- 1Department of Cell Biology, Physiology, and Immunology, Universitat Autònoma de Barcelona, Barcelona, Spain
- 2Centro de Biotecnología Acuícola, Facultad de Química y Biología, Universidad de Santiago de Chile, Santiago, Chile
- 3Facultad de Medicina Veterinaria y Agronomía, Universidad de Las Américas, Santiago, Chile
- 4Centro de Genómica y Bioinformática, Facultad de Ciencias, Universidad Mayor, Santiago, Chile
- 5Escuela de Biotecnología, Facultad de Ciencias, Universidad Mayor, Santiago, Chile
- 6APC Europe SL, Granollers, Spain
- 7Aquaculture Program, Centre de Sant Carles de la Ràpita (IRTA-SCR), Institut de Recerca i Tecnologia Agroalimnetaries, Sant Carles de la Rápita, Spain
The spray-dried porcine plasma (SDPP) is an abattoir by-product used in animal nutrition with beneficial effects reported in livestock and commercial aquatic species. Previous results have found that the dietary inclusion of SDPP in gilthead sea bream (Sparus aurata) increased the density of intestinal goblet cells, and it did not result in significant changes in the autochthonous microbiota. However, there is no comprehensive data on the mechanisms that could take place on the intestine of gilthead sea bream fed with an SDPP-supplemented diet. For this reason, this study aimed to unveil the biological mechanisms modulated in response to the dietary administration of SDPP in the gilthead sea bream gut. To achieve this goal, we made a microarrays-based transcriptomic approach in gut samples from gilthead sea bream fed with an SDPP-supplemented diet for 95 days. As control diet, we used a protein-rich commercial feed (51% crude protein, 17% crude fat, and 20.6 MJ/kg gross energy) which was supplemented with 3% SDPP at the expense of LT70 fishmeal. The microarray analyses showed a total of 803 (468 up- and 335 down-regulated) differential expressed genes (DEGs). The functional network analysis revealed that dietary inclusion of SDPP induced sustained changes in 120 biological processes, grouped in 12-clusters. Among them, the metabolic-related process (cellular catabolic process, organic substance catabolic process, protein metabolism process), protein transport, and leukocyte mediated immunity interacted in the leading interactome network. This evidence confirms the previous evidence of the enhancement of the mucosal health status in response to the dietary administration of SDPP and provides further understanding of the mode of action of this ingredient in aquafeeds.
Introduction
In fish, the intestine is a complex multifunctional organ with critical physiological roles, including water and electrolyte homeostasis, endocrine modulation, metabolism and immune regulation, and commensal microbiota balance (Salinas and Parra, 2015; Khansari et al., 2018; Firmino et al., 2021c; Salomón et al., 2021). Notably, the intestine is one of the leading portals of pathogens’ entry into the organism. Accordingly, the intestine has a mucus layer that acts as a physical and chemical barrier (Parra et al., 2015); thus, protecting the gut epithelium by defense mechanisms that help for maintaining the tissue homeostasis (Ellis, 2001; Gomez et al., 2013). The intestine is considered a mucosal-associated lymphoid tissue (MALT) because of its capacity for controlling the innate and adaptive immune response at the mucosal site [also called gut-associated lymphoid tissue (GALT)] (Parra et al., 2015).
Functional feeds are achieving significant attention globally due to their growth-promoting, antimicrobial, immunostimulant, antioxidant, anti-inflammatory, and sedative properties (Reyes-Cerpa et al., 2018; Firmino et al., 2021b). Accordingly, the nutritional requirements must be fully integrated into management strategies for aquaculture (Tacchi et al., 2011). Considerable advances have been made in studying nutritional requirements and sustainable feeding in fish, with deep knowledge about the optimal dietary composition for most important cultured fish species. Furthermore, the aquaculture industry has developed functional feeds to improve growth, immune response; physiological functions, and health performance in fishes that promote specific benefits to the host beyond the primary nutritional requirement. Functional feed additives include prebiotics, probiotics, vitamins, minerals, and plant, animal, or algae extracts (Parra et al., 2015; Salinas and Parra, 2015; Khansari et al., 2018; Salomón et al., 2021). The spray-dried porcine plasma (SDPP) is an abattoir by-product used in animal nutrition due to its excellent amino acid profile and digestibility close to 99% (Bureau et al., 1999). Several studies in livestock have shown that SDPP has positive effects on productivity and physiological parameters, immunity, and gut health in pigs and poultry (Ellis, 2001; Gomez et al., 2013; Reyes-Cerpa et al., 2018; Firmino et al., 2021b). In addition, its beneficial effect has also been reported in commercial aquatic species such as rainbow trout (Oncorhynchus mykiss) (Campbell et al., 2003), gilthead seabream (Sparus aurata) (Gisbert et al., 2015; Reyes-López et al., 2021), and Nile tilapia (Oreochromis niloticus) (de Araújo et al., 2017). In these fish species, SDPP has shown a repertoire of beneficial effect attributes on the host, including an improvement in somatic growth performance, digestibility, feed intake, feed efficiency, immunity, and improved antioxidant system capacity (Johnson and Summerfelt, 2000; Gisbert et al., 2015; de Araújo et al., 2017; Reyes-López et al., 2021). Furthermore, the use of porcine blood derivatives has environmental benefits for the sustainability and circular economy associated with animal production. It helps to provide added value to the industry and has less carbon footprint than plant protein sources that may be used in aquafeeds.
The importance of the diet lies not only in its nutritional contribution to the host but also in protecting the fish by maintaining intestinal health and improving digestion and immunity (Dawood, 2021). A previous report showed the inclusion of SDPP in the diet for gilthead sea bream promoted their somatic growth and increased intestinal goblet cells’ density, and it did not result in major changes in autochthonous microbiota (Tapia-Paniagua et al., 2020). However, there is no comprehensive data on the mechanisms that could take place on the intestine of gilthead sea bream fed with an SDPP-supplemented diet. For that reason, this study aimed to unveil the biological mechanisms modulated by the dietary administration of SDPP in the gilthead sea bream gut. To do it, we used a microarrays-based transcriptomic approach to increase the coverage of those modulated processes by a high throughput screening strategy.
Materials and Methods
Ethics Statement
Animal experimental procedures were conducted in compliance with the research protocol approved by the IRTA’s Committee of Ethics and Animal Experimentation and following the Guidelines of the European Union Council (86/609/EU) for the use of laboratory animals.
Diets
Two diets were formulated as follows: (1) a control diet (Diet C) containing 51% crude protein, 17% crude fat, and 20.6 MJ/kg gross energy that fulfill the nutritional requirements of juvenile sea bream; (2) the SDPP diet was manufactured using this basal formulation, substituting Fishmeal LT70 by 3% SDPP (Apetein GS, APC Europe SL, Granollers, Spain; Table 1). The inclusion of 3% SDPP was used in this study because the evidence from a previous study showing an improved fish growth performance in terms of BWf, SLf, K, and SGRBW compared to 6% SDPP inclusion (Gisbert et al., 2015). The diets were manufactured by extrusion at SPAROS LDA (Olhão, Portugal). All powder ingredients were mixed accordingly to the target formulation in a double-helix mixer (model 500L, TGC Extrusion, France) and ground (<400 μm) in a micropulverizer hammer mill (model SH1, Hosokawa-Alpine, Germany). Diets (pellet size: 2.5 mm) were manufactured with a twin-screw extruder (model BC45, Clextral, France) with a screw diameter of 55.5 mm. Extrusion conditions were as follows: feeder rate (80–85 kg/h), screw speed (247–266 rpm), water addition in barrel 1 (345 mL/min), temperature in barrel 1 (32–34°C), temperature in barrel 2 (59–62°C) and temperature in barrel 3 (111–114°C). Extruded pellets were dried in a vibrating fluid bed dryer (model DR100, TGC Extrusion, France). After cooling, oils were added by vacuum coating (model PG-10VCLAB, Dinnissen, Netherlands). Coating conditions were: pressure (700 mbar), spraying time under vacuum (approximately 90 s), and return to atmospheric pressure (120 s). Immediately after coating, diets were packed in sealed plastic buckets and shipped to IRTA research facilities (Sant Carles de la Ràpita, Spain).
Fish and Experimental Design
Gilthead seabream fry (average body size 9.5 g) were obtained from a commercial hatchery (Piscimar SL, Andromeda Group, Spain) and transported by road to IRTA-Sant Carles de la Ràpita research facilities (Sant Carles de la Ràpita, Spain). Fish were acclimated for 2 weeks into 2,000-L tanks. After their acclimation period, all fish were anesthetized [tricaine methanesulfonate (MS-222), 150 mg/L] and individually weighed for initial body weight (BWi) and measured for standard length (SLi) to the nearest 0.1 g and 1 mm, respectively. Then, fish were distributed into eight 500-L cyclindroconical tanks at a density of 50 fish per tank (4 tanks/replicates per diet).
Fish (BWi = 10.6 ± 0.1 g, n = 400, mean ± standard deviation, SD) were fed during 95 days with C and SDPP diets using automatic feeders (ARVO-TEC T Drum 2000; Arvotec, Huutokosk, Finland) at a rate of 2.5% of the stocked biomass, which approached apparent satiation. The daily feed ratio was evenly distributed in 7 meals per day from 08:00 to 18:00 h. During the trial, water temperature and pH (pH meter 507; Crison Instruments, Barcelona, Spain), salinity (MASTER-20T; ATAGO Co., Ltd., Tokyo, Japan), and dissolved oxygen (OXI330; Crison Instruments) were 22.1 ± 0.4°C, 7.0 ± 0.01, 36 mg/L, and 7.2 ± 0.3 mg/L (mean ± SD), respectively. The water flow rate was maintained at approximately 9.0–10.1 L/min in a recirculation system (IRTAmar®; Spain) that provided the experimental tanks with adequate water quality (total ammonia and nitrite were ≤ 0.15 and 0.6 mg/L, respectively) through UV, biological, and mechanical filtration. Photoperiod followed natural changes according to the season of the year (November to February; 40°37′41″ N). Fish were regularly sampled monthly to evaluate their growth in BW and adjust the feeding ratio to stocked biomass.
At the end of the trial, fish were anesthetized as described above. All fish in experimental tanks were measured for final BW and SL as indicated. Then, 10 fish per tank were sacrificed with an overdose of MS-222 (200 mg/L) for tissue sampling purposes. The intestinal cavity was exposed, and the intestine was removed with a scalpel. A small piece of the anterior intestine (0.5 cm) was dissected and immediately transferred into RNAlater (Ambion®) incubated at 4°C overnight and frozen at −80°C until further RNA extraction. The anterior intestine was chosen because of its immunological relevance in comparison with other intestinal sections (Oehlers et al., 2011; Salomón et al., 2021). Fish growth and feed utilization from Control and SDPP groups were evaluated using the following indices: Fulton’s condition factor (K) = (BW/SL3) × 100; specific growth rate in BW (SGR,%) = [(ln BWf −ln BWi) × 100]/time (d) and feed conversion ratio (FCR, g/g) = FI/(Bf −Bi), where FI was the total feed intake during the experimental period (g) and, Bi and Bf were the initial and final biomass (g), respectively. In particular, BWf and SGR values were 6.2 and 4.1% higher in fish fed the SDPP diet compared to the control group (p < 0.05), respectively. No significant differences were found in terms of standard length (SL) and the condition factor K (p > 0.05). In addition, FCR values were lower in fish fed the SDPP diet in comparison to those fed the control diet (p < 0.05) (Table 2). These data have been previously reported elsewhere (Reyes-López et al., 2021).
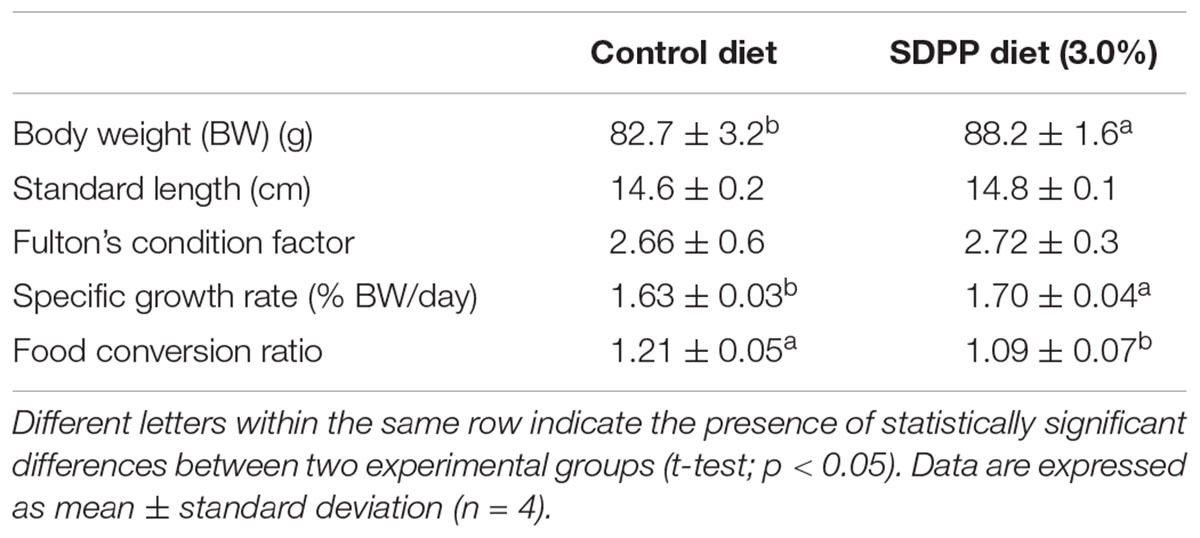
Table 2. Somatic growth and feed efficiency in gilthead sea bream (Sparus aurata) fed diets containing spray-dried porcine plasma (SDPP).
Transcriptional Analysis
Transcriptomic analysis was conducted to determine differences in gene expression level in the gut of the fish feed the control and SDPP diets at the end of the feeding trial (95 days). In particular, total RNA was extracted individually from fish gut using QIAGEN RNeasy® Mini Kit following the manufacturer’s recommendations. Total RNA concentration was quantified using a NanoDrop ND-2000 (Thermo Fisher Scientific), and RNA quality and integrity were checked with Experion (Automated Electrophoresis Station, Bio-Rad) using the Experion Standard Sens RNA chip (Bio-Rad). Samples with an RNA integrity number (RIN) > 8.0 were chosen for further analysis. Transcriptional analysis was carried out using the AquaGenomic Sparus aurata oligonucleotide microarray (SAQ) platform. The complete information on this platform is available through the public repository Gene Expression Omnibus (GEO) (accession numbers GPL13442) at the United States National Center for Biotechnology Information (NCBI). For each experimental group, total RNA samples were pooled (n = 4 pools each group; n = 4 fish each pool, including one fish randomly taken from each experimental tank) at a final concentration (133 ng/μL each pool). This methodological approach of pooling RNA from four different specimens in each replicate allowed authors to evaluate the population’s variability. However, the information regarding individual variability was lost with this choice. One-color microarray was carried out according to the manufacturer’s protocols and as previously described by our group (Reyes-López et al., 2021). Microarray slides were scanned with Agilent Technologies Scanner model G2505B. Spot intensities and other quality control features were extracted with Agilent’s Feature Extraction software version 10.4.0.0 (Agilent Technologies). Quality reports were checked for each array. Although validation by mean of qPCR is required when there is a high risk of obtaining paralog genes and unspecific hybridization; in our study, we used an oligonucleotide-based microarray, which has probes with a reduced number of bases and high affinity, which avoids the necessity of conducting the validation of gene expression results by qPCR.
The extracted raw data were imported and analyzed with GeneSpring (version 14.5 GX software, Agilent Technologies). To standardize the arrays intensity, 75% percentile normalization was used, and data were filtered by expression levels. The differential expressed genes (DEGs) were obtained from a gene-level differential expression analysis. Expression values with a p-value < 0.05 were considered statistically significant. The DEGs were grouped according to their fold-change value (p-value < 0.05) and represented using the GraphPad software v7.0 for Windows. The Principal Component Analysis (PCA) was carried out using GeneSpring software (Agilent), four eigenvectors were calculated to describe the aggrupation of the control and SDPP groups in a 3D plot. The gene expression values (log2-expression ratios) were represented by a hierarchical clustering heatmap analysis using GeneSpring software.
Functional Network Analyses: Interactomes
The complete map of interactions that can occur in a living organism (interactome) was obtained from the DEGs obtained in the microarrays-based transcriptomics analysis. For this purpose, the Search Tool for the Retrieval of Interacting Genes (STRING) public repository version 10.01 was used. A Protein-Protein interaction (PPI) network for the differentially expressed genes was conducted with a high-confidence interaction score (value = 0.4). The mechanisms of response in which DEGs are involved were obtained from a comparative analysis based on Homo sapiens as a reference organism to extract the maximum information currently available. Thus, an ortholog H. sapiens Entrez Gene acronym was assigned based on sequence homology. Briefly, we selected the best tBlastX (NCBI) hit between the DEGs and DSPs query sequence for Sparus aurata and the human transcriptome database. We only consider those matches with at least E value ≤ 1e-10. The Uniprot and Genecards databases were used to confirm the gene acronym tag match between both species. Gene ontology (GO) pathway enrichment analysis for biological processes (GO_BiologicalProcess-EBI-UniProt-GOA-ACAP-ARAP_10.11.2020_00h00) was obtained using ClueGO v2.5.7 app through Cytoscape 3.8.2 platform. The Statistical analysis used was Enrichment/Depletion (two-sided hypergeometric test) with a P-value cut-off = 0.05 and corrected by Benjamin-Hochberg. A GO Fusion was performed to avoid redundant terms with a Kappa Score Threshold = 0.4 to propose more stringent GO terms associated with the mechanism of response for the experimental diet. In addition, grouping the GO terms was conducted when the sharing groups’ percentage was above 50, a P-value of < 0.05 was considered significant. The statistically significant GOs obtained from the enrichment analysis were assigned to each one of the nodes represented in the functional network. The nodes classified in different clusters according to their functionality were represented with ClueGo v2.5.7. Later, the MCC (Maximal Clique Centrality) of each node was calculated by CytoHubba (version 0.1) to find hub nodes (Chin et al., 2014). Those genes with the top 10 MCC values were considered hub genes.
Statistical Analysis
An unpaired t-test was conducted for microarrays data using the GeneSpring software GX 14.5 to detect DEGs (p < 0.05) between the control and SDPP groups. The DEGs were represented with GraphPad software version 7.0. The PCA analysis and the heatmap for DEGs were obtained from GeneSpring software version 14.5 (Agilent Technologies). The hierarchical map was clustered by the normalized intensity values (p < 0.05), with Euclidean distance and Ward linkage.
Results
Intestine Transcriptional Response From Fish Fed Spray-Dried Porcine Plasma -Supplemented Diet
The transcriptional analysis of the anterior intestine was carried out using the S. aurata oligonucleotide custom microarray, and the results are shown in Figure 1. A total of 803 DEGs were found in the anterior intestine of fish fed the SDPP diet. From the total number of DEGs, 441 DEGs were annotated, and 362 were unknown genes. Among them, 468 DEGs were up-regulated; in particular, 350 DEGs showed a modulation between 1.0- and 1.5-fold change (FC), 73 DEGs a modulation between 1.5 and 2.0 FC, 34 DEGs a modulation between 2.0 and 3.0, and 11 DEGs with a modulation higher than 3.0 FC. On the other hand, a total of 335 DEGs were down-regulated with 195 DEGs with modulation between −1.0 and −1.5 FC, showing 90 DEGs a modulation between −1.5 and −2.0 FC, 35 DEGs with modulation between −2.0 and −3.0, and 15 DEGs with a modulation lower than −3.0 FC (Figure 1A). The PCA analysis divided the dataset into three principal components and revealed a clear grouping between the microarrays for each of the tested diets (Figure 1B). The hierarchical clustering heatmap found a clear differential expression profile with a clear set of DEGs of fish fed with the control diet (Figure 1C; the top half of the panel) compared to those fed the SDPP diet (Figure 1C; bottom half).
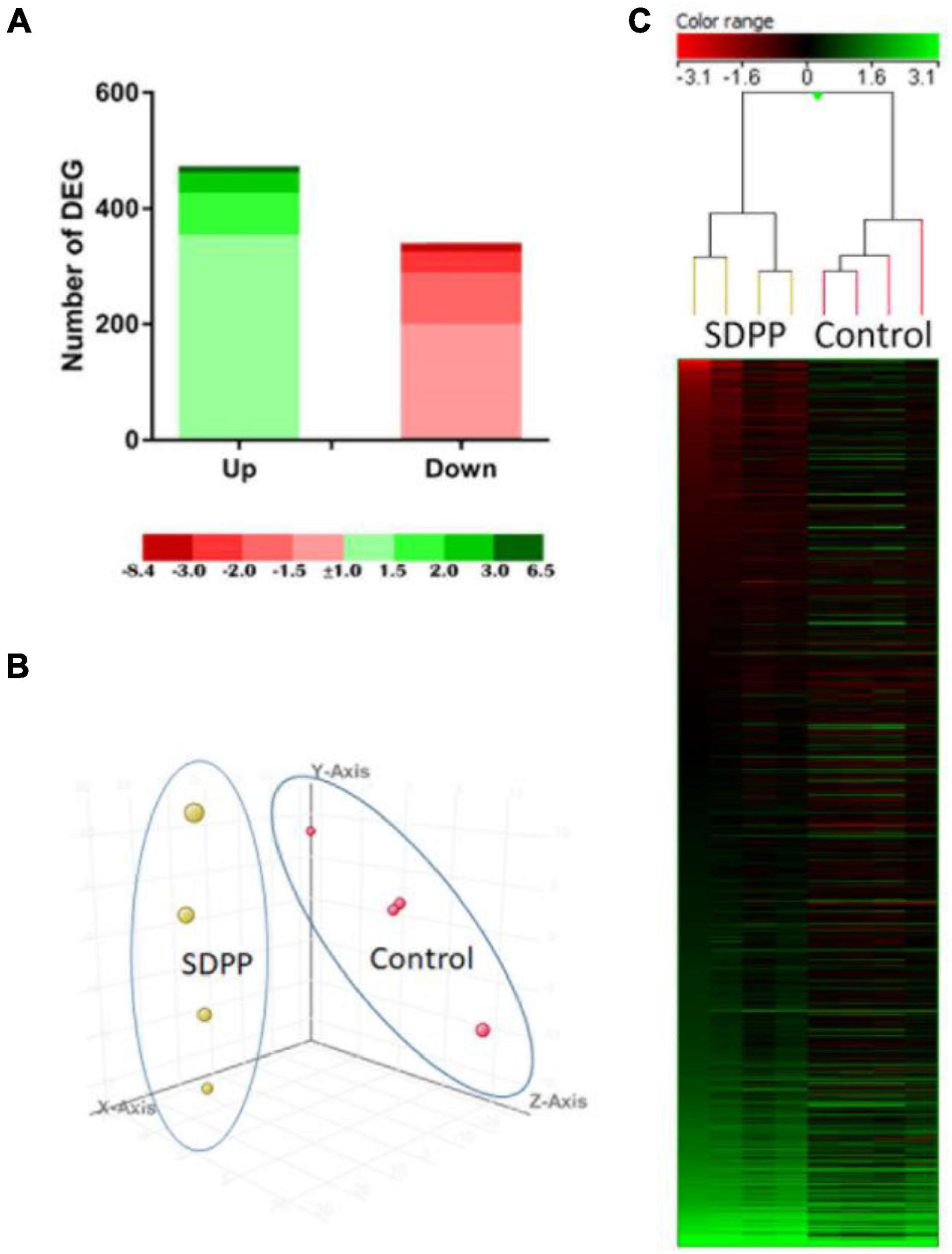
Figure 1. Anterior intestine microarrays-based transcriptomic analysis for gilthead sea bream fed the SDPP diet. (A) Number of total differential expressed genes (DEGs). The green (upregulation) and red (downregulation) color scheme indicate the gene modulation according to its magnitude interval (fold-change). (B) Principal component analysis (PCA). Control (red spheres) and SDPP groups (yellow spheres) are represented. Each array included in the study is represented by one sphere. (C) Hierarchical clustering heatmap representing the 803 DEGs. The results of each microarray analyzed for the control and SDPP group are shown. The green (up-regulation) and red (down-regulation) color scheme indicates the gene modulation according to its magnitude interval (fold-change).
In addition, a functional network analysis was carried out based on the DEGs obtained from the transcriptional analysis of gut samples from fish fed with the SDPP diet. From the 441 annotated DEGs, we got a functional association between 430 DEGs with 2,688 interactions (edges). In particular, 393 DEGs belonged to the central core interaction network (233 up-regulated DGEs; 160 down-regulated DEGs), while 37 DEGs (20 up-regulated DEGs; 17 down-regulated DEGs) did not have direct interaction with the central core (Figure 2).
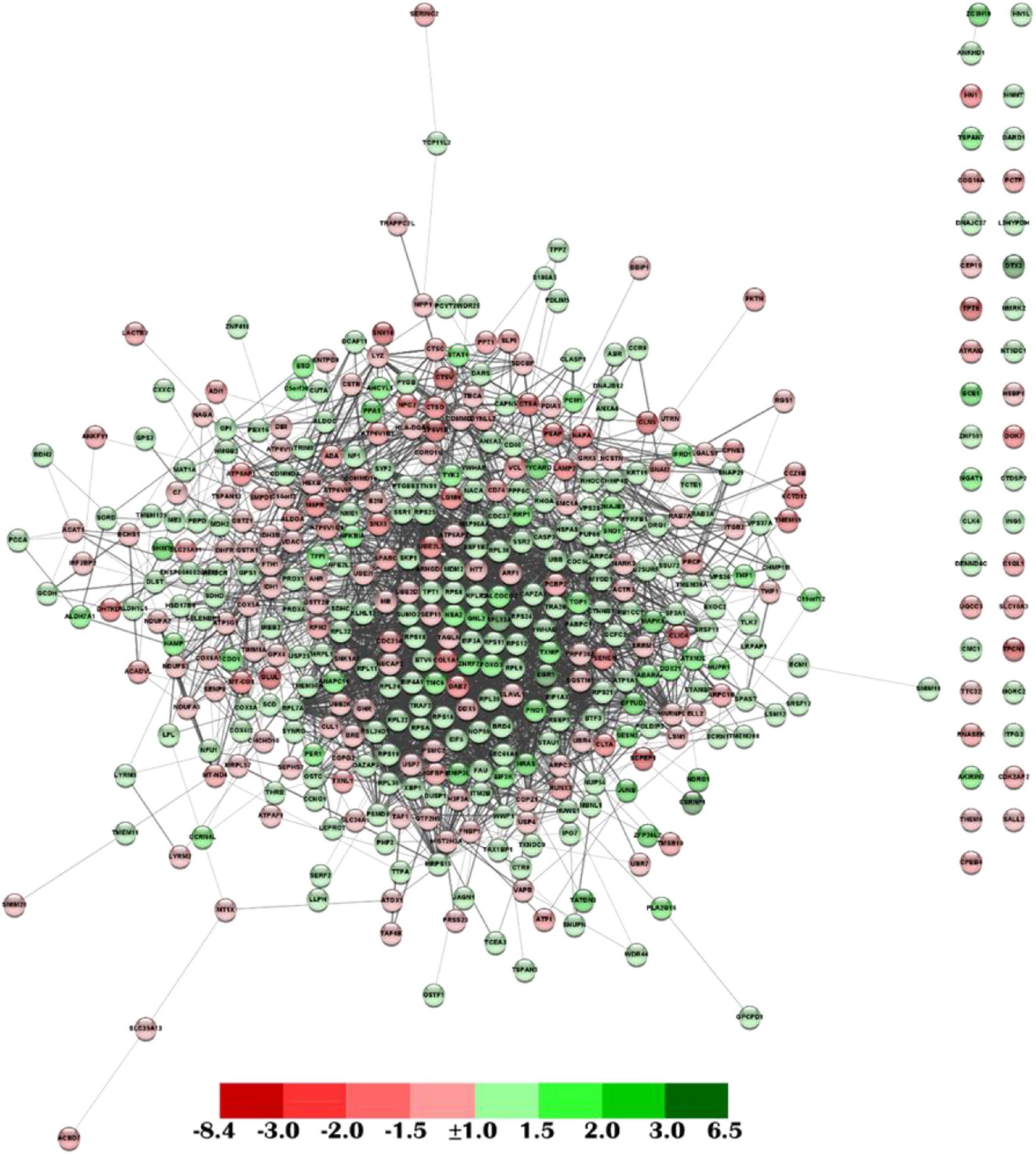
Figure 2. Functional network analysis from the anterior intestine of the gilthead sea bream fed the SDPP diet. Each node represents one differential expressed gene (DEG) obtained from the anterior intestine transcriptomic analysis. The DEG modulatory profile is represented with green (up-regulated) and red (down-regulated) into each node according to its fold-change magnitude interval. The green (up-regulation) and red (down-regulation) color scheme indicates the gene modulation according to its magnitude interval (fold-change).
To identify the function of these DEGs, a gene ontology (GO) pathway enrichment analysis was performed with the ClueGO platform. The results showed that the dietary inclusion of SDPP induced changes in 120 biological processes, grouped in 12 clusters. The sharing nodes between clusters are considered evidence of the interacting networks within biological processes. The most significant biological term names each cluster. For instance, the “Cellular catabolic process (GO:0044248)” is the most abundant category, with 69 terms corresponding to 42.3% of the total retrieved terms. Other biological processes clusters were the “Energy derivation by oxidation of organic compounds (GO:0015980)” with 16 terms corresponding to 9.8% of the total; the “Response to organic substance (GO:0010033)” with 15 terms related to 9.2%; the “Protein transport (GO:0015031)” with 12 terms corresponding to 74.4%; the “Leukocyte mediated immunity (GO:0002443)” with 11 terms related to a 6.8%; the “Organic substance catabolic process (GO:1901575)” and “Protein metabolic process (GO:0019538)” both with 10 terms corresponding to a 6.1%. Biological processes representing less than 5% of the total terms obtained were: the “Carboxylic acid metabolic process (GO:0019752)” with 6 GO terms corresponding to 3.7%; the “Generation of precursor metabolites and energy (GO:0006091)” and the “Protein catabolic process (GO:0030163)” both with five terms corresponding to 3.1%; the “Protein containing complex subunit organization (GO:0043933)” with three terms related to 1.8%; and the “G protein-coupled receptor signaling pathway (GO:0007186)” with one-term corresponding to a 0.6% of the total of enriched biological process (Figures 3, 4).
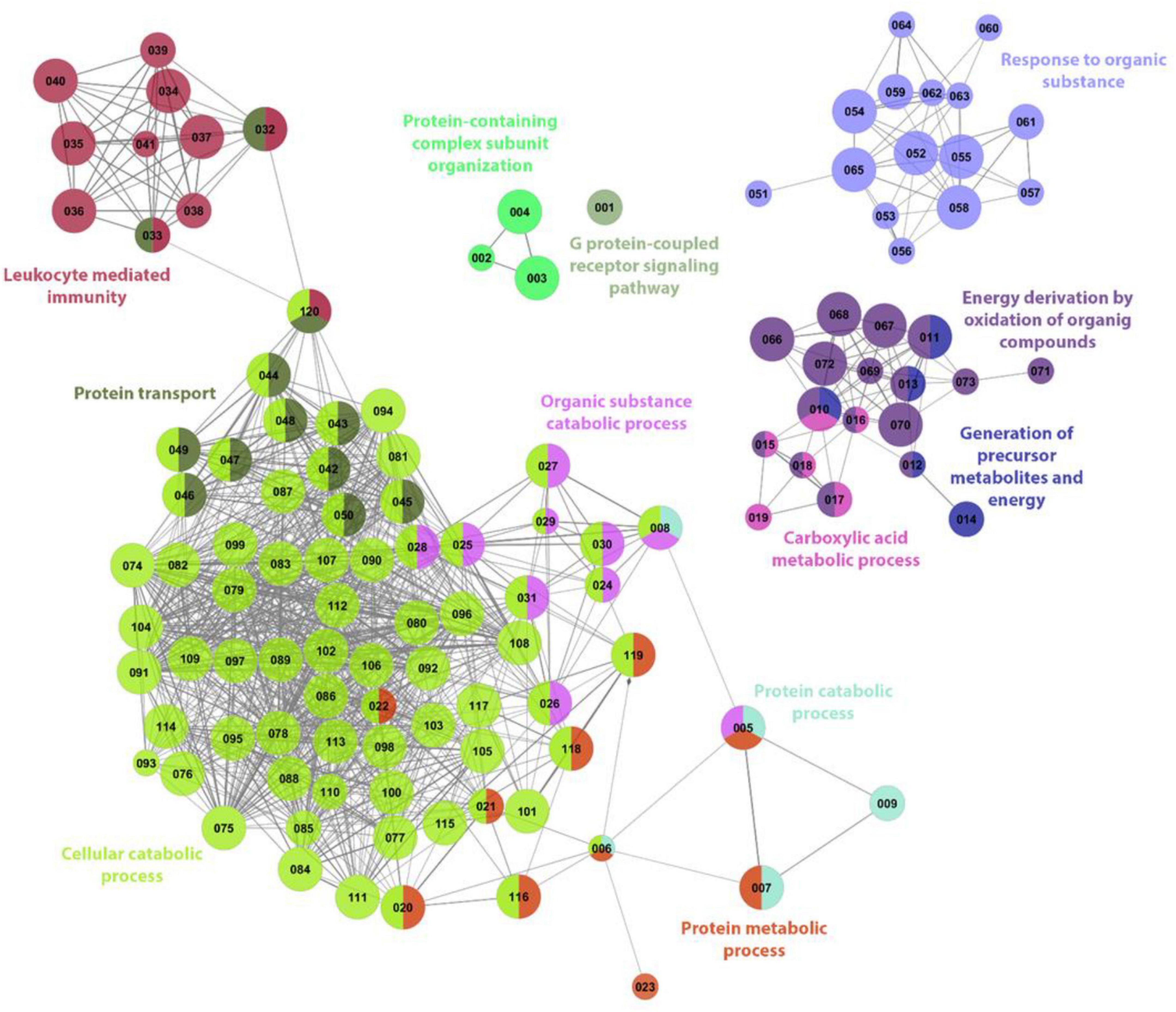
Figure 3. ClueGo analysis of significantly enriched terms based on the biological functions in the anterior intestine of gilthead sea bream fed the SDPP diet. Each node represents one GO enrichment pathway (please refers to Supplementary Table 1 for details). The size of the node means its significance. Each color indicates a cluster of closely related biological processes. The colored biological term denominates the leading group term. The lines into the cluster showed a closed relationship between biological processes.
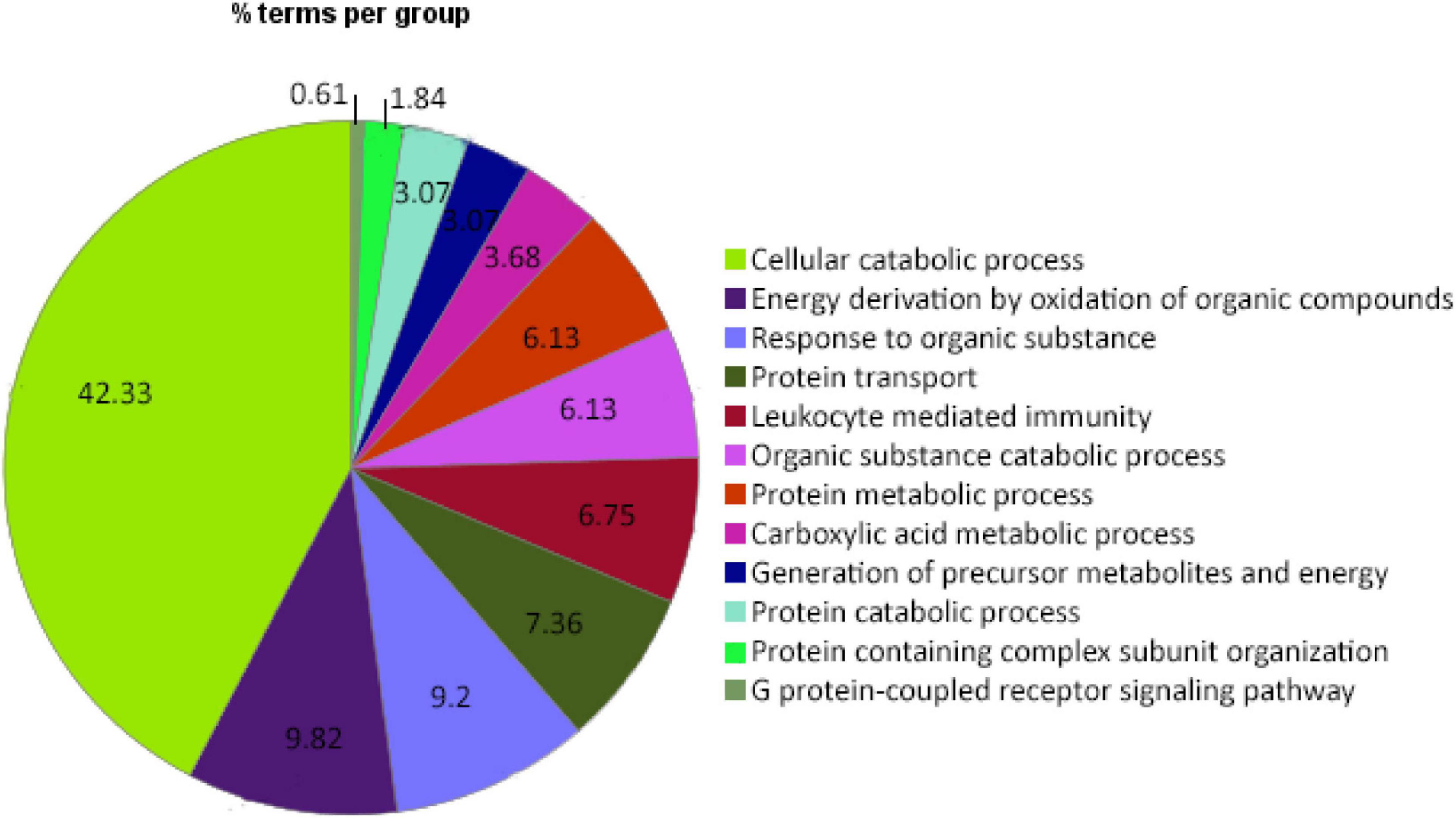
Figure 4. Pie chart distribution of significantly enriched terms based on the biological functions in the anterior intestine of gilthead sea bream fed the SDPP diet. It is represented the percentage of the significantly differentially expressed genes in each principal cluster.
According to the data obtained from biological processes GO terms, the functional network showed a strong association between leukocyte-mediated immunity, protein transport, cellular catabolic process, protein metabolic process and organic substance catabolic process. Another association was also registered between carboxylic acid metabolic processes, energy derivation by oxidation of organic compounds, and generation of precursor metabolites and energy (Figure 3).
From the enrichment analysis performed by the ClueGO platform, those DEGs belonging to the most representative GO terms from the biological process were represented in an individual functional network (Figure 5). The diet containing SDPP resulted in the positive regulation for the “G protein-coupled receptor signaling pathway” (7 up-regulated DEGs; 4 down-regulated DEGs) (Figure 5L), the “Protein containing complex subunit organization” (45 up-regulated DEGs; 37 down-regulated DEGs) (Figure 5K), the “Protein metabolic process” (121 up-regulated DEGs; 65 down-regulated DEGs) (Figure 5G), the “Organic substance catabolic process” (80 up-regulated DEGs; 45 down-regulated DEGs) (Figure 5F), the “Protein transport” (66 up-regulated DEGs; 34 down-regulated DEGs) (Figure 5D), the “Response to organic substance” (80 up-regulated DEGs; 61 down-regulated DEGs) (Figure 5C), and the “Cellular catabolic process” (80 up-regulated DEGs; 43 down-regulated DEGs) (Figure 5A). In addition, the diet containing SDPP resulted in a balanced regulation (i.e., similar number of up- and down-regulated DEGs) for the “Energy derivation by oxidation of organic compounds” (14 up-regulated DEGs; 14 down-regulated DEGs) (Figure 5B), the “Leukocyte mediated immunity” (23 up-regulated DEGs; 27 down-regulated DEGs) (Figure 5E), the “Carboxylic acid metabolic process” (29 up-regulated DEGs; 23 down-regulated DEGs) (Figure 5H), the “Generation of precursor metabolites and energy” (20 up-regulated DEGs; 18 down-regulated DEGs) (Figure 5I), and the “Protein catabolic process” (22 up-regulated DEGs; 27 down-regulated DEGs) (Figure 5J). Notably, in almost all the functional networks identified for the DEGs belonging to the selected GO enrichment from the biological process, the up-regulated genes show a close interaction (Figures 5A,D,F,G,J,K).
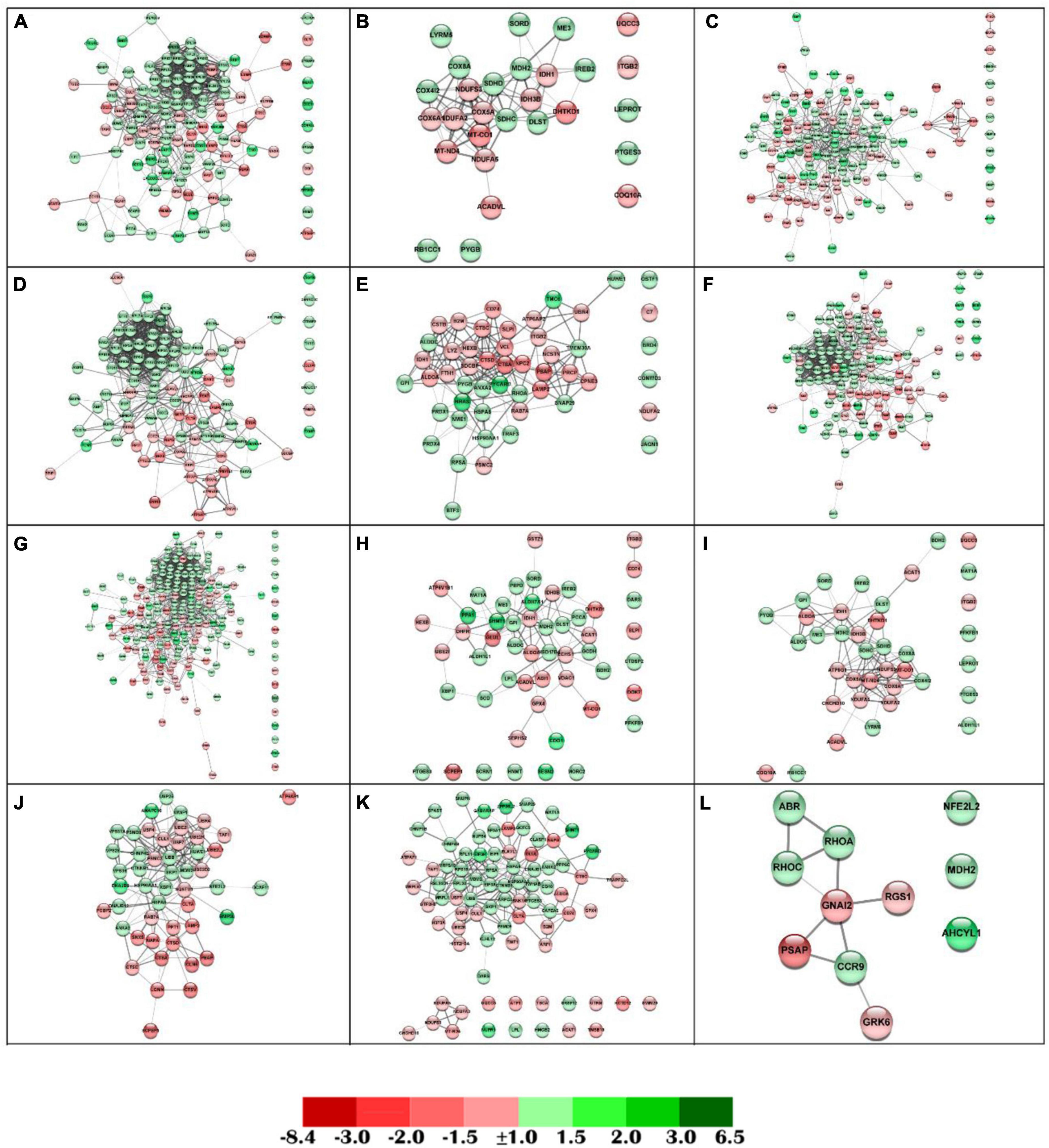
Figure 5. Detailed functional networks for those DEGs belonging to the most representative GO terms for each enrichment cluster for biological functions. Each node represents one differential expressed gene (DEG) obtained from the anterior intestine transcriptomic analysis. (A) Cellular catabolic process. (B) Energy derivation by oxidation of organic compounds. (C) Response to organic substance. (D) Protein transport. (E) Leukocyte mediated immunity. (F) Organic substance catabolic process. (G) Protein metabolic process. (H) Carboxylic acid metabolic process. (I) Generation of precursor metabolites and energy. (J) Protein catabolic process. (K) Protein containing complex subunit organization. (L) G protein-coupled receptor signaling pathway. The DEG modulatory profile is represented with green (up-regulated) and red (down-regulated) into each node according to its fold-change magnitude interval scheme (detailed at the bottom of the figure).
The top 10 hub genes with a high level of correlation for the 12 clusters obtained from the enriched biological functions were selected with CytoHubba software. Thus, from the “Cellular catabolic process”, 10 transcripts have a higher level of connection in the network (rps8, rps24, rps18, rps16, rps11,rps12, rpl7a, rpl11, rpl13A, rpl29). Importantly, all these transcripts were up-regulated (Figure 6A). For the cluster “Energy derivation by oxidation of organic compounds”, among the top 10 hub genes, seven transcripts were down-regulated (ndufs3, cox5a, cox6a1, ndufa5, ndufa2, mt-nd4, mt-co1), and three of them up-regulated (sdhc, sdhd, cox4i2) (Figure 6B). For the cluster “Response to organic substance”, most of the hub genes were up-regulated (rpsa, ubb, rps16, skp1, ctnnb1, hspa8, hsp90aa1, eftud2) compared to the two down-regulated hub genes (psmc2, cul1) (Figure 6C). Considering the cluster “Protein transport”, all ten hub genes were up-regulated (rps8, rps24, rps18, rps16, rps11, rplp2, rpl9, rpl7a, rpl13, rpl11) (Figure 6D). For the “Leukocyte mediated immunity” cluster, we identified three up-regulated transcripts (pycard, anxa2, pygb) and seven down-regulated hub genes (sdcbp, lyz, hexb, ctsc, ctsd, npc2, ctsa) (Figure 6E). Regarding the clusters “Organic substance catabolic process” and “Protein metabolic process”, the same hub genes were identified (rps8, rps24, rps18, rps16, rps12, rps11, rpl9, rpl7a, rpl13a, rpl11). Remarkably, all of them were up-regulated genes (Figures 6F,G). For the cluster “Carboxylic acid metabolic process”, seven hub genes were up-regulated (shmt1, sord, me3, aldoc, gpi, mdh2, pepd), and three down-regulated (idh1, aldoa, glul) (Figure 6H). Considering the cluster “Generation of precursor metabolites and energy”, just two genes were up-regulated (sdhd and sdhc), whereas eight genes showed a down-regulation (ndufs3, atp5g1, cox6a1, ndufa2, cox5a, ndufa5, mt-nd4, mt-co1) (Figure 6I). The “Protein catabolic process” cluster showed nine hub genes. From them, five genes were upregulated (wwp1, mdm2, hwew1, skp1, ubb), and four of them were downregulated (ube2d2, cul1, ubr4, and ube2l3) (Figure 6J). For the cluster “Protein containing complex subunit organization”, all hub genes were upregulated (eif3k, rsl24d1, eif3a, mrpl1, mrps15, eif5, rps19, rpl11, rpl24, rpsa) (Figure 6K). For the cluster “G protein-coupled receptor signaling pathway”, six hub genes were up-regulated (ahcyl1, abr, rhoa, ccr9, mdh2, rhoc), and four down-regulated (grk6, rgs1, gnai2, psap) (Figure 6L).
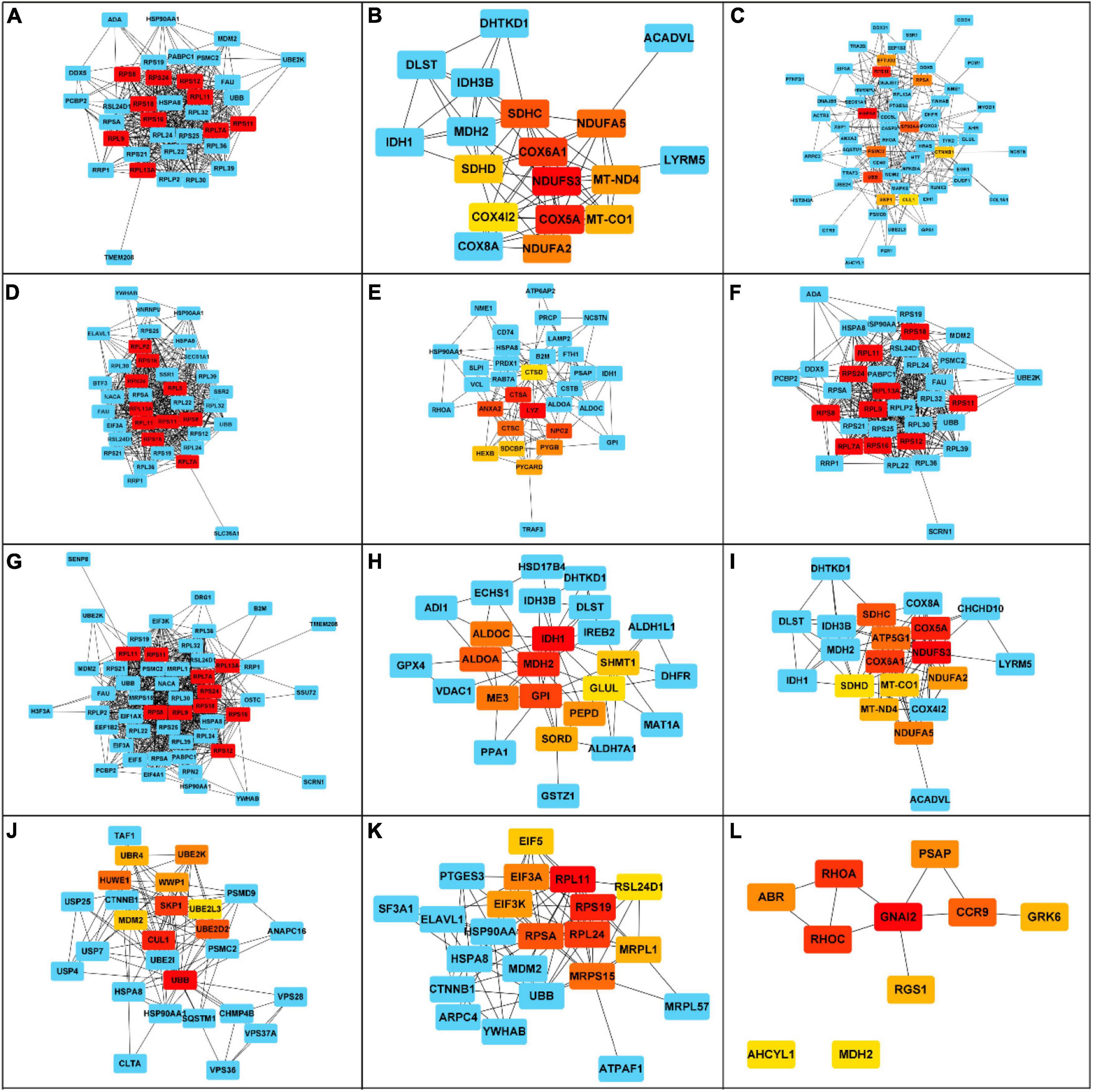
Figure 6. Hub genes in each cluster term identified from the enriched biological functions in the anterior intestine of gilthead sea bream fed the SDPP diet. The principal cluster shows the 10-hub genes and the closest neighbor’s genes for each network. The color intensity from yellow to red (i.e., yellow, orange, red) represents the degree of the hub gene (i.e., the relevance degree) in each network, being red those most relevant hub genes. The blue nodes represent the closest neighbors. (A) Cellular catabolic process. (B) Energy derivation by oxidation of organic compounds. (C) Response to organic substance. (D) Protein transport. (E) Leukocyte mediated immunity. (F) Organic substance catabolic process. (G) Protein metabolic process. (H) Carboxylic acid metabolic process. (I) Generation of precursor metabolites and energy. (J) Protein catabolic process. (K) Protein containing complex subunit organization. (L) G protein-coupled receptor signaling pathway.
Collectively, the transcriptomic data showed a strong association between the genes associated with the catabolic process, protein transport, and leukocyte mediated immunity, suggesting that SDPP favors digestive- and defensive-related functions in the gilthead sea bream intestine.
Discussion
In the present study, we evaluated the potential benefits of the dietary administration of SDPP in the gut of a marine teleost species by a detailed transcripteractome built from the enriched biological processes obtained to elucidate how this functional ingredient acted upon the maintenance of the gut mucosal tissue. Transcripteractome-based functional network analysis on teleost fish has gained attention in the last years (Firmino et al., 2020, 2021a,2021c; Reyes-López et al., 2021; Salomón et al., 2021). Previously, a similar strategy was followed by Reyes-López et al. (2021) to analyze the effects of SDPP at skin level. In our study, the transcriptomic analysis revealed that DEGs obtained corresponded mainly to biological processes related to “Cellular catabolic process (GO:0044248)”, “Energy derivation by oxidation of organic compounds (GO:0015980)”, and “Response to organic substance (GO:0010033)”. These processes are probably correlated with the previous reported improved growth performance, feed intake, and feed efficiency promoted by dietary inclusion of SDPP in this species (Gisbert et al., 2015; Reyes-López et al., 2021), rainbow trout (Campbell et al., 2003), Nile tilapia (de Araújo et al., 2017), and higher vertebrates including pigs (Angulo and Cubiló, 1998; Van Dijk et al., 2002; Kósa et al., 2019), poultry (Beski et al., 2016; Dabbou et al., 2020), and cats (Rodríguez et al., 2016). In 2001, van Dijk et al. (2001) reported that dietary SDPP levels up to 6% increased both average daily gain and feed intake in the first 2 weeks in a dose-dependent fashion and improved feed conversion ratio (FCR) in piglets. Similar results were reported by Gisbert et al. (2015) in gilthead sea bream fed diets containing 3% SDPP in diets for gilthead sea bream fingerlings in terms of these two common key performance indicators related to somatic growth and feed efficiency. Remarkably, the ten transcripts that showed the higher level of connection in the “Cellular catabolic process” network were up-regulated, including mostly ribosomal proteins, such as rps8, rps24, rps18, rps16, rps11,rps12, rpl7a, rpl11, rpl13A, and rpl29. Ribosome biogenesis is a highly regulated process associated directly with cell growth and the most active proteins in the cell. In the case of rapidly growing yeast cells, the rRNA constitutes approximately 80% of total cellular nucleic acid, being 50% of all RNA polymerase II transcription initiation events that occur in ribosomal protein genes (Warner, 1999; Lempiäinen and Shore, 2009). Previously, in a similar experimental dietary approach, Reyes-López et al. (2021) evaluated the skin multi-omics interactome merging the transcripteractomic response with a proteomic profile at mucosal skin level in this species, showing a significant linkage for “cellular catabolic process”, “biogenesis”, and “protein transport” processes. Additionally, the GO analysis revealed that several biological processes were up-regulated, including “ribonucleoprotein complex biogenesis”; and “ribosome biogenesis.” The authors suggested that the results may be attributed to the higher digestibility of protein in SDPP, which may promote protein turnover and exudation machinery in mucosal tissues (Reyes-López et al., 2021). Protein catabolism is the breakdown of proteins into absorbable monomers, which may be the only source of essential amino acids (Gurina and Mohiuddin, 2021), reflecting the relationship between the cellular catabolic process and the biogenesis process. However, among the 10 up-regulated ribosomal proteins found in the gut under current experimental conditions, rps8, rps18, and rps16 were differentially down-regulated in the skin mucosa (Reyes-López et al., 2021). Differences in the gene expression profile between mucosal tissues to the same stimulus have been described elsewhere (Khansari et al., 2018). The scope of these differences remains to be elucidated in further studies, although it may be related to differences in tissue function, physiology, and cellular turnover.
Blood plasma is a complex fluid that contains a wide variety of proteins, cytokines, growth factors, hormones, bioactive peptides, and amino acids that are involved in the health benefits found at systemic and different mucosal levels when SDPP is included in animal diets (Pérez-Bosque et al., 2016). The exact mechanism of action of SDPP is not fully understood. Some authors suggest that immunoglobulins are the main proteins involved in the growth rate and feed intake enhancement, together with an improved immune and intestinal function (Pierce et al., 2005; Petschow et al., 2014). However, its mechanism of action is not fully understood (Petschow et al., 2014). In addition, blood plasma contains more than 695 proteins in its composition (Schenk et al., 2008), and probably some of these proteins are also involved in the mechanism of action of SDPP. Although the number of peptides in blood plasma is relatively scarce about the number of proteins with MW > 10 kDa, some authors suggest that bioactive peptides present in plasma proteins may have beneficial effect after enzymatic hydrolysis at the intestinal level and may partially explain the improved performance and health benefits associated with the inclusion of SDPP in animal diets (Bah et al., 2013).
In the present study, we identified the modulation of DEGs associated with the energy derivation by oxidation of organic compounds. Most DEGs were down-regulated (ndufs3, cox5a, cox6a1, ndufa5, ndufa2, mt-nd4, and mt-co1) in comparison to the up-regulated ones (sdhc, sdhd, and cox4i2). Importantly, all these transcripts are related to mitochondrial processes associated with obtaining energy. In this way, NDUFS3 is one of the central subunits in the mitochondrial complex I. NDUFS3 is a catalytic subunit that has been shown to play a vital role in the proper assembly of functional complex I in the human mitochondrial respiratory chain (Suhane et al., 2011). On the other hand, NDUFA5 and NDUFA2 are accessory subunits of the mitochondrial complex I (Brandt, 2006). Similarly, MT-ND4 is also a central subunit of the mitochondrial complex 1 encoded in the mitochondrial genome (Brandt, 2006). In addition, COX5A (Cytochrome c oxidase 5A) and COX6A1, and COX4I2 are subunits of the cytochrome c oxidase (complex IV), which is the terminal complex of eukaryotic oxidative phosphorylation in mitochondria (Sommer et al., 2017; Watson and McStay, 2020). MT-CO1 is also part of the complex IV but is encoded in the mitochondrial genome (Carr and Winge, 2003). Finally, SDHC and SDHD are subunits of the Succinate dehydrogenase (SDH or Complex II), which is a heterotetrameric complex that links the tricarboxylic acid cycle (TCA) with the electron transport chain (Van Vranken et al., 2015). Taking together, the up- and down-regulatory expression profile of these genes in response to the SDPP administration showed that this ingredient resulted in the controlled activation of this very dynamic and strictly regulated cooperation interaction between nuclear and mitochondrial DNA for orchestrating a coordinated biogenesis of the mitochondrial complexes; thus, preserving the mitochondrial activity and homeostasis (Piomboni et al., 2012; Eisenberg-Bord and Schuldiner, 2017; Gómez-Serrano et al., 2018).
In the present study, the protein transport process was also enriched. The up-regulation of all protein transport hub genes identified (rps8, rps24, rps18, rps16, rps11, and rpl7a) coincided with the higher level of connection in the functional network between cellular catabolic processes and protein transport. In a previous study focused on the skin mucosa, a strong relationship between protein transport and the cellular catabolic process was also described in gilthead sea bream fed the SDPP (Reyes-López et al., 2021), results that may be attributed to the nutritional profile of this functional ingredient (Almeida et al., 2013). Such association was shown to be related to enhanced integrity of the mucosal tissue by the enhancement of tight junctions at the cell-cell level and/or between the cell and the extracellular matrix (Reyes-López et al., 2021), thus reinforcing the physical barrier favoring the protection of the organism in front of an environment fluctuation (Esteban, 2012; Parra et al., 2015). According to this idea, in our study we also found the up-regulation of syntenin. This protein has been associated with the cytoskeletal-membrane organization, cell adhesion, protein trafficking, and the activation of transcription factors. Importantly, this protein is primarily localized to membrane-associated adherens junctions and focal adhesion, reinforcing the idea of higher mucosal robustness by administrating SDPP. In the same line, in our study the up-regulation of rhoa was also registered, and whose function is related to the organization of the cytoskeleton, cell cycle progression, and cell adhesion by the maintenance of adherents and tight junctions (Terry et al., 2010; Citalán-Madrid et al., 2013). Taking together, these antecedents suggest that SDPP improves the intestinal barrier function, which is supported by previous results of SDPP tested in terrestrial animals (Peace et al., 2011; Pérez-Bosque et al., 2016).
In the context of a higher mucosal robustness, there is evidence that the SDPP oral administration also improved the activity of the intestinal antioxidant defense system and increased the density of goblet cells in intestinal mucosa; thus, favoring the intestinal health and innate immune function (Gisbert et al., 2015). The intestine plays a vital role in fish health as a physiological and immunological barrier to a wide range of microorganisms and foreign substances (Lalles, 2010; Gisbert et al., 2015). Similar results have been reported in pigs (Peace et al., 2011; Tran et al., 2014) and poultry (Beski et al., 2016), being the intestinal mucosa one of the best-studied tissues under the administration of SDPP. In fact, SDPP has been recommended for livestock nutrition as a source of immunological support due to its content in immunoglobulins and bioactive peptides (Bureau et al., 1999; Pérez-Bosque et al., 2010, 2016; Peace et al., 2011; Chin et al., 2014). That composition is related to enterocyte nutrition, mucin production, energetic resources, nucleotides synthesis, mechanical barrier stimulation, and innate immune response stimulation through the increase in leukocytes and immunoglobulins (Pérez-Bosque et al., 2006; Gao et al., 2011; Gisbert et al., 2015; de Araújo et al., 2017). In this way, in this study we also observed a strong relationship between the genes associated with immunity and protein transport, as it was previously reported for the skin mucosa (Reyes-López et al., 2021). At the innate immune level, lysozyme expression was down-regulated in our study. By contrast, it was previously reported that oral administration of SDPP enhanced the serum immune function in fish, increasing the serum lysozyme levels (Gisbert et al., 2015). This apparent discrepancy between the response observed for serum and intestine could be strictly associated with a specific response pattern for each tissue, even coming from the same organism. In fact, there is evidence in gilthead sea bream that the same stimulus may provoke different response patterns between tissues (Khansari et al., 2019), even at the mucosal level (Khansari et al., 2018). Lysozyme is a crucial antibacterial defense molecule of the innate immune system produced by leukocytes. Certain nutrients and compounds present in the diet might modulate the lysozyme activity in fish, which is generally associated with an increase in the protection against various bacterial infections (Saurabh and Sahoo, 2008; Gisbert et al., 2015). This aspect reaches particular relevance considering the high protein content in our diet. In mammals, a diet rich in animal protein promotes a gut pro-inflammatory response (Jantchou et al., 2010; Kostovcikova et al., 2019). In fish, there is evidence of gut inflammation by the use of soybean protein diet, inducing enteropathy and secretions that lead to greater immune sensitivity with migration of eosinophilic granulocytes, high levels of lysozyme, and IgM (Krogdahl et al., 2000; Uran et al., 2009; Hedrera et al., 2013; Estruch et al., 2015). Importantly, a previous report revealed an average intestinal mucosa organization in gilthead sea bream fed with an SDPP supplemented diet (Tapia-Paniagua et al., 2020). Similarly, in our study, ctsa, ctsc, and ctsd were also down-regulated. Their protein products are cathepsins, lysosomal proteases active in a slightly acidic environment involved at different levels in the process of the innate and adaptive immune responses (Turk et al., 2012; Perisic Nanut et al., 2014) that appears to be a central coordinator for activation of many serine proteinases in cells of the immune system. Thus, the down-regulation of genes associated with pro-inflammatory promotion reinforces the hypothesis of tight regulation of the inflammatory response in gilthead sea bream gut fed with the SDPP diet.
According to the tight regulation of the immune response, we observed in our transcripteractome analysis just 6.8% of the up-regulated transcripts associated with the biological process “Leukocyte mediated immunity (GO:0002443).” Among them, it stands out the gene pycard and whose protein product, called ASC [apoptosis-associated speck-like protein containing a caspase-recruitment domain (CARD)], is an adaptor protein involved in the formation of inflammasomes (Li et al., 2018). The inflammasome activation is a tightly regulated process, including transcriptional and post-transcriptional regulation mechanisms of their components, including upstream regulators and downstream effectors. Remarkably, the expression of ASC has been suggested to be constitutive in many cells and does not appear to be inducible by inflammatory signaling (Christgen et al., 2020). However, cells stimulated with LPS in the human intestinal mucosa, ASC can be up-regulated but posteriorly repressed (Masumoto et al., 2006). Similarly, in peripheral blood human neutrophils, ASC expression was transiently up-regulated by the pro-inflammatory cytokines IL-1α, IL-1β, IFNα, IFNγ, TNFα, and the stimulus by LPS (Shiohara et al., 2002). Recently, ASC was described in the inflammasome activation pathway on zebrafish with conserved and unique structural/functional features (Li et al., 2018). However, more studies are required to characterize the expression of ASC in gilthead sea bream and the scope of its up-regulation in response to the dietary administration of SDPP.
In the same context of the tight control of the immune system for a successful response, we also found the up-regulation of sdcbp. In mammals, its protein product (Syntenin-1) is an important positive regulator of transforming growth factor-beta (TGF-β) signaling (Hwangbo et al., 2016). At the same time, it has a role as a negative regulator of intestinal immunoglobulin production to maintain intestinal homeostasis in vivo (Tamura et al., 2015). In teleost, information about the role of Syntenin in the immune response is scarce. Currently, in zebrafish it is associated with epiboly and gastrulation movements (Lambaerts et al., 2012), the exosome biogenesis and release from yolk syncytial layer (Verweij et al., 2019), and in adults to the regeneration after traumatic insult to the central nervous system (Yu and Schachner, 2013). In kuruma shrimp (Marsupenaeus japonicus), there is some evidence of syntenin-like genes in antibacterial response (Liu et al., 2015), increasing a conserved evolutionary role in immunity.
Conclusion
Taking together, in this study we observed a strong association between immunity, protein transport, and process related to metabolism-associated processes. This evidence confirms the previous evidence of the immune, metabolic, and protein transport processes that take place at the mucosal tissue level in response to SDPP (Reyes-López et al., 2021). Thus, the integrative analysis of gilthead sea bream fed with SDPP supported the better key performance indicators related to growth and feed performances of gilthead sea bream, which are also associated with a balanced immune response and an enhancement of the mucosal health status.
Data Availability Statement
The datasets presented in this study can be found in online repositories. The names of the repository/repositories and accession number(s) can be found below: Gene Expression Omnibus (GEO) (accession number GSE193972) at the National Center for Biotechnology Information (NCBI).
Ethics Statement
Animal experimental procedures were conducted in compliance with the research protocol approved by the IRTA’s Committee of Ethics and following the Guidelines of the European Union Council (86/609/EU) for the use of laboratory animals.
Author Contributions
JP and EG designed the study. EG carried out the nutritional trial. LT, FR-L, and EG supervised the study. EV-V, SR-C, and FR-L made microarrays hybridizations, raw data output and processing and transcriptomic data analysis and co-wrote the original draft, were in charge of the conceptualization, methodology, and interpretation of the integrative analysis, including interactomes, GO terms enrichment, and cluster analysis, and made the conceptualization and design of figures. All authors provided critical feedback and approved the final manuscript.
Funding
This work has been financially supported by the project “AQUOLIVE by NATAC,” funded by European Union’s Horizon 2020 Research and Innovation Program (Grant Agreement No. 830202) and partially by the Agencia Española de Investigación (grant AGL2016-76069-C2-2-R to UAB).
Conflict of Interest
JP was employed by APC Europe SL.
The remaining authors declare that the research was conducted in the absence of any commercial or financial relationships that could be construed as a potential conflict of interest.
Publisher’s Note
All claims expressed in this article are solely those of the authors and do not necessarily represent those of their affiliated organizations, or those of the publisher, the editors and the reviewers. Any product that may be evaluated in this article, or claim that may be made by its manufacturer, is not guaranteed or endorsed by the publisher.
Acknowledgments
We thank (1) DIETAplus project of the JACUMAR (Junta de Cultivos Marinos, MAPAMA; Spanish government), which is co-funded with FEMP funds (EU); (2) ERC (European Research Council) in MedAID project (Mediterranean Aquaculture Integrated Development; Grant Agreement no. 727315); (3) Fondecyt regular [project number 1211841; Agencia. Nacional de Investigación y Desarrollo de Chile (ANID); Government of Chile]; and (4) Fondecyt iniciación grant (project number 11221308; ANID, Government of Chile). The funder bodies were not involved in the study design, collection, analysis, interpretation of data, the writing of this article, or the decision to submit it for publication.
Supplementary Material
The Supplementary Material for this article can be found online at: https://www.frontiersin.org/articles/10.3389/fmars.2022.814233/full#supplementary-material
Footnote
References
Almeida, F. N., Htoo, J. K., Thomson, J., and Stein, H. H. (2013). Comparative amino acid digestibility in US blood products fed to weanling pigs. Anim. Feed Sci. Technol. 181, 80–86. doi: 10.1016/j.anifeedsci.2013.03.002
Angulo, E., and Cubiló, D. (1998). Effect of different dietary concentrations of spray-dried porcine plasma and a modified soyprotein product on the growth performance of piglets weaned at 6 kg body weight. Anim. Feed Sci. Technol. 72, 71–79.
Bah, C. S. F., Bekhit, A. E. D. A., Carne, A., and Mcconnell, M. A. (2013). Slaughterhouse blood: an emerging source of bioactive compounds. Compr. Rev. Food Sci. Food Saf. 12, 314–331. doi: 10.1111/1541-4337.12013
Beski, S. S., Swick, R. A., and Iji, P. A. (2016). Effect of dietary inclusion of spray-dried porcine plasma on performance, some physiological and immunological response of broiler chickens challenged with Salmonella sofia. J. Anim. Physiol. Anim. Nutr. 100, 957–966. doi: 10.1111/jpn.12414
Brandt, U. (2006). Energy converting NADH:quinone oxidoreductase (complex I). Annu. Rev. Biochem. 75, 69–92. doi: 10.1146/annurev.biochem.75.103004.142539
Bureau, D., Harris, A., and Cho, C. (1999). Apparent digestibility of rendered animal protein ingredients for rainbow trout (Oncorhynchus mykiss). Aquaculture 180, 345–358. doi: 10.1016/s0044-8486(99)00210-0
Campbell, J. M., Quigley, J. D., Russell, L. E., and Kidd, M. T. (2003). Effect of spray-dried bovine serum on intake, health, and growth of broilers housed in different environments. J. Anim. Sci. 81, 2776–2782. doi: 10.2527/2003.81112776x
Carr, H. S., and Winge, D. R. (2003). Assembly of cytochrome c oxidase within the mitochondrion. Acc. Chem. Res. 36, 309–316. doi: 10.1021/ar0200807
Chin, C. H., Chen, S. H., Wu, H. H., Ho, C. W., Ko, M. T., and Lin, C. Y. (2014). cytoHubba: identifying hub objects and sub-networks from complex interactome. BMC Syst. Biol. 8:S11. doi: 10.1186/1752-0509-8-S4-S11
Christgen, S., Place, D. E., and Kanneganti, T. D. (2020). Toward targeting inflammasomes: insights into their regulation and activation. Cell Res. 30, 315–327. doi: 10.1038/s41422-020-0295-298
Citalán-Madrid, A. F., García-Ponce, A., Vargas-Robles, H., Betanzos, A., and Schnoor, M. (2013). Small GTPases of the Ras superfamily regulate intestinal epithelial homeostasis and barrier function via common and unique mechanisms. Tissue Barriers 1:e26938. doi: 10.4161/tisb.26938
Dabbou, S., Trocino, A., Xiccato, G., Nery, J., Madrid, J., Martinez, S., et al. (2020). The effect of dietary supplementation with globin and spray-dried porcine plasma on performance, digestibility and histomorphological traits in broiler chickens. J. Anim. Physiol. Anim. Nutr. 105, (Suppl. 2), 42–51. doi: 10.1111/jpn.13356
Dawood, M. A. O. (2021). Nutritional immunity of fish intestines: important insights for sustainable aquaculture. Rev. Aquac. 13, 642–663. doi: 10.1111/raq.12492
de Araújo, E. P., de Carvalho, P. L. P. F., de Freitas, J. M. A., da Silva, R. L., Rocha, M. K. H. R., Teixeira, C. P., et al. (2017). Dietary spray-dried plasma enhances the growth performance, villus:crypt ratio and cold-induced stress resistance in Nile tilapia (Oreochromis niloticus). Aquaculture 479, 675–681. doi: 10.1016/j.aquaculture.2017.07.003
Eisenberg-Bord, M., and Schuldiner, M. (2017). Ground control to major TOM: mitochondria–nucleus communication. FEBS J. 284, 196–210. doi: 10.1111/febs.13778
Ellis, A. E. (2001). Innate host defense mechanisms of fish against viruses and bacteria. Dev. Comp. Immunol. 25, 827–839. doi: 10.1016/s0145-305x(01)00038-6
Esteban, M. Á (2012). An overview of the immunological defenses in fish skin. Int. Sch. Res. Netw. Immunol. 2012:853470. doi: 10.5402/2012/853470
Estruch, G., Collado, M. C., Penaranda, D. S., Tomas Vidal, A., Jover Cerda, M., Perez Martinez, G., et al. (2015). Impact of fishmeal replacement in diets for gilthead sea bream (Sparus aurata) on the gastrointestinal microbiota determined by pyrosequencing the 16S rRNA Gene. PLoS One 10:e0136389. doi: 10.1371/journal.pone.0136389
Firmino, J. P., Fernández-Alacid, L., Vallejos-Vidal, E., Salomón, R., Ignasi Sanahuja, Tort, L., et al. (2021a). Carvacrol, thymol, and garlic essential oil promote skin innate immunity in gilthead seabream (Sparus aurata) through the multifactorial modulation of the secretory pathway and enhancement of mucus protective capacity. Front. Immunol. 12:633621. doi: 10.3389/fimmu.2021.633621
Firmino, J. P., Galindo-Villegas, J., Reyes-López, F. E., and Gisbert, E. (2021b). Phytogenic bioactive compounds shape fish mucosal immunity. Front. Immunol. 12:695973. doi: 10.3389/fimmu.2021.695973
Firmino, J. P., Vallejos-Vidal, E., Balebona, M. C., Ramayo-Caldas, Y., Cerezo, I. M., Salomón, R., et al. (2021c). Diet. Front. Immunol. 12:625297. doi: 10.3389/fimmu.2021.625297
Firmino, J. P., Vallejos-Vidal, E., Sarasquete, C., Delgado, J. B. O., Balasch, J. C., Tort, L., et al. (2020). Unveiling the effect of dietary essential oils supplementation in Sparus aurata gills and its efficiency against the infestation by Sparicotyle chrysophrii. Sci. Rep. 10:17764. doi: 10.1038/s41598-020-74625-5
Gao, Y. Y., Jiang, Z. Y., Lin, Y. C., Zheng, C. T., Zhou, G. L., and Chen, F. (2011). Effects of spray-dried animal plasma on serous and intestinal redox status and cytokines of neonatal piglets. J. Anim. Sci. 89, 150–157. doi: 10.2527/jas.2010-2967
Gisbert, E., Skalli, A., Campbell, J., Solovyev, M. M., Rodríguez, C., Dias, J., et al. (2015). Spray-dried plasma promotes growth, modulates the activity of antioxidant defenses, and enhances the immune status of gilthead sea bream (Sparus aurata) fingerlings. J. Anim. Sci. 93, 278–286. doi: 10.2527/jas2014-7491
Gomez, D., Sunyer, J. O., and Salinas, placeI. (2013). The mucosal immune system of fish: the evolution of tolerating commensals while fighting pathogens. Fish Shellfish Immunol. 35, 1729–1739. doi: 10.1016/j.fsi.2013.09.032
Gómez-Serrano, M., Camafeita, E., Loureiro, M., and Peral, B. (2018). Mitoproteomics: tackling mitochondrial dysfunction in human disease. Oxid. Med. Cell. Longev. 2018:1435934. doi: 10.1155/2018/1435934
Gurina, T., and Mohiuddin, S. (2021). Biochemistry, Protein Catabolism. Treasure StateFL: StatPearls Publ.
Hedrera, M. I., Galdames, J. A., Jimenez-Reyes, M. F., Reyes, A. E., Avendano-Herrera, R., Romero, J., et al. (2013). Soybean meal induces intestinal inflammation in zebrafish larvae. PLoS One 8:e69983. doi: 10.1371/journal.pone.0069983
Hwangbo, C., Tae, N., Lee, S., Kim, O., Park, O. K., Kim, J., et al. (2016). Syntenin regulates TGF-beta1-induced Smad activation and the epithelial-to-mesenchymal transition by inhibiting caveolin-mediated TGF-beta type I receptor internalization. Oncogene 35, 389–401. doi: 10.1038/onc.2015.100
Jantchou, P., Morois, S., Clavel-Chapelon, F., Boutron-Ruault, M. C., and Carbonnel, F. (2010). Animal protein intake and risk of inflammatory bowel disease: the E3N prospective study. Am. J. Gastroenterol. 105, 2195–2201. doi: 10.1038/ajg.2010.192
Johnson, J. A., and Summerfelt, R. C. (2000). Spray-dried blood cells as a partial replacement for fishmeal in diets for rainbow trout Oncorhynchus mykiss. J. World Aquac. Soc. 31, 96–104. doi: 10.1111/j.1749-7345.2000.tb00703.x
Khansari, A. R., Balasch, J. C., Vallejos-Vidal, E., Parra, D., Reyes-López, F. E., and Tort, L. (2018). Comparative immune- and stress-related transcript response induced by air exposure and Vibrio anguillarum bacterin in rainbow trout (Oncorhynchus mykiss) and Gilthead Seabream (Sparus aurata) mucosal surfaces. Front. Immunol. 9:856. doi: 10.3389/fimmu.2018.00856
Khansari, A. R., Balasch, J. C., Vallejos-Vidal, E., Teles, M., Fierro-Castro, C., Tort, L., et al. (2019). Comparative study of stress and immune-related transcript outcomes triggered by Vibrio anguillarum bacterin and air exposure stress in liver and spleen of gilthead seabream (Sparus aurata), zebrafish (Danio rerio) and rainbow trout (Oncorhynchus mykiss). Fish Shellfish Immunol. 86, 436–448. doi: 10.1016/j.fsi.2018.11.063
Kósa, B., Bárdová, K., Lešková, L., Reichel, P., Novotný, J., Link, R., et al. (2019). The effect of a feed additive supplemented with porcine plasma protein on growth performance and selected biochemical indices of nursing piglets. Acta Vet. Brno 88, 393–399. doi: 10.2754/avb201988040393
Kostovcikova, K., Coufal, S., Galanova, N., Fajstova, A., Hudcovic, T., Kostovcik, M., et al. (2019). Diet rich in animal protein promotes pro-inflammatory macrophage response and exacerbates colitis in mice. Front. Immunol. 10:919. doi: 10.3389/fimmu.2019.00919
Krogdahl, A., Bakke-McKellep, A. M., Roed, K. H., and Baeverdfjord, G. (2000). Feeding Atlantic Salmon Salmo salar L. soybean products: effects on disease resistance (furunculosis), and lysozyme and IgM levels in the intestinal mucosa. Aquac. Nutr. 6, 77–84. doi: 10.1046/j.1365-2095.2000.00129.x
Lalles, J. P. (2010). Intestinal alkaline phosphatase: multiple biological roles in maintenance of intestinal homeostasis and modulation by diet. Nutr. Rev. 68, 323–332. doi: 10.1111/j.1753-4887.2010.00292.x
Lambaerts, K., Van Dyck, S., Mortier, E., Ivarsson, Y., Degeest, G., Luyten, A., et al. (2012). Syntenin, a syndecan adaptor and an Arf6 phosphatidylinositol 4,5-bisphosphate effector, is essential for epiboly and gastrulation cell movements in zebrafish. J. Cell Sci. 125, 1129–1140. doi: 10.1242/jcs.089987
Lempiäinen, H., and Shore, D. (2009). Growth control and ribosome biogenesis. Curr. Opin. Cell Biol. 21, 855–863. doi: 10.1016/j.ceb.2009.09.002
Li, Y., Huang, Y., Cao, X., Yin, X., Jin, X., Liu, S., et al. (2018). Functional and structural characterization of zebrafish ASC. FEBS J. 285, 2691–2707. doi: 10.1111/febs.14514
Liu, Q., Chen, X. W., Che, C. J., Ding, D., and Kang, C. J. (2015). Syntenin is involved in the bacteria clearance response of kuruma shrimp (Marsupenaeus japonicus). Fish Shellfish Immunol. 44, 453–461. doi: 10.1016/j.fsi.2015.02.016
Masumoto, J., Kobayashi, H., Nakamura, T., Kaneko, Y., Ota, H., Hasegawa, M., et al. (2006). Regulation of the ASC expression in response to LPS stimulation is related to IL-8 secretion in the human intestinal mucosa. Biochem. Biophys. Res. Commun. 346, 968–973. doi: 10.1016/j.bbrc.2006.06.002
Oehlers, S. H., Flores, M. V., Chen, T., Hall, C. J., Crosier, K. E., and Crosier, P. S. (2011). Topographical distribution of antimicrobial genes in the zebrafish intestine. Dev. Comp. Immunol. 35, 385–391. doi: 10.1016/j.dci.2010.11.008
Parra, D., Reyes-Lopez, F. E., and Tort, L. (2015). Mucosal immunity and B cells in teleosts: effect of vaccination and stress. Front. Immunol. 6:354. doi: 10.3389/fimmu.2015.00354
Peace, R. M., Campbell, J., Polo, J., Crenshaw, J., Russell, L., and Moeser, A. (2011). Spray-dried porcine plasma influences intestinal barrier function, inflammation, and diarrhea in weaned pigs. J. Nutr. 141, 1312–1317. doi: 10.3945/jn.110.136796
Pérez-Bosque, A., Amat, C., Polo, J., Campbell, J. M., Crenshaw, J., Russell, L., et al. (2006). Spray-Dried animal plasma prevents the effects of staphylococcus aureus enterotoxin b on intestinal barrier function in weaned rats. J. Nutr. 136, 2838–2843. doi: 10.1093/jn/136.11.2838
Pérez-Bosque, A., Miró, L., Polo, J., Russell, L., Campbell, J., Weaver, E., et al. (2010). Dietary plasma protein supplements prevent the release of mucosal proinflammatory mediators in intestinal inflammation in rats. J. Nutr. 140, 25–30. doi: 10.3945/jn.109.112466
Pérez-Bosque, A., Polo, J., and Torrallardona, D. (2016). Spray dried plasma as an alternative to antibiotics in piglet feeds, mode of action and biosafety. Porc. Heal. Manag. 2:16. doi: 10.1186/s40813-016-0034-31
Perisic Nanut, M., Sabotic, J., Jewett, A., and Kos, J. (2014). Cysteine cathepsins as regulators of the cytotoxicity of NK and T cells. Front. Immunol. 5:616. doi: 10.3389/fimmu.2014.00616
Petschow, B. W., Blikslager, A. T., Weaver, E. M., Campbell, J. M., Polo, J., Shaw, A. L., et al. (2014). Bovine immunoglobulin protein isolates for the nutritional management of enteropathy. World J. Gastroenterol. 20, 11713–11726. doi: 10.3748/wjg.v20.i33.11713
Pierce, J. L., Cromwell, G. L., Lindemann, M. D., Russell, L. E., and Weaver, E. M. (2005). Effects of spray-dried animal plasma and immunoglobulins on performance of early weaned pigs. J. Anim. Sci. 83, 2876–2885. doi: 10.2527/2005.83122876x
Piomboni, P., Focarelli, R., Stendardi, A., Ferramosca, A., and Zara, V. (2012). The role of mitochondria in energy production for human sperm motility. Int. J. Androl. 35, 109–124. doi: 10.1111/j.1365-2605.2011.01218.x
Reyes-Cerpa, S., Vallejos-Vidal, E., José Gonzalez-Bown, M., Morales-Reyes, J., Pérez-Stuardo, D., Vargas, D., et al. (2018). Effect of yeast (Xanthophyllomyces dendrorhous) and plant (Saint John’s wort, lemon balm, and rosemary) extract based functional diets on antioxidant and immune status of Atlantic salmon (Salmo salar) subjected to crowding stress. Fish Shellfish Immunol. 74, 250–259. doi: 10.1016/j.fsi.2017.12.061
Reyes-López, F. E., Ibarz, A., Ordóñez-grande, B., Vallejos-Vidal, E., Sánchez-Nuño, S., Firmino, J. P., et al. (2021). Skin multi-omics-based interactome analysis: integrating the tissue and mucus exuded layer for a comprehensive understanding of the teleost mucosa functionality as model of study. Front. Immunol. 11:613824. doi: 10.3389/fimmu.2020.613824
Rodríguez, C., Saborido, N., Ródenas, J., and Polo, J. (2016). Effects of spray-dried animal plasma on food intake and apparent nutrient digestibility by cats when added to a wet pet food recipe. Anim. Feed Sci. Technol. 216, 243–250. doi: 10.1016/j.anifeedsci.2016.03.026
Salomón, R., Reyes-López, F. E., Tort, L., Firmino, J. P., Sarasquete, C., Ortiz-Delgado, J. B., et al. (2021). Medicinal plant leaf extract from sage and lemon verbena promotes intestinal immunity and barrier function in gilthead seabream (Sparus aurata). Front. Immunol. 12:670279. doi: 10.3389/fimmu.2021.670279
Saurabh, S., and Sahoo, P. K. (2008). Lysozyme: an important defence molecule of fish innate immune system. Aquac. Res. 39, 223–239. doi: 10.1111/j.1365-2109.2007.01883.x
Schenk, S., Schoenhals, G. J., de Souza, G., and Mann, M. (2008). A high confidence, manually validated human blood plasma protein reference set. BMC Med. Genomics 1:41. doi: 10.1186/1755-8794-1-41
Shiohara, M., Taniguchi, S., Masumoto, J., Yasui, K., Koike, K., Komiyama, A., et al. (2002). ASC, which is composed of a PYD and a CARD, is up-regulated by inflammation and apoptosis in human neutrophils. Biochem. Biophys. Res. Commun. 293, 1314–1318. doi: 10.1016/S0006-291X(02)00384-4
Sommer, N., Hüttemann, M., Pak, O., Scheibe, S., Knoepp, F., Sinkler, C., et al. (2017). Mitochondrial complex IV Subunit 4 Isoform 2 is essential for acute pulmonary oxygen sensing. Circ. Res. 121, 424–438. doi: 10.1161/CIRCRESAHA.116.310482
Suhane, S., Berel, D., and Ramanujan, V. K. (2011). Biomarker signatures of mitochondrial NDUFS3 in invasive breast carcinoma. Biochem. Biophys. Res. Commun. 412, 590–595. doi: 10.1016/j.bbrc.2011.08.003
Tacchi, L., Bickerdike, R., Douglas, A., Secombes, C. J., and Martin, S. A. M. (2011). Transcriptomic responses to functional feeds in Atlantic salmon (Salmo salar). Fish Shellfish Immunol. 31, 704–715. doi: 10.1016/j.fsi.2011.02.023
Tamura, K., Ikutani, M., Yoshida, T., Tanaka-Hayashi, A., Yanagibashi, T., Inoue, R., et al. (2015). Increased production of intestinal immunoglobulins in Syntenin-1-deficient mice. Immunobiology 220, 597–604. doi: 10.1016/j.imbio.2014.12.003
Tapia-Paniagua, S. T., Balebona, M., del, C., Firmino, J. P., Rodríguez, C., Polo, J., et al. (2020). The effect of spray-dried porcine plasma on gilthead seabream (Sparus aurata) intestinal microbiota. Aquac. Nutr. 26, 801–811. doi: 10.1111/anu.13039
Terry, S., Nie, M., Matter, K., and Balda, M. S. (2010). Rho signaling and tight junction functions. Physiology 25, 16–26. doi: 10.1152/physiol.00034.2009
Tran, H., Bundy, J. W., Li, Y. S., Carney-Hinkle, E. E., Miller, P. S., and Burkey, T. E. (2014). Effects of spray-dried porcine plasma on growth performance, immune response, total antioxidant capacity, and gut morphology of nursery pigs. J. Anim. Sci. 92, 4494–4504. doi: 10.2527/jas.2014-7620
Turk, V., Stoka, V., Vasiljeva, O., Renko, M., Sun, T., Turk, B., et al. (2012). Cysteine cathepsins: from structure, function and regulation to new frontiers. Biochim. Biophys. Acta 1824, 68–88. doi: 10.1016/j.bbapap.2011.10.002
Uran, P. A., Schrama, J. W., Rombout, J. H., Taverne-Thiele, J. J., Obach, A., Koppe, W., et al. (2009). Time-related changes of the intestinal morphology of Atlantic salmon, Salmo salar L., at two different soybean meal inclusion levels. J. Fish Dis. 32, 733–744. doi: 10.1111/j.1365-2761.2009.01049.x
Van Dijk, A. J., Enthoven, P. M., Van den Hoven, S. G., Van Laarhoven, M. M., Niewold, T. A., Nabuurs, M. J., et al. (2002). The effect of dietary spray-dried porcine plasma on clinical response in weaned piglets challenged with a pathogenic Escherichia coli. Vet. Microbiol. 84, 207–218.
van Dijk, A. J., Everts, H., Nabuurs, M. J. A., Margry, R. J. C. F., and Beynen, A. C. (2001). Growth performance of weanling pigs fed spray-dried animal plasma: a review. Livest. Prod. Sci. 68, 263–274. doi: 10.1080/10495390600956946
Van Vranken, J. G., Na, U., Winge, D. R., and Rutter, J. (2015). Protein-mediated assembly of succinate dehydrogenase and its cofactors. Crit. Rev. Biochem. Mol. Biol. 50, 168–180. doi: 10.3109/10409238.2014.990556
Verweij, F. J., Revenu, C., Arras, G., Dingli, F., Loew, D., Pegtel, D. M., et al. (2019). Live tracking of inter-organ communication by endogenous exosomes in vivo. Dev. Cell 48, 573–589.e4. doi: 10.1016/j.devcel.2019.01.004.
Warner, J. R. (1999). The economics of ribosome biosynthesis in yeast. Trends Biochem. Sci. 24, 437–440. doi: 10.1016/s0968-0004(99)01460-7
Watson, S. A., and McStay, G. P. (2020). Functions of cytochrome c oxidase assembly factors. Int. J. Mol. Sci. 21:7254. doi: 10.3390/ijms21197254
Keywords: teleost fishes, dietary additives, immune response, mucosal-associated lymphoid tissue (MALT), spray-dried porcine plasma (SDPP), aquaculture
Citation: Vallejos-Vidal E, Reyes-Cerpa S, Tort L, Polo J, Reyes-López FE and Gisbert E (2022) Spray-Dried Porcine Plasma Promotes the Association Between Metabolic and Immunological Processes at Transcriptional Level in Gilthead Sea Bream (Sparus aurata) Gut. Front. Mar. Sci. 9:814233. doi: 10.3389/fmars.2022.814233
Received: 13 November 2021; Accepted: 07 January 2022;
Published: 18 February 2022.
Edited by:
Samad Rahimnejad, University of South Bohemia in České Buděıjovice, CzechiaReviewed by:
Omid Safari, Ferdowsi University of Mashhad, IranFrancisco Javier Moyano, University of Almería, Spain
Jaume Pérez-Sánchez, Spanish National Research Council (CSIC), Spain
Copyright © 2022 Vallejos-Vidal, Reyes-Cerpa, Tort, Polo, Reyes-López and Gisbert. This is an open-access article distributed under the terms of the Creative Commons Attribution License (CC BY). The use, distribution or reproduction in other forums is permitted, provided the original author(s) and the copyright owner(s) are credited and that the original publication in this journal is cited, in accordance with accepted academic practice. No use, distribution or reproduction is permitted which does not comply with these terms.
*Correspondence: Felipe E. Reyes-López, ZmVsaXBlLnJleWVzLmxAdXNhY2guY2w=; ZmVsaXBlLnJleWVzQHVhYi5jYXQ=
†These authors share first authorship