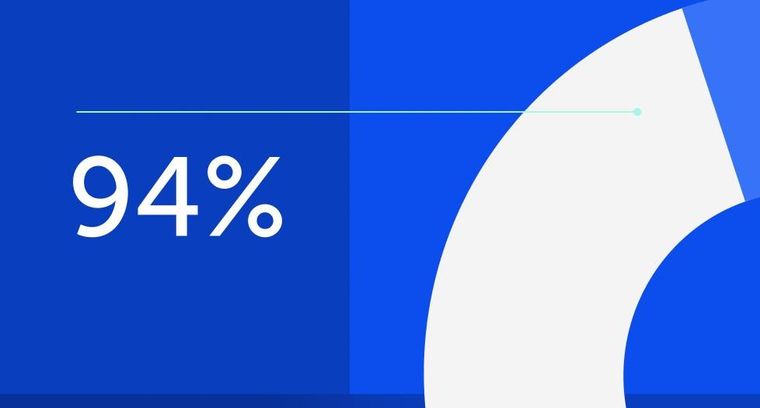
94% of researchers rate our articles as excellent or good
Learn more about the work of our research integrity team to safeguard the quality of each article we publish.
Find out more
ORIGINAL RESEARCH article
Front. Mar. Sci., 18 February 2022
Sec. Marine Biogeochemistry
Volume 9 - 2022 | https://doi.org/10.3389/fmars.2022.831937
This article is part of the Research TopicNatural and Artificial Radionuclides as Tracers of Ocean ProcessesView all 12 articles
The 234Th–238U disequilibrium has been widely used to quantify the sinking flux of particulate organic carbon (POC) out of the upper ocean. However, the influence of the advection on the quantification is poorly understood due to the lack of in situ measured physical parameters. Here, a Lagrangian observation was deployed for 39 h to track the variability of 234Th along with the current on the slope of the northeastern South China Sea (SCS). Contrasting to the general ocean interior, 234Th showed deficits relative to 238U in the mesopelagic waters, indicating an enhanced removal of 234Th. Concurrently, elevated total particulate matter (TPM) and POC contents were observed in the mesopelagic waters, supporting the driving force of the cross-shelf dispersion of re-suspended shelf/slope sediments for the 234Th removal. The widely used 234Th-model (ignoring physical processes) produced a much lower sinking flux of POC than the sediment trap-derived POC flux during the same observation, indicating an unneglectable influence of advection and diffusion. By considering the main horizontal advection and vertical diffusion, the 234Th–238U method gave rise to comparable results to sediment trap. 234Th-derived POC fluxes showed an increased pattern from 300 to 1,000 m, consistent with the more abundant POC where. These results indicated that advection represents an unneglectable process during the quantification of the sinking flux of 234Th over the slope of the SCS.
Particulate organic carbon (POC) settling largely dominates the ability of the oceans to sequestrate carbon dioxide. Various types of sediment traps have been used to directly determine the flux of POC (Li et al., 2018; Shih et al., 2020). Due to the requirements of long collection time (≥24 h), professional technique, and manpower for deployment and recovery of sediment traps, the radioactive method of 234Th–238U has later been developed and widely used in past decades (Buesseler et al., 2020). 234Th (T1/2 = 24.1 days), naturally generated from its parent of 238U, is a typical particle-reactive radionuclide in seawater (Black et al., 2018). Owing to its sinking with particles, 234Th is usually deficient to 238U in the upper ocean (Roca-Martí et al., 2017; Umhau et al., 2019). This deficit has thus been used to quantify the sinking flux of particulate components (especially POC) out of the euphotic zone (Buesseler et al., 2009; Maiti et al., 2016). In general, the mass balance model of 234Th is applied to constrain its sinking flux. Owing to the difficulty in obtaining in situ physical parameters (e.g., current velocity, diffusion coefficient, etc.) and collecting up-downstream gradient of 234Th, the widely adopted model usually neglects advection and diffusion processes (Coppola et al., 2002; Yang et al., 2016, 2021a). A few studies indicate that physical processes can largely affect the 234Th and POC fluxes in some oceanic settings, e.g., upwelling in the Equatorial Pacific (Bacon et al., 1996) and anticyclonic eddies (Zhou et al., 2013). In marginal seas and shallow waters with dynamic currents and tides, advection, and diffusion are expected to have a larger influence on the sinking flux of 234Th (Zhao et al., 2019). In addition, steady-state is often assumed to quantify the flux of 234Th due to the lack of time-series observation. However, non-steady-state, to a varying degree, influences the flux calculation (Martin et al., 2011; Resplandy et al., 2012). Besides, the ratio of POC to particulate 234Th used to convert 234Th flux to POC flux is another factor influencing the 234Th–238U method. It is generally expected that POC/234Th on large particles (>50 μm) represent sinking particles. However, results from sediment traps reveal that 234Th is mainly carried by small sinking particles (∼70–80% of the total) in the northern South China Sea (SCS), representing the majority sinking of 234Th (Hung et al., 2012). Less than 50 μm particles account for most of the bulk POC in the northeastern SCS (Hung and Gong, 2010). The largest fractions of both 234Th and POC in medium-sized (10–15 μm) particles are also observed in the northwestern Pacific Ocean (Hung et al., 2010). Hence, the influence of physical processes, temporal variability, and particle size on the 234Th–238U method needs extensive investigations to improve the application of 234Th–238U.
Usually, coastal and marginal seas show complicated hydrological conditions and significant spatiotemporal variability in the abundance of both 234Th and POC (Feng et al., 2021a,b), which challenges the widely used advection-excluded 234Th model (Zhao et al., 2019). Based on model-simulated physical parameters, i.e., advective velocity and diffusion coefficients, a few studies indicate that ignoring advection and diffusion would lead to a large deviation of 234Th fluxes in some marginal seas, e.g., the basin of the SCS (Cai et al., 2008; Zhou et al., 2013). However, another study reports that including model-simulated physical parameters in 234Th-model produces unreasonable results in the northern shelf/slope of the SCS due to the poorly constrained parameters (Cai et al., 2015). Thus, we hypothesize that dynamic hydrological fields result in the complex influence of physical processes on the quantification of 234Th flux and 234Th-derived POC flux in the shelf/slope areas. Thus, it is necessary to conduct in situ Lagrangian observation to obtain parameters and examine the influence of physical processes on the application of the 234Th/238U method especially in oceanic settings with strong physical dynamics.
In this study, the northeastern SCS was selected to investigate the influence of horizontal advection on the estimation of 234Th flux (Figure 1). Over the past decade, increasing studies report the widely observed cross-shelf dispersion of particulate matter (Shih et al., 2019; Shen et al., 2020; Yang et al., 2021b; Ge et al., 2022) driven by the dynamic physical processes (Li et al., 2018; Jia et al., 2019). Thus, the northeastern SCS could be an ideal site for attesting to the influence of physical processes on the application of the 234Th/238U method. Here, we conducted a Lagrangian observation of 234Th on the northeastern slope of the SCS. The measured horizontal advection velocity and 234Th in both upstream and downstream waters enable us to evaluate the influence of physical processes on the application of 234Th in the SCS.
Figure 1. Sampling stations in the northeastern South China Sea and the trajectory of the Lagrangian observation. The intervals between adjacent points denote the trajectory within an hour. The conceptual schematic of the nepheloid layer over the shelf/slope region highlights its ubiquity.
The study site is located in the northeastern SCS over the slope with water depths of hundreds of meters to more than a thousand meters (Figure 1). Recent researches have observed ubiquitous nepheloid layers (NLs) either in the mesopelagic water or in the bottom water of the northern SCS (Ma et al., 2017; Shih et al., 2019). A preliminary study indicates that internal solitary waves drive the resuspension of sediments on the slope (Jia et al., 2019). With the influence of currents, re-suspended particles disperse over a large scope as supported by the elevated particle fluxes in deeper sediment traps in the basin of the SCS (Schroeder et al., 2015; Hong et al., 2021). This dispersion of the shelf/slope sediments, mainly driven by advection, results in enhanced scavenging of particle-reactive nuclides (e.g., 210Po) in the mesopelagic water of the SCS (Figure 3; Ma et al., 2017) and thus the sinking flux of POC (Yang et al., 2021b).
Figure 2. Profiles of temperature, salinity at stations T1 and T2 (A), and particulate organic carbon (POC) and total particulate matter (TPM) at T2 (B).
Figure 3. Profiles of activity concentrations of total 234Th (A), dissolved 234Th (B), and particulate 234Th (C), and the activity ratios of total 234Th to 238U (D) at stations T1 and T2.
The buoy, assembled a sediment trap array with three traps at 50, 100, and 200 m, was deployed from August 9 to 11, 2016 in the northeastern SCS (Figure 1). A Lagrangian observation was conducted, starting at station T1 and ending at station T2 with a duration of 39 h. Seawater was collected at both T1 and T2 from surface to 1,000 m (Table 1) using Niskin bottles attached to a conductivity, temperature, and depth (CTD)-rosette system aboard the R/V Yanpin 2. In brief, 8 L of seawater at each specific depth were collected and filtered through a pre-combusted (450°C for 4 h) and weighted quartz fiber (QMA) filter (Whatman™, Maidstone, United Kingdom). The total particulate matter (TPM) retained on the filter was de-salted using Milli-Q water for the measurements of particulate 234Th (234ThP), POC, and TPM. Additionally, 4 L of the filtrate was collected for the analysis of dissolved 234Th (234ThD). The euphotic zone of 0–150 m was determined by using a photosynthetically available radiation (PAR) scalar quantum irradiance sensor, defined as the depth of 0.1% of the surface light intensity.
Table 1. Temperature, salinity, the content of total particulate matter (TPM), the concentration of particulate organic carbon (POC), activity concentrations of dissolved 234Th (234ThD), particulate 234Th (234ThP), total 234Th (234ThT), and 238U, and the activity ratios of 234ThT to 238U during the Lagrangian observation from station T1 to station T2.
The particulate samples were dried at 60°C after collection and weighted to determine the difference between pure QMA filter and TPM-contained filter, i.e., the content of TPM. The activity of 234ThP was counted using a gas-flow proportional low-level beta counter (RISØ GM-25-5A) as reported in our recent studies (Feng et al., 2021a,b) until the counting error was less than ±7%. A total of 150 days later, a second counting was conducted to assay the contribution of other beta emitters. 234ThD in the filtrate was concentrated using the small volume MnO2 co-precipitation method (Benitez-Nelson et al., 2001). In brief, the pH value of filtrate was adjusted to 9.0 using ammonium hydroxide, then KMnO4 and MnCl2 solution were added to form MnO2 particles. After stirring and sanding for over 6 h to adsorb 234Th onto MnO2 particles, MnO2 precipitate was separated from the solution via filtration using a QMA filter. The average recovery was 94.7 ± 2.3%, showing a stable yield. Then, the sample was dried at 60°C, the activity of 234ThD was counted using the same beta counter like 234ThP but with < 5% counting error. The concentrations of both 234ThD and 234ThP were calculated based on the two measurements and corrected for recovery, blank counts, and sampling time. The activity concentrations of the total 238U were calculated using the newly updated 238U-salinity relationship (Owens et al., 2011). The presented uncertainties of 234Th were propagated from the counting errors. The total 234Th (234ThT) and 234Th/238U ratio uncertainties were propagated from 234ThD and 234ThP.
The Lagrangian observation was designed to constrain the in situ current velocity (horizontal advection) and the gradient of 234Th activity between T1 and T2. On the northern SCS shelf with prominent cross-shelf transport of re-suspended sediments, this strategy could help us constrain the influence of advection on the flux estimation of 234Th and 234Th-derived POC flux at station T2. Hence, POC samples collected at station T2 were determined. After the measurements of 234ThP, particulate-containing QMA filters were fumigated using concentrated HCl for no less than 48 h to remove inorganic carbon. Then, samples were dried at 60°C. POC content was measured using an elemental analyzer (Perkin Elmer CHN, Waltham, United States). The standard material used was IAEA-C8.
The fluxes of TPM and POC was calculated from the 234Th fluxes (Buesseler et al., 1992), i.e.,
where FTPM and FPOC represent the flux of TPM (in g m–2 day–1) and POC (in mmol-C m–2 day–1), respectively. CTPM and CPOC are the concentrations of TPM and POC. ThP denotes the activity concentration of 234ThP.
Overall, the buoy floated northeast-ward along the surface current over the slope (Figure 1) with an average velocity of 19.5 cm s–1, which is close to the mean of 15 cm s–1 obtained from the model simulation for the upper 200 m in the study area (Gan et al., 2006). At stations T1 and T2 sampled, it is clear that the difference in salinity was discernible though temperature showed little difference (Figure 2A), corresponding to water mixing during the transport of seawater from T1 to T2. The tracking of the movement of the buoy enables us to obtain the real viability of parameters along the trajectory of the buoy.
The concentrations of TPM varied from 0.09 to 0.21 mg L–1 with an average of 0.15 ± 0.04 mg L–1 (Figure 2B). POC concentrations showed a range of 0.25–1.42 μmol L–1, averaging 0.67 μmol L–1. POC exhibited the highest values between 50 and 75 m (Figure 2B), corresponding to the widely observed depth of chlorophyll-a maximum in the SCS (Cai et al., 2015; Yang et al., 2021b). Notably, below the euphotic base (i.e., 150 m), both TPM and POC showed a downward increasing pattern (Figure 2B), increasing from 0.11 to 0.19 mg L–1 and 0.25 to 0.59 μmol L–1, respectively. This phenomenon was also reported at a station near T1 during the same cruise (Yang et al., 2021b), pointing to the cross-shelf transport of re-suspended sediments.
The activity concentrations of 234ThT varied from 1.00 to 2.03 (avg.: 1.64 dpm L–1) and 1.29–2.03 dpm L–1 (avg.: 1.68 dpm L–1) at stations T1 and T2, respectively (Table 1 and Figure 3A). On average, 234ThD accounted for 67 and 63% of 234ThT at T1 and T2 (Figures 3B,C), coinciding with the major dissolved form of 234Th in the open SCS (Zhou et al., 2013; Hong et al., 2021) and contrasting with the major particulate form in the coastal SCS (Feng et al., 2021a). The activity ratios of 234ThT to 238U (i.e., 234Th/238U) ranged from 0.42 to 0.84 at T1 and 0.54 to 0.86 at T2, averaging 0.67 and 0.70, respectively. Unlike the equilibrium between 234Th and 238U widely observed in mesopelagic waters, 234Th/238U showed deficits of 234Th relative to 238U at both T1 and T2 (Figure 3D), which was similar to the deficient 210Po relative to 210Pb observed near T1 during the same cruise (Yang et al., 2021b).
234ThT was lower than 238U with the 234Th/238U ratios of 0.58–0.84 at T1 and 0.57–0.86 at T2 between surface and 150 m (Table 1), indicating an efficient removal of 234Th from the euphotic zone (Figure 3). This observation is a typical scenario in the study area as was reported in all seasons (Cai et al., 2015). Below the euphotic zone (200–1,000 m), the 234ThT deficits were also observed at both T1 and T2 with the 234Th/238U ratios of 0.42–0.84. Although 234Th activity in the mesopelagic water of the SCS has not been reported, it is generally expected that 234Th should reach equilibrium with 238U due to the sparse TPM and long residence time of 234Th. In fact, equilibrium states have been observed in most mesopelagic waters in the open oceans, e.g., the southeastern tropical Pacific (Black et al., 2018), the Atlantic Ocean (Owens and Buesseler, 2015), the Indian Ocean (Anand et al., 2018), and the Atlantic sector of the Southern Ocean (Roca-Martí et al., 2017). Considering the strong particle reactivity of 234Th, the deficits of 234Th were attributed to its enhanced removal along with TPM sinking in the mesopelagic waters at stations T1 and T2 (Figure 3). On the one hand, the elevated TPM and POC concentrations (Figure 2) provided direct evidence for the abundant particulate matter in mesopelagic water. Another particle-reactive radionuclide 210Po also showed enhanced removal together with increased TPM in the mesopelagic water during the same cruise (Yang et al., 2021b). Shelf/slope sediment resuspension and successive dispersion are thought to be the provenance of the elevated TPM in the mesopelagic water of the northern SCS (Shih et al., 2019; Yang et al., 2021b), mechanistically driven by the internal solitary waves (Jia et al., 2019). On the other hand, abundant TPM usually leads to the deficits of 234Th not only in the widely investigated euphotic zone but also in the less-studied ocean interior. For instance, evident 234Th deficits have been observed in deep water near the active hydrothermal vents (Yang et al., 2016, 2021a) and the hydrothermal plume (Owens and Buesseler, 2015). Thus, the deficits of 234Th, together with the elevated TPM and POC contents in the mesopelagic water over the SCS slope, indicated that re-suspended sediments disperse over a large scope, supporting the influence of current advection on the spatial pattern of 234Th in the study area.
The trajectory of the buoy revealed that seawater flows northeastward over the slope of the SCS (Figure 1). Station T1 was located upstream of the current, and T2 the downstream. Thus, the difference in 234Th activities between T1 and T2 reflected the variability of 234Th along the current, representing the real variation of 234Th with time induced by its decay, generation from 238U, and water exchange mainly horizontal advection as illustrated by the buoy movement. By taking advantage of the Lagrangian observation, these results enable us to evaluate the real advection flux of 234Th and its influence on the sinking flux of 234Th for the first time on the dynamic slope of the SCS.
Ignoring the physical processes, the change of the amount of 234ThT with time can be expressed by:
where IThT and IU are the inventories of the total 234Th and 238U from surface to the export depth (in dpm m–2), which are calculated through the trapezoidal integration method. λTh is the decay constant of 234Th (0.02876 day–1). FTh is the sinking flux of 234Th in dpm m–2 day–1. A sediment trap, deployed near station T2 on August 6, 2016, reports that the POC flux at 150 m was 8.4 ± 0.9 mmol-C m–2 day–1 (Li et al., 2018). The other array of sediment traps assembled to the buoy (from August 9 to 11, 2016) show the POC fluxes of 9.0 ± 0.6 mmol-C m–2 day–1 at 100 m and 9.3 ± 1.0 mmol-C m–2 day–1 at 200 m, respectively (Qiu, 2018). These consistent results indicated that particle sinking exhibited little variability during our sampling, lending support to the steady-state assumption. In addition, our sampling only covered 1.6 day, which could not enable the non-steady-state model to work well (Savoye et al., 2006). Thus, the steady-state is the best option in our study. At a steady-state, the sinking fluxes of 234Th estimated from Eq. (2) varied from 3,176 to 21,197 dpm m–2 day–1 [i.e., λTh(IU−IThT) in Table 2]. The sinking flux of POC, estimated from Eq. (1), was 3.6 ± 0.3 mmol-C m–2 day–1 at 200 m, significantly lower than 9.3 ± 1.0 mmol-C m–2 day–1 determined by the sediment trap attached to the buoy (Qiu, 2018). Such an inconsistency indicated that physical processes, e.g., advection and diffusion, could be non-negligible during calculating the sinking flux of 234Th. Thus, the variability in total 234Th should be better expressed as:
Table 2. Fluxes of 234ThT and the fluxes of particulate organic carbon (POC) calculated from 234ThT fluxes below the euphotic zone (150 m).
where u and kz denote the horizontal advection velocity of the total 234Th in cm s–1 and vertical diffusive coefficient in cm2 s–1. ∂IThT/∂x and ∂AThT/∂z represent the gradient of inventory and activity of the total 234Th, respectively. To resolve Eq. (3), we need to assume (1) that the change rate of the inventory was uniform during the buoy transport; and (2) that the net changes of 234Th inventory were caused by the sinking of transported particles on the slope. For assumption (1), the consistent POC fluxes from two sediment traps (i.e., Li et al., 2018; Qiu, 2018) seemed to favor the little variability in the sinking flux of particles during our sampling. However, water mixing appeared to influence the second assumption. As shown in Figure 2, both temperature and salinity revealed the mixing of the traced water body with surrounding water masses during its transport from T1 to T2. The mixing processes of various waters, probably carrying different 234Th contents, could affect the estimate of 234Th flux by changing the activity of 234Th. The influence was evaluated through the variations of both 234Th and 238U between T1 and T2 by taking 234Th decay into account. Results indicated that the mixing process accounted for <4% of the total 234Th fluxes (ignoring physical processes from Eq. 2) estimated in the upper 200 m. Below 200 m, mixing resulted in a large influence with an average of 18%. Although this influence seemed to be acceptable for the assumption (2), Eq. (3) is still not resolved due to the lack of advection velocity and spatial gradients of 234Th inventory.
The Lagrangian observation provided in situ velocity of current (i.e., 19.5 cm s–1). However, this velocity is different from u in the second term on the right side of Eq. (3) in that particles have different transport speeds though basin-wide observations of sediment trap indicate that the transport of re-suspended sediment from the shelves is driven by lateral advection in the SCS (Schroeder et al., 2015). As an alternative strategy, we evaluated the influence of physical processes by comparing the flux of 234Th at 200 m (ignoring physical processes) with that calculated through trap-derived POC at 200 m (i.e., trap-POC flux divided by the CPOC/ThP ratio in Eq. 1). The sinking flux of 234Th estimated from trap-POC flux was 10,775 ± 611 dpm m–2 day–1, which was much higher than 4,136 ± 148 dpm m–2 day–1 obtained by Eq. (2). In other words, physical processes resulted in 6,639 ± 629 dpm m–2 day–1 flux of 234Th. Although in situ observed velocity and the gradients of 234Th could not be directly applied to calculate the advection term in Eq. (3), they may, to a first-order approximation, be used to highlight the difference between the actual physical influence and our observations. The transport speed of particles is usually smaller than the current. If the observed velocity was used as an upper limit of the particle transport, and the derivative (i.e., the second term on the right side of Eq. 3) was substituted for the finite difference [i.e., u(IThT,T1−IThT,T2)/Δx, commonly estimated from the linear interpolation, Resplandy et al., 2012], the upper limit of advection influence was estimated to be 4,733 ± 1,831 dpm m–2 day–1 at 200 m. The vertical gradients of the total 234Th (i.e., ∂AThT/∂z) were estimated through the fitting curves obtained from its vertical distribution (Figure 3A), i.e., AThT = 1.90e–0.0154z (r2 = 0.82) for 100–300 m. In the upper SCS, the diffusive coefficient ranges from 10–4 to 10–5 m2 s–1 even though it could reach 10–3 m2 s–1 in the mid-deep water (Wang et al., 2019). To evaluate the largest influence of the diffusion term, we conservatively adopted 10–3 m2 s–1. At 200 m, the estimated diffusion contribution to the sinking flux of 234Th was 116 ± 39 dpm m–2 day–1. Taken together, physical processes maximally accounted for 4,850 ± 1,831 dpm m–2 day–1, lower than the actual physical effect of 6,639 ± 629 dpm m–2 day–1. Since velocity was overestimated, the estimated lower flux indicated that there existed an addition of 234Th from mixing or other processes during transport from T1 to T2, which led lower gradient and thus lower advection flux. It should be noted that although the product of the velocity of the buoy and the gradient of 234Th accounted for the majority (73%) of the physical-contributed flux, it did not mean that Lagrangian observation provided actual physical parameters. Thus, Eq. (3) could not be completely resolved. Considering the fact that using these not-rigorous assumptions can present better results than those estimated by ignoring physical processes (Figure 4A), the observed data were used to constrain the fluxes of 234Th and POC at other depths (Table 2). The results were compared with those obtained in the study area to further assay the rationality.
Figure 4. Fluxes of 234Th and particles on the northeastern shelf of the South China Sea (SCS), including (A) 234Th fluxes ignoring physical processes and considering advection and diffusion, (B) 234Th-derived TPM fluxes including physical processes, and (C) POC fluxes estimated from the 234Th–238U method (this study) and the 210Po–210Pb method (Yang et al., 2021b).
According to the model simulation (Gan et al., 2006), the current in the upper 200 m of the study area is somewhat different from that at 500 m. Simulated advection velocity of 10 cm s–1 below 200 m was adopted in calculating the advection flux of 234Th. For diffusion, 234ThT followed AThT = 1.90e–0.0154z (r2 = 0.71) between 500 and 1,000 m. The estimated flux for each process were presented in Table 2.
The 234Th-derived sinking fluxes of TPM varied from 0.88 to 7.70 g m–2 day–1, averaging 3.76 g m–2 day–1 at station T2 (Table 2). Over the western slope of the SCS, the 210Po-derived sinking fluxes of TPM out of 1,000 m varied from 0.4 to 10.2 g m–2 day–1 with an average of 4.4 g m–2 day–1 (Ma et al., 2017). Our result of 7.27g m–2 day–1 at 1,000 m fell within this range. The minimum occurred at 300 m, corresponding to the low TPM contents (Figures 2, 4B). With the increasing of TPM content to the mesopelagic bottom (i.e., 1,000 m), TPM also showed elevated sinking fluxes, indicating the cross-shelf dispersion of re-suspended sediments.
At the base of the euphotic zone (150 m), the sinking flux of POC (collected from August 9 to 11, 2016) was 7.8 mmol-C m–2 day–1 (Table 2), very coincident with 8.4 ± 0.9 mmol-C m–2 day–1 derived from a sediment trap near T2 (collected on August 6, 2016, Li et al., 2018), and falling in the range of sediment trap-derived scope of 2.8–9.0 mmol-C m–2 day–1 (Hung and Gong, 2010; Shih et al., 2019, 2020), satellite-derived 5.0–13.6 mmol-C m–2 day–1 (Chow et al., 2021), and 234Th-derived scope of 4.6–11.8 mmol-C m–2 day–1 (Cai et al., 2015) in the northeastern SCS. In general, sediment trap directly collects particles, which largely represent the total effect of all processes including biogenic, physical, and chemical ones (Hung et al., 2016; Shih et al., 2019), though it is sometimes influenced by trapping efficiency (Coppola et al., 2002). The comparability between our results and published datasets also indicated that the considering physical processes produced better fluxes of particles than those ignoring physical processes. Owing to the requirements for technical expertise and high cost, the sediment traps are usually deployed with low spatial resolution. In contrast, the 234Th method can provide POC flux data with high-resolution (Cai et al., 2008), but it suffers from poorly-constrained physical processes (Zhou et al., 2013). Biogeochemical models can decipher regional to global characteristics of POC flux, however, they often deviate from in situ measurements within small scope (Shih et al., 2021). Thus, accurate and high-resolution carbon export could be obtained in field studies by taking multiple methods’ merits (Baker et al., 2020).
Conventionally, large particles (>53 μm) are assumed to be the representative of sinking particles for determining POC/234Th ratios. However, some studies suggest that small particles (<50 μm) contribute the majority sinking of POC and 234Th. For example, 1–10 and 10–50 μm fractions account for 26–35 and 25–29% of the total POC based on the drifting sediment traps in the northern SCS (Hung and Gong, 2010). 234Th carried by <50 μm particles contributes ∼70–80% of the total 234Th in the northwestern Pacific Ocean (Hung et al., 2010, 2012). These results thus lend support to the conclusion that particles collected in our study represent the majority of sinking particles in the northern SCS. In addition, the POC/234Th ratios varied from 0.75 to 1.48 μmol dpm–1 (Table 2), which are comparable to 0.8–1.3 μmol dpm–1 obtained on 1–50 μm particles from sediment traps in the northern SCS (Hung and Gong, 2010), also supporting this conclusion. Notably, the POC flux out of the euphotic zone was much higher than those observed in the northern basin (Zhou et al., 2013) and the southern basin of the SCS (mostly <5.0 mmol-C m–2 day–1) (Cai et al., 2008; Yang et al., 2015), partly ascribed to the internal wave-induced input of nutrient-replete waters to the euphotic zone and successive phytoplankton flourish and POC settling (Li et al., 2018).
Compared with the flux at 150 m, POC showed comparable and significantly lower fluxes at 200 and 300 m, respectively (Table 2). In general, POC decreases with depth below the euphotic zone due to remineralization (Yang et al., 2009). Based on the 234Th fluxes and POC/234Th ratios (Table 2), it is clear that POC/234Th ratios largely result in the flux descending with depth. Below 300 m, evident increases in POC flux occurred, from 4.2 to 23.7 mmol-C m–2 day–1 (Figure 4C). Obviously, there are additional sources of POC besides its settling from local upper water. The vertical profile of TPM (Figure 2) illustrates additional contributions. Internal waves can lead to the resuspension of shelf/slope sediments in the northern SCS (Jia et al., 2019). Just before our sampling, strong internal waves were observed in the study area (Li et al., 2018). Probably, internal wave-induced resuspension of organic matter resulted in the elevated POC fluxes via cross-shelf dispersion. Similar scenarios were also observed at a station near T1 during the same cruise (Yang et al., 2021b) and in another study conducted in the same area (Shih et al., 2019). The scanning electron microscope images of the sinking particles below 150 m indicate a higher proportion of lithogenic material (Shih et al., 2019), lending support to its sediment origin.
A recent study reveals that a large amount of POC is required to support the consumption of deep microbes in the SCS interior (Shen et al., 2020). Our results provide direct evidence for the cross-transport of POC. Using the lowest value at 300 m as a reference, shelf/slope sediments contributed 1.7–21.3 mmol-C m–2 day–1 to the mesopelagic waters of the SCS. At a station close to T1 during the same cruise, the sinking fluxes of POC were estimated to vary from 5.4 to 33.6 mmol-C m–2 day–1 based on the 210Po–210Pb method (Yang et al., 2021b). Considering the uncertainties, the 210Po-derived fluxes by neglecting the horizontal advection and diffusion, were consistent with our 234Th-derived results (Figure 4), indicating less influence of advection on the 210Po–210Pb method.
A Lagrangian observation of 234Th was conducted over the slope of the northeastern SCS. Enhanced removal of 234Th was observed in the mesopelagic waters compared with general mesopelagic oceans, pointing to the active particle dynamics below the euphotic zone of the SCS. Concurrently elevated TPM and POC concentrations with depth in the mesopelagic water revealed that re-suspended sediments from the shelf/slope dominated the removal of 234Th. Combining the sediment trap results, the influence of physical processes on the sinking flux estimates of 234Th was evaluated, revealing unneglectable effects of horizontal advection and vertical diffusion. Although considering the physical processes seemed to produce better sinking fluxes of 234Th and POC, well-constraining the advection effect is still challenging due to the difficulty in obtaining the actual transport velocity of particles and spatial gradients.
The original contributions presented in the study are included in the article/supplementary material, further inquiries can be directed to the corresponding author.
XZ, YQ, and MZ collected and analyzed the samples. WY conceived and wrote the manuscript. MC edited the manuscript. All authors approved the submitted version.
This study was financially supported by the National Natural Science Foundation of China (Grant Nos. 42076030 and 41476061 to WY). Data and samples were collected onboard of R/V “Yanping 2” implementing the open research cruise NORC2016-04 supported by NSFC Shiptime Sharing Project (Grant No. 41549904).
The authors declare that the research was conducted in the absence of any commercial or financial relationships that could be construed as a potential conflict of interest.
All claims expressed in this article are solely those of the authors and do not necessarily represent those of their affiliated organizations, or those of the publisher, the editors and the reviewers. Any product that may be evaluated in this article, or claim that may be made by its manufacturer, is not guaranteed or endorsed by the publisher.
We thank the reviewers and editors for their constructive suggestions. We also thank YQ for his technical assistance during field observation and crew members of the R/V Yanping 2 for their help during sample collection.
Anand, S. S., Rengarajan, R., and Sarma, V. V. S. S. (2018). 234Th-based carbon export flux along the Indian GEOTRACES LI02 section in the Arabian Sea and the Indian Ocean. Glob. Biogeochem. Cy. 32, 417–436. doi: 10.1002/2017GB005847
Bacon, M. P., Cochran, J. K., Hirschberg, D., Hammar, T. R., and Fleer, A. P. (1996). Export flux of carbon at the equator during the EqPac time-series cruises estimated from 234Th measurements. Deep Sea Res. II 43, 1133–1153. doi: 10.1016/0967-0645(96)00016-1
Baker, C. A., Estapa, M. L., Iversen, M., Lampitt, R., and Buesseler, K. O. (2020). Are all sediment traps created equal? An intercomparison study of carbon export methodologies at the PAP-SO site. Progr. Oceanogr. 184:102317. doi: 10.1016/j.pocean.2020.102317
Benitez-Nelson, C. R., Buesseler, K. O., Rutgers van der Loeff, M. M., Andrews, J., Ball, L., Crossin, G., et al. (2001). Testing a new small-volume technique for determining 234Th in seawater. J. Radioanal. Nucl. Chem. 248, 795–799. doi: 10.1023/A:1010621618652
Black, E. E., Buesseler, K. O., Pike, S. M., and Lam, P. J. (2018). 234Th as a tracer of particulate export and remineralization in the southeastern tropical Pacific. Mar. Chem. 201, 35–50. doi: 10.1016/j.marchem.2017.06.009
Buesseler, K. O., Bacon, M. P., Cochran, J. K., and Livingston, H. D. (1992). Carbon and nitrogen export during the JGOFS North Atlantic bloom experiment estimated from 234Th:238U disequilibria. Deep-Sea Res. I 39, 1115–1137. doi: 10.1016/0198-0149(92)90060-7
Buesseler, K. O., Benitez-Nelson, C. R., Roca-Martí, M., Wyatt, A. M., Resplandy, L., Clevenger, S. J., et al. (2020). High-resolution spatial and temporal measurements of particulate organic carbon flux using 234Th in the northeast Pacific Ocean during the export processes in the ocean from RemoTe Sensing field campaign. Elem. Sci. Anth. 8:1. doi: 10.1525/elementa.030
Buesseler, K. O., Pike, S., Maiti, K., Lamborg, C. H., Siegel, D. A., and Trull, T. W. (2009). 234Th as a tracer of spatial, temporal and vertical variability in particle flux in the North Pacific. Deep Sea Res. I 56, 1143–1167. doi: 10.1016/j.dsr.2009.04.001
Cai, P., Chen, W., Dai, M., Wan, Z., Wang, D., Li, Q., et al. (2008). A high-resolution study of particle export in the southern South China Sea based on 234Th:238U disequilibrium. J. Geophys. Res. 113:C04019. doi: 10.1029/2007JC004268
Cai, P., Zhao, D., Wang, L., Huang, B., and Dai, M. (2015). Role of particle stock and phytoplankton community structure in regulating particulate organic carbon export in a large marginal sea. J. Geophys. Res. Oceans 120, 2063–2095. doi: 10.1002/2014JC010432
Chow, C. H., Shih, Y.-Y., Chien, Y.-T., Chen, J. Y., Fan, N., Wu, W.-C., et al. (2021). The wind effect on biogeochemistry in eddy cores in the northern South China Sea. Front. Mar. Sci. 8:717576. doi: 10.3389/fmars.2021.717576
Coppola, L., Roy-Barman, M., Wassmann, P., Mulsow, S., and Jeandel, C. (2002). Calibration of sediment traps and particulate organic carbon export using 234Th in the Barents Sea. Mar. Chem. 80, 11–26. doi: 10.1016/S0304-4203(02)00071-3
Feng, N., Yang, W., Zhao, X., Chen, M., Qiu, Y., and Zheng, M. (2021a). Seasonal export of 234Th and POC in Daya Bay, northern South China Sea. Cont. Shelf Res. 216:104359. doi: 10.1016/j.csr.2021.104359
Feng, N., Yang, W., Zhao, X., Chen, M., Qiu, Y., and Zheng, M. (2021b). Semi-enclosed bays server as hotspots for black carbon burial: a case study in Jiaozhou Bay, western Yellow Sea. Sci. Total Environ. 797:149100. doi: 10.1016/j.scitotenv.2021.149100
Gan, J., Li, H., Curchitser, E. N., and Haidvogel, D. B. (2006). Modeling South China Sea circulation: response to seasonal forcing regimes. J. Geophys. Res. 111:C06034. doi: 10.1029/2005JC003298
Ge, Z., Li, Q. P., Yang, W., Liu, X., and Wu, Z. (2022). Transparent exopolymer particle dynamics along a shelf-to-sea gradient and impacts on the regional carbon cycle. Sci. Total Environ. 808:152117. doi: 10.1016/j.scitotenv.2021.152117
Hong, Q., Peng, S., Zhao, D., and Cai, P. (2021). Cross-shelf export of particulate organic carbon in the northern South China Sea: insights from a 234Th mass balance. Progr. Oceanogr. 193:102532. doi: 10.1016/j.pocean.2021.102532
Hung, C.-C., Chen, Y.-F., Hsu, S.-C., Wang, K., Chen, J., and Burdige, D. J. (2016). Using rare earth elements to constrain particulate organic carbon flux in the East China Sea. Sci. Rep. 6:33880. doi: 10.1038/srep33880
Hung, C.-C., and Gong, G.-C. (2010). POC/234Th ratios in particles collected in sediment traps in the northern South China Sea. Estuar. Coast. Shelf Sci. 88, 303–310. doi: 10.1016/j.ecss.2010.04.008
Hung, C.-C., Gong, G.-C., and Santschi, H. (2012). 234Th in different size classes of sediment trap collected particles from the Northwestern Pacific Ocean. Geochim. Cosmochim. Acta 91, 60–74. doi: 10.1016/j.gca.2012.05.017
Hung, C.-C., Xu, C., Santschi, P. H., Zhang, S.-J., Schwehr, K. A., Quigg, A., et al. (2010). Comparative evaluation of sediment trap and 234Th-derived POC fluxes from the upper oligotrophic waters of the Gulf of Mexico and the subtropical northwestern Pacific Ocean. Mar. Chem. 121, 132–144. doi: 10.1016/j.marchem.2010.03.011
Jia, Y., Tian, Z., Shi, X., Liu, J. P., Chen, J., and Liu, X. (2019). Deep-sea sediment resuspension by internal solitary waves in the Northern South China Sea. Sci. Rep. 9:12137. doi: 10.1038/s41598-019-47886-y
Li, D., Chou, W.-C., Shih, Y.-Y., Chen, G.-Y., Chang, Y., Chow, C. H., et al. (2018). Elevated particulate organic carbon export flux induced by internal waves in the oligotrophic northern South China Sea. Sci. Rep. 8:2042. doi: 10.1038/s41598-018-20184-9
Ma, H., Yang, W., Zhang, L., Zhang, R., Chen, M., Qiu, Y., et al. (2017). Utilizing 210Po deficit to constrain particle dynamics in mesopelagic water, western South China Sea. Geochem. Geophys. Geosyst. 18, 1594–1607. doi: 10.1002/2017GC006899
Maiti, K., Bosu, S., Dsa, E. J., Adhikari, P. L., Sutor, M., and Longnecker, K. (2016). Export fluxes in northern Gulf of Mexico–comparative evaluation of direct, indirect and satellite-based estimates. Mar. Chem. 184, 60–77. doi: 10.1016/j.marchem.2016.06.001
Martin, P., Lampitt, R. S., Perry, J. J., Sanders, R., Lee, C., and D’Asaro, E. (2011). Export and mesopelagic particle flux during a North Atlantic spring diatom bloom. Deep Sea Res. I 58, 338–349. doi: 10.1016/j.dsr.2011.01.006
Owens, S. A., and Buesseler, K. O. (2015). 234Th as a tracer of particle dynamics and upper ocean export in the Atlantic Ocean. Deep Sea Res. II 116, 42–59. doi: 10.1016/j.dsr2.2014.11.010
Owens, S. A., Buesseler, K. O., and Sims, K. W. W. (2011). Re-evaluating the 238U salinity relationship in seawater: implications for the 238U-234Th disequilibrium method. Mar. Chem. 127, 31–39. doi: 10.1016/j.marchem.2011.07.005
Qiu, Y. (2018). Regulatory Mechanism of Plankton Community Structure on Particulate Organic Carbon Export in Different Ecosystems of Subtropical Marginal Seas. Ph.D. Dissertation. Xiamen: Xiamen University, 42–65.
Resplandy, L., Martin, A. P., Le Moigne, F., Martin, P., Aquilina, A., Mémery, L., et al. (2012). How does dynamical variability impact 234Th-derived estimates of organic export? Deep Sea Res. I 68, 24–45. doi: 10.1016/j.dsr2012.05.015
Roca-Martí, M., Puigcorbé, V., Iversen, M. H., Rutgers van der Leoff, M., Klaas, C., Cheah, W., et al. (2017). High particulate organic carbon export during the decline of a vast diatom bloom in the Atlantic sector of the Southern Ocean. Deep Sea Res. II 138, 102–115. doi: 10.1016/j.dsr2.2015.12.007
Savoye, N., Benitez-Nelson, C., Burd, A. B., Cochran, J. K., Charette, M., Buesseler, K. O., et al. (2006). 234Th sorption and export models in the water column: a review. Mar. Chem. 100, 234–249. doi: 10.1016/j.marchem.2005.10.014
Schroeder, A., Wiesner, M. G., and Liu, Z. (2015). Fluxes of clay minerals in the South China Sea. Earth Planet. Sci. Lett. 430, 30–42. doi: 10.1016/j.epsl.2015.08.001
Shen, J., Jiao, N., Dai, M., Wang, H., Qiu, G., Chen, J., et al. (2020). Laterally transported particles from margins serve as a major carbon and energy source for dark ocean ecosystems. Geophys. Res. Lett. 47:e2020GL088971. doi: 10.1029/2020GL088971
Shih, Y.-Y., Hung, C.-C., Tuo, S.-H., Shao, H.-J., Chow, C. H., Muller, F. L. L., et al. (2020). The impact of eddies on nutrient supply, diatom biomass and carbon export in the northern South China Sea. Front. Earth Sci. 8:537332. doi: 10.3389/feart.2020.537332
Shih, Y.-Y., Lin, H.-H., Li, D., Hsieh, H.-H., Hung, C.-C., and Chen, C.-T. A. (2019). Elevated carbon flux in deep waters of the South China Sea. Sci. Rep. 9:1496. doi: 10.1038/s41598-018-37726-w
Shih, Y.-Y., Shiah, F.-K., Lai, C.-C., Chou, W.-C., Tai, J.-H., Wu, Y.-S., et al. (2021). Comparison of primary production using in situ and satellite-derived values at the SEATS station in the South China Sea. Front. Mar. Sci. 8:747763. doi: 10.3389/fmars.2021.747763
Umhau, B. P., Benitez-Nelson, C. R., Close, H. G., Hannides, C. S., Motta, L., Popp, B. N., et al. (2019). Seasonal and spatial changes in carbon and nitrogen fluxes estimated using 234Th:238U disequilibria in the North Pacific tropical and subtropical gyre. Mar. Chem. 217:103705. doi: 10.1016/j.marchem.2019.103705
Wang, D., Wang, Q., Cai, S., Shang, X., Peng, S., Shu, Q., et al. (2019). Advances in research of the mid-deep South China Sea circulation. Sci. China Earth Sci. 62, 1992–2004. doi: 10.1007/s11430-019-9546-3
Yang, W., Chen, M., Zheng, M., He, Z., Zhang, X., Qiu, Y., et al. (2015). Influence of a decaying cyclonic eddy on biogenic silica and particulate organic carbon in the tropical South China Sea based on 234Th-238U disequilibrium. PLoS One 10:e0136948. doi: 10.1371/journal.pone.0136948
Yang, W., Huang, Y., Chen, M., Qiu, Y., Peng, A., and Zhang, L. (2009). Export and remineralization of POM in the Southern Ocean and the South China Sea estimated from 210Po/210Pb disequilibria. Chin. Sci. Bull. 54, 2118–2123. doi: 10.1007/s11434-009-0043-4
Yang, W., Zhang, X., Chen, M., Fang, Z., and Qiu, Y. (2021a). Utilizing 234Th/238U disequilibrium to constrain particle dynamics in hydrothermal plumes in the Southwest Indian Ocean. Acta Oceanol. Sin. 40, 16–25. doi: 10.1007/s13131-021-1786-2
Yang, W., Zhao, X., Guo, L., Huang, B., Chen, M., Fang, Z., et al. (2021b). Utilization of soot and 210Po-210Pb disequilibria to constrain POC fluxes in the northeastern South China Sea. Front. Mar. Sci. 8:694428. doi: 10.3389/fmars.2021.694428
Yang, W., Zhang, X., Chen, M., and Qiu, Y. (2016). Unusually low 234Th in a hydrothermal effluent plume over the Southwest Indian Ridge. Geochem. Geophys. Geosyst. 17, 3815–3824. doi: 10.1002/2016GC006580
Zhao, X., Yang, W., Ma, H., Li, J., Chen, M., Fang, Z., et al. (2019). Seasonal variations in the abundance and sinking flux of biogenic silica in Daya Bay, northern South China Sea. Oceanologia 61, 239–251. doi: 10.1016/j.oceano.2018
Keywords: thorium-234, mesopelagic water, export flux, resuspension, South China Sea
Citation: Yang W, Zhao X, Chen M, Qiu Y and Zheng M (2022) Lagrangian Observation of 234Th and Its Application in Constraining the Sinking of Particulate Organic Carbon on the Slope of the Northeastern South China Sea. Front. Mar. Sci. 9:831937. doi: 10.3389/fmars.2022.831937
Received: 09 December 2021; Accepted: 27 January 2022;
Published: 18 February 2022.
Edited by:
Jixin Qiao, Technical University of Denmark, DenmarkReviewed by:
Chin-Chang Hung, National Sun Yat-sen University, TaiwanCopyright © 2022 Yang, Zhao, Chen, Qiu and Zheng. This is an open-access article distributed under the terms of the Creative Commons Attribution License (CC BY). The use, distribution or reproduction in other forums is permitted, provided the original author(s) and the copyright owner(s) are credited and that the original publication in this journal is cited, in accordance with accepted academic practice. No use, distribution or reproduction is permitted which does not comply with these terms.
*Correspondence: Weifeng Yang, d3lhbmdAeG11LmVkdS5jbg==
Disclaimer: All claims expressed in this article are solely those of the authors and do not necessarily represent those of their affiliated organizations, or those of the publisher, the editors and the reviewers. Any product that may be evaluated in this article or claim that may be made by its manufacturer is not guaranteed or endorsed by the publisher.
Research integrity at Frontiers
Learn more about the work of our research integrity team to safeguard the quality of each article we publish.