- 1Old Dominion University, Department of Ocean and Earth Sciences, Norfolk, VA, United States
- 2Rutgers University, Department of Marine and Coastal Sciences, New Brunswick, NJ, United States
- 3Department of Biology Haverford College, Haverford, PA, United States
- 4University of South Florida, College of Marine Science, St. Petersburg, FL, United States
- 5University of Washington, School of Oceanography, Seattle, WA, United States
- 6Florida State University, Department of Earth, Ocean, and Atmospheric Sciences, Tallahassee, FL, United States
The cyanobacterium Trichodesmium plays an essential role supporting ocean productivity by relieving nitrogen limitation via dinitrogen (N2) fixation. The two common Trichodesmium clades, T. erythraeum and T. thiebautii, are both observed in waters along the West Florida Shelf (WFS). We hypothesized that these taxa occupy distinct realized niches, where T. thiebautii is the more oceanic clade. Samples for DNA and water chemistry analyses were collected on three separate WFS expeditions (2015, 2018, and 2019) spanning multiple seasons; abundances of the single copy housekeeping gene rnpB from both clades were enumerated via quantitative PCR. We conducted a suite of statistical analyses to assess Trichodesmium clade abundances in the context of the physicochemical data. We observed a consistent coastal vs. open ocean separation of the two clades: T. erythraeum was found in shallow waters where the concentrations of dissolved iron (dFe) and the groundwater tracer Ba were significantly higher, while T. thiebautii abundance was positively correlated with water column depth. The Loop Current intrusion in 2015 with entrained Missisippi River water brought higher dFe and elevated abundance of both clades offshore of the 50 m isobath, suggesting that both clades are subject to Fe limitation on the outer shelf. Whereas, previous work has observed that T. thiebautii is more abundant than T. erythraeum in open ocean surface waters, this is the first study to examine Trichodesmium niche differentiation in a coastal environment. Understanding the environmental niches of these two key taxa bears important implications for their contributions to global nitrogen and carbon cycling and their response to global climate change.
Introduction
Trichodesmium is an important genus of marine cyanobacteria that converts dinitrogen gas (N2) into bioaccessible ammonia via N2 fixation (Capone et al., 2005). Once fixed, new dissolved nitrogen (N) can be released, fueling local food webs (Capone, 2001) and, ultimately, playing an essential role in total ocean productivity by relieving N limitation (Carpenter and Capone, 2008). Indeed, Trichodesmium’s new N inputs are estimated at 60-80 Tg N yr (Bergman et al., 2013)—a large fraction of the ocean’s total estimated N2 fixation rate of 100-200 Tg N yr-1 (Karl et al., 2002). Thus, Trichodesmium’s N contributions to the biosphere impacts both the global N and carbon (C) cycles, and is thought to influence global carbon dioxide (CO2) sequestration on hundred-to-thousand-year timescales (Gruber and Sarmiento, 1997; Haselkorn and Buikema, 1997; Falkowski, 1998).
The Trichodesmium genus is composed of six different species, grouped into two major clades (Hynes et al., 2011; Rouco et al., 2014). Trichodesmium thiebautii and Trichodesmium erythraeum are considered the representative species of the two different clades (Rouco et al., 2014), with T. thiebautii being the more abundant clade in open ocean regions (Hynes et al., 2009; Chappell et al., 2012; Rouco et al., 2014). The groups differ in their ecophysiology, including in their optimal growth temperatures and iron (Fe) stress responses (Breitbarth et al., 2007; Chappell and Webb, 2010). Nevertheless, most culture-based studies rely on IMS101, a lab strain of T. erythraeum first isolated off the North Carolina coast roughly 30 years ago (Prufert-Bebout et al., 1993). Work with IMS101 has suggested that N2 fixation rates increase commensurately with atmospheric CO2 (Hutchins et al., 2007; Levitan et al., 2007); however, the growth and N2 fixation responses of T. thiebautii and T. erythraeum differ under rising CO2 concentrations (Hutchins et al., 2013). Understanding how the environmental sensitivities and realized niches of the two clades differ is essential to leveraging culture-based work and predicting their response to climate change.
Trichodesmium is found at tropical latitudes of the ocean (Capone et al., 2005), including along the West Florida Shelf (WFS) (Lenes et al., 2001). Surface waters beyond the 50 m isobath offshore of Tampa Bay bear low dissolved Fe (dFe) concentrations (Lenes et al., 2001; Mellett and Buck, 2020) that are highly influenced by seasonal dust deposition (Mellett and Buck, 2020). Siderophore production in open ocean Trichodesmium colonies may enable the uptake of dust Fe via siderophore-mediated dissolution, making the dust Fe readily available to species with this capability (Basu et al., 2019). However, the T. erythraeum strain IMS101 is not known to produce siderophores (Basu et al., 2019), implying that differences in the capability to produce siderophores in Trichodesmium communities may lead to niche specialization related to dust deposition.
Low Fe has been shown to limit both N2 fixation and growth of Trichodesmium (Berman-Frank et al., 2001; Kustka et al., 2003). Trichodesmium in the open ocean can be co-limited by phosphorus (P) and Fe (Mills et al., 2004; Basu et al., 2019), and has been shown to be more strongly P-limited in North Atlantic waters in comparison to the North Pacific (Sañudo-Wilhelmy et al., 2001; Sohm et al., 2008). Modeling work has shown that an increase in Fe availability can lead to N2-fixation by Trichodesmium becoming P-limited rather than Fe-limited (Ye et al., 2012). Recently it has been found that some Trichodesmium species appear to not fix N at all, presumably as a result of evolutionary adaptations to resource availability (Delmont, 2021). Little is known about the physiology of the non-N2-fixing Trichodesmium species, which have been identified solely through metagenomic analysis. Macronutrient concentrations at the WFS are negligible, and well below the half-saturation constant for phosphate uptake by Trichodesmium (Lenes et al., 2008), suggesting these trace macronutrient concentrations are likely to have little impact on clade distribution or inhibition of N2 fixation by nitrate (Knapp et al., 2012).
Previous work examining clade distributions in the open ocean showed evidence of niche separation due to potential resource competition, where T. thiebautii distributions extended from the surface down to ≥80 m, while T. erythraeum was only observed in the mixed layer of the ocean (Rouco et al., 2016). Multiple studies have found that the T. thiebautii clade is more abundant than the T. erythraeum clade in open ocean waters (Hynes et al., 2009; Chappell et al., 2012; Rouco et al., 2014; Rouco et al., 2016). Whereas, N2 fixation rates have been shown to positively correlate with dFe concentrations in T. thiebautii-dominated open ocean waters (Chappell et al., 2012), the niche preferences of the two clades and the environmental controls on their abundance remain poorly constrained in coastal areas. Here, we assess the realized niches of the primary Trichodesmium clades (T. erythraeum and T. thiebautii) along the WFS by comparing the abundance of a housekeeping gene (rnpB) diagnostic of clade identity to physical and chemical variables. By elucidating clade-level niche preferences, this work provides insight into the sensitivity of this biogeochemically important genus to climate forcings.
Methods and Materials
Hydrographic Data
Samples were collected on the R/V Weatherbird II from June 18-21 in 2015 and from April 9-12 in 2019, as well as on the R/V Hogarth from February 27- March 2 in 2018 along the WFS (Figure 1 and Supplementary Image 1). Surface salinity and temperature measurements were collected on all cruises using the ships’ flow-through hydrographic systems (SeaBird). Hydrographic variables such as sea surface salinity and sea surface height (SSH) (Figure 1) for the calendar day and region that each cruise sampled were obtained using Daily CMEMS GLORYS12V1 global reanalysis (0.083° x 0.083° resolution) (Lien et al., 2021). SSH was used to outline the edge of the Loop Current for each sampling year, while low salinity indicated waters from the Mississippi river were entrained near the edge of the Loop Current in each sampling year. Samples for chemical analyses were obtained from the surface mixed layer as described below.
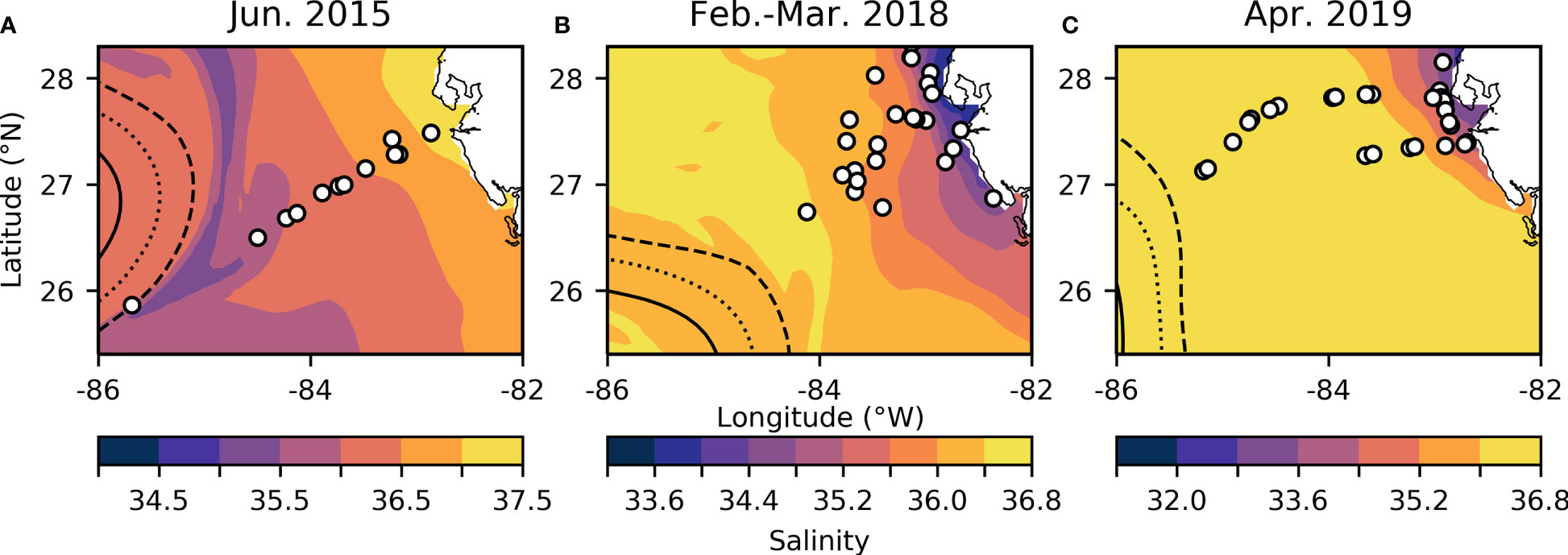
Figure 1 WFS study region for June 2015 (A), February-March 2018 (B), and April 2019 (C). Overlying sea surface salinity (note different color scales for each panel) extracted from the GLORYS12V1 reanalysis product (Lien et al., 2021). White dots indicate stations. Dashed, dotted, and solid lines indicate a SSH of 0 m, 0.2 m, and 0.4 m respectively, outlining the edge of the Loop Current.
Macronutrient and Trace Metal Analyses
Surface (~2 m) dFe, barium (Ba), and macronutrient samples were collected using a trace metal clean “towfish” system (Mellett and Buck, 2020). The specific sampling system and analytical details for trace metal and macronutrient concentration measurements are described in Mellett and Buck (2020). Briefly, trace metal samples were collected with a towfish sampling system and in-line filtered through 0.2 µm Acropak capsule filters into acid-cleaned low-density polyethylene bottles (dFe and Ba) or polypropylene tubes (silicic acid, nitrate+nitrite, and soluble reactive phosphorus, Si, N+N, and PO4, respectively); samples for dissolved trace metals were acidified to pH ~1.8 with ultrapure hydrochloric acid (Optima HCl, Fisher; final concentration 0.024 M). Macronutrient samples were stored frozen (-20 °C) until analysis on a Lachat 8500 QuickChem analyzer using colorimetric methods (Parsons et al., 1984). Phosphate (PO4) and N+N concentrations were mostly below the detection limit (Supplementary Table 1), which is consistent with long term nutrient concentration measurements from the region (Heil et al., 2014). We note that the methodology we used for analyzing macronutrients does not incorporate techniques optimized for low-level concentration analysis, so PO4 and N+N were excluded in later statistical analyses. Dissolved Fe samples were UV-oxidized, preconcentrated onto a Nobias PA1 chelating resin using an automated seaFAST-pico system, and the resulting eluents analyzed using standard addition on a Thermo Scientific Element XR Inductively Coupled Plasma Mass Spectrometer in medium resolution and counting mode at the University of South Florida (Hollister et al., 2020). Dissolved Ba was measured after a 1:50 dilution in 5% ultrapure nitric acid and quantified by standard addition within 24 hours of the dilution preparation directly on the Element XR.
DNA Sample Collection
Surface DNA samples were collected either via the “towfish” system (Mellett and Buck, 2020) or from the ship’s Niskin bottle rosette and filtered onto 0.2 μm polyethersulfone (PES) filters using a MasterFlex® peristaltic pumping system (Avantor™, Pennsylvania, USA). Up to 4 L of water was filtered, with lower volumes of water (1-2 L) collected in 2015. In 2018 and 2019, lower volumes (~1 L) were sometimes collected in the very nearshore waters because of filter clogging. In 2015, flat PES filters were stored with Qiagen® RLT Plus buffer (Qiagen, Germany), flash frozen in liquid N2 at sea, and then stored at -80°C until analysis. On the 2018 and 2019 cruises, Sterivex® cartridge filters (MilliporeSigma, Burlington, MA) were preserved in RNAlater™ (Life Technologies, Carlsbad, CA) at 4°C for approximately 12-18 hours before being secured in a dry shipper for transport and then storage at -80°C until analysis.
DNA Extraction
Filters were extracted using the Allprep RNA/DNA Mini Kit (Qiagen, Germany) following the manufacturer’s protocol with the addition of bead-beating and homogenization using the QIAshredder® column (Qiagen, Germany). All extractions were performed in a HEPA filtered UV sterilized AC600 PCR workstation (AirClean® Systems, Creedmore, NC). For samples collected on Sterivex® filters, ethanol cleaned PVC pipe cutters were used to open the Sterivex® tube. The filter was then cut out with autoclave-sterilized scalpel blades into two parts, then placed into RLT+ Buffer tubes. DNA samples were eluted in 80 µL buffer EB and stored at -80°C until qPCR analysis.
Quantitative Polymerase Chain Reaction
An established qPCR procedure was used for distinguishing between the two primary Trichodesmium clades (Rouco et al., 2014). Clade-specific primers targeted the single-copy housekeeping gene rnpB, which encodes for ribonuclease P and is frequently used in qPCR studies of cyanobacteria (Chappell and Webb, 2010; Rouco et al., 2014). Quantitative PCR amplification was performed using a Step One Plus Real Time PCR thermal cycler (Life Technologies, Carlsbad, CA). The only deviation from the original protocol (Chappell and Webb, 2010; Rouco et al., 2014) was that clade-specific gene abundances were absolutely quantified using standard curves prepared from T. erythraeum and T. thiebautii rnpB plasmids generated and quantified following the protocol of Chappell and Webb (2010). All standards, no-template controls, and samples were measured in triplicate with PowerSYBR® Green Mastermix fluorescent dye (Life Technologies, Carlsbad, CA) using 96-well plates. No-template control wells, used to monitor contamination, contained the qPCR master-mix, clade-specific primers, and Rnase-free water. Samples were run as 1:10 dilutions of the original extracted DNA with 2 µL of sample in a 20 µL reaction. Melt curves were incorporated to ensure that single products were successfully amplified (Rouco et al., 2014) and all qPCR efficiencies were above 93%.
Standard curves were created in triplicate with known gene abundances of serially diluted plasmid standards of each clade. The critical threshold, the point at which fluorescence intensity crosses the detectible level and corresponds to the initial abundance of DNA in samples (Kralik and Ricchi, 2017), was determined by the Step One Plus software and values from the triplicate qPCR reactions for each unknown were averaged and compared with the standard curve. Limits of detection and limits of quantification were determined for each sample as described in Selden et al. (2021). In subsequent statistical analyses, we chose a conservative approach whereby samples that were below detection limit (BDL, < 3 gene copies/PCR) were given the value of zero and samples that were detectable but unquantifiable (BQL, <10 gene copies/PCR) were given the value that was the limit of detection. Effective limit of quantification (ELOQ) are defined as 2000-3125 copies/L in 2015, 948-5000 copies/L in 2018, and 625 – 2000 copies/L in 2019.
Statistical Analyses
Kolmogorov-Smirnov tests were performed to test residuals for normality; however, residuals did not follow a normal distribution. Consequently, non-parametric tests (Spearman’s Correlation and Kruskal-Wallis) using MATLAB, R2020a and ordination statistics [canonical correspondence analysis (CCA)] using the VEGAN package in RStudio (Dixon, 2003), were employed to compare clade abundances and correlations with environmental data. CCA was done with only one constrained axis. Environmental data evaluated for correlation with Trichodesmium spp. clades included dFe, Si, and Ba concentrations, as well as salinity and water column depth. Water column depths were obtained from ETOPO1 (bedrock) bathymetry data grid extraction (Amante and Eakins, 2009).
Results and Discussion
Physiochemical Data
On the WFS, surface dFe concentrations were highest near the coast (mean = 3.84 ± 3.14 nM, Table 1) and declined towards the shelf-break where waters were more oceanic (mean = 0.70 ± 0.62 nM, Table 1, Figures 2A–C and Supplementary Table 1). Areas near the shelf-break and west of the 50 m isobath are defined as offshore, while areas on the mid-shelf with bottom depths <50 m are defined as inshore (Figure 2). Concentrations of dFe were negatively correlated with water column depth (Spearman: 5.07x10-9, Rho = -0.736). The dFe concentrations were likely elevated in surface waters on the shelf due to proximity to continental sources, including riverine and submarine groundwater discharge (SGD), and shallow water column mixing with shelf sediments.

Table 1 Average values and standard deviations of physiochemical data from 2019 (first two columns) and from all three cruises combined (last two columns).
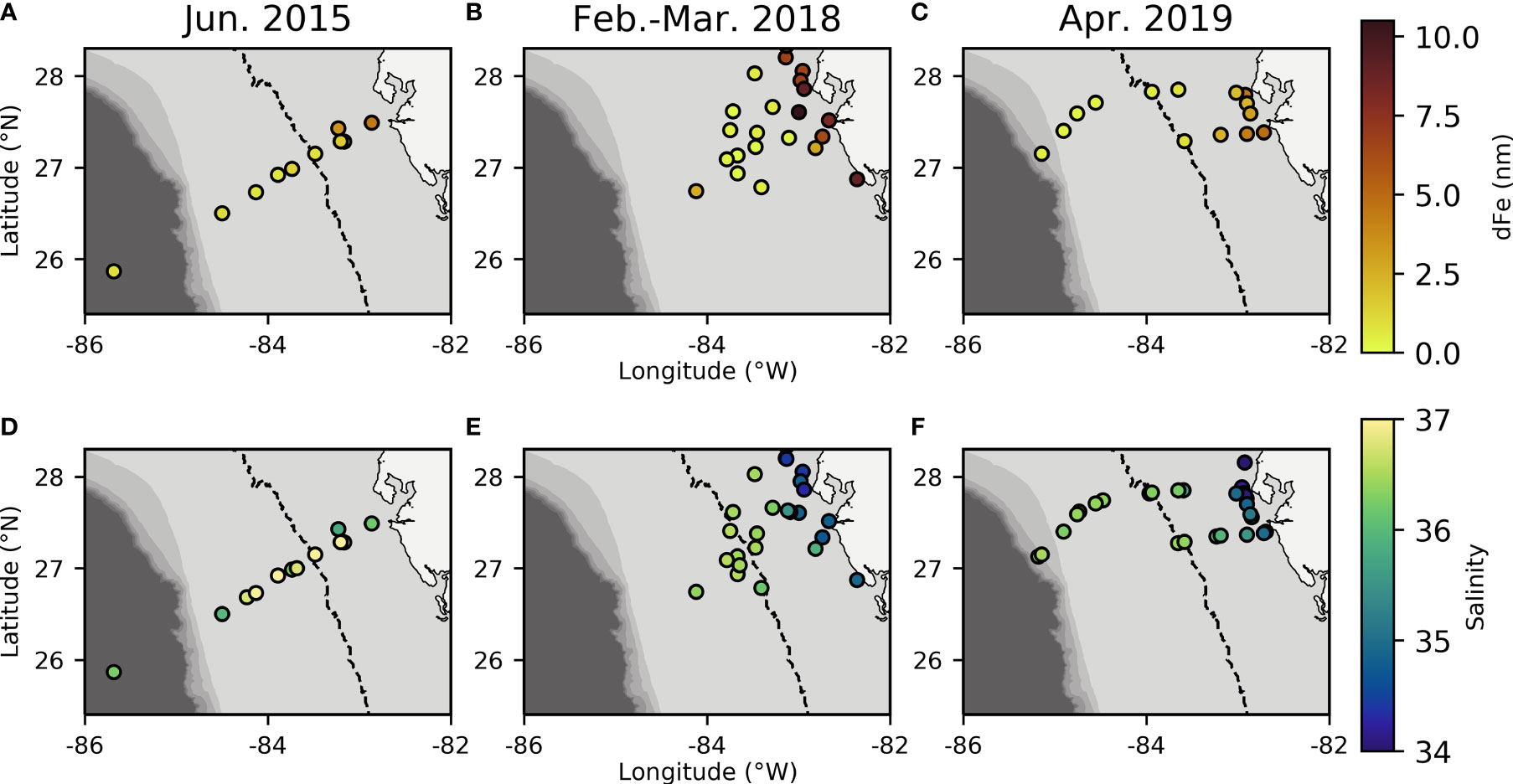
Figure 2 Dissolved Fe concentration (A–C) and salinity (D–F) measurements from each sampling year plotted over bathymetry extracted from ETOPO1 bedrock (Amante and Eakins, 2009). Dashed line indicates the 50m isobath which is used to distinguish between offshore and inshore samples and indicates where there are known SGD inputs inshore of this area (Hu et al., 2006).
We used Ba and Si to trace continental runoff sources, including inputs from rivers and SGD (Shaw et al., 1998; Oehler et al., 2019). Continental runoff can deliver essential nutrients to macro- and microbiota living in coastal areas (Charette et al., 2013; Wang et al., 2018), and SGD in particular has been previously observed to be important on the inner WFS (<50 m) (Hu et al., 2006). As expected, Ba and Si were more abundant at stations with shallower bottom depths (Ba- Spearman: 1.38x10-12, Rho=-0.817, Si- Spearman: 1.48x10-8, Rho=-0.711, Table 1) and were also positively correlated with one another (Spearman: 3.48x10-07, Rho=0.659), supporting the hypothesis that continental runoff was an important source of these elements on the inner shelf at the time of this study.
Based on salinity and SSH, we collected samples at or near the Loop Current edge on all three cruises (Figure 1) (Mellett and Buck, 2020). The Loop Current originates from warm Caribbean waters, enters the Gulf of Mexico through the Yucatan Channel and exits via the Florida Strait (Morrison et al., 1983). This water mass has been shown to be low in trace metals and macronutrients, but serves as a physical vector for bringing elevated dFe concentrations to the outer WFS region, by entrainment of Mississippi River plume water along the edge of the Loop that is advected south to the WFS (Mellett and Buck, 2020). We observed evidence of this entrainment across all three of our cruises (Figures 1, 2), though the effect on offshore dFe was most pronounced in 2018 against the backdrop of much lower wintertime surface dFe (Figure 2B).
Trichodesmium Clade Gene Abundance in Coastal vs. Offshore Samples
Our results support a distinct niche distribution between the two Trichodesmium clades along the WFS, where T. erythraeum dominates the inner shelf (Kruskal-Wallis: p=0.0242, Chisq=5.08, df=66, std.dev=200,427) and T. thiebautii dominates the outer shelf (Kruskal-Wallis: p=0.000439, Chi-sq=12.4, df=66, std.dev=214,000) (Figure 3). Absolute gene abundances of T. thiebautii consistently averaged between 1.0 x105-1.0 x106 gene copies/L offshore of the 50 m isobath (Figures 3A–C), comparable with the average cells per L seen in prior studies at further offshore surface waters of the Atlantic (Rouco et al., 2014). In many inshore stations, T. thiebautii was below detection limits (Supplementary Table 1). T. erythraeum, on the other hand, had average ranges of 1.0 x105-1.0 x106 gene copies/L inshore of the 50 m isobath (Figures 3G–I). In many offshore stations, T. erythraeum was not detectable (Supplementary Table 1). Notably, T. erythraeum was not detected inshore during the 2015 cruise (Figure 3G), but we ascribe this anomaly to a higher detection limit for that specific sampling event that precluded quantification (Figure 3G, Supplementary Table 1).
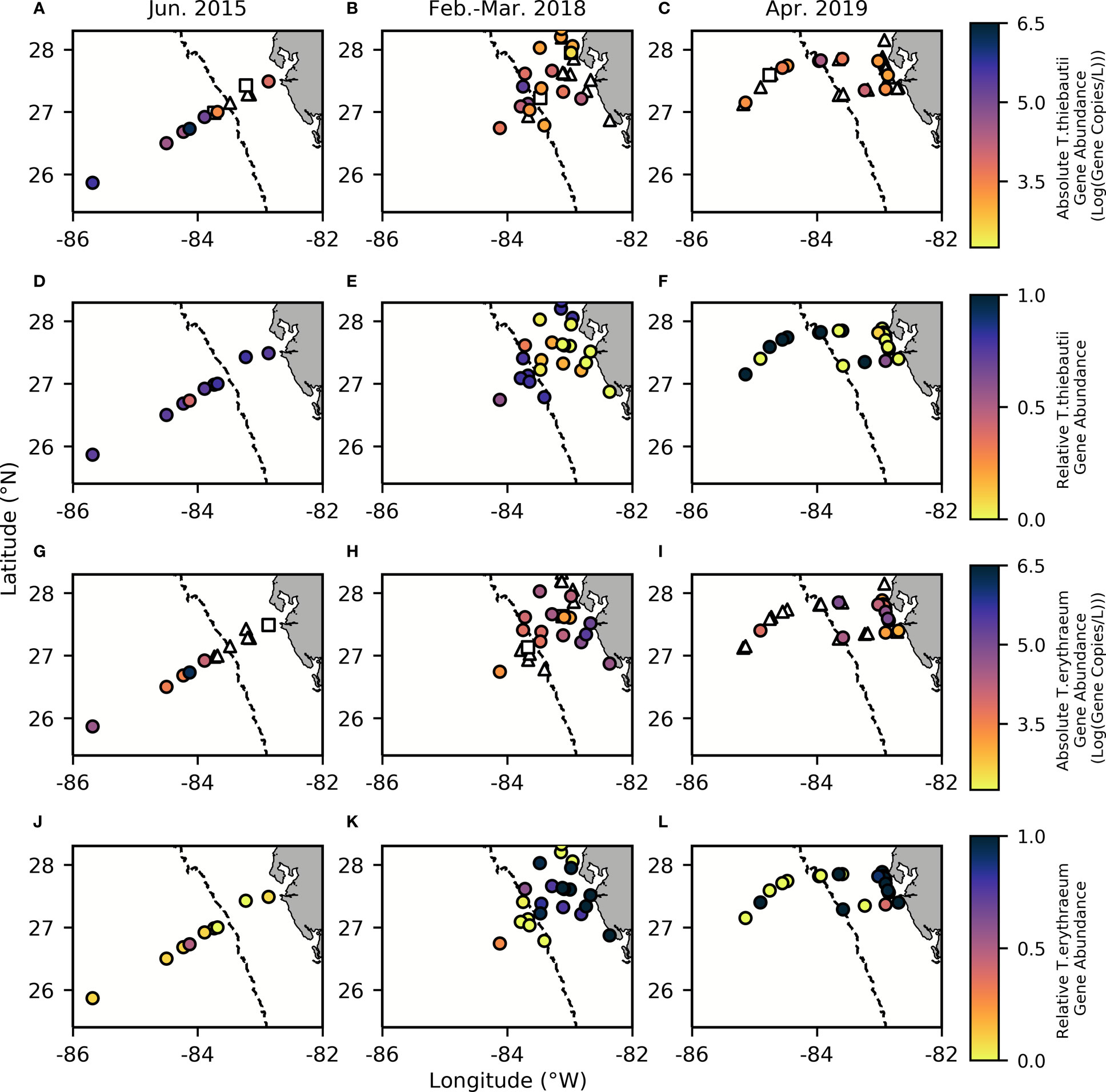
Figure 3 Absolute gene abundance for T. thiebautii (A–C) and T. erythraeum (G–I), as well as relative gene abundance for T. thiebautii (D–F) and T. erythraeum (J–L) with contours reflecting bathymetry extracted from ETOPO1 bedrock (Amante and Eakins, 2009). In all panels, the dashed line indicates the 50 m isobath, which is used to distinguish between offshore and inshore samples and indicates where there are known SGD inputs inshore of this area (Hu et al., 2006). Triangles represent Trichodesmium spp. abundances below the detection limit (BDL), while squares represent data that is below the quantification limit (BQL). (Effective limit of quantification (ELOQ): 2000-3125 copies/L in 2015, 948-5000 copies/L in 2018, and 625 – 2000 copies/L in 2019).
Altogether, our results support prior studies suggesting that T. thiebautii is the more oceanic clade (Chappell et al., 2012). It is important to note that on each cruise, stations sampled close to the Loop Current had elevated abundances of both Trichodesmium clades, which may have contributed to a higher mean abundance at offshore stations in 2015 (Figure 3). This may have resulted from the edge of the Loop Current delivering dFe and other nutrients to the shelf from entrainment of Mississippi River plume water as well as simultaneous dust deposition offshore in this season. The known SGD inputs inshore of the 50 m isobath (Hu et al., 2006) may also contribute to the differences in gene abundances and perceived environmental niches.
Before evaluating physicochemical drivers of clade distributions, we focused on water column depth as a way to distinguish between inshore and offshore samples. Water column depth and gene abundances were compared using multiple non-parametric tests (Spearman correlation and Kruskal-Wallis tests) to ensure that results were consistent regardless of analytical tool. Water column depth and T. thiebautii abundance were positively correlated (Spearman: p=0.0009, Rho=0.4063) while bottom depth and T. erythraeum abundance were negatively correlated (Spearman: p=0.0123, Rho=-0.3114). T. erythraeum was most often observed in waters <50 m depth. Based on these initial analyses, we distinguished stations deeper than 50 m as ‘offshore’ and those shallower as ‘inshore’. This distinction was used in subsequent statistical analyses. Additionally, prior work has indicated that WFS waters <50 m are more influenced by SGD (Hu et al., 2006), which is consistent with the elevated dFe and groundwater tracers observed inshore of the 50 m isobath (Figures 2, 3, Table 1).
Gene Abundance Correlations With Physiochemical Data
To identify potential drivers of niche differentiation between the clades that might explain differences between inshore and offshore distributions, we examined correlations between each clade’s gene abundance with a number of physicochemical variables. Spearman correlations were employed to evaluate clade abundances with physiochemical data, while CCA was used to evaluate associations between both clades and environmental parameters. Using CCA, significant correlations were found between the two clades and dFe and Ba concentrations, as well as water column depth (Figure 4). Significant positive correlations were found between salinity and T. thiebautii abundance (Spearman Correlation (right tail): p= 0.0003, Rho=0.4079), consistent with this being the more oceanic clade as salinity increased offshore (Figures 2D–F). In the CCA analysis, there was no significant correlation between the abundance of both clades with salinity; rather, water column depth was significantly correlated with the abundance of both the clades (Figure 4). This is consistent with T. thiebautii being the more oceanic clade as water column depth increased, while T. erythraeum is the more coastal clade associated with shallower water columns.
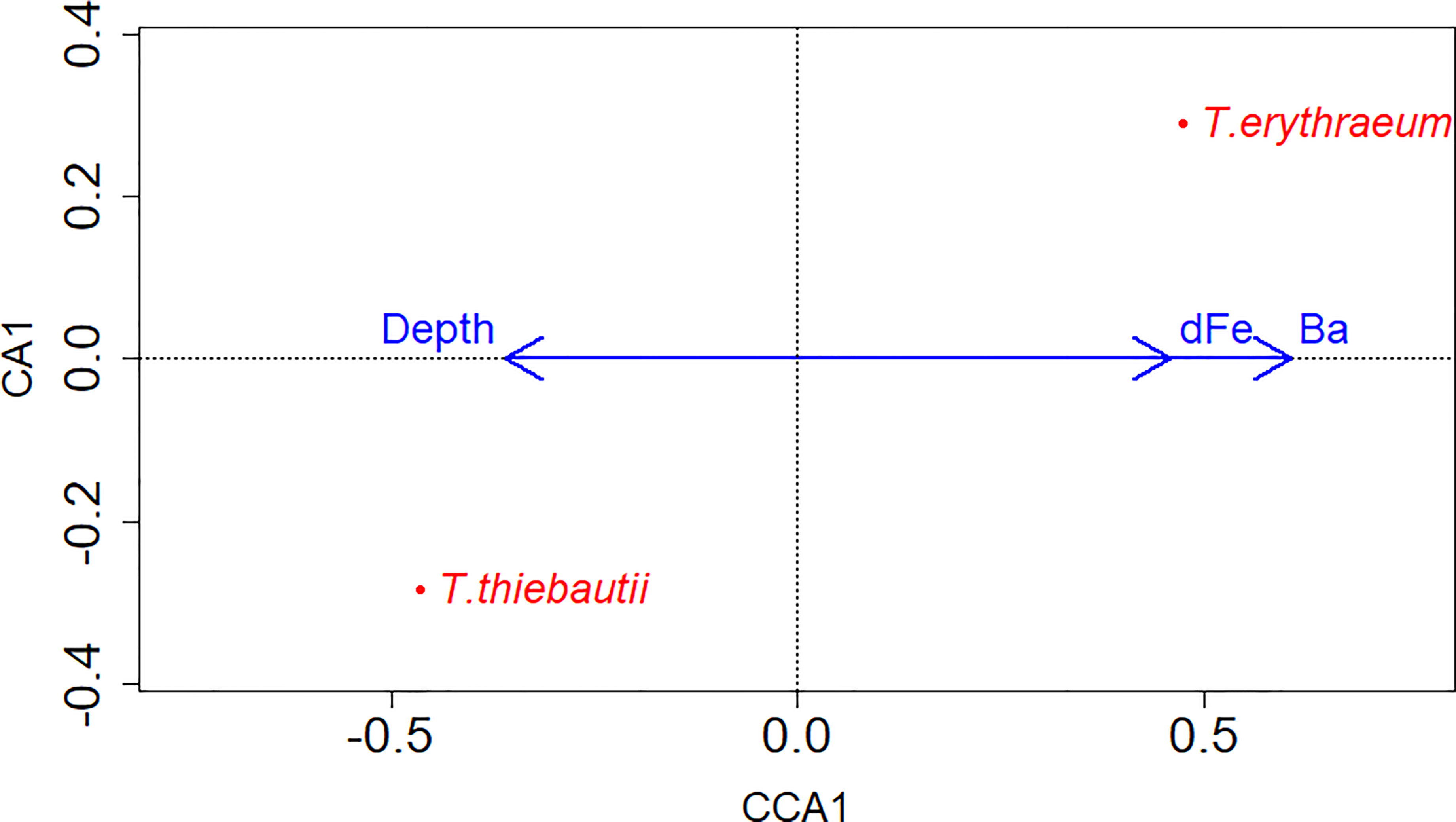
Figure 4 CCA model of gene abundances of both clades in relation to physiochemical data and water column depth (extracted from ETOPO1 bedrock (Amante and Eakins, 2009). Clades are labeled on the data points, while blue arrows show the x-axis directionality of physiochemical data and environmental parameters. There was only one constrained axis as there were only two clades of interest. Significant environmental parameters and the two clades included dFe (nM) (p-value= 0.001, Chi-square=0.116), Water column depth (m) (p-value=0.001,Chi-square=0.058), and Ba (nM) (p-value=0.001, Chi-square=0.490).
Niche differentiation may also be related to ecological competition associated with dFe availability. In support of this hypothesis, T. thiebautii was negatively correlated with dFe concentration (Spearman Correlation, Table 2). CCA, but not Spearman, revealed a correlation between dFe and clade abundances (Figure 4), where T. erythraeum was positively correlated with dFe, but negative correlated with T. thiebautii. Prior studies have shown that in Fe-limited open ocean waters, T. thiebautii is the dominant Trichodesmium clade (Chappell and Webb, 2010); our results suggest that when dFe is higher inshore, the oceanic Trichodesmium clade representative, T. thiebautii, is outcompeted by T. erythraeum.
We proffer that this trend is driven by competition with T. erythraeum and other phytoplankton inshore, meaning that T. thiebautii is outcompeted by T. erythraeum when dFe from SGD and/or other sources is elevated inshore. This is supported by the observations that T. thiebautii was also negatively correlated with Ba and Si concentrations (Spearman Correlation, Table 2), while dFe and Ba were positively correlated with T. erythraeum (CCA, Figure 4), in particular during the Loop Current intrusion in 2015. The Loop Current intrusion resulted in enhanced offshore T. erythraeum abundance in 2015 (Figure 3G and Supplementary Table 1). However, we do not see T. erythraeum abundance elevated offshore in 2018 (Figure 3E and Supplementary Table 1), even though there was elevated dFe offshore as a result of the Loop Current intrusion (Figure 2B and Supplementary Table 1). This suggests that T. erythraeum is also Fe-limited offshore of the 50 m isobath on the WFS, potentially due to the lack of siderophore production mechanisms that open ocean Trichodesmium colonies have (Basu et al., 2019), except in situations where entrainment of dFe from continental runoff sources is carried by Loop Current to offshore locations.
Conclusion
Our findings show that the two main Trichodesmium clades, T. thiebautii and T. erythraeum, occupy distinct realized niches on the WFS. T. thiebautii is the more oceanic clade: its abundance was elevated in samples collected at deeper stations (>50 m), with elevated salinity and lower dFe. T. erythraeum is more coastal: it was most abundant at shallow stations with significant continental runoff inputs and higher dFe, and appears to outcompete T. thiebautii in coastal regions. These distinct niche occupations are likely due to resource competition between the two clades at stations with higher dFe. More work is needed to identify the metabolic pathways that distinguish the two clades and lead to the observed distinctions in distribution patterns. The intrusion of the Loop Current and associated entrainment of Missisippi River water brought higher dFe and elevates gene abundances of the two clades offshore of the 50 m isobath, suggesting that both clades are subject to Fe limitation on the outer shelf. Understanding the environmental niches of these two key taxa bears important implications for their contributions to global N and C cycling and their response to global climate change.
Data Availability Statement
The datasets analyzed for this study is available for direct download (Supplementary Table 1) and are archived in the Biological and Chemical Oceanography Data Management Office (https://www.bco-dmo.org/project/814733).
Author Contributions
ANK, KNB, and PDC designed the sampling program. CRS, KAC, and PDC designed the Trichodesmium analysis plan. All authors were involved in sample collection and/or analysis. KAC, CRS, and PDC wrote the initial manuscript draft. All other authors contributed to manuscript editing and approved the final manuscript.
Funding
Funding for this study was provided by Old Dominion University, the Jeffress Trust Awards Program in Interdisciplinary Research, a FSU Planning Grant (ANK), the National Science Foundation through the Research Experience for Undergraduates Program OCE-1659543 (ODU) and OCE-2148812 to PDC, and subsidized ship-time from the Florida Institute of Oceanography to KNB and ANK.
Conflict of Interest
The authors declare that the research was conducted in the absence of any commercial or financial relationships that could be construed as a potential conflict of interest.
Publisher’s Note
All claims expressed in this article are solely those of the authors and do not necessarily represent those of their affiliated organizations, or those of the publisher, the editors and the reviewers. Any product that may be evaluated in this article, or claim that may be made by its manufacturer, is not guaranteed or endorsed by the publisher.
Acknowledgments
We thank the Captain and crews of the R/V Weatherbird II and R/V Hogarth. We also thank Zuzanna Abdala, Brooke Barber, Chelsea Bonnain Chase, Adrienne Hollister, Zhou Liang, Carlos Miranda, Eric Rabinowitz, and Rachel Thomas for their help with field sampling, Sveinn Einarsson for assistance with Python coding, and Gabe Browning for analyzing Ba in a subset of these samples.
Supplementary Material
The Supplementary Material for this article can be found online at: https://www.frontiersin.org/articles/10.3389/fmars.2022.821655/full#supplementary-material
References
Amante C., Eakins B. W. (2009). ETOPO1 1 ArcMinute Global Relief Model: Procedures, Data Sources and Analysis (NOAA Technical Memorandum NMFS F/SPO: National Marine Fisheries Service, NOAA). Boulder, Colorado.
Basu S., Gledhill M., De Beer D., Prabhu Matondkar S. G., Shaked Y. (2019). Colonies of Marine Cyanobacteria Trichodesmium Interact With Associated Bacteria to Acquire Iron From Dust. Commun. Biol. 2 (1), 284. doi: 10.1038/s420030190534z
Bergman B., Sandh G., Lin S., Larsson J., Carpenter E. J. (2013). Trichodesmium a Widespread Marine Cyanobacterium With Unusual Nitrogen Fixation Properties. FEMS Microbiol. Rev. 37 (3), 286–302. doi: 10.1111/j.15746976.2012.00352.x
Berman Frank I., Cullen J. T., Shaked Y., Sherrell R. M., Falkowski P. G. (2001). Iron Availability, Cellular Iron Quotas, and Nitrogen Fixation in Trichodesmium. Limnol. Oceanogr. 46 (6), 1249–1260. doi: 10.4319/lo.2001.46.6.1249
Breitbarth E., Oschlies A., Laroche J. (2007). Physiological Constraints on the Global Distribution of Trichodesmium-Effect of Temperature on Diazotrophy. Biogeosciences 4 (1), 53–61. doi: 10.5194/bg4532007
Capone D. G. (2001). Marine Nitrogen Fixation: What's the Fuss? Curr. Opin. Microbiol. 4 (3), 341–348. doi: 10.1016/s1369-5274(00)00215-0
Capone D. G., Burns J. A., Montoya J. P., Subramaniam A., Mahaffey C., Gunderson T., et al. (2005). Nitrogen Fixation by Trichodesmium Spp.: An Important Source of New Nitrogen to the Tropical and Subtropical North Atlantic Ocean. Global Biogeochem. Cycles 19 (2), 1–14. doi: 10.1029/2004GB002331
Carpenter E. J., Capone D. G. (2008). Nitrogen Fixation in the Marine Environment (New York:Elsevier), 141–198.
Chappell P. D., Moffett J. W., Hynes A. M., Webb E. A. (2012). Molecular Evidence of Iron Limitation and Availability in the Global Diazotroph Trichodesmium. ISME J. 6 (9), 1728–1739. doi: 10.1038/ismej.2012.13
Chappell P. D., Webb E. A. (2010). A Molecular Assessment of the Iron Stress Response in the Two Phylogenetic Clades of Trichodesmium. Environ. Microbiol. 12 (1), 13–27. doi: 10.1111/j.14622920.2009.02026.x
Charette M. A., Henderson P. B., Breier C. F., Liu Q. (2013). Submarine Ground water Discharge in a River Dominated Florida Estuary. Mar. Chem. 156, 3–17. doi: 10.1016/j.marchem.2013.04.001
Delmont T. O. (2021). Discovery of Nondiazotrophic Trichodesmium; Species Abundant and Widespread in the Open Ocean. Proc. Natl. Acad. Sci. 118 (46), e2112355118. doi: 10.1073/pnas.2112355118
Dixon P. (2003). VEGAN, a Package of R Functions for Community Ecology. J. Veg. Sci. 14 (6), 927–930. doi: 10.1111/j.16541103.2003.tb02228.x
Falkowski P. G. (1998). The Oceanic Photosynthetic Engine: Origins, Evolution, and Role in Global Biogeochemical Cycles (Netherlands: Springer), 3941–3947.
Gruber N., Sarmiento J. L. (1997). Global Patterns of Marine Nitrogen Fixation and Denitrification. Global Biogeochem. Cycles 11 (2), 235–266. doi: 10.1029/97GB00077
Haselkorn R., Buikema W. J. (1997). Heterocyst Differentiation and Nitrogen Fixation in Cyanobacteria (Berlin Heidelberg: Springer), 163–166.
Heil C. A., Dixon L. K., Hall E., Garrett M., Lenes J. M., Oneil J. M., et al (2014). Blooms of Karenia Brevis on the West Florida Shelf: Nutrient Sources and Potential Management Strategies Based on a MultiYear Regional Study. Harmful Algae 38, 127–140. doi: 10.1016/j.hal.2014.07.016
Hollister A. P., Kerr M., Malki K., Muhlbach E., Robert M., Tilney C. L., et al. (2020). Regeneration of Macronutrients and Trace Metals During Phytoplankton Decay: An Experimental Study. Limnol. Oceanogr. 65 (8), 1936–1960. doi: 10.1002/lno.11429
Hu C., Muller Karger F. E., Swarzenski P. W. (2006). Hurricanes, Submarine Groundwater Discharge, and Florida's Red Tides. Geophys. Res. Lett. 33 (11), 1–4. doi: 10.1029/2005GL025449
Hutchins D. A., Fu F.X., Webb E. A., Walworth N., Tagliabue A. (2013). Taxon Specific Response of Marine Nitrogen Fixers to Elevated Carbon Dioxide Concentrations. Nat. Geosci. 6 (9), 790–795. doi: 10.1038/ngeo1858
Hutchins D. A., Fu F. X., Zhang Y., Warner M. E., Feng Y., Portune K., et al (2007). CO2 Control of TrichodesmiumN2 Fixation, Photosynthesis, Growth Rates, and Elemental Ratios: Implications for Past, Present, and Future Ocean Biogeochemistry. Limnol. Oceanogr. 52 (4), 1293–1304. doi: 10.4319/lo.2007.52.4.1293
Hynes A. M. (2009). Diversity of the marine Cyanobacterium Trichodesmium: Characterization of the Woods Hole Culture Collection and Quantification of Field Populations. Dissertation. Cambridge (MA): MIT/WHOI Joint Program.
Hynes A. M., Webb E. A., Doney S. C., Waterbury J. B. (2011). Comparison of Cultured Trichodesmium (Cyanophyceae) With Species Characterized From the Field. J. Phycol. 48 (1), 196–210. doi: 10.1111/j.1529-8817.2011.01096.x
Karl D., Michaels A., Bergman B., Capone D., Carpenter E., Letelier R., et al. (2002). Dinitrogen Fixation in the World's Oceans. Biogeochemistry 57 (1), 47–98. doi: 10.1023/A:1015798105851
Knapp A. N., Dekaezemacker J., Bonnet S., Sohm J. A., Capone D. G. (2012). Sensitivity of Trichodesmium Erythraeum and Crocosphaera Watsonii Abundance and N2 Fixation Rates to Varying NO3- and PO43- Concentrations in Batch Cultures. Aquat. Microb. Ecol. 66, 223–236. doi: 10.3354/ame01577
Kralik P., Ricchi M. (2017). A Basic Guide to Real Time PCR in Microbial Diagnostics: Definitions, Parameters, and Everything. Front. Microbiol. 8, 108–108. doi: 10.3389/fmicb.2017.00108
Kustka A. B., Saudo-Wilhelmy S. A., Carpenter E. J., Capone D., Burns J., Sunda W. G. (2003). Iron Requirements for Dinitrogen and Ammonium Supported Growth in Cultures of Trichodesmium (IMS 101): Comparison With Nitrogen Fixation Rates and Iron: Carbon Ratios of Field Populations. Limnol. Oceanogr. 48 (5), 1869–1884. doi: 10.4319/lo.2003.48.5.1869
Lenes J. M., Darrow B. P., Cattrall C., Heil C. A., Callahan M., Vargo G. A., et al. (2001). Iron Fertilization and the Trichodesmium Response on the West Florida Shelf. Limnol. Oceanogr. 46 (6), 1261–1277. doi: 10.4319/lo.2001.46.6.1261
Lenes J. M., Darrow B. A., Walsh J. J., Prospero J. M., He R., Weisberg R. H., et al (2008). Saharan Dust and Phosphatic Fidelity: A Three Dimensional Biogeochemical Model of Trichodesmium as a Nutrient Source for Red Tides on the West Florida Shelf. Continental Shelf Res. 28 (9), 1091–1115. doi: 10.1016/j.csr.2008.02.009
Levitan O., Rosenberg G., Setlik I., Setlikova E., Grigel J., Klepetar J., et al. (2007). Elevated CO2 Enhances Nitrogen Fixation and Growth in the Marine Cyanobacterium Trichodesmium. Global Change Biol. 13 (2), 531–538. doi: 10.1111/j.13652486.2006.01314.x
Lien V. S., Nilsen J. E., Perivoliotis L., Sotiropoulou M., Denaxa D., Ehrhart S., et al. (2021). BioGeoChemical Product Provided by the Copernicus Marine Service (Göttingen, Germany: Copernicus GmbH).
Mellett T., Buck K. N. (2020). Spatial and Temporal Variability of Trace Metals (Fe, Cu, Mn, Zn, Co, Ni, Cd, Pb), Iron and Copper Speciation, and Electroactive Fe Binding Humic Substances in Surface Waters of the Eastern Gulf of Mexico. Mar. Chem. 227, 103891. doi: 10.1016/j.marchem.2020.103891
Mills M., Ridame C., Davey M., Laroche J., Geider R. (2004). Iron and Phosphorus Co-Limit Nitrogen Fixation in the Eastern Tropical North Atlantic. Nature 429, 292–294. doi: 10.1038/nature02550
Morrison J. M., Merrell W. J., Key R. M., Key T. C. (1983). Property Distributions and Deep Chemical Measurements Within the Western Gulf of Mexico. J. Geophys. Res. 88 (C4), 2601. doi: 10.1029/JC088iC04p02601
Oehler T., Tamborski J., Rahman S., Moosdorf N., Ahrens J., Mori C., et al. (2019). DSi as a Tracer for Submarine Groundwater Discharge. Front. Mar. Sci. 6. doi: 10.3389/fmars.2019.00563
Parsons T. R., Maita Y., Lalli C. M. (1984). A Manual of Chemical & Biological Methods for Seawater Analysis. Eds. Parsons T. R., Maita Y., Lalli C. M. (Amsterdam: Pergamon), xiii–xxiv.
Prufert-Bebout L., Paerl H., Lassen C. (1993). Growth, Nitrogen Fixation, and Spectral Attenuation in Cultivated Trichodesmium Species. Appl. Environ. Microbiol. 59, 1367–1375. doi: 10.1128/aem.59.5.13671375.1993
Rouco M., Haley S. T., Alexander H., Wilson S. T., Karl D. M., Dyhrman S. T. (2016). Variable Depth Distribution of Trichodesmium Clades in the North Pacific Ocean. Environ. Microbiol. Rep. 8 (6), 1058–1066. doi: 10.1111/17582229.12488
Rouco M., Warren H. J., McGillicuddy D. J., Waterbury J. B., Dyhrman S. T. (2014). Trichodesmium Sp. Clade Distributions in the Western North Atlantic Ocean. Limnol. Oceanogr. 59 (6), 1899–1909. doi: 10.4319/lo.2014.59.6.1899
Sanudo-Wilhelmy S. A., Kustka A. B., Gobler C. J., Hutchins D. A., Yang M., Lwiza K., et al (2001). Phosphorus Limitation of Nitrogen Fixation by Trichodesmium in the Central Atlantic Ocean. Nature 411 (6833), 66–69. doi: 10.1038/35075041
Selden C. R., Chappell P. D., Clayton S., Macas Tapia A., Bernhardt P. W., Mulholland M. R. (2021). A Coastal N2 Fixation Hotspot at the Cape Hatteras Front: Elucidating Spatial Heterogeneity in Diazotroph Activity via Supervised Machine Learning. Limnol. Oceanogr. 66 (5), 1832–1849. doi: 10.1002/lno.11727
Shaw T. J., Moore W. S., Kloepfer J., Sochaski M. A. (1998). The Flux of Barium to the Coastal Waters of the Southeastern USA: The Importance of Submarine Groundwater Discharge. Geochim. Cosmochim. Acta 62 (18), 3047–3054. doi: 10.1016/S00167037(98)00218X
Sohm J. A., Mahaffey C., Capone D. G. (2008). Assessment of Relative Phosphorus Limitation of Trichodesmium Spp. in the North Pacific, North Atlantic, and the North Coast of Australia. Limnol. Oceanogr. 53 (6), 2495–2502. doi: 10.4319/lo.2008.53.6.2495
Wang X., Li H., Zheng C., Yang J., Zhang Y., Zhang M., et al. (2018). Submarine Groundwater Discharge as an Important Nutrient Source Influencing Nutrient Structure in Coastal Water of Daya Bay, China. Geochim. Cosmochim. Acta 225, 52–65. doi: 10.1016/j.gca.2018.01.029
Keywords: Trichodesmium, diazotrophs, niche separation, coastal/ocean separation, West Florida Shelf, groundwater
Citation: Confesor KA, Selden CR, Powell KE, Donahue LA, Mellett T, Caprara S, Knapp AN, Buck KN and Chappell PD (2022) Defining the Realized Niche of the Two Major Clades of Trichodesmium: A Study on the West Florida Shelf. Front. Mar. Sci. 9:821655. doi: 10.3389/fmars.2022.821655
Received: 24 November 2021; Accepted: 21 April 2022;
Published: 30 June 2022.
Edited by:
Rachel Ann Foster, Stockholm University, SwedenReviewed by:
Nicola Wannicke, Leibniz Institute for Plasma Research and Technology e.V. (INP), GermanyMichael William Lomas, Bigelow Laboratory For Ocean Sciences, United States
Copyright © 2022 Confesor, Selden, Powell, Donahue, Mellett, Caprara, Knapp, Buck and Chappell. This is an open-access article distributed under the terms of the Creative Commons Attribution License (CC BY). The use, distribution or reproduction in other forums is permitted, provided the original author(s) and the copyright owner(s) are credited and that the original publication in this journal is cited, in accordance with accepted academic practice. No use, distribution or reproduction is permitted which does not comply with these terms.
*Correspondence: P. Dreux Chappell, cGRjaGFwcGVAb2R1LmVkdQ==