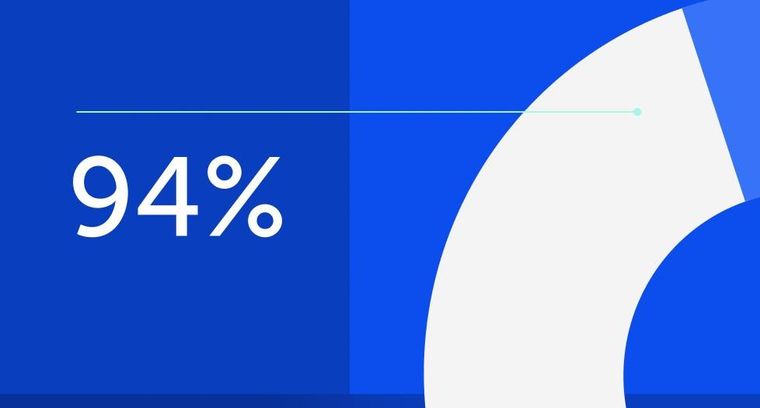
94% of researchers rate our articles as excellent or good
Learn more about the work of our research integrity team to safeguard the quality of each article we publish.
Find out more
ORIGINAL RESEARCH article
Front. Mar. Sci., 13 June 2022
Sec. Marine Biology
Volume 9 - 2022 | https://doi.org/10.3389/fmars.2022.830425
This article is part of the Research TopicEffects of Environmental Stress on Marine Mollusks: Biomarkers, Physiological Responses and Underlying MechanismsView all 7 articles
Water temperature is a major abiotic driver, controlling the rates and nature of biochemical reactions and subsequently affecting the physiology of marine organisms. However, relatively little is known about the implications of heat stress or predicted ocean climate change on marine secondary metabolites. The predatory gastropod Dicathais orbita is a useful model organism for climate change and natural product studies. Here we determine the upper thermal limit (CTMax) of D. orbita and investigate the effects of thermal stress on the bioactive compounds stored in their biosynthetic organ, the hypobranchial gland. Two CTMax experiments were undertaken, along with a static heat stress experiment where whelks were exposed to an elevated temperature of 30°C for one week, compared to a 20°C seawater control. An additional 35-day ocean climate change experiment used combinations of temperature (ambient: 23°C and future: 25°C) and pCO2 (ambient: ~380 ppm and future: ~765 ppm). The impacts on secondary metabolites in all experiments were assessed using liquid chromatography-mass spectrometry. The mean CTMax of the whelks, from the northern limit of their distribution, was found to be 35.2°C using a rapid temperature increase rate of 1°C/1 h, but was only 30.6°C when a gradual heating rate of 1°C/12 h was used. The overall composition of the secondary metabolites was significantly affected by heat stress in all four experiments, but not by elevated pCO2 in the ocean climate change experiment. The proportion of the choline ester murexine was significantly reduced in heat-stressed snails compared to the controls. Tyrindoxyl sulphate was significantly reduced under prolonged exposure to future temperature, whereas the relative abundance of the oxidation product, 6-bromoisatin significantly increased with elevated temperature exposure. Despite the fact that intertidal gastropods like D. orbita might be able to buffer the impact of external temperatures within the predicted future range, this study provides evidence that ocean warming could have significant implications for secondary metabolite production and/or storage in marine invertebrates. Impacts on bioactive molecules with multifunctional ecological roles could have implications for predator populations with possible flow on effects in some marine communities.
There is growing appreciation for the biomedical value and multiple ecological roles of marine natural products. Secondary metabolites are organic compounds that are not essential to growth, reproduction or development, but typically have a selective advantage by mediating ecological interactions and increasing the survivorship or fecundity of the species. In mollusks, chemically diverse secondary metabolites function as venoms for predation (Roseghini et al., 1996; Olivera, 2002), defense against predators (Pawlik, 1993; Berenbaum, 1995; Pawlik, 2012) and pathogens (Benkendorff et al., 2001; Dang et al., 2015), regulators of reproduction (Cimino and Sodano, 2005; Rudd et al., 2015b) and as pheromones (Cimino et al., 1989; Cimino et al., 1991; Cummins et al., 2004). Some secondary metabolites, appear to be “molecules of keystone significance”, vital for influencing the behavior and functioning of keystone species (Derby and Aggio, 2011; Di Cosmo and William, 2014; Zimmer et al., 2017). Impacts on the biosynthesis or bioavailability of these secondary metabolites could alter population structure, diversity, and function of marine systems. In some cases, bioactive molecules have more than one specific ecological function (Derby and Aggio, 2011; Benkendorff, 2014) and their associated biological activity can be useful for human applications, such as medicine e.g. (Shinde et al., 2019; Summer et al., 2020). Therefore, there is a need for a deeper understanding on how marine mollusks respond to environmental stresses and how these threats may affect their secondary metabolism.
Temperature is one of the most pervasive abiotic variables that influences all life forms. The average sea surface temperature (SST) has increased over recent decades and is predicted to continue rising, along with increased duration and intensity of extreme weather events, such as marine heatwaves (Frölicher et al., 2018; IPCC, 2019). Marine heatwaves and ocean warming can impact the physiology, survivorship and distribution of marine organisms (Helmuth et al., 2002; Garrabou et al., 2009; Smale et al., 2019) and result in changes to ecological community structure (Mouthon and Daufresne, 2006; Wernberg et al., 2013). Even minor variations in ambient temperature can impact growth, development and survivorship by affecting the physiological setting of an organism (Helmuth et al., 2005; Helmuth et al., 2010; Tattersall et al., 2012). At elevated temperatures, increased metabolic rates require higher oxygen uptake and energy consumption (Portner, 2001). At the biochemical level, thermal stress may result in a suite of metabolic changes. For example, increased mortality coincided with altered metabolic pathways in heat-stress abalone, including enhanced gluconeogenesis, and alterations in adenosine nucleotide and some amino acid concentrations (Lu et al., 2016). Ocean warming has been shown to negatively affect the nutritional quality of mollusks, causing a reduction in protein, lipid and essential omega-3 fatty acids (Valles-Regino et al., 2015; Tate et al., 2017; Ab Lah et al., 2018; Lemasson et al., 2019). These biochemical changes in response to thermal stress are expected to reduce the overall fitness of marine organisms and could have consequences for their bioresource value to humans.
Anthropogenic carbon dioxide (CO2) emissions resulting in ocean warming is occurring simultaneously with the ocean acidification (OA), a phenomenon that results in the gradual reduction of seawater pH due to increased dissolution of CO2 in surface waters (Caldeira and Wickett, 2005; IPCC, 2013). Ocean warming and acidification have been extensively studied, independently or combined, on the reproduction, growth, calcification, population abundance and behavior of a wide variety of marine invertebrates (e.g., reviews by Byrne, 2011; Byrne and Przeslawski, 2013; Kroeker et al., 2013). Mollusks are known to be among the most sensitive marine organisms to ocean warming and acidification (Pörtner, 2002; Przeslawski et al., 2008a; Doney et al., 2009; Byrne, 2011; Hale et al., 2011; Gazeau et al., 2013; Fortunato, 2015), hence they are good models for climate change studies. Primary metabolites in mollusks can also be impacted by ocean acidification. The abundance of proteins involved in carbohydrate and lipid metabolism was reported to be significantly affected by high levels of CO2 in Pacific oyster Magallana (Crassostrea) gigas (Timmins-Schiffman et al., 2014). Furthermore, total protein, lipids and both saturated and monounsaturated fatty acids in the marine whelk Dicathais orbita were significantly reduced with elevated CO2 concentration (Valles-Regino et al., 2015; Tate et al., 2017). These studies indicate that pCO2-induced acidification can be an important determining factor for metabolism and may exacerbate the effects of ocean warming in some species.
Studies on primary metabolites indicate that temperature stress and pCO2-induced acidification are important abiotic factors influencing marine physiology and metabolism, which could therefore also impact the biosynthesis of bioactive compounds. The evidence that temperature stress can affect bioactive secondary metabolites has focused mostly on plants and bacteria (e.g Doull et al., 1993; Malin and Lapidot, 1996; Mori et al., 2007; Wahid, 2007). Nevertheless, one in-depth metabolomic study on sea cucumbers has revealed changes in 84 metabolites under heat stress, including the antibiotic gentamicin and the antineoplastic compound Epothilone A, along with many changes to amino acid biosynthesis, carbohydrate and lipid metabolism (Huo et al., 2019). Few studies have specifically investigated the effects of CO2-induced acidification on secondary metabolism, with only marine plants (e.g. Arnold et al., 2012) and algae (e.g. Swanson and Fox, 2007; Kerrison et al., 2012; Borell et al., 2013; Figueroa et al., 2014) receiving much of the research attention and with variable results. However, very little is currently known about the potential impacts of ocean climate change on secondary metabolism in mollusks.
The Muricidae are a family of predatory marine neogastropods, commonly known as murex snails or rock whelks. They are well-known for the production of a purple dye, 6,6-dibromoindigo, also known as Tyrian purple (Baker, 1974) or Shellfish purple (Cooksey, 2006). The Australian species Dicathais orbita has been identified as a useful model species for both natural products (Benkendorff, 2013) and ocean climate change research (Valles-Regino et al., 2015; Tate et al., 2017). D. orbita produces a single precursor to shellfish purple, the brominated indole tyrindoxyl sulphate, which is held as a salt of the choline ester murexine (Baker and Duke, 1976). The precursor is hydrolysed by an arylsulphatase enzyme to form a suite of bioactive choline esters and brominated indoles, which subsequently undergo photolytic reactions and oxidation (Cooksey, 2001; Benkendorff et al., 2015). These secondary metabolites are of biomedical (Benkendorff et al., 2015; Ahmad et al., 2017; Rudd et al., 2019; Rahman et al., 2021) and historical interest (Cooksey, 2006; Cooksey, 2013), as well as playing ecological roles in the reproduction, predation and defense of the snails (Benkendorff et al., 2000; Westley et al., 2006; Rudd et al., 2015b).
Muricids are important predators on intertidal and shallow subtidal reefs (Durán and Castilla, 1989; Morton, 2008) and are known to be physiologically impacted by ocean climate change (Valles-Regino et al., 2015; Manríquez et al., 2016; Tate et al., 2017; Détrée et al., 2020). Marine invertebrates inhabiting intertidal and shallow rocky reefs often live close to their critical thermal limit (Helmuth et al., 2002; Somero, 2002; Somero, 2010). These environments are exposed to the most extreme variations in temperature, daily and seasonally, particularly in temperate regions (Helmuth, 1999; Madeira et al., 2012). Because of their thermal sensitivity, ectotherms from rocky intertidal zones are considered good models for investigating the biological effects of climate change (Southward et al., 1995; Ab Lah et al., 2017). The coastal waters of south east Australia have been identified as an ocean climate hotspot, with actual and projected increases of temperature three times faster than the global average (Beger et al., 2011). Under these extreme conditions, ectotherms will have to adapt quickly to ocean warming, or there will be consequences for the long-term survivorship of populations (Guerrero et al., 2013) and/or shifts in their range distributions (Przeslawski et al., 2008b). In the southern hemisphere, range contractions are most likely at the northern limit of the species distribution.
The aims of this study were threefold. Firstly, we investigated the upper thermal limit of D. orbita from near the northern limits of its distribution by exposing the whelks to elevated water temperature in a controlled laboratory environment to establish the critical thermal maxima based on loss of foot muscle attachment strength. Secondly, using specimens from these dynamic temperature experiments, as well as a complementary static controlled temperature experiment, we investigated the impact of elevated temperature stress on the secondary metabolite composition of D. orbita. Finally, we used a 35-day ocean climate change experiment in a flow through seawater system to investigate the impacts of future ocean warming and pCO2 induced acidification on the secondary metabolites. These results provide insights into the sublethal impacts of ocean warming on marine species, with implications for the sustainable production of marine bioactive compounds.
Four separate experiments were undertaken to investigate the effects of heat stress on D. orbita. The number of snails collected for each experiment, the experimental design and the numbers used for secondary metabolism extraction are summarised in Table 1.
Table 1 Summary of the experimental design and number of independent replicates analyzed for testing the effects of heat stress on Dicathais orbita secondary metabolites across four separate manipulative experiments.
For the first three experiements, a total of 120 D. orbita individuals were collected by hand from the intertidal reefs of Shelly Beach, East Ballina, New South Wales (NSW), Australia (28°51’56.7”S 153°35’39.3”E) and Angels Flat Rock, East Ballina, NSW, Australia (28°50’33.4”S 153°36’30.1”E) under NSW Fisheries Permit # P13/0002-1.0 and F89/1171-6.0. Immediately following collection, the snails were transported to Southern Cross University, Lismore, NSW, Australia, in seawater and transferred to a 200L recirculating holding tank at temperatures corresponding to their source water for one week before different temperature treatments were manipulated. The acclimatisation tank was connected to a filter tank, equipped with bio-balls and pump (Resun King 3 AF, Fountain Pump, Sydney, NSW, Australia) for water recirculation.
For the longer-term ocean acidification and warming experiment 144 adult D. orbita individuals were collected from intertidal reefs in Coffs Harbour, New South Wales, Australia during the low austral winter tides. These were transferred to mesocosms at the National Marine Science Centre (NMSC) (30° 16’3.70”S, 153° 8’15.31”E) and held in a flow through seawater system for two weeks acclimatization under ambient conditions prior to the experiment.
During the acclimatisation and experimental periods for all experiments, temperature, salinity and pH were measured twice daily. Salinity, measured using a Eutech Instruments Salinity Meter Cond 6+ (Sydney, NSW, Australia), ranged from 35-36 ppt. S Eutech Instruments EcoScan pH/mV/°C meter (Sydney, NSW, Australia) was used to monitor the seawater pH (8.1-8.2). Temperature was recorded using a mercury thermometer. The bottom of the tanks was siphoned every day with ~ 30% of the seawater replenished in each tank. During acclimatization, the whelks were fed Lunella undulata for a minimum of 5 days ad libitum, then starved 24hr prior to transfer into the experimental tanks. The snails were not fed during short-term heat stress experiments in static tanks. However, during the longer-term ocean acidification and warming mesocosm experiment, the whelks were fed Sydney rock oysters (Saccostrea glomerata) ad libitum for the duration of the experiment. All tanks were checked daily for mortality.
In order to assess the sublethal effects of temperature stress on biological processes, the tolerable temperature range of the model species first needs to be established. Critical thermal maximum (CTMax) is often used to evaluate the thermal resistance of ectothermic mollusks (Diaz et al., 2006; Diaz et al., 2013; Ab Lah et al., 2017) and is defined as the temperature for a particular species at which its motor coordination becomes uncontrolled or disorganised, subjecting the animal to likely death (Diaz et al., 2006). CTMax experiments were undertaken according to the general protocols of Diaz et al. (2011, 2006, 2000) with modifications according to Ab Lah et al. (2017). These experiments involved a dynamic method where the temperature of the seawater was gradually increased until the snails under investigation showed symptoms of thermal stress, specifically the complete loss of grip of the foot muscle from the substrate (wall of the glass tank), such that the snails became detached but without mortality. Two CTMax experiments using different individuals were conducted to assess the critical thermal limit in D. orbita (Table 1). One was a short-term experiment with a fast warming rate of 1°C increase for every one-hour temperature interval. The other one was a longer-term experiment with a slow warming rate of 1°C rise in temperature for every 12 hours. The one-hour thermal increment experiments have been conducted in previous CTMax studies on other marine gastropods (e.g. abalone Gilroy and Edwards, 1998) and the 12-hour thermal experiments have been recently applied to turban snails (Ab Lah et al., 2017).
Eight snails were randomly transferred to each of three control tanks and three experimental tanks (12.5 cm x 22.5 cm x 25.5 cm), which all contained a separate glass plate (22 cm x 45 cm) placed horizontally on the bottom. To determine the health condition of the snails, they were first placed in an inverted position and were left to upright themselves (Ab Lah et al., 2017) within three to five minutes; otherwise, they were immediately replaced with other individuals (6/96). The snails were then left undisturbed for 12 hours, after which time the glass plates were vertically suspended. Each tank was provided with constant aeration, a thermometer and a Sonpar automatic heater HA-50W. Floating polystyrene sheets with holes, where the heater and thermometer were inserted, were used for insulation to stabilize the temperature gradient due to the interface with the surroundings (Mora and Maya, 2006). These floating cover sheets also ensured that no snails could crawl up and escape out of the heated seawater during the experiment. The control tanks also contained water heaters but these were set on the ambient temperature of 20°C and were not increased during the experiment. The seawater in the treatment tanks was slowly heated by manually increasing the temperature by 1.0 ± 0.5°C every one hour for the CTMax short-term experiment, which was performed between 0600 to 2300 hours. The time, temperature and the number of snails that lost attachment were recorded every hour.
The same procedure was used for the CTMax longer-term experiment except that the tanks were placed in a Labec BLC 20 water bath (Marrickville, NSW, Australia) with a digital thermostat. The control tanks were maintained at 20°C for the entire duration of the experiment. The treatment tanks commenced at 20°C but were incrementally increased by 1°C every 12 hours. The number of snails that lost attachment to the horizontal glass plate was recorded. This experiment ran for seven days.
At the end of each experiment, at least one snail per tank that lost their grip at each temperature increment on or above the CTMax, were individually tagged before storing in a −20°C freezer, along with the same number of snails from control tanks. The remaining whelks were kept in aerated tanks for a week to check whether they were able to recuperate (active, responsive and able to upright within 5 min). To provide replicate samples above the CTMax for chemical extraction and analysis of the secondary metabolites, four snails that lost attachment at 37°C in the short term CTMax experiment and three snails that lost attachment at 31°C in the longer-term experiment were randomly selected, defrosted and dissected, along with corresponding controls (Table 1).
Eighteen snails were acclimatized in a large tank with constant aeration for one week then randomly allocated to each of six tanks (Table 1). The same 7 L experimental tanks were used as for the CTMax experiments and siphoned daily with water replacement. Three tanks were used as the controls (ambient seawater temperatures set at 20 °C based on local conditions) and three other tanks were used as the elevated temperature treatments, starting at 20 °C and increasing to 30 °C over a 24-h period, then held at 30°C for a period of one week (based on the CTMax from the previous slower warming rate experiment). The treatment tanks were supplied with a Sonpar automatic heater for a uniform heating rate and to guarantee that the temperature did not exceed the desired limit. Tanks were also covered with floating polystyrene sheets with holes. Snails were regularly monitored (at least every eight hours). After the experiment, one to two snails per tank were selected for dissection of the hypobranchial glands, with the remaining snails left to recover at ambient temperature. In total four independent replicate extracts were analysed from the treatment and control tanks (Table 1).
The mesocosm design and monitoring for this experiment has been described in detail in Valles-Regino et al. (2015) and Tate et al. (2017) and flow-through seawater system filtered at 50 μm. D. orbita were exposed to four treatments in a two-factorial design: 1) ambient conditions, 22.9 ± 0.6°C and 378.6 ± 35.6 ppm; 2) elevated temperature, 25.2 ± 0.6°C and 382.2 ± 35.5 ppm; 3) elevated pCO2 = 22.9 ± 0.5°C and 749.9 ± 80.6 ppm; and 4) increased temperature 25.3 ± 0.6°C and elevated pCO2 = 763.0 ± 104.6 ppm. There were twelve 1100L header tanks where temperature was controlled using heater chiller units (Aquahort Ltd, OmanaBeach NZ) and pH was manipulated by bubbling CO2 enriched air via a gas mixer (PEGAS 4000MF), with three replicate header tanks for each temperature and pCO2 combination. Seawater flowed from each header tank at 3 Lmin−1 into trays (860 × 650 × 96 mm), each containing 12 D. orbita, divided equally into four mesh cages (305 mm × 360 mm × 90 mm), to prevent escape. After 35-day exposure to the experimental conditions, three whelks were randomly selected from separate cages in each replicate tray for dissection, extraction and chemical analysis. However, due to the degradation of some samples during dissection and extraction (see Liquid Chromatography-Mass Spectrometry (LC-MS) Analysis), the final number of replicate extracts per treatment was reduced to n = 8 per temperature x pCO2 treatment by randomly excluding some samples for a balanced design (Table 1).
The water temperature, pH, conductivity and salinity were measured daily and total alkalinity was measured weekly through potentiometric titration using an automated titrator (888 Titrando, Metrohom, Riverview, FL, USA). This data was used to calculate the partial pressure of CO2 using the CO2SYS program (Pierrot et al., 2006) according to Tate et al. (2017).
The dissection, chemical extraction and analysis of the hypobranchial glands of D. orbita followed the validated procedure of Valles-Regino et al. (2016). The shell of the snail was removed by rupturing with a bench-top vice. The whole soft tissue of the snail was transferred to a dish and the hypobranchial gland was identified according to Westley et al. (2010), excised with surgical scissors, and weighed on an analytical balance (Mettler Toledo ML 204 Greifensee, Switzerland, with precision ±0.001g). Each gland was homogenised using a mortar and pestle and extracted using 15 mL of analytical grade ethanol (Sigma-Aldrich, Castle Hill, NSW, Australia) at 4°C in the dark according to Valles-Regino et al. (2016). The mixture was then filtered using Sigma-Aldrich Whatman filter paper 90 mm and concentrated to dryness on a rotary evaporator (R-114 Buchi Vacuum System, Buchi Labortechnik AG, Flawil, Switzerland) at 40°C under reduced pressure, dried further under a stream of 100% nitrogen gas, then weighed and stored at −20°C until analysed.
An Agilent (Santa Clara, CA, USA) 1260 Infinity High Performance Liquid Chromatography (HPLC) equipped with a 6120 Quad mass spectrometer was used for the analysis of the ethanol extracts. The secondary metabolites were separated on a Phenomenex luna C18 (100 x 4.6 mm) using a gradient mobile phase of 20% to 95% acetonitrile (Scharlau supragradient HPLC grade) and Milli-Q water whilst keeping the flow rate at 0.5 mL/min for 18 mins. Trifluoroacetic acid 0.005% (TFA, Sigma Aldrich, Castle Hill, NSW, Australia) was added to both solvents to increase the separation efficiency. The samples were run using electrospray ionisation-mass spectrometry (ESI-MS) in both positive and negative ion modes, with the mass detector set to scan from 100-1000 mass units. Selective ion monitoring for 224/226 ions was also undertaken as these fragment ions are characteristic of a range of brominated indoles produced by D. orbita (Valles-Regino et al., 2016).
The secondary metabolites that were identified and quantified from the extracts are summarised in Table 2. Agilent ChemStation (2000-2-11, Agilent Technologies, Mulgrave, Vic, Australia) was used for data analysis and integration of the compound peaks in the chromatographs. Brominated compounds were identified by the characteristic major doublet and triplet mass ion clusters for the 79Br, 81Br and all secondary metabolites were identified by the comparison of retention times, UV profiles and specific mass spectral fragmentation patterns by comparison to published studies on D. orbita hypobranchial glands (Westley and Benkendorff, 2008; Rudd and Benkendorff, 2014; Valles-Regino et al., 2016). Compounds were quantified using standard curves with purified reference compounds for the precurors tyrindoxyl sulphate and murexine, as well as commercially available 6-bromoisatin (Valles-Regino et al., 2016). Tyrian purple 6,6’ dibromoindigo was not quantified due to the insoluable nature of this compound and extracts with high levels of this compound (>5% of the extract) were considered degraded and not used in the statistical analyses.
Table 2 Details of the secondary metabolites analyzed in the extracts of Dicathais orbita using liquid chromatography (LC) - Mass spectrometry (MS).
The mean CTMax was determined by computing the average temperature at which snails lost attachment from each tank. Median CTMax, the temperature at which 50% of the whelks were detached from the glass, was calculated by Probit analysis followed by nonlinear regression analysis (by fitting a sigmoidal curve) using IBM SPSS Statistics 22. Independent T-tests using IBM SPSS Statistics version 22 (Chicago, IL, USA) were used to compare the differences in mean CTMax between fast and slow warming rates.
Multivariate analyses of the chemical composition in the hypobranchial glands were undertaken using PRIMER v 6.1.13 and PERMANOVA+ v 1.0.3 add-on (PRIMERe Pty. Ltd., Plymouth, UK). Euclidean distance similarity matrices were constructed and 9999 permutations were run to identify differences in the quantity of the secondary metabolites in the hypobranchial gland extracts between control and heat-treated snails from the CT-max and static heat experiments. For the ocean warming and acidification experiment, initially, a three-factor analysis was run on the multivariate data with tank (random) nested in temperature and pH, but due to the lack of tank effects, the model was reduced to the main factors generating eight replicates per treatment group. For the visualization of composition patterns in the multivariate data, principal component ordination (PCO) was used with vector overlay using Pearson correlation > 0.9. For the analysis of the total extract yield and individual secondary metabolites, univariate PERMANOVAs were undertaken to compare mean differences between heat-treated and control whelks. A significance level of P < 0.05 was applied in all analyses and Monte Carlo tests were used in cases where there were less than 30 permutations of the data.
All snails remained 100% attached in all the control tanks for the entire duration of the experiments. For the short-term, fast warming rate experiment, the first snails lost grip at 32°C (Figure 1A). At 35°C, 50% of the snails detached and at 37°C, all the remaining snails were detached from the glass plates or walls of the tanks (Figure 1A). The mean CTMax from all replicate treatment tanks in the short-term experiment was at 35.2°C, whilst the median CTMax calculated using nonlinear regression was recorded at 34.9°C (Figure 1A).
Figure 1 Percentage of Dicathais orbita remaining attached to the glass tank (blue diamonds) ± SD and mean CTMax (purple triangle) ± SD (n = 6 per tank) for (A) CTMax short-term with fast warming rate of 1°C/1 h temperature increment; (B) CTMax long-term with slow warming rate of 1°C/12 h temperature increment; and (C) the mean CTMax of D. orbita (pooled data from all the replicate tanks) at 1°C/1 h and 1°C/12 h (n = 6 per tank). Different superscript letters indicate significant difference.
For the longer-term, slow warming rate experiment, 28% of the snails started to lose attachment from the glass plate or walls of the tanks at 30°C (Figure 1B). At 31°C, 60% of the snails were detached and at 32°C, all snails had fallen off. Mean CTMax from the experimental tanks was 31.1°C and the median CTMax was 30.6°C (Figure 1B). The mean CTMax was significantly lower for snails in the slower warming rate experiment compared to those treated in the faster, short-term experiment (Pseudo F = 75.07; P (Perm) < 0.01) (Figure 1C). There were no mortalities during the CTMax experiments and all snails retained at the end of the experiment recuperated within 24hrs when returned to ambient temperature.
LC-MS analysis of the hypobranchial gland ethanol extracts of D. orbita revealed six compounds corresponding to the known brominated and non-brominated precursors of Tyrian purple (Figures 2, 3 and Table 2), consistent with the findings of Valles-Regino et al. (2016). In the CTMax and static experiments whelks from Ballina, showed a dominant peak in the diode array chromatogram at tR 1.5 mins (Figure 2A) corresponding to the UV profile of a non-brominated choline ester murexine (β [imidizoyl – 4 (5) acrylcholine]), with M+ 224 and fragment ions at m/z 104, 121, and 165 in the ESI/MS (total ion current (TIC), positive ion) (Figure 3A). The second most intense peak from the Ballina whelks in the UV diode array chromatograms was tyrindoxyl sulphate at 210 nm with a retention time (tR) 12.7 mins (Figure 2A). The ESI/MS, TIC negative ion of the base peak, shows a duplet ion cluster for bromine (Br79 Br81), consistent with the molecular weight of tyrindoxyl sulphate [M]- 336, 338) (Figure 3D). Tyrindoxyl sulphate was the dominant compound in the whelks from Coffs Harbour used in the 35 day ocean climate change experiment (Figure 2B). A small peak also detected in the negative ion mode eluting at tR 9.7 -11 mins (Figure 2) was tentatively identified as tyrindoxyl S-oxide sulphate, with strong signals at m/z 354 and 356 (Figure 3C) (Valles-Regino et al., 2016). A minor HPLC peak at tR 7.1 mins (Figure 2) registered major ions at m/z 226, 228 in positive ion mode that can be attributed to the pseudomolecular ion MH+ of 6-bromoisatin for Br79, Br81, with the sodium ion adduct at MNa+ at 248, 250 (Figure 3B). Another peak in the chromatogram at 12.8 mins was identified as tyrindoleninone (Figure 3), with ESI/MS ions [MH]+ 256, 258 (Figure 4E). An additional peak at 16.4 mins registered an isotopic cluster at m/z 535, 537, 539 (in a peak height ratio of 1:2:1) corresponding to the sodium adduct of tyriverdin ([M + Na]+ Br79 Br79, Br79 Br81, Br81 Br81) (Figure 3F).
Figure 2 Representative HPLC chromatograms of the secondary metabolites obtained from ethanol extracts from the hypobranchial gland of Dicathais orbita from (A) the diode array of whelks collected from Balliina for the CTMax experiments and (B) 210 nm from whelks collected from Coffs Harbour for the 35 day ocean climate change experiment. Peaks are shown as (a) murexine, (b) 6-bromoisatin, (c) tyrindoxyl S-oxide sulphate, (d) tyrindoxyl sulphate, (e) tyrindoleninone, and (f) tyriverdin.
Figure 3 Representative mass spectra of the secondary metabolites obtained from ethanol extracts from the hypobranchial gland of Dicathais orbita (A) murexine, (B) 6-bromoisatin, (C) tyrindoxyl S-oxide sulphate, (D) tyrindoxyl sulphate, (E) tyrindoleninone, and (F) tyriverdin.
Figure 4 Total yield in mg/g (mean % ± SD) of the ethanol extracts from the hypobranchial gland of Dicathais orbita: (A) Critical thermal maxima (CTMax) short-term, fast warming rate; (B) CTMax long-term, slow warming rate; (C) 7 day static heat stress; and (D) the 35 day ocean climate change experiment.
The total mean yield of the ethanol extracts from D. orbita hypobranchial glands ranged between 80 to 196 mg/g across all heat stress experiments (Figure 4). In all these experiments, there was a slight tendency towards a drop in the mean yield of the extracts, especially in the most severe heat stress conditions (i.e., the CTMax short term). However, the total yield did not significantly differ between the control and treatment tanks (CTMax short term: Pseudo F = 3.27; P (Perm) = 0.33; CTMax long term: Pseudo F = 0.12; P (Perm) = 0.92; static heat stress: Pseudo F = 1.12; P (Perm) = 0.98). In the 35 day ocean climate change experiment, the highest yield of the ethanol extract was obtained from samples held under current temperature and future pCO2 concentrations (Figure 4D). However, permutational univariate analysis (PERMANOVA) revealed no significant effect of temperature (Pseudo F = 0.62; P (Perm) = 0.44) or pCO2-induced acidification (Pseudo F = 0.06; P (Perm) = 0.80) on the total yield of the extracts across all treatments and no interaction between these factors was found (Pseudo F = 0.04; P (Perm) = 0.85).
In the CTMax short-term experiment, permutational multivariate analysis (PERMANOVA) revealed a significant difference in the overall composition of compounds extracted from the hypobranchial gland from whelks in control tanks compared to those that lost attachment at 37°C in heat treatment tanks (Pseudo F = 8.18; P (Perm) = 0.049) (Table 3 and Supplementary File). Principal coordinate ordination (PCO) revealed a distinct separation between the control and heat-treated snails along the x axis of the plot (Figure 5A). The snails sampled from the control tanks (20°C) are clustered together across the right side of the ordination plot, with a tendency toward higher murexine. By comparison, snails that lost attachment from the substrate at 37°C are much more variable and scattered towards the left side of the plot (Figure 5A). These results from the CTMax short-term experiment are supported by univariate analysis which revealed a significant decrease in murexine at 37°C compared to the control at 20°C (Pseudo F = 18.10; P (Perm) = 0.022, Table 3).
Table 3 Mean ( ± SD) weight of precursor compounds (mg/g) extracted from the hypobranchial gland of D. orbita exposed to heat treatment compared to ambient controls in four separate experiments (see Table 1).
Figure 5 Principal component ordination (PCO) based on a Euclidian distance similarity matrix on the secondary metabolite composition from the hypobranchial gland of Dicathais orbita, with vector overlay from Pearson correlation > 0.9. (A) Critical Thermal Maxima (CTMax) short-term fast warming rate with temperature increment of 1°C/1 h; (B) Critical Thermal Maxima (CTMax) longer-term with slow warming rate temperature increment of 1°C/12 h; (C) 7 day static heat stress; and (D) 35 day ocean climate change experiment.
The CTMax longer-term experiment showed similar changes in the chemical composition between the heat-stressed snails that lost attachment at 31°C and control snails at 20°C (Table 3, Figure 5A). In the PCO plot, the percent composition of the extracts shows separation along the x axis, which clearly distinguishes the heat-stressed individuals from those in the controls, which are clustered with a tendency toward higher murexine (Figure 5B). A significant difference in the overall secondary metabolite composition (Pseudo F = 16.92; P (MC) = 0.0036) was found after loss of muscle control at the elevated temperature, with a significant decrease in murexine levels (Pseudo F = 192.76; P (MC) = 0.0003, Table 3 and Supplementary File).
In the static heat stress experiment, the difference in the overall composition of extracts from treatment compared to control tanks was statistically significant (multivariate Pseudo F = 4.509; P (Perm) = 0.029, Supplementary File). The PCO analysis shows a similar trend to the CTMax experiments, with separation along the x axis distinguishing the controls from the heat stressed whelks (Figure 5C). Univariate analysis revealed that murexine significantly decreased in heat exposed whelks (Pseudo F = 7.44; P (Perm) = 0.032, Table 3). Tyrindoleninone also decreased significantly at the elevated temperature (Pseudo F = 4.51; P (Perm) = 0.033), whilst tyriverdin significantly increased at 30°C compared to the control at 20°C (Pseudo F = 4.51; P (Perm) = 0.038) (Table 3 and Supplementary File).
Across all three heat stress experiments, tyrindoxyl sulfate tended to increase in extracts from snails that were exposed to high temperature relative to controls (Table 2), although this was not statistically significant in any of the experiments due to high variability in quantification between replicates. Tyrindoleninone also showed a trend towards a drop after exposure to high temperature across all three experiments, but this was only significant in the static seven-day exposure experiment (Table 3). Conversely, tyriverdin tended to increase after exposure to elevated temperature and 6-bromoisatin increased in the acute heat stress (short-term CT Max and static heat experiments, Table 3). Tyrindoxyl S-oxide sulphate was below the limit for reliable quantification across all three heat experiments.
Examination of the secondary metabolites recovered from D. orbita hypobranchial glands after a 35 day ocean climate change experiment revealed a significant effect of temperature (Pseudo F = 6.66; P (Perm) = 0.015), but not pCO2-induced acidification treatments (Pseudo F = 1.94; P (Perm) = 0.09) and there was no interaction between these two factors (Pseudo F = 0.50; P (Perm) = 0.51, Supplementary File). PCO based on Euclidian distance similarity revealed overlapping of points between the two temperature treatments, however, there is a cluster of points dominated by extracts from snails held under current temperature at the right side of the plot (Figure 5D). Vector overlay using Pearson rank correlation suggests that this cluster of whelks held at ambient conditions (23°C) showed a tendency towards higher murexine and tyrindoxyl sulphate (Figure 5D).
Univariate PERMANOVA on all the individual compounds revealed that they responded significantly to elevated temperature (P (Perm) < 0.05, Table 2), but not to pCO2-induced acidification and there was no significant interaction between these factors (P (Perm) > 0.05, Supplementary File). In particular, murexine which ranged from 106–800 mg/g was found to significantly decrease by 32% on average in whelks held at 25°C compared to those exposed to 23°C (Table 3). Similarly, the ulimate precursor tyrindoxyl sulfate was found to decrease by 19% at elevated compared to ambient temperatures. The same pattern was observed for tyriverdin which recorded a significant mean decrease of about 45% at higher temperature (Table 2). Conversely, tyrindoleininone and the oxidised product, 6-bromoisatin, nearly doubled at the elevated temperature (Table 3).
Temperature is an important environmental factor that mediates the physiology and biochemical processes of all organisms. Organisms exposed to elevated temperature are likely to suffer poor physiological function as oxygen demand increases at high temperature and if not met, a transition to mitochondrial anaerobiosis occurs which may lead to limited circulatory and ventilatory capacity until the organisms fail to sustain life (Portner, 2001). This is clearly manifested by the significant loss of locomotory control at elevated temperatures and changes in the overall secondary metabolite composition from the hypobranchial gland of adult D. orbita under thermal stress. Consistent trends were observed on the dominant secondary metabolites after dynamic and static heat stress exteriments, irrespective of acute short-term, or longer-term thermal exposure treatments. The choline ester murexine showed a consistent and significant decrease at elevated temperature across all three heat stress and the ocean climate change experiments. Tyrindoxyl sulfate was also negatively impacted by elevated temperature, with a signficant decrease after 35 days exposure to ocean warming. However, there were no effects from elevated pCO2 or synergistic effects between these two ocean climate change stressors This highlights the potential for future ocean warming and marine heat waves to impact marine secondary metabolite precursor biosynthesis and/or storage in a predatory gastropod.
Murexine, is the dominant compound in the hypobranchial glands of D. orbita and the main secondary metabolite impacted by heat stress in this study. The relative proportion of this choline ester signficantly decreased in heat exposed snails in all four experiments. Murexine is a pharmacologically active muscle-relaxing compound that has significant neuromuscular blocking and nicotinic actions in a range of invertebrates and vertebrates, including humans (Erspamer and Glasser, 1957). It is found in a large number of predatory neogastropods (Roseghini et al., 1996) and is most likely used to subdue prey (Westley et al., 2006), although this has not been formally tested to date. Other choline esters found in Neogastropods, such as tigloylcholine, are considerably more toxic to mammals than murexine (Shiomi et al., 1998) and can present a potenial risk to human consumers. Therefore, further research into the impacts of ocean warming and marine heat waves on this class of invertebrate toxins would be worthwhile to assess whether any of these compounds are preferentially biosynthesised or more stable under elevated temperature.
Murexine is an example of a potential molecule of keystone signficance. In addition to its purported role in the predatory activities of whelks (Westley et al., 2006), in D. orbita, murexine is found in the reproductive tract of egg laying females and in the egg capsules, where it appears to sedate the trocophore larvae (Rudd et al., 2015b). Under elevated temperatures, larvae are expected to have higher metabolic activity and combined with increased swimming activity this could result shell damage and hypoxia from reduced oxygen availability during encapsulated development (e.g. Fernandez et al., 2007; Cancino et al., 2011). Therefore, any decreases in the availability of murexine under heat stress may reduce successful egg laying, larval development and recruitment, in neogastropods, in addition to impacting the feeding activites of these important rocky shore predators.
Coinciding with the drop in murexine at elevated temperatures was a consistent decrease in the ultimate precursor tyrindoxyl sulphate. Murexine has been shown to co-localise with tyrindoxyl sulphate in the medial region of the biosynthetic hypobranchial gland of muricids (Baker and Duke, 1976; Rudd et al., 2015a; Rudd et al., 2015b). The salt complex is presumed to be more stable than tyrindoxyl hydrogen sulfate to faciliate storage of the bioactive precursor. Murexine can be enzymatically hydrolysed by choline esterases (Grelis and Tabachnick, 1957), whereas tyrindoxyl sulphate is hydrolysed by arylsulphatase to inititiate a cascade of spontaneous, oxidative and photolytic reactions (Baker and Duke, 1976; Cooksey, 2001; Benkendorff, 2013). Recent transcriptomic studies of D. orbita confirm that this species produces both acetylcholine esterase and arylsulphatase (Baten et al., 2016), and these enzymes are upregulated in the hypobranchial glands (Laffy et al., 2013). The results from our current study suggests that heat stress may destablise the murexine-tryindoxyl sulphate salt complex and/or increase the activity of the endogenous hydrolytic enzymes.
Once secreted from the tissue, aryl sulfatase is suggested to be both temperature and pH-dependent (Elsner and Spanier, 1985). Molluskan arylsulphatase activity has been reported to increase by eight times when the incubation temperature was increased from 10°C to 38°C (Dodgson et al., 1953). Therefore, increased enzyme activity at higher temperatures could be responsible for the observed overall changes in the composition of secondary metabolites from D. orbita hypobranchial glands. At warmer temperatures, faster dissociation of murexine from tyrindoxyl sulphate might have occurred followed by hydrolysis of tyrindoxyl sulphate with the action of arylsulphatase to form the intermediate compounds. Consistent with this, after exposure to elevated temperature for a longer time frame in the ocean climate change experiment, there was a significant increase in the mean proportion of tyrindoleninone. After the removal of the sulphate group from tyrindoxyl sulphate, a transient compound tyrindoxyl is formed which readily oxidizes to give tyrindoleninone (Baker and Duke, 1973). However, this compound can then be converted to tyriverdin or oxidised to 6-bromoistatin. The relative balance between these three intermediate precursors may be impacted directly by the external conditions resulting in inconsistent trends between the three experiments. The milder conditions in the ocean climate change experiment (25°C) may faciliate the persistence of tyrindoleninone, resulting in less tyriverdin, whereas the higher temperatures (≥ 30°C) in the CTMax and static heat stress may favor further chemical reactions. Conversley, we found no impact of pCO2 driven changes in pH on the secondary metabolites of D. orbita implying aryl sulfatase activity is not impacted in seawarer in a pH range of 7.6- 8.2.
The proportional drop in tyrindoxyl sulphate in the extracts from snails that have been exposed to heat stress is consistent with increased hydrolysis by arylsulphatase, but also requires the precursor compound to come into contact with the enzyme. There is an indication that arylsulphatase activity in human blood serum increases due to lysosomal disruption after exposure to heat stress (Mila-Kierzenkowska et al., 2012). It has been suggested that tyrindoxyl sulphate is stored within vesicles in specific cells of the hypobranchial gland (Westley et al., 2010), whereas arylsulphatase activity occurs primarily on the epithelial surface. This suggests heat stress may compromise some of the cell or vesicle lipid bilayers. In our previous study on the effects of climate change on the fatty acid composition of D. orbita, we found a significant effect of elevated temperature on the fatty acid composition and in particular the balance of saturated to polyunsaturated fatty acids, which could have implications for altered permeability of lipid bilayers (Valles-Regino et al., 2015). Under heat stress, secretion of the tyrindoxyl sulphate salt precursor onto the epithelial layer of the hypobranchial glands would expose it to arylsulphatase (Baker and Duke, 1976) and potentially more oxygen, thus resulting in the decrease in tyrindoxyl sulphate and the observed proportional increase in secondary oxidation products [e.g. 6-bromoisatin, Cooksey (2001)]. With ongoing oxidation of the secondary metabolites, it could become energetically costly to replace them over prolonged period of heat stress, as would be expected from ocean warming. The production of secondary metabolites can be metabolically expensive in marine invertebrates (Rogers et al., 2002; Ivanisevic et al., 2011), and under stressful conditions a reduced allocation of energy to the production of these compounds would be expected, due to prioritised production of primary metabolites that are more immediately essential for biological functions (e.g Lu et al., 2016; Huo et al., 2019). The trade-off between secondary metabolism and other physiological functions (growth and reproduction) requires greater examination under stressful future climate conditions.
Critical thermal maxima based on loss of muscle control can be useful for establishing temperature regimes to investigate sublethal effects on marine gastropods. However, the predicted thermal limit can significantly differ depending on the rate of temperature change and ambient temperatures (Ribeiro et al., 2012). In the current study, D. orbita exhibited a significantly lower thermal tolerance under the slow warming rate compared to the fast heating rate. These results are consistent with previous studies on other marine species, such as fish (Mora and Maya, 2006; Vinagre et al., 2015), crustaceans (Faulkner et al., 2014; Vinagre et al., 2015) and the herbivorous gastropods Turbo militaris and Lunella undulata (Ab Lah et al., 2017). This different response to the rate of temperature rise may be due to the chronic build-up of heat stress over a longer period leading to physiological exhaustion (Ribeiro et al., 2012). The slow warming rate is more realistic for ocean climate change, and with a CTMax of ~31°C, D. orbita could be close to their upper thermal tolerance in northern NSW. Sea water temperature data collected from northern NSW from 2001 to 2008 showed maximum temperatures of 27°C in summer (Malcolm et al., 2011). However, intertidal species that are exposed at low tide must also be able to withstand the high air and substrate temperatures. A study by Lathlean et al. (2014) recorded temperatures up to 44°C on emersed intertidal rock walls, at the extreme end of the microhabitats where D. orbita occurs, on the mid-NSW coast near Sydney, Australia. The specimens used in our study were collected closer to their northern limit of distribution (> 800 km north) in a subtropical environment likely to experience even higher extreme environmental temperatures. Nevertheless, a previous study by Poloczanska et al. (2011) found little evidence for a southward shift in the distribution of many intertidal species, including D. orbita, thus indicating some resilience to sea surface temperature rise over the previous 50 years. However, with the ongoing increase in thermal stress expected to occur on rocky shores in eastern Australia due to global warming (Hobday and Pecl, 2014), along with strengthening of the East Australian current and regular coastal eddies (Suthers et al., 2011), it is possible a tipping point will occur as peak temperatures at the upper range of species distributions move closer to their critical thermal maxima.
Nevertheless, based on the CTMax assessment from the faster warming rate, it seems likely that D. orbita is able to withstand high water temperatures increases over short periods (up to 37°C). This apparent tolerance to acute heat stress might be expected for intertidal species that can experience seawater temperature flucuations from 10°C to more than 40°C during a single low tide on temperate shores (Harley and Helmuth, 2003) and up to 50°C on tropical shores (Williams and Morritt, 1995). In a recent study, microhabitat variation in the body temperature of D. orbita was estimated on intertidal reefs using biomimetic temperature loggers (Lathlean et al., 2014). It was found that D. orbita shells can buffer the internal temperature by nearly 9°C on extremely hot days (44°C) and on average were 2°C cooler than the habitat temperatures (Lathlean et al., 2014). This ability to thermoregulate may reduce the impacts of exposure to air temperature at low tide, but is unlikely to protect them against unconditionally warm water currents during extreme heat wave events (e.g,. Pearce and Feng, 2013; Wernberg et al., 2013; Garrabou et al., 2009; Selig et al., 2010; Lima and Wethey, 2012). D. orbita was able to recuperate after the heat treatments in our experiments suggesting some potential resilience, however, their detachment from the wall of the tank resulted from loss of muscle activity and in their natural intertidal habiats, this response would increase the chance of being washed ashore, or offshore to unsuitable habitats. Furthermore, longer term exposure to even slight increases in temperature can generate accumulated sublethal effects, on both the secondary metabolites observed here and previously reported primary metabolites (Valles-Regino et al., 2015; Tate et al., 2017). Understanding the temperature tolerance levels of aquatic organisms is critical for predicting the extent to which climate change and other thermal alteration will impact the overall fitness of species. Given the potential for sublethal impacts leading to mortality in local populations, along with the reduced foraging rates for D. orbita on exposed rocky substrate predicted by Lathlean et al. (2014), there could be significant flow-on effects for intertidal community structure on the east coast of Australia.
This manipulative study on the critical thermal maxima and impacts of heat stress and ocean warming on marine secondary metabolism has important implications for species reproductive physiology, natural defense mechanisms and the sustainable supply of bioactive compounds under future oceanic conditions. Secondary metabolites are important modulators of cellular reactions and mediate many interactions between organisms (Williams et al., 1989). The choline ester and brominated indole secondary metabolites in Muricidae mollusks appear to have a broad suite of biological activities (Benkendorff et al., 2015) and play multiple roles in the life history of the mollusks including predation, reproduction and defense (Benkendorff et al., 2000; Westley and Benkendorff, 2008; Rudd et al., 2015b). Given that invertebrate predators often act as keystone species with disproportional impact on intertidal communities (Paine, 1974), and the multifunctional role of the Muricidae metabolites, these compounds could prove to be “molecules of keystone significance” (Derby and Aggio, 2011; Benkendorff, 2014). This suggests that any impacts of ocean warming on these biologically important marine natural products may not only threaten the populations of Muricidae, but potentially the structure of intertidal communities where they are dominant predators.
This study has provided a novel insight into the upper thermal limit and effects of elevated temperature on the secondary metabolism of a marine invertebrate predator. From the results, it is likely that D. orbita could be vulnerable to future ocean warming. Despite high short-term thermal tolerance, chronic exposure to elevated temperatures could reduce resilience to extreme temperatures and result in cumulative sublethal impacts on physiology, metabolism and secondary metabolite production and storage. The increased temperatures on rocky intertidal shores may lead to foraging and range reductions whilst impacts on secondary metabolites may have implications for successful reproduction and encapsulated larval development of this species, with potential long-term effects on intertidal community structure.
The original contributions presented in the study are included in the article/Supplementary Material. Further inquiries can be directed to the corresponding author.
RV-R, KB, and LY conceived and designed the experiments; RV-R performed the manipulative experiments with supervision from KB and LY; RV-R and PM performed the LC-MS experiments and validated the data; RV-R, PM, and KB interpreted the LC-MS data; RV-R and KB ran the statistical analyses; KB and RV-R wrote the manuscript with editorial contributions from PM and LY. All authors contributed to the article and approved the submitted version.
This research was supported by an Australia Awards Scholarship to RV-R and funding from the Marine Ecology Research Centre, Southern Cross University.
The authors declare that the research was conducted in the absence of any commercial or financial relationships that could be construed as a potential conflict of interest.
All claims expressed in this article are solely those of the authors and do not necessarily represent those of their affiliated organizations, or those of the publisher, the editors and the reviewers. Any product that may be evaluated in this article, or claim that may be made by its manufacturer, is not guaranteed or endorsed by the publisher.
The authors are grateful for assistance from Dr Roslizawati Ab Lah for whelk sampling and help with CTMax experiments, Prof. Brendan Kelaher and Ricky Tate for experimental set up and maintenance of the 35-day ocean change experiment. We appreciate access to laboratory facilities in the Faculty of Science and Engineering, Lismore and National Marine Science Centre, as well as the Southern Cross Analytical Research Laboratory, Southern Cross University. RV-R is grateful to Davao Oriental State College of Science and Technology, Mati City, Davao Oriental, Philippines for the opportunity to pursue doctoral studies in Australia.
The Supplementary Material for this article can be found online at: https://www.frontiersin.org/articles/10.3389/fmars.2022.830425/full#supplementary-material
Ab Lah R., Benkendorff K., Bucher D. (2017). Thermal Tolerance and Preference of Exploited Turbinid Snails Near Their Range Limit in a Global Warming Hotspot. J. Therm. Biol. 64, 100–108. doi: 10.1016/j.jtherbio.2017.01.008
Ab Lah R., Kelaher B. P., Bucher D., Benkendorff K. (2018). Ocean Warming and Acidification Affect the Nutritional Quality of the Commercially-Harvested Turbinid Snail Turbo Militaris. Mar. Environ. Res. 141, 100–108. doi: 10.1016/j.marenvres.2018.08.009
Ahmad T., Rudd D., Benkendorff K., Mahdi L. K., Pratt K.-A., Dooley L., et al. (2017). Brominated Indoles From a Marine Mollusc Inhibit Inflammation in a Murine Model of Acute Lung Injury. PloS One 12, e0186904. doi: 10.1371/journal.pone.0186904
Arnold T., Mealey C., Leahey H., Miller A. W., Hall-Spencer J. M., Milazzo M., et al. (2012). Ocean Acidification and the Loss of Phenolic Substances in Marine Plants. PloS One 7, 1–10. doi: 10.1371/journal.pone.0035107
Baker J. T. (1974). Tyrian Purple: An Ancient Dye, a Modern Problem. Endeavor 33, 11–17. doi: 10.1016/0160-9327(74)90003-9
Baker J. T., Duke C. C. (1973). Chemistry of the Indoleninone. II. Isolation From the Hypobrancial Glands of Marine Mollusks 6-Bromo-2,2-Dimethylthoindolin-3-One and 6-Bromo-2-Methylthioindoleninone as Alternative Precursors to Tyrian Purple. Aust. J. Chem. 26, 2153–2157. doi: 10.1071/CH9732153
Baker J. T., Duke C. C. (1976). Isolation of Choline and Choline Ester Salts of Tyrindoxyl Sulphate From the Marine Mollusks Dicathais Orbita and Mancinella Keineri. Tetrahedron Lett. 15, 1233–1234. doi: 10.1016/S0040-4039(00)78026-9
Baten A., Ngangbam A. K., Waters D. L., Benkendorff K. (2016). Transcriptome of the Australian Mollusk Dicathais Orbita Provides Insights Into the Biosynthesis of Indoles and Choline Esters. Mar. Drugs 14, 135. doi: 10.3390/md14070135
Beger M., Babcock R., Booth D. J., Bucher D., Condie S. A., Creese B., et al. (2011). Research Challenges to Improve the Management and Conservation of Subtropical Reefs to Tackle Climate Change Threats. Ecol. Manag. Restor. 12, e7–e10. doi: 10.1111/j.1442-8903.2011.00573.x
Benkendorff K. (2013). Natural Products in the Australian Marine Invertebrate Dicathais Orbita. Mar. Drugs 11, 1370–1398. doi: 10.3390/md11041370
Benkendorff K. (2014). “Chemical Diversity in Molluskan Communities: From Natural Products to Chemical Ecology,” in Neuroecology and Neuroethology in Mollusks: The Interface Between Behavior and Environment. Eds. Di cosmo A., Willow W. (New York, USA: Nova Science Publishers, Inc).
Benkendorff K., Bremner J. B., Davis A. R. (2000). Tyrian Purple Precursors in the Egg Masses of the Australian Muricid, Dicathais Orbita: A Possible Role. J. Chem. Ecol. 26, 1037–1050. doi: 10.1023/a:1005441116095
Benkendorff K., Davis A. R., Bremner J. B. (2001). Chemical Defense in the Egg Masses of Benthic Invertebrates: An Assessment of Antibacterial Activity in 39 Mollusks and 4 Polychaetes. J. Invertebr. Pathol. 78, 109–118. doi: 10.1006/jipa.2001.5047
Benkendorff K., Rudd D., Nongmaithem B. D., Liu L., Young F., Edwards V., et al. (2015). Are the Traditional Medical Uses of Muricidae Molluscs Substantiated by Their Pharmacological Properties and Bioactive Compounds? Mar. Drugs 13, 5237–5275. doi: 10.3390/md13085237
Berenbaum M. R. (1995). The Chemistry of Defense: Theory and Practice. Proc. Natl. Acad. Sci. 92, 2–8. doi: 10.1073/pnas.92.1.2
Borell E., Steinke M., Fine M. (2013). Direct and Indirect Effects of High Pco2 on Algal Grazing by Coral Reef Herbivores From the Gulf of Aqaba (Red Sea). Coral Reefs 32, 937–947. doi: 10.1007/s00338-013-1066-5
Byrne M. (2011). Impact of Ocean Warming and Ocean Acidification on Marine Invertebrate Life History Stages : Vulnerabilities and Potential for Persistence in a Changing Ocean. Oceanogr. Mar. Biol.: Annu. Rev. 49, 1–42. doi: 10.1201/b11009-2
Byrne M., Przeslawski R. (2013). Multistressor Impacts of Warming and Acidification of the Ocean on Marine Invertebrates’ Life Histories. Integr. Comp. Biol. 53, 582–596. doi: 10.1093/icb/ict049
Caldeira K., Wickett M. E. (2005). Ocean Model Predictions of Chemical Changes From Carbon Dioxide Emissions to the Atmosphere and Ocean. J. Geophys. Res. Oceans 110, 1–12. doi: 10.1029/2004JC002671
Cancino J. M., Gallardo J. A., Brante A. (2011). The Relationship Between Temperature, Oxygen Condition and Embryo Encapsulation in the Marine Gastropod Chorus Giganteus. J. Mar. Biol. Assoc. U. K. 91, 727–733. doi: 10.1017/S0025315410001335
Cimino G., Passeggio A., Sodano G., Spinella A., Villani G. (1991). Alarm Pheromones From the Mediterranean Opisthobranch Haminoea Navicula. Cell Mol. Life Sci. 47, 61–63. doi: 10.1007/BF02041253
Cimino G., Sodano G. (2005). “Biosynthesis of Secondary Metabolites in Marine Mollusks,” in Marine Natural Products - Diversity and Biosynthesis. Ed. Scheuer P. (Germany: Springer-Verlag Berlin Heidelberg).
Cimino G., Spinella A., Sodano G. (1989). Potential Alarm Pheromones From the Mediterranean Opisthobranch Scaphander Lignarius. Tetrahedron Lett. 30, 5003–5004. doi: 10.1016/S0040-4039(01)80566-9
Cooksey C. J. (2001). Tyrian Purple: 6,6'-Dibromoindigo and Related Compounds. Molecules 6, 736–769. doi: 10.3390/60900736
Cooksey C. J. (2006). “Marine Indirubins,” in Indirubin, the Red Shade of Indigo. Eds. Meijer L., Guyard N., Skatsounis L. & Eisenbrand G. (Roscoff, France: Progress in Life Series).
Cooksey C. (2013). Tyrian Purple: The First Four Thousand Years. Sci. Prog. 96, 171–186. doi: 10.3184/003685013X13680345111425
Cummins S. F., Nichols A. E., Amare A., Hummon A. B., Sweedler J. V., Nagle G. T. (2004). Characterization of Aplysia Enticin and Temptin, Two Novel Water-Borne Protein Pheromones That Act in Concert With Attractin to Stimulate Mate Attraction. J. Biol. Chem. 279, 25614–25622. doi: 10.1074/jbc.M313585200
Dang V. T., Benkendorff K., Green T., Speck P. (2015). Marine Snails and Slugs: A Great Place to Look for Antiviral Drugs. J. Virol. 89, 8114–8118. doi: 10.1128/JVI.00287-15
Derby C. D., Aggio J. F. (2011). The Neuroecology of Chemical Defenses. Integr. Comp. Biol. 51, 771–780. doi: 10.1093/icb/icr063
Détrée C., Ortiz A., Navarro J. M. (2020). Combined Effects of Warming and Freshening on the Physiological Energetics of the Edible Whelk Trophon Geversianus. Mar. Environ. Res. 153, 104840. doi: 10.1016/j.marenvres.2019.104840
Diaz F., Portilla M., Aguilar M., Re-araujo A. D. (2000). Preferred Temperature and Critical Thermal Maxima of Red Abalone Haliotis Rufescen. J. Therm. Biol. 25, 257–261. doi: 10.1016/S0306-4565(99)00032-7
Diaz F., Re A. D., Medina Z., Re G., Valdez G., Valenzuela F. (2006). Thermal Preference and Tolerance of Green Abalone Haliotis Fulgens (Philippi 1845) and Pink Abalone Haliotis Corrugatta (Gray 1828). Aquac. Res. 37, 877–844. doi: 10.1111/j.1365-2109.2006.01506.x
Diaz F., Re A. D., Salas A., Galindo-Sanchez C. E., Gonzales M. A., Sanchez A., et al. (2013). Behavioral Thermoregulation and Critical Thermal Limits of Giant Keyhole Limpet Megathura Crenulata (Sowerby 1825) (Mollusca; Vetigastropoda). J. Therm. Biol. 36, 34–37. doi: 10.1016/j.jtherbio.2013.05.007
Diaz F., Salas A., Re A. D., Gonzalez M., Reyes I. (2011). Thermal Preference and Tolerance of Megastrea (Lithopoma) Undosa (Wood 1828;Gastropoda:Turbinidae). J. Therm. Biol. 36, 34–37. doi: 10.1016/j.jtherbio.2010.10.004
Di Cosmo A., William W. (2014). Neuroecology and Neuroethology in Mollusks: The Interface Between Behaviour and Environment (New Your, NY, USA: Nova Science Publishers).
Dodgson K., Lewis J., Spencer B. (1953). Studies on Sulphatases. 3. The Arylsulphatase and β-Glucuronidase of Marine Mollusks. Biochem. J. 55, 253. doi: 10.1042/bj0550253
Doney S. C., Fabry V. J., Feely R. A., Kleypas J. A. (2009). Ocean Acidification: The Other CO2 Problem. Annu. Rev. Mar. Sci. 1, 169–192. doi: 10.1146/annurev.marine.010908.163834
Doull J. L., Ayer S. W., Singh A. K., Thilbault P. (1993). Production of a Novel Polyketide Antibiotic, Jadomycin B, by Streptomyces Venezuelae Following Heat Shock. J. Antibiot. 46, 869–871. doi: 10.7164/antibiotics.46.869
Durán L. R., Castilla J. C. (1989). Variation and Persistence of the Middle Rocky Intertidal Community of Central Chile, With and Without Human Harvesting. Mar. Biol. 103, 555–562. doi: 10.1007/BF00399588
Elsner O., Spanier E. (1985). The Dyeing With Purpura Haemastoma. 7th International Symposium on Fiber Science & Technology (Hakone, Japan: Japanese Society of Fiber and Technology).
Erspamer V., Glasser A. (1957). The Pharmacological Actions of Murexine (Urocanylcholine). Br. J. Pharmacol. Chemother. 12, 176–184. doi: 10.1111/j.1476-5381.1957.tb00117.x
Faulkner K. T., Clusella-Trullas S., Peck L. S., Chown S. L. (2014). Lack of Coherence in the Warming Responses of Marine Crustaceans. Funct. Ecol. 28, 895–903. doi: 10.1111/1365-2435.12219
Fernandez M., Calderon R., Cancino J., Jeno K. (2007). Effect of Temperature on the Development of Encapsulated Embryos of Concholepas Concholepas Along a Latitudinal Cline. Mar. Ecol. Prog. Ser. 348, 229–237. doi: 10.3354/meps07032
Figueroa F., Barufi J. B., Malta E., Conde-álvarez R., Nitschke U., Arenas F., et al. (2014). Short-Term Effects of Increasing CO2, Nitrate and Temperature on Three Mediterranean Macroalgae: Biochemical Composition. Aquat. Biol. 22, 177–193. doi: 10.3354/ab00610
Fortunato H. (2015). Mollusks: Tools in Environmental and Climate Research. Am. Malacol. Bull. 33, 310–324. doi: 10.4003/006.033.0208
Frölicher T. L., Fischer E. M., Gruber N. (2018). Marine Heatwaves Under Global Warming. Nature 560, 360–364. doi: 10.1038/s41586-018-0383-9
Garrabou J., Coma R., Bensoussan N., Bally M., Chevaldonné P., Cigliano M., et al. (2009). Mass Mortality in Northwestern Mediterranean Rocky Benthic Communities: Effects of the 2003 Heat Wave. Glob. Change Biol. 15, 1090–1103. doi: 10.1111/j.1365-2486.2008.01823.x
Gazeau F., Parker L. M., Comeau S., Gattuso J.-P., O'connor W. A., Martin S., et al. (2013). Impacts of Ocean Acidification on Marine Shelled Mollusks. Mar. Biol. 160, 2207–2245. doi: 10.1007/s00227-013-2219-3
Gilroy A., Edwards S. J. (1998). Optimum Temperature for Growth of Australian Abalone: Preferred Temperature and Critical Thermal Maximum for Blacklip Abalone Haliotis Rubra (Leach), and Greenlip Abalone Haliotis Laevigata (Leach). Aquac. Res. 29, 481–485. doi: 10.1111/j.1365-2109.1998.tb01157.x
Grelis M., Tabachnick I. (1957). The Enzymatic Hydrolysis of Imidazoleacryloylcholine (Murexine) and Imidazolepropionylcholine (Dihydromurexine) by Various Cholinesterases. Br. J. Pharm. Chemother. 12, 320–322. doi: 10.1111/j.1476-5381.1957.tb00141.x
Guerrero M. G., Sandoval P. H., Rojas J. O., Jacinto E. C. (2013). Effect of Temperature on Weight Increase, Survival, and Thermal Preference of Juvenile Redclaw Crayfish Cherax Quadricarinatus. Hidrobiologica 23, 9–17.
Hale R., Calosi P., Mcneill L., Mieszkowska N., Widdicombe S. (2011). Predicted Levels of Future Ocean Acidification and Temperature Rise Could Alter Community Structure and Biodiversity in Marine Benthic Communities. Oikos 120, 661–674. doi: 10.1111/j.1600-0706.2010.19469.x
Harley C. D., Helmuth B. S. (2003). Local- and Regional-Scale Effects of Wave Exposure, Thermal Stress, and Absolute Versus Effective Shore Level on Patterns of Intertidal Zonation. Limnol. Oceanogr. 48, 1498–1508. doi: 10.4319/lo.2003.48.4.1498
Helmuth B. (1999). Thermal Biology of Rocky Intertidal Mussels: Quantifying Body Temperatures Using Climatological Data. Ecology 80, 15–34. doi: 10.1890/0012-9658(1999)080[0015:TBORIM]2.0.CO;2
Helmuth B., Broitman B. R., Yamane L., Gilman S. E., Mach K., Mislan K., et al. (2010). Organismal Climatology: Analyzing Environmental Variability at Scales Relevant to Physiological Stress. J. Exp. Biol. 213, 995–1003. doi: 10.1242/jeb.038463
Helmuth B., Harley C. D., Halpin P. M., O'donnell M., Hofmann G. E., Blanchette C. A. (2002). Climate Change and Latitudinal Patterns of Intertidal Thermal Stress. Science 298, 1015–1017. doi: 10.1126/science.1076814
Helmuth B., Kingsolver J. G., Carrington E. (2005). Biophysics, Physiological Ecology, and Climate Change: Does Mechanism Matter? Annu. Rev. Physiol. 67, 177–201. doi: 10.1146/annurev.physiol.67.040403.105027
Hobday A. J., Pecl G. T. (2014). Identification of Global Marine Hotspots: Sentinels for Change and Vanguards for Adaptation Action. Rev. Fish Biol. Fish. 24, 414–425. doi: 10.1007/s11160-013-9326-6
Huo D., Sun L., Zhang L., Ru X., Liu S., Yang H. (2019). Metabolome Responses of the Sea Cucumber Apostichopus Japonicus to Multiple Environmental Stresses: Heat and Hypoxia. Mar. Pollut. Bull. 138, 407–420. doi: 10.1016/j.marpolbul.2018.11.063
IPCC (2013). Summary for Policymakers in Climate Change 2013: The Physical Science Basis, Contribution of Working Group I to the Fifth Assessment Report of the Intergovernmental Panel on Climate Change. Eds. Stocker T., Qin D., Plattner G., Tignor M., Allen S., Boschung J., Nauels A., Xia Y., Bex V., Midgley P. (Cambridge, New York, USA: Cambridge University Press).
IPCC (2019). “IPCC Special Report on the Ocean and Cryosphere in a Changing Climate,” in Report of the Intergovernmental Panel on Climate Change. Eds. Pörtner H.-O., Roberts D. C., Masson-Delmotte V., Zhai P., Tignor M., Poloczanska E., Mintenbeck K., Nicolai M., Okem A., Petzold J. (Geneva, Switzerland: IPCC).
Ivanisevic J., Thomas O., Pedel L., Penez N., Ereskovsky A., Culioli G., et al. (2011). Biochemical Trade-Offs: Evidence for Ecologically Linked Secondary Metabolism of the Sponge Oscarella Balibaloi. PloS One 6, e28059. doi: 10.1371/journal.pone.0028059
Kerrison P., Suggett D. J., Hepburn L. J., Steinke M. (2012). Effect of Elevated Pco2 on the Production of Dimethylsulphoniopropionate (DMSP) and Dimethylsulphide (DMS) in Two Species of Ulva (Chlorophyceae). Biogeochemistry 110, 5–16. doi: 10.1007/s10533-012-9707-2
Kroeker K. J., Kordas R. L., Crim R., Hendriks I. E., Ramajo L., Singh G. S., et al. (2013). Impacts of Ocean Acidification on Marine Organisms: Quantifying Sensitivities and Interaction With Warming. Glob. Change Biol. 19, 1884–1896. doi: 10.1111/gcb.12179
Laffy P. W., Benkendorff K., Abbott C. A. (2013). Suppressive Subtractive Hybridisation Transcriptomics Provides a Novel Insight Into the Functional Role of the Hypobranchial Gland in a Marine Mollusk. Comp. Biochem. Physiol. D 8, 111–122. doi: 10.1016/j.cbd.2013.01.001
Lathlean J. A., Ayre D. J., Coleman R. A., Minchinton T. E. (2014). Using Biomimetic Loggers to Measure Interspecific and Microhabitat Variation in Body Temperatures of Rocky Intertidal Invertebrates. Mar. Freshw. Res. 66, 86–94. doi: 10.1071/MF13287
Lemasson A. J., Hall-Spencer J. M., Kuri V., Knights A. M. (2019). Changes in the Biochemical and Nutrient Composition of Seafood Due to Ocean Acidification and Warming. Mar. Environ. Res. 143, 82–92. doi: 10.1016/j.marenvres.2018.11.006
Lima F. P., Wethey D. S. (2012). Three Decades of High-Resolution Coastal Sea Surface Temperatures Reveal More Than Warming. Nat. Commun. 3, 704. doi: 10.1038/ncomms1713
Lu J., Shi Y., Wang S., Chen H., Cai S., Feng J. (2016). NMR-Based Metabolomic Analysis of Haliotis Diversicolor Exposed to Thermal and Hypoxic Stresses. Sci. Total Environ., 545–546, 280–288. doi: 10.1016/j.scitotenv.2015.12.071
Madeira D., Narciso L., Cabral H. N., Vinagre C. (2012). Thermal Tolerance and Potential Impacts of Climate Change on Coastal and Estuarine Organisms. J. Sea Res. 70, 32–41. doi: 10.1016/j.seares.2012.03.002
Malcolm H. A., Davies P. L., Jordan A., Smith S. D. (2011). Variation in Sea Temperature and the East Australian Current in the Solitary Islands Region Between 2001–2008. Deep Sea Res. Part II 58, 616–627. doi: 10.1016/j.dsr2.2010.09.030
Malin G., Lapidot A. (1996). Induction of Synthesis of Tetrahydropyrimidine Derivatives in Streptomyces Strains and Their Effect on Escherichia Coli in Response to Osmotic and Heat Stress. J. Bacteriol. 178, 385–395. doi: 10.1128/jb.178.2.385-395.1996
Manríquez P. H., Jara M. E., Seguel M. E., Torres R., Alarcon E., Lee M. R. (2016). Ocean Acidification and Increased Temperature Have Both Positive and Negative Effects on Early Ontogenetic Traits of a Rocky Shore Keystone Predator Species. PloS One 11, e0151920. doi: 10.1371/journal.pone.0151920
Mila-Kierzenkowska C., Woźniak A., Szpinda M., Boraczyński T., Woźniak B., Rajewski P., et al. (2012). Effects of Thermal Stress on the Activity of Selected Lysosomal Enzymes in Blood of Experienced and Novice Winter Swimmers. Scand. J. Clin. Lab. Invest. 72, 635–641. doi: 10.3109/00365513.2012.727214
Mora C., Maya M. F. (2006). Effect of the Rate of Temperature Increase of the Dynamic Method on the Heat Tolerance of fishes. J. Therm. Biol. 31, 337–341. doi: 10.1016/j.jtherbio.2006.01.005
Mori K., Goto-Yamamoto N., Kitayama M., Hashizume K. (2007). Loss of Anthocyanins in Red-Wine Grape Under High Temperature. J. Exp. Bot. 58, 1935–1945. doi: 10.1093/jxb/erm055
Morton B. (2008). Attack Responses of the Southern Australian Whelk, Lepsiella Vinosa (Lamarck 1822) (Gastropoda: Muricidae), to Novel Bivalve Prey: An Experimental Approach. Biol. Invasions 10, 1265–1275. doi: 10.1007/s10530-007-9201-2
Mouthon J., Daufresne M. (2006). Effects of the 2003 Heatwave and Climatic Warming on Mollusk Communities of the Saône: A Large Lowland River and of its Two Main Tributaries (France). Glob. Change Biol. 12, 441–449. doi: 10.1111/j.1365-2486.2006.01095.x
Olivera B. M. (2002). Conus Venom Peptides: Reflections From the Biology of Clades and Species. Annu. Rev. Ecol. Syst. 33, 25–47. doi: 10.1146/annurev.ecolsys.33.010802.150424
Pawlik J. R. (1993). Marine Invertebrate Chemical Defense. Chem. Rev. 93, 1911–1922. doi: 10.1021/cr00021a012
Pawlik J. R. (2012). Antipredatory Defensive Roles of Natural Products From Marine Invertebrates. In: Fattorusso E., Gerwick W., Taglialatela-Scafati O. (eds) Handbook of Marine Natural Products. Springer, Dordrecht. doi: 10.1007/978-90-481-3834-0_12
Pearce A. F., Feng M. (2013). The Rise and Fall of the “Marine Heat Wave” Off Western Australia During the Summer of 2010/2011. J. Mar. Syst. 111, 139–156. doi: 10.1016/j.jmarsys.2012.10.009
Pierrot D., Lewis E., Wallace D. W. R. (2006). MS Excel Program Developed for CO2 System Calculations. ORNL/CDIAC-105a. Carbon Dioxide Information Analysis Centre (Oak Ridge, TN: Oak Ridge National Laboratory, US Department of Energy).
Poloczanska E. S., Smith S., Fauconnet L., Healy J., Tibbetts I. R., Burrows M. T., et al. (2011). Little Change in the Distribution of Rocky Shore Faunal Communities on the Australian East Coast After 50 Years of Rapid Warming. J. Exp. Mar. Biol. Ecol. 400, 154–154. doi: 10.1016/j.jembe.2011.02.018
Portner H. O. (2001). Climate Change an Temperature-Dependent Biogeography: Oxygen Limitation of Thermal Tolerance in Animals. Naturwissenschaften 88, 137–146. doi: 10.1007/s001140100216
Pörtner H.-O. (2002). Climate Variations and the Physiological Basis of Temperature Dependent Biogeography: Systemic to Molecular Hierarchy of Thermal Tolerance in Animals. Comp. Biochem. Physiol. Part A: Mol. Integr. Physiol. 132, 739–761. doi: 10.1016/S1095-6433(02)00045-4
Przeslawski R., Ahyong S., Byrne M., Wörheide G., Hutchings P. A. T. (2008a). Beyond Corals and Fish: The Effects of Climate Change on Noncoral Benthic Invertebrates of Tropical Reefs. Glob. Change Biol. 14, 2773–2795. doi: 10.1111/j.1365-2486.2008.01693.x
Przeslawski R., Ahyong S., Byrne M., Wörheide G., Hutchings P. A. T. (2008b). Beyond Corals and Fish: The Effects of Climate Change on Noncoral Benthic Invertebrates of Tropical Reefs. Glob. Change Biol. 14, 2773–2795. doi: 10.1111/j.1365-2486.2008.01693.x
Rahman M. M., Junaid M., Hosen S. M. Z., Mostafa M., Liu L., Benkendorff K. (2021). Mollusc-Dervied Brominated Indoles for the Selective Inhibition of Cyclooxygenase: A Computational Expedition. Molecules 26, 6538. 1-27. doi: 10.3390/molecules26216538
Ribeiro P. L., Camacho A., Navas C. A. (2012). Considerations for Assessing Maximum Critical Temperatures in Small Ectothermic Animals: Insights From Leaf-Cutting Ants. PloS One 7, 1–7. doi: 10.1371/journal.pone.0032083
Rogers C. N., De Nys R., Steinberg P. D. (2002). Effects of Algal Diet on the Performance and Susceptibility to Predation of the Sea Hare Aplysia Parvula. Mar. Ecol. Prog. Ser. 236, 241–254. doi: 10.3354/meps236241
Roseghini M., Severini C., Erspamer G. F., Erspamer V. (1996). Choline Esters and Biogenic Amines in the Hypobranchial Gland of 55 Molluskan Species of the Neogastropod Muricoidea Superfamily. Toxicon 34, 33–55. doi: 10.1016/0041-0101(95)00104-2
Rudd D., Benkendorff K. (2014). Supercritical CO2 Extraction of Bioactive Tyrian Purple Precursors From the Hypobranchial Gland of a Marine Gastropod. J. Supercrit. Fluids 94, 1–7. doi: 10.1016/j.supflu.2014.06.016
Rudd D., Benkendorff K., Chahal C., Guinan T., Gustafsson O. J. R., Esmaeelian B., et al. (2019). Mapping Insoluble Indole Metabolites in the Gastrointestinal Environment of a Murine Colorectal Cancer Model Using Desortption/Ionisation on Porpus Silicon Imaging. Sci. Rep. 9, 12342–12355. doi: 10.1038/s41598-019-48533-2
Rudd D., Benkendorff K., Voelcker N. H. (2015a). Solvent Separating Secondary Metabolites Directly From Biosynthetic Tissue for Surface-Assisted Laser Desorption Ionisation Mass Spectrometry. Mar. Drugs 13, 1410–1431. doi: 10.3390/md13031410
Rudd D., Ronci M., Johnston M. R., Guinan T., Voelcker N. H., Benkendorff K. (2015b). Mass Spectrometry Imaging Reveals New Biological Roles for Choline Esters and Tyrian Purple Precursors in Muricid Mollusks. Sci. Rep. 5, 1–13. doi: 10.1038/srep13408
Selig E. R., Casey K. S., Bruno J. F. (2010). New Insights Into Global Patterns of Ocean Temperature Anomalies: Implications for Coral Reef Health and Management. Global Ecol. Biogeogr. 19, 397–411. doi: 10.1111/j.1466-8238.2009.00522.x
Shinde P., Banerjee P., Mandhare A. (2019). Marine Natural Products as Source of New Drugs: A Patent Review, (2015–2018). Expert Opin. Ther. Pat. 29, 283–309. doi: 10.1080/13543776.2019.1598972
Shiomi K., Ishii M., Shimakura K., Nagashima Y., Chino M. (1998). Tigloylcholine: A New Choline Ester Toxin From the Hypobranchial Gland of Two Species of Muricid Gastropods (Thais Clavigera and Thais Bronni). Toxicon 36, 795–798. doi: 10.1016/S0041-0101(97)00166-9
Smale D. A., Wernberg T., Oliver E. C. J., Thomsen M., Harvey B. P., Straub S. C., et al. (2019). Marine Heatwaves Threaten Global Biodiversity and the Provision of Ecosystem Services. Nat. Clim. Change 9, 306–312. doi: 10.1038/s41558-019-0412-1
Somero G. N. (2002). Thermal Physiology and Vertical Zonation of Intertidal Animals: Optima, Limits, and Costs of Living. Integr. Comp. Biol. 42, 780–789. doi: 10.1093/icb/42.4.780
Somero G. N. (2010). The Physiology of Climate Change: How Potentials for Acclimatization and Genetic Adaptation Will Determine ‘Winners’ and ‘Losers’. J. Exp. Biol. 213, 912–920. doi: 10.1242/jeb.037473
Southward A. J., Hawkins S. J., Burrows M. T. (1995). Seventy Years' Observations of Changes in Distribution and Abundance of Zooplankton and Intertidal Organisms in the Western English Channel in Relation to Rising Sea Temperature. J. Therm. Biol. 20, 127–155. doi: 10.1016/0306-4565(94)00043-I
Summer K., Browne J., Liu L., Benkendorff K. (2020). Molluscan Compounds Provide Drug Leads for the Treatment and Prevention of Respiratory Disease. Mar. Drugs 18 (570), 50. doi: 10.3390/md18110570
Suthers I. M., Young J. W., Baird M. E., Roughan M., Everett J. D., Brassington G. B., et al. (2011). The Strengthening East Australian Current, its Eddies and Biological Effects—an Introduction and Overview. Deep Sea Res. Part II Top. Stud. Oceanogr. 58, 538–546. doi: 10.1016/j.dsr2.2010.09.029
Swanson A. K., Fox C. H. (2007). Altered Kelp (Laminariales) Phlorotannins and Growth Under Elevated Carbon Dioxide and Ultraviolet-B Treatments can Influence Associated Intertidal Food Webs. Glob. Change Biol. 13, 1696–1709. doi: 10.1111/j.1365-2486.2007.01384.x
Tate R. D., Benkendorff K., Ab Lah R., Kelaher B. P. (2017). Ocean Acidification and Warming Impact the Nutritional Properties of the Predatory Whelk, Dicathais Orbita. J. Exp. Mar. Biol. Ecol. 493, 7–13. doi: 10.1016/j.jembe.2017.03.006
Tattersall G. J., Sinclair B. J., Withers P. C., Fields P. A., Seebacher F., CoopeR C. E., et al. (2012). Coping With Thermal Challenges: Physiological Adaptations to Environmental Temperatures. Comp. Physiol. 2, 2151–2202. doi: 10.1002/cphy.c110055
Timmins-Schiffman E., Coffey W. D., Hua W., Nunn B. L., Dickinson G. H., Roberts S. B. (2014). Shotgun Proteomics Reveals Physiological Response to Ocean Acidification in Crassostrea Gigas. BMC Genomics 15, 1. doi: 10.1186/1471-2164-15-951
Valles-Regino R., Mouatt P., Rudd D., Yee L. H., Benkendorff K. (2016). Extraction and Quantification of Bioactive Tyrian Purple Precursors: A Comparative and Validation Study From the Hypobranchial Gland of a Muricid Dicathais Orbita. Molecules 21, 1672. doi: 10.3390/molecules21121672
Valles-Regino R., Tate R., Kelaher B., Savins D., Dowell A., Benkendorff K. (2015). Ocean Warming and CO2-Induced Acidification Impact the Lipid Content of a Marine Predatory Gastropod. Mar. Drugs 13, 6019–6037. doi: 10.3390/md13106019
Vinagre C., Leal I., Mendonça V., Flores A. A. B. (2015). Effect of Warming Rate on the Critical Thermal Maxima of Crabs, Shrimp and Fish. J. Therm. Biol. 47, 19–25. doi: 10.1016/j.jtherbio.2014.10.012
Wahid A. (2007). Physiological Implications of Metabolite Biosynthesis for Net?A3B2 show [#,32] ?> Assimilation and Heat-Stress Tolerance of Sugarcane (Saccharum Officinarum) Sprouts. J. Plant Res. 120, 219–228. doi: 10.1007/s10265-006-0040-5
Wernberg T., Smale D. A., Tuya F., Thomsen M. S., Langlois T. J., De Bettignies T., et al. (2013). An Extreme Climatic Event Alters Marine Ecosystem Structure in a Global Biodiversity Hotspot. Nat. Clim. Change 3, 78. doi: 10.1038/nclimate1627
Westley C., Benkendorff K. (2008). Sex-Specific Tyrian Purple Genesis: Precursor and Pigment Distribution in the Reproductive System of the Marine Mollusc, Dicathais Orbita. J. Chem. Ecol. 34, 44–56. doi: 10.1007/s10886-007-9402-2
Westley C., Lewis M. C., Benkendorff K. (2010). Histomorphology of the Hypobranchial Gland in Dicathais Orbita (Gmelin 1791) (Neogastropoda: Muricidae). J. Molluscan Stud. 76, 1–10. doi: 10.1093/mollus/eyp056
Westley C. B., Vine K. L., Benkendorff K. (2006). “A Proposed Functional Role for Indole Derivatives in Reproduction and Defense of the Muricidae (Neogastropoda: Mollusca),”. Eds. Meijer L., Guyard N., Skaltsounis L., Eisenbrand G. Indirubin, the Red Shade of Indigo, (Roscoff, France: Life in Progress Editions), 31–44. 2006.
Williams G. A., Morritt D. (1995). Habitat Partitioning and Thermal Tolerance in a Tropical Limpet, Cellana Grata. Mar. Ecol. Prog. Ser. 124, 89–103. doi: 10.3354/meps124089
Williams D. H., StonE M. J., Hauck P. R., Rahman S. K. (1989). Why are Secondary Metabolites (Natural Products) Biosynthesized? J. Nat. Prod. 52, 1189–1208. doi: 10.1021/np50066a001
Keywords: ocean warming, mollusk, hypobranchial gland, murexine, brominated indoles, marine natural products
Citation: Valles-Regino R, Mouatt P, Yee LH and Benkendorff K (2022) Ocean Warming and Heat Stress Impact Molecules of Keystone Significance in a Predatory Marine Gastropod. Front. Mar. Sci. 9:830425. doi: 10.3389/fmars.2022.830425
Received: 07 December 2021; Accepted: 16 May 2022;
Published: 13 June 2022.
Edited by:
Pengzhi Qi, Zhejiang Ocean University, ChinaReviewed by:
Carolina Madeira, NOVA University Lisbon, PortugalCopyright © 2022 Valles-Regino, Mouatt, Yee and Benkendorff. This is an open-access article distributed under the terms of the Creative Commons Attribution License (CC BY). The use, distribution or reproduction in other forums is permitted, provided the original author(s) and the copyright owner(s) are credited and that the original publication in this journal is cited, in accordance with accepted academic practice. No use, distribution or reproduction is permitted which does not comply with these terms.
*Correspondence: Kirsten Benkendorff, a2lyc3Rlbi5iZW5rZW5kb3JmZkBzY3UuZWR1LmF1
†Present address: Roselyn Valles-Regino, Davao Oriental State University Dahican, Mati City, Philippines
Disclaimer: All claims expressed in this article are solely those of the authors and do not necessarily represent those of their affiliated organizations, or those of the publisher, the editors and the reviewers. Any product that may be evaluated in this article or claim that may be made by its manufacturer is not guaranteed or endorsed by the publisher.
Research integrity at Frontiers
Learn more about the work of our research integrity team to safeguard the quality of each article we publish.