trimborn, S., Rost, B., Langer, G., Richter, K.-U., et al. (2010). Carbon and nitrogen fluxes in the marine coccolithophore Emiliania huxleyi grown under different nitrate concentrations. J. Exp. Mar. Biol. Ecol. 393, 1–8. doi: 10.1016/j.jembe.2010.06.004
Klaas, C., and Archer, D. E. (2002). Association of sinking organic matter with various types of mineral ballast in the deep sea: implications for the rain ratio. Glob. Biogeochem. Cycles 16:1116. doi: 10.1029/2001gb001765
Kolber, Z., Zehr, J., and Falkowski, P. (1988). Effects of growth irradiance and nitrogen limitation on photosynthetic energy conversion in photosystem II. Plant Physiol. 88, 923–929. doi: 10.1104/pp.88.3.923
Kumar, B. R., Deviram, G., Mathimani, T., Duc, P. A., and Pugazhendhi, A. (2019). Microalgae as rich source of polyunsaturated fatty acids. Biocatal. Agric. Biotechnol. 17, 583–588. doi: 10.1016/j.bcab.2019.01.017
Lam, P. J., Doney, S. C., and Bishop, J. K. B. (2011). The dynamic ocean biological pump: insights from a global compilation of particulate organic carbon, CaCO3, and opal concentration profiles from the mesopelagic. Glob. Biogeochem. Cycles 25:GB3009. doi: 10.1029/2010gb003868
Langer, G., Oetjen, K., and Brenneis, T. (2012). Calcification of Calcidiscus leptoporus under nitrogen and phosphorus limitation. J. Exp. Mar. Biol. Ecol. 413, 131–137. doi: 10.1016/j.jembe.2011.11.028
Li, G., Brown, C. M., Jeans, J. A., Donaher, N. A., McCarthy, A., and Campbell, D. A. (2015). The nitrogen costs of photosynthesis in a diatom under current and future pCO2. New Phytol. 205, 533–543. doi: 10.1111/nph.13037
Litchman, E., Klausmeier, C. A., and Bossard, P. (2004). Phytoplankton nutrient competition under dynamic light regimes. Limnol. Oceanogr. 49, 1457–1462. doi: 10.4319/lo.2004.49.4_part_2.1457
Liu, H., and Wu, C.-J. (2016). Effect of the silica content of diatom prey on the production, decomposition and sinking of fecal pellets of the copepod Calanus sinicus. Biogeosciences. 13, 4767–4775. doi: 10.5194/bg-13-4767-2016
Loebl, M., Cockshutt, A. M., Campbell, D. A., and Finkel, Z. V. (2010). Physiological basis for high resistance to photoinhibition under nitrogen depletion in Emiliania huxleyi. Limnol. Oceanogr. 55, 2150–2160. doi: 10.4319/lo.2010.55.5.2150
Marañón, E., Lorenzo, M. P., Cermeno, P., and Mouriño-Carballido, B. (2018). Nutrient limitation suppresses the temperature dependence of phytoplankton metabolic rates. ISME J. 12, 1836–1845. doi: 10.1038/s41396-018-0105-1
Milner, S., Langer, G., Grelaud, M., and Ziveri, P. (2016). Ocean warming modulates the effects of acidification on Emiliania huxleyi calcification and sinking. Limnol. Oceanogr. 61, 1322–1336. doi: 10.1002/lno.10292
Monteiro, F. M., Bach, L. T., Brownlee, C., Bown, P., Rickaby, R. E. M., Poulton, A. J., et al. (2016). Why marine phytoplankton calcify. Sci. Adv. 2:e1501822. doi: 10.1126/sciadv.1501822
Moore, C. M., Mills, M. M., Arrigo, K. R., Berman-Frank, I., Bopp, L., Boyd, P. W., et al. (2013). Processes and patterns of oceanic nutrient limitation. Nat. Geosci. 6, 701–710. doi: 10.1038/ngeo1765
Müller, M. N., Beaufort, L., Bernard, O., Pedrotti, M. L., Talec, A., and Sciandra, A. (2012). Influence of CO2 and nitrogen limitation on the coccolith volume of Emiliania huxleyi (Haptophyta). Biogeosciences. 9, 4155–4167. doi: 10.5194/bg-9-4155-2012
Paasche, E. (2001). A review of the coccolithophorid Emiliania huxleyi (Prymnesiophyceae), with particular reference to growth, coccolith formation, and calcification-photosynthesis interactions. Phycologia 40, 503–529. doi: 10.2216/i0031-8884-40-6-503.1
Pantorno, A., Holland, D. P., Stojkovic, S., and Beardall, J. (2013). Impacts of nitrogen limitation on the sinking rate of the coccolithophorid Emiliania huxleyi (Prymnesiophyceae). Phycologia 52, 288–294. doi: 10.2216/12-064.1
Ploug, H., Iversen, M. H., Koski, M., and Buitenhuis, E. T. (2008). Production, oxygen respiration rates, and sinking velocity of copepod fecal pellets: direct measurements of ballasting by opal and calcite. Limnol. Oceanogr. 53, 469–476. doi: 10.2307/40006431
Porra, R. J. (2002). The chequered history of the development and use of simultaneous equations for the accurate determination of chlorophylls a and b. Photosynth. Res. 73, 149–156. doi: 10.1023/A:1020470224740
Ralph, P. J., Macinnis-Ng, C. M. O., and Frankart, C. (2005). Fluorescence imaging application: effect of leaf age on seagrass photokinetics. Aquat. Bot. 81, 69–84. doi: 10.1016/j.aquabot.2004.11.003
Raven, J. A., and Crawfurd, K. (2012). Environmental controls on coccolithophore calcification. Mar. Ecol. Prog. Ser. 470, 137–166. doi: 10.3354/meps09993
Riebesell, U., Bach, L. T., Bellerby, R. G. J., Rafael Bermúdez Monsalve, J., Boxhammer, T., Czerny, J., et al. (2016). Competitive fitness of a predominant pelagic calcifier impaired by ocean acidification. Nat. Geosci. 10, 19–23. doi: 10.1038/ngeo2854
Riebesell, U., Zondervan, I., Rost, B., Tortell, P. D., Zeebe, R. E., and Morel, F. M. M. (2000). Reduced calcification of marine plankton in response to increased atmospheric CO2. Nature 407, 364–367. doi: 10.1038/35030078
Rokitta, S. D., Dassow, P. V., and Rost, B. (2014). Emiliania huxleyi endures N-limitation with an efficient metabolic budgeting and effective ATP synthesis. BMC Genomics 15:1051. doi: 10.1186/1471-2164-15-1051
Rokitta, S. D., and Rost, B. (2012). Effects of CO2 and their modulation by light in the life-cycle stages of the coccolithophore Emiliania huxleyi. Limnol. Oceanogr. 57, 607–618. doi: 10.4319/lo.2012.57.2.0607
Sunda, W. G., Price, N. M., and Morel, F. M. (2005). “Trace metal ion buffers and their use in culture studies,” in Algal Culturing Techniques, ed. R. A. Andersen (London: Academic Press), 35–63. doi: 10.1007/s13398-0140173-7.2
Talmy, D., Blackford, J., Hardman-Mountford, N. J., Dumbrell, A. J., and Geider, R. J. (2013). An optimality model of photoadaptation in contrasting aquatic light regimes. Limnol. Oceanogr. 58, 1802–1818. doi: 10.4319/lo.2013.58.5.180
Tong, S., Hutchins, D. A., and Gao, K. (2019). Physiological and biochemical responses of Emiliania huxleyi to ocean acidification and warming are modulated by UV radiation. Biogeosciences 16, 561–572. doi: 10.5194/bg-16-561-2019
Xu, K., and Gao, K. (2012). Reduced Calcification decreases photoprotective capability in the Coccolithophorid Emiliania huxleyi. Plant Cell Physiol. 53, 1267–1274. doi: 10.1093/pcp/pcs066
Zhang, Y., Fu, F., Hutchins, D. A., and Gao, K. (2019). Combined effects of CO2 level, light intensity, and nutrient availability on the coccolithophore Emiliania huxleyi. Hydrobiologia 842, 127–141. doi: 10.1007/s10750-019-04031-0
Zhang, Y., and Gao, K. (2021). Photosynthesis and calcification of the coccolithophore Emiliania huxleyi are more sensitive to changed levels of light and CO2 under nutrient limitation. J. Photochem. Photobiol. B 217, 112145. doi: 10.1016/j.jphotobiol.2021.112145
Keywords: calcification, coccolithophore, growth, light, nitrate-limitation, photosynthesis, sinking rate
Citation: Jiang X, Li H, Tong S and Gao K (2022) Nitrogen Limitation Enhanced Calcification and Sinking Rate in the Coccolithophorid Gephyrocapsa oceanica Along With Its Growth Being Reduced. Front. Mar. Sci. 9:834358. doi: 10.3389/fmars.2022.834358
Received: 13 December 2021; Accepted: 04 February 2022;
Published: 25 February 2022.
Edited by:
Kai G. Schulz, Southern Cross University, AustraliaReviewed by:
Kai Xu, Jimei University, ChinaAnne Willem Omta, Massachusetts Institute of Technology, United States
Sebastian Dominik Rokitta, Alfred Wegener Institute Helmholtz Centre for Polar and Marine Research (AWI), Germany
Copyright © 2022 Jiang, Li, Tong and Gao. This is an open-access article distributed under the terms of the Creative Commons Attribution License (CC BY). The use, distribution or reproduction in other forums is permitted, provided the original author(s) and the copyright owner(s) are credited and that the original publication in this journal is cited, in accordance with accepted academic practice. No use, distribution or reproduction is permitted which does not comply with these terms.
*Correspondence: Kunshan Gao, a3NnYW9AeG11LmVkdS5jbg==