- 1Quèbec-Ocèan, Dèpartement de biologie, Universitè Laval, Quèbec, QC, Canada
- 2Department of Zoology and Entomology, Rhodes University, Grahamstown, South Africa
- 3Fisheries and Oceans Canada, Institut Maurice-Lamontagne, Mont-Joli, QC, Canada
The choice of the duration and frequency of sampling to detect relevant patterns in field experiments or for environmental monitoring is always challenging since time and material resources are limited. In practice, duration and frequency of sampling are often chosen based on logistical constraints, experience, or practices described in published works but are rarely justified and almost never optimized before initiating sampling. Settlement plates are commonly used as a passive sampling tool to study recruitment patterns of fouling organisms (including non-indigenous species) and their deployment is amenable to experimentation with respect to manipulating duration and frequency of sampling. This study aimed to determine the optimal sampling strategy to detect rare species (e.g., a non-indigenous species early in the invasion process when its population size is still small). To do so, we deployed a series of settlement plates of various durations (1–32 days) and sampling frequencies (daily to biweekly) during the seasonal onset of recruitment, when larval supply was low, a situation that mimics the low propagule pressure of the early stages of the invasion process. We demonstrated that a combination of longer sampling duration and higher sampling frequency was the best strategy to maximize taxonomic richness. However, we found that an intermediate sampling duration of 1–2 weeks was optimal for detecting most species. These results can guide species-specific and assemblage-level sampling strategies using settlement plates. Additionally, this study can serve as a practical template for optimizing sampling of other taxonomic groups that were not examined in the present study as well as for the use of other methods.
Introduction
The natural environment is often sampled to describe the distributional patterns of species and to experimentally test hypotheses of biological mechanisms and processes, i.e., mensurative and manipulative studies, respectively (Hurlbert, 1984; Tilman, 1989; Underwood et al., 2000). Determining the appropriate spatial and temporal scales for sampling is, however, difficult with major consequences if the “wrong” scales are selected to address a particular research question (Richmond and Seed, 1991; Underwood, 1994). For temporal aspects, there are many dimensions to consider, including timing (when samples are taken), sampling duration (how long sampling is conducted), and sampling frequency (how often samples are taken within a given period; Thompson et al., 2002; Langhans and Tockner, 2006; Vaz-Pinto et al., 2014; Fletcher et al., 2018). In practice, temporal scales of sampling appear to be often chosen based on logistical constraints, past experience, or published work (e.g., in deference to established practices) but is rarely justified and almost never optimized before initiating a study, whether an ecological field experiment or an environmental monitoring program.
Failure to determine the relevant temporal scale for sampling may have serious implications for protecting endangered species, monitoring global changes in biodiversity and habitats, and detecting and eradicating invasive or potentially invasive (e.g., non-indigenous) species (Karanth et al., 2006; Underwood and Fisher, 2006; Robertson et al., 2010). These crucial monitoring and conservation efforts are especially challenging when limited resources need to be focused strategically to detect rare species while reducing the probability of false negatives, i.e., the conclusion that a species is not present when indeed it is. For biological invasions specifically, populations at the earliest stages of establishment are by their very nature rare (i.e., at low densities) and their spatial extent geographically localized. Likewise, when they undergo geographic spread, they are rare along invasion fronts. Finally, indigenous species (including endemic and endangered species) tend to be rare at the edges of their distributional range because the environmental or habitat suitability is often suboptimal for reproduction and growth (Canning-Clode et al., 2011; Cannizzo and Griffen, 2019).
Decisions concerning spatial and temporal aspects of sampling are particularly challenging when sampling for rare species because sampling methods may not be sufficiently sensitive for species detection. To overcome the high probability of false negatives, diversification of sampling tools and intensification of sampling effort are two techniques that are commonly used for invasive species management (Hayes et al., 2005; MacKenzie et al., 2005; Harvey et al., 2009; Tait et al., 2018). These approaches have been utilized to monitor for non-indigenous species because early detection of nascent non-indigenous populations, ideally before establishment, followed by rapid response can drastically increase the probability of eradication (Myers et al., 2000; Rejmánek and Pitcairn, 2002; Mehta et al., 2007). For instance, a diverse combination of sampling tools that consisted of active sampling (e.g., SCUBA surveys), passive sampling (e.g., settlement plates), and molecular tools (e.g., PCR-based assays) has successfully detected marine and estuarine non-indigenous species at early stages of their invasion process many years before established populations became evident (e.g., Chang et al., 2011; Moore et al., 2014; Ma et al., 2016; Kakkonen et al., 2019). Likewise, rapid assessments at locations deemed to have a high risk of invasions and “bio-blitzes” with the assistance of taxonomic experts and citizen scientists concentrate sampling effort over a short period of time to identify non-indigenous species at a given location (but not necessarily an area of high risk), which substantially reduces the chances of false negatives and misidentification of rare species (Arenas et al., 2006; Minchin, 2007; Bishop et al., 2015; Collin et al., 2015). However, in spite of the success of these approaches, temporal aspects of sampling (e.g., frequency or duration of sampling periods) have largely been ignored.
Many marine and estuarine non-indigenous species are associated with fouling communities on human-made structures, including dock structures, submerged aquaculture equipment, and boat hulls (Arenas et al., 2006; Lambert, 2007; Mineur et al., 2012; Pelletier-Rousseau et al., 2019). These anthropogenic structures are made of a variety of artificial materials, such as plastic and metal, which can be used as substrata for studying non-indigenous species (Gartner et al., 2016; Ma et al., 2017; Tait et al., 2018). In particular, settlement plates made of such material can be used as passive sampling tools to detect marine and estuarine non-indigenous species (predominantly invertebrate groups) because many species have biphasic life cycles, which include a small planktonic stage that disperses widely (Roughgarden et al., 1988; Hurlbut, 1992; Pineda et al., 2007; Floerl et al., 2012; Marraffini et al., 2017). These planktonic stages, typically spores or larvae, colonize suitable habitats and transition to the more sessile or sedentary adult stage of the life cycle (Pineda et al., 2007). Over time, a community of fouling species then develops on the bare surfaces of settlement plates (e.g., Allen and Ferguson Wood, 1950; Chalmer, 1982; Floerl et al., 2010). Because the biofilm that develops on submerged surfaces can act as a cue for settlement for some marine and estuarine species (Keough and Raimondi, 1995, 1996; Johnson et al., 1997; Wieczorek and Todd, 1997), some studies “season” experimental settlement plates by allowing them to develop biofilms before they are deployed or experimentally manipulated to enhance propagule recruitment (Johnson and Strathmann, 1989; Walters et al., 1999; Howes et al., 2007; Ma et al., 2017).
Over short time scales, the number of recruits found on settlement plates is an efficient estimate for propagule supply (i.e., the concentration of propagules in the water column; Gaines et al., 1985; Hurlbut, 1991, 1992; Minchinton and Scheibling, 1991; Yund et al., 1991; Miron et al., 1995; Todd et al., 2006). Therefore, short-term deployment of settlement plates can serve as a relatively sensitive sampling tool to detect competent propagules, even at low abundances, in marine and estuarine systems (e.g., Ma et al., 2020). The presence and abundance of propagules will, however, vary across space and time in response to biotic and abiotic processes, including the size, distribution and phenology of source populations, hydrodynamics, and sources of mortality during the dispersive stage (Kinlan and Gaines, 2003; Pineda et al., 2007, 2010). Regardless, determining the appropriate timescales for sampling can be difficult without proper evaluation. For passive sampling tools, two major temporal aspects that require immediate decision-making before an ecological study can proceed are the duration (somewhat comparable to the grain of sampling in spatial ecology) and the frequency of sampling (comparable to spatial lag; Thompson et al., 2002; Vaz-Pinto et al., 2014).
Intuitively, longer sampling duration (i.e., deployment) of settlement plates should increase the probability of detecting a given species by (1) allowing for the development of a biofilm, (2) increasing the numbers of competent that propagules encounter and settle on a plate, especially if gregarious settlement occurs, and (3) permitting organisms to grow to a larger size and, thereby, become easier to identify (Keough and Raimondi, 1996; Floerl et al., 2010; Vaz-Pinto et al., 2014; Tait et al., 2016). In contrast, shorter durations may encourage recruitment due to the availability of unoccupied space and can decrease the probability of juvenile mortality before plates are retrieved (i.e., before the need to account for natural sources of early post-settlement mortality, including predation and competition; Keough and Downes, 1982; Hunt and Scheibling, 1997). Moreover, observer or visibility bias may contribute to lower detectability of rare species on longer duration plates as an observer could easily miss a small rare species if a three-dimensional fouling assemblage has developed on a plate.
If larval settlement was simply determined by the plate encounter rate by competent larvae, then the expectation would be that the arithmetic addition of recruitment on two plates of shorter duration deployed one after another (i.e., additive recruitment) should be the same as the that measured on a single longer duration plate deployed over the same temporal period (i.e., cumulative recruitment). However, if longer sampling durations accumulate a higher number of recruits for a given species due to effects of biofilms or gregarious recruitment behavior, then additive recruitment should be lower than cumulative recruitment over the same period. In contrast, if larvae avoid predators or competitors during settlement (Grosberg, 1981; Johnson and Strathmann, 1989), then additive recruitment could be greater than cumulative recruitment over the same period.
Increasing sampling frequency (i.e., frequency of occurrence) is another temporal strategy that can also enhance rare species detection by (1) increasing the probability of sampling during peaks of propagule availability, (2) increasing the sampling effort, i.e., total plate surface area available over time for settlement, and (3) reducing the effect of biotic resistance from a more developed fouling assemblage or from dominant species (e.g., Dafforn et al., 2009; Collin and Johnson, 2014). However, lower sampling frequency uses fewer resources (time and material) and is generally favored. Moreover, it typically involves longer deployments, in which biotic interactions such as competition for space play a large role in structuring fouling assemblages. The trade-offs between short and long durations and between low and high sampling frequencies thus require careful consideration, especially under the usual constraints of a fixed sampling effort (e.g., the number of plates deployed during the entire sampling period or standardizing effort by time of plate exposure), to choose the optimal strategy.
A given temporal sampling strategy (e.g., sampling duration, sampling frequency, or a combination of both) may be considered optimal when the probability of rare species detection is greater than other strategies. However, there are several possible criteria for detection including (1) the earliest timing of species detection, (2) the greatest proportion of replicate samples with a positive detection, (3) the highest recruitment density (i.e., number of recruits per unit area), and (4) the highest recruitment rate (i.e., recruitment density per unit time or, even, per unit effort). If a majority of these four criteria yield the same optimal strategy, then this strategy should be the most sensitive for rare species detection. The first criterion is the most applicable for the objective of early detection (e.g., linked to the timing of an introduction event or the phenology of the species), and the remaining criteria are proxies for maximal detectability since they are linked to recruitment patterns. However, the second, third, and fourth criteria address different aspects of rare species detection: the chance of encountering the species, the overall probability of species detection (e.g., by human observers), and the methodological efficiency in sampling for a given species.
To conceptualize different strategies to optimize sampling duration and frequency, different scenarios of the propagule supply of rare species should also be considered. For example, if propagule supply is constant (i.e., “press” conditions), a one-time deployment of a settlement plate would be all that is needed for species detection. One would need a longer sampling duration if propagule supply was low (Figure 1A), but a shorter sampling duration would be sufficient if supply is higher (Figure 1B). An episodic pattern of propagule supply (“pulse” conditions) is, however, more realistic (e.g., Hurlbut, 1992) due to seasonal reproduction and patchy distributions of propagules in the water column. Unless one has prior knowledge of temporal patterns to guide timing of sampling (e.g., seasonal, lunar phasic, hourly patterns), increasing sampling frequency would increase the probability of species detection, regardless of the underlying temporal supply structure (Figures 1C,D).
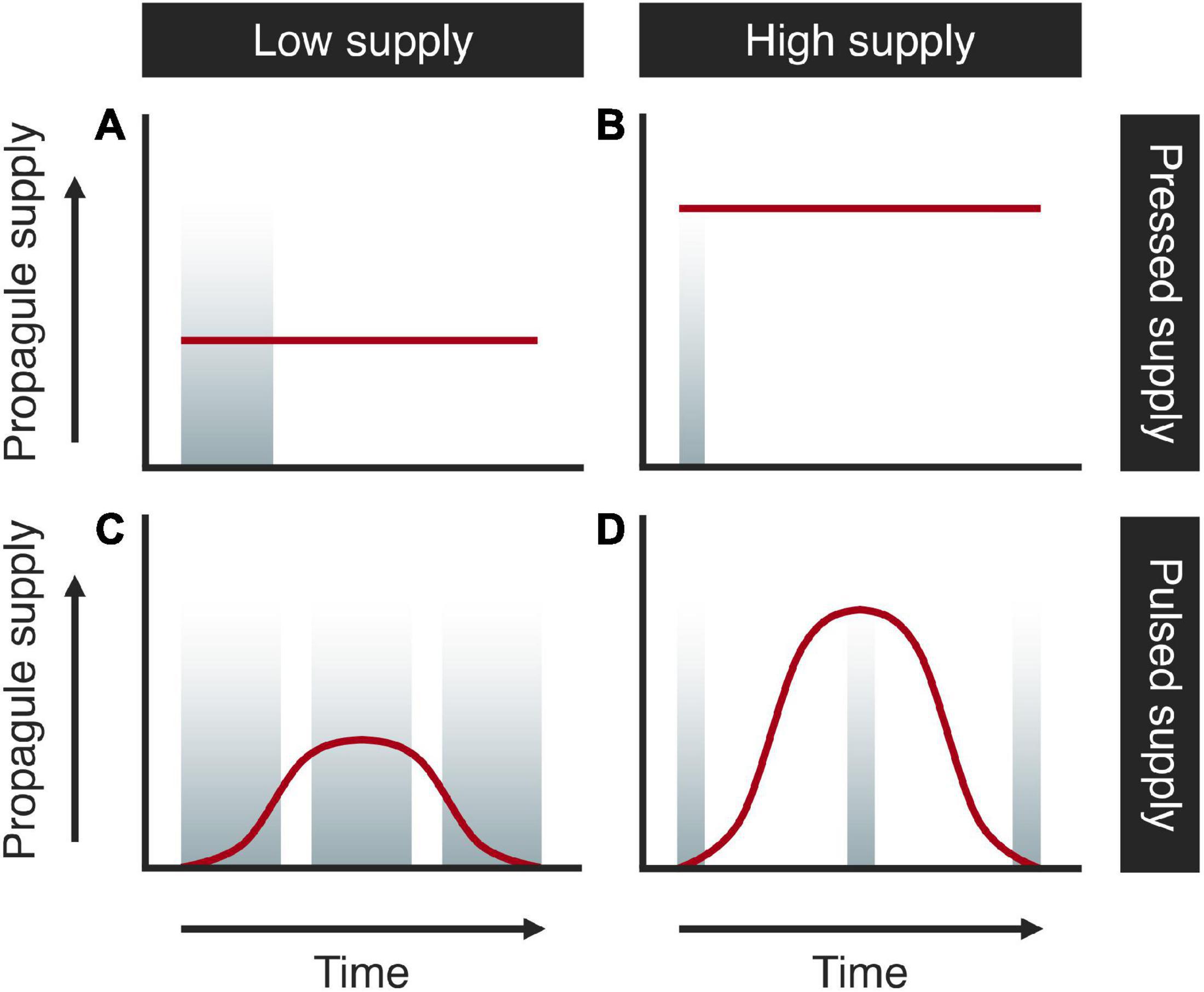
Figure 1. Temporal strategies to optimize sampling duration and sampling frequency under different scenarios of propagule supply (red line). (A) Pressed low supply; (B) pressed high supply; (C) pulsed low supply; (D) pulsed high supply. Width of shaded periods represents sampling duration (short vs. long) and number of shaded periods represents sampling frequency (low vs. high).
Despite these important considerations, few studies have evaluated how propagule supply dynamics influence sampling strategies using passive sampling tools (but see Simkanin et al., 2017). The objectives of this study were thus threefold. First, we evaluated relationships between the recruitment rate of benthic taxa and sampling duration. Specifically, we examined the relationship between additive and cumulative recruitment over a range of sampling durations. We focused on the short-term timescales of days to months because this is within the temporal scale at which settlement of propagules is expected to occur when a suitable substratum is made available (Wahl, 1989; Floerl et al., 2010). Second, we evaluated the optimal sampling duration for four different criteria for the detection of rare species (i.e., recruitment to plates under conditions of low propagule supply). In particular, we exploited the conditions of the rarity of recruitment at the onset of their reproductive season because the probability of detecting newly introduced non-indigenous species or rare indigenous species was deemed very unlikely. Third, we evaluated the optimal sampling strategy among combinations of different durations and frequencies to maximize taxonomic richness of rare species.
Materials and Methods
Sampling Protocol and Experimental Design
Settlement plates were used to assess the presence of benthic populations (mostly invertebrates) within a marina. Plates (dimensions: 14.6 × 14.6 cm) were cut from a 3-mm-thick sheet of dark gray polyvinyl chloride (PVC) with one surface roughened with a handheld belt sander. Plates were suspended horizontally from floating docks in the water column 1 m below the water surface with the roughened surface facing down. Each plate was suspended on a separate line from floating docks, such that the distance from any given plate to the nearest neighboring plate was >1 m. The study site, Ben Eoin marina (45.9818°N, 60.4314°W), was located in East Bay of Bras d’Or Lake, Nova Scotia, Canada, and covered an area of approximately 1.5 ha (average depth of 4 m).
Settlement plates were deployed for varying durations and frequencies during a 32-d period (1 July to 2 August 2014) during the seasonal onset of recruitment for many of the fouling organisms found in the marina basin. This study period was chosen based on the site-specific recruitment phenology of many local taxa determined during the preceding year (Ma, 2020). Six duration treatments (1, 2, 4, 8, 16, and 32 days) and five frequency treatments (1 [daily], 0.5 [every second day], 0.25 [every fourth day], 0.125 [every eighth day], and 0.031 [once during the study period] sampling events ⋅ d–1) were created by deploying, retrieving, and replacing plates at different times during the experiment (Figure 2), ranging from the daily replacement of plates deployed for a single day to a single deployment of plates for 32 days. For each unique combination of sampling duration and deployment time-point, six replicate plates were used (N = 738 total). All plates were deployed and collected between 09:00 and 10:00. Supplemental settlement plates were also deployed for an additional 8 days to extend the experiment for certain durations (4, 8, and 16 days, deployed every fourth day) and retrieved on 6 and 10 August 2014.
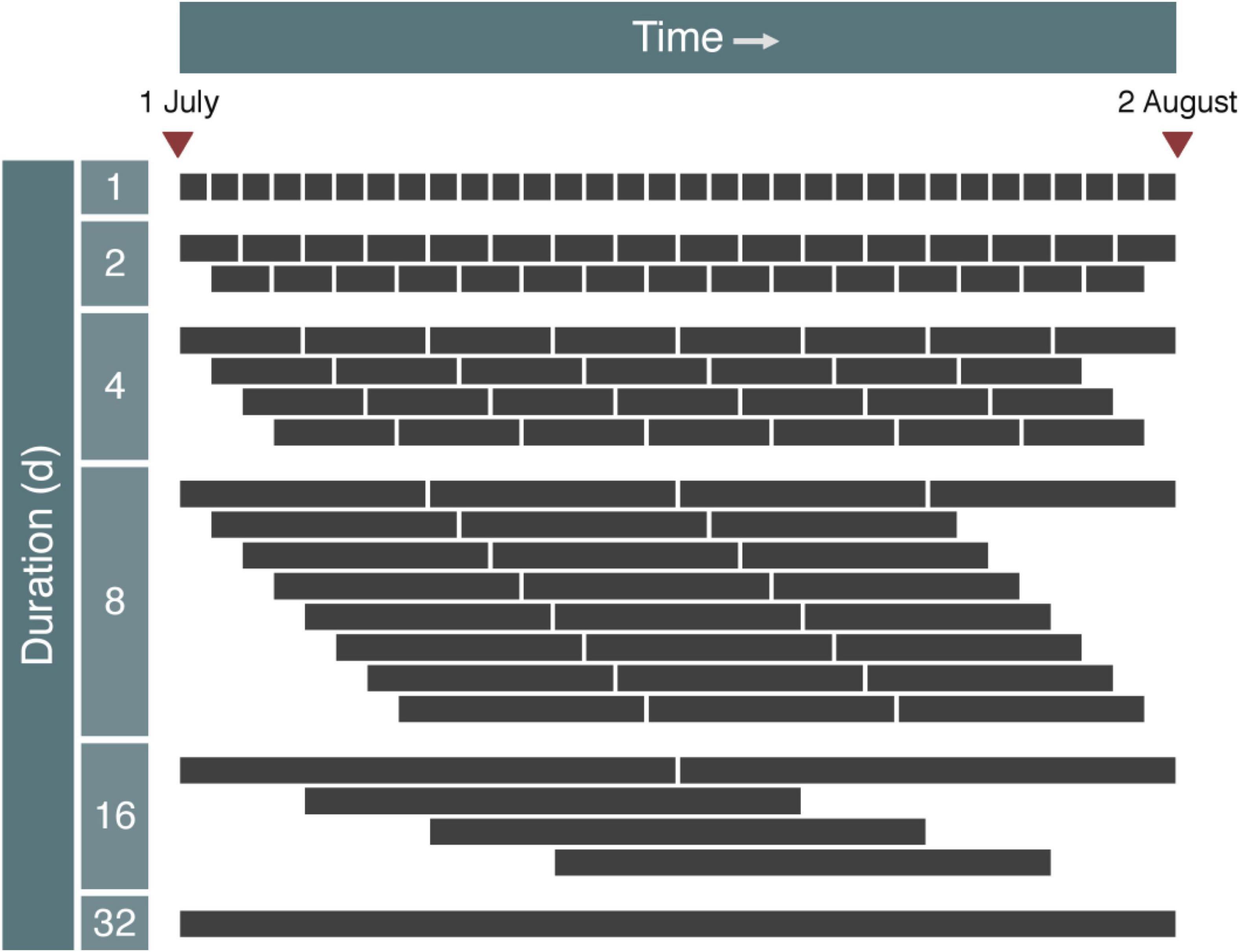
Figure 2. High-frequency sampling program using settlement plates with six different sampling durations ranging from 1 to 32 days. The experiment began on 1 July 2014 and terminated on 2 August 2014. Sampling program of supplemental settlement plates is not shown.
Within 1 or 2 h after retrieval, the entire downward-facing surface of each plate was examined under a stereomicroscope and all recruits counted. During the course of the experiment, a total of 22 benthic taxa were observed and identified to the lowest level possible, usually to species (Supplementary Table 1). For taxonomic identifications, we primarily consulted Pollock (1998) and Bullard and Whitlatch (2004). Further, we deployed six additional (i.e., sacrificial) settlement plates that were not part of our experiment design to periodically observe the development of newly recruited fouling organisms to aid in taxonomic identification, because more mature (and larger) specimens can exhibit additional morphological features (or render features more visible) that were key to identification.
Environmental Data
Near the geographic center of the marina, seawater temperature and salinity at a depth of 1 m below the water surface were determined daily around 10:00 using a portable YSI probe. From 1 July to 2 August, temperatures increased steadily from about 19.0 to 23.5°C. Salinity exhibited little variation during this period with a mean of 19.5 psu (range: 19.1–19.6; one standard deviation: 0.11).
Relationship Between Additive and Cumulative Recruitment
Nine benthic invertebrates (Amathia gracilis, an erect, uncalcified bryozoan; Botryllus schlosseri, a colonial ascidian; Bougainvillia carolinensis, an anthoathecate hydroid; Conopeum tenuissimum, an encrusting, calcified bryozoan; Crassostrea virginica, an oyster; Crisularia turrita, an erect, calcified bryozoan; Hiatella arctica, a clam; Lacuna vincta, a gastropod; and Mytilus sp., a mussel) were used to examine the relationship between additive and cumulative recruitment (recruits ⋅ plate–1 ⋅ d–1). These species were selected because there were sufficient data for the analysis, i.e., each of these species had more than 100 recruits counted throughout the 32-d experiment.
Each pair of data consisted of recruitment determined from two shorter duration plates (additive recruitment) vs. recruitment determined from one longer duration plate (cumulative recruitment) for the same unit effort (hereafter referred to as plate-days) and over the same temporal period. The experimental design allowed the comparison of a total of five possible plate-day conditions (2-, 4-, 8-, 16-, and 32-plate-days). Data from supplemental plates were also used to calculate some additive and cumulative recruitment values. Any two plates used to calculate additive recruitment were collectively deployed for the same length of time (duration) and during the same absolute period of time as the comparative single plate. For each species and plate-day condition, a linear regression was fitted to log10 (x + 0.01) transformed data. The addition of 0.01 in the data transformation was used to minimize distortions in the relationship due to the small recruitment values (e.g., McCune and Grace, 2002).
Species-by-Species Optimization
For any taxon detected, the optimal sampling duration was determined based on four criteria: (1) the earliest day (i.e., day of plate retrieval) of detection, (2) the greatest proportion of colonized replicate plates (a proxy for the least spatially patchy), (3) the highest recruitment density (recruits ⋅ plate–1), and (4) the highest recruitment rate (recruits ⋅ plate–1 ⋅ d–1). For each taxon and criterion combination, the six sampling durations (1–32 days) were ranked from optimal to least optimal. For each criterion, the distribution of taxon-specific optimal sampling durations was examined to summarize the results for all taxa detected, and then the overall optimal sampling duration (i.e., duration with the greatest proportion of species) was identified. To evaluate whether there were differences among the four criteria, a chi-square test was used to ascertain if there was a significant difference between the null distribution (i.e., no differences among durations) and the observed distribution of optimal sampling durations.
Assemblage-Level Optimization
Cumulative taxonomic richness was tracked over the course of the study period under 24 sampling strategies to determine the optimal temporal strategy [see the different duration treatments (Figure 2); the different sampling frequencies refer to the various rates of deploying a new set of replicate plates over the course of the 32-d experiment]. Based on our sampling program (Figure 2), 18 strategies consisted of various combinations of sampling durations (1, 2, 4, 8, 16 days) and frequencies (daily, every second day, every fourth day, every eighth day) and the other six strategies comprised a combination of different sampling durations (1, 2, 4, 8, 16, and 32-days) and a one-time deployment sampling frequency. A new set of replicate plates for four duration treatments (namely, 1, 2, 4, and 8 days) was deployed each day throughout the experiment, providing daily frequency (1 d–1) for these durations. However, these serial deployments could also be resampled (i.e., parsed) to generate taxonomic richness data for the lower frequencies for every second day, every fourth day, and every eighth day over the course of the 32-d experiment (frequencies of 0.5, 0.25, 0.125 sampling events ⋅ d–1, respectively). Similarly, plates for the 16-d duration treatment were deployed every fourth day and, again, could be resampled every eighth day to provide frequencies of 0.25 and 0.125 sampling events ⋅ d–1, respectively. From this dataset, the full complement of richness data, both from the high-frequency sampling program and the resampling procedure, was first used to construct species accumulation curves. The relationship of each strategy was then fitted to a logarithmic regression. Lastly, the mean taxonomic richness (number of taxa) and the mean richness standardized by effort (number of taxa per plate deployed) accumulated during the 32-d experiment, for each strategy, were plotted to evaluate the optimal sampling strategy. An analysis of variance [ANOVA, generalized linear model (GLM) procedure with Poisson error distribution] was used to evaluate the effects of Duration, Frequency, and Duration × Frequency on the number of taxa cumulated by the end of the 32-d experiment. Due to the unbalanced nature of the data, a GLM procedure (based on type III sums of squares) was applied to this ANOVA using IBM SPSS (Version 25). All other data manipulations and statistical analyses in this study were performed in the R programming environment (R Core Team, 2019).
Results
Seasonal Onset of Recruitment
A total of 22 benthic taxa was detected in this study (Supplementary Table 1). These taxa are considered indigenous except for the colonial ascidian B. schlosseri, which is considered a non-indigenous or a cryptogenic species in eastern Canada (Yund et al., 2015; Ma et al., 2016). Five taxa in this study, however, are non-indigenous elsewhere in the world where they have been introduced (A. gracilis, an erect, uncalcified bryozoan; Asterias rubens, a sea star; C. tenuissimum, an encrusting, calcified bryozoan; C. virginica, an oyster; and Crepidula fornicata, a gastropod; World Register of Introduced Marine Species [WRiMS], 2021).
Recruitment rates (data combined from all durations treatments), the seasonal onset of recruitment (determined as the first day of their observation) over the 32-d study period and the seasonal trajectory of recruitment on 8- and 16-d duration plates varied among the nine most commonly observed invertebrates (Figure 3). Besides periphytic diatoms, which were detected on plates at the beginning of the experiment, Anaperus gardineri (a flatworm), Folliculina sp. (a protist), Gammarus sp. (an amphipod), L. vincta (a gastropod; Figure 3H), and Mytilus sp. (a mussel; Figure 3I) were among the earliest species to recruit on plates. Conversely, the seasonal onset of A. rubens, B. schlosseri (Figure 3B), C. virginica (Figure 3E), C. fornicata, Littorina sp. (a gastropod), and Tenellia fuscata (a nudibranch) recruitment began later, during the latter part of the experiment.
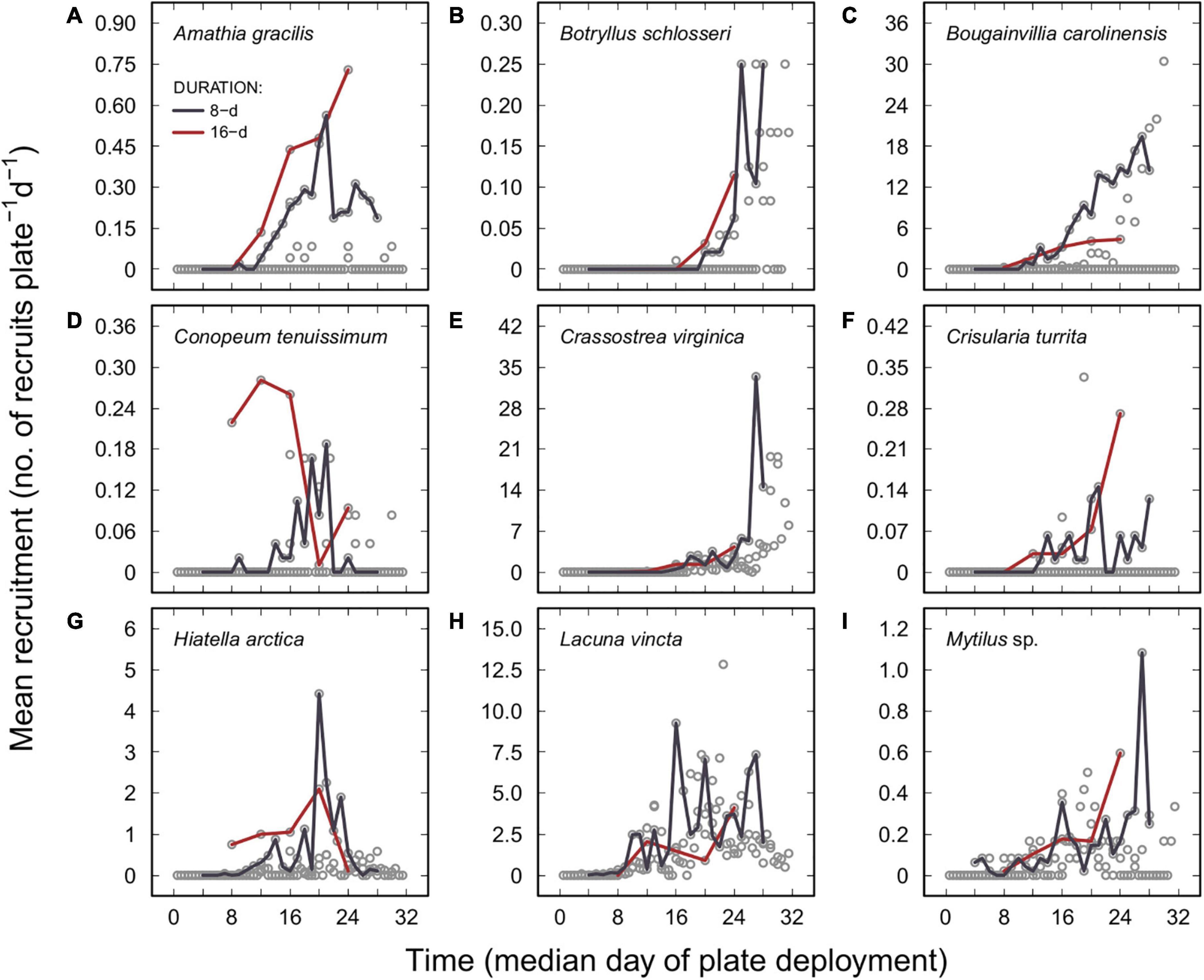
Figure 3. Recruitment rates, data combined from all duration treatments (e.g., 1-, 2-, 4-, 8-, 16-, and 32-d duration plates), of the nine most commonly encountered benthic invertebrates between 1 July and 2 August 2014. (A) Amathia gracilis; (B) Botryllus schlosseri; (C) Bougainvillia carolinensis; (D) Conopeum tenuissimum; (E) Crassostrea virginica; (F) Crisularia turrita; (G) Hiatella arctica; (H) Lacuna vincta; and (I) Mytilus sp. Open circles (°) = mean recruitment rates (mean of six replicates, including all zero values). Lines show seasonal trajectory of recruitment based on data from 8-d duration plates (purple lines) and 16-duration plates (red lines).
Six taxa exhibited relatively sustained recruitment rates from the date of their seasonal onset of recruitment until the end of the 32-d study period. These taxa included A. gardineri, Actiniaria (comprising a single morphotype), Bittiolum alternatum (a gastropod), C. fornicata, Gammarus sp., and periphytic diatoms. By contrast, recruitment densities of nine taxa tended to increase over time. These taxa included A. gracilis (Figure 3A), B. schlosseri (Figure 3B), B. carolinensis (an anthoathecate hydroid; Figure 3C), C. virginica (Figure 3E), C. turrita (an erect, calcified bryozoan; Figure 3F), Folliculina sp., L. vincta (Figure 3H), Mytilus sp. (Figure 3I), and Spionidae (a morphotype of polychaete worms). Two other taxa, C. tenuissimum (Figure 3D) and H. arctica (a clam; Figure 3G), exhibited pulsed recruitment patterns, peaking during the middle of the experiment (Figure 3). The pattern of recruitment of Sterreria psammicola (a flatworm) and Nereididae (a morphotype of polychaete worms) was temporally disjunct over the course of the experiment. Patterns, however, were unclear for A. rubens, Littorina sp., and T. fuscata, due to the onset of their recruitment occurring near the end of the study.
With a maximal rate of ≤1.0 recruit ⋅ plate–1 ⋅ d–1, recruitment rates on plates were lowest for eight benthic invertebrates (A. rubens, C. fornicata, C. tenuissimum, Littorina sp., Nereididae, S. psammicola, Spionidae, and T. fuscata) over the course of the 32-d study period. Seven other invertebrates (e.g., A. gardineri, A. gracilis, Actiniaria, B. alternatum, B. schlosseri, C. turrita, and Mytilus sp.) had maximal recruitment densities ranging from 1.5 to 3.1 recruits ⋅ plate–1 ⋅ d–1. Finally, high recruitment rates were observed for the five other invertebrates: B. carolinensis (maximal rate: 35.8 recruits ⋅ plate–1 ⋅ d–1), C. virginica (65.8 recruits ⋅ plate–1 ⋅ d–1), Gammarus sp. (6.0 recruits ⋅ plate–1 ⋅ d–1), H. arctica (10.0 recruits ⋅ plate–1 ⋅ d–1), and L. vincta (26.6 recruits ⋅ plate–1 ⋅ d–1).
Relationship Between Additive and Cumulative Recruitment
For all possible species-specific plate-day conditions, significant positive relationships between additive and cumulative recruitment were found for a majority (five out of nine) of the species with sufficient recruitment for analysis (B. carolinensis, B. schlosseri, C. turrita, C. virginica, and L. vincta; p ≤ 0.037) and mostly associated with a good fit between the (log-transformed) data and the regression (R2 > 0.50, except for the 2-plate-day condition for B. schlosseri [R2 = 0.46, p < 0.001] and the 8-plate-day condition for C. turrita [R2 = 0.16, p = 0.037]; Supplementary Table 2). The remaining four taxa (A. gracilis, C. tenuissimum, H. arctica, and Mytilus sp.) had at least one non-significant plate day conditions and, for significant conditions, a weaker fit between the (log-transformed) data and the regression (R2 < 0.50, except for the 16-plate-day condition of A. gracilis [R2 = 0.99, p < 0.001]; Supplementary Table 2). Even considering that the data were plotted on a log–log scale, some of the significant relationships were generally close to a 1:1 ratio such that slopes ranging from 0.72 to 1.32 were found in all plate-day conditions (Figure 4 and Supplementary Table 2). In particular, slopes ranged from 0.50 to 1.08 for five species with significant positive relationships between additive and cumulative recruitment in the 2-plate-day condition and only three of these species were close to the 1:1 ratio (i.e., slope > 0.70). In the 4-plate-day condition, slopes ranged from 0.43 to 1.32 and the relationships of four out of six species were close to the 1:1 ratio. In the 8-plate-day condition, slopes ranged from 0.52 to 1.32 and the relationships of six out of eight species were close to the 1:1 ratio. Lastly, in the 16-plate-day condition, slopes ranged from 0.79 to 1.99 and the relationships of four out of six species were close to the 1:1 ratio. Taken altogether, the slopes of a majority of species in each plate-day condition were close to the 1:1 ratio and the 2-, 4-, and 8-plate-day conditions tended to have slopes less than one (i.e., additive recruitment < cumulative recruitment) and the 16-plate-day condition tended to have slopes greater than one (i.e., additive recruitment > cumulative recruitment; Figure 4 and Supplementary Table 2).
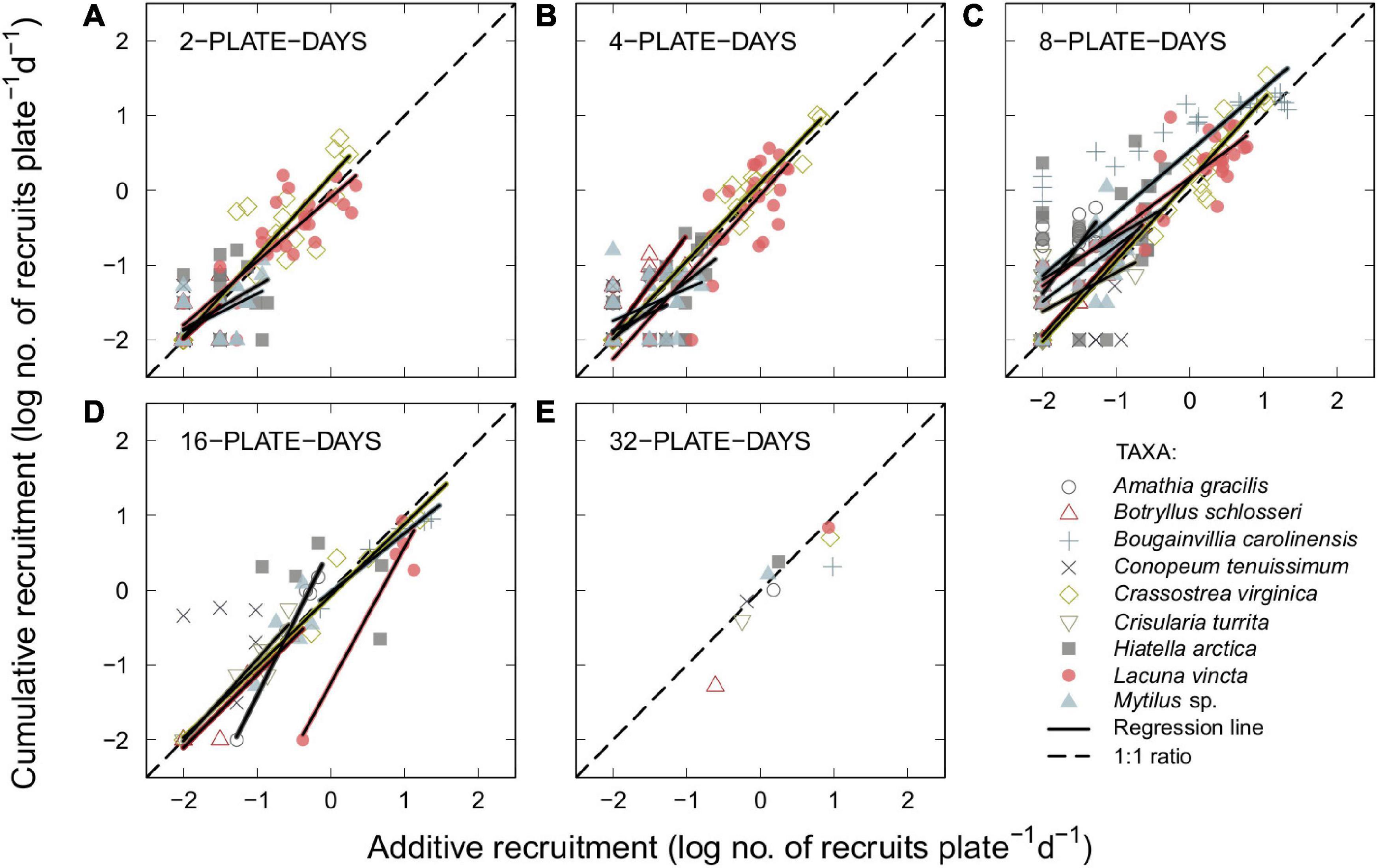
Figure 4. Relationships between additive and cumulative recruitment evaluated for five plate-day conditions. Black solid line (—) = linear regression based on log10 (x + 0.01) transformed data for each individual benthic taxon; non-significant regressions are not shown; black dashed line (—) = 1:1 ratio for visual reference. (A) 2-plate-days (n = 186 data points); (B) 4-plate-days (n = 203); (C) 8-plate-days (n = 243); (D) 16-plate-days (n = 171); and (E) 32-plate-days (n = 9). No data for Amathia gracilis for the 2-plate-day condition and for Bougainvillia carolinensis and Crisularia turrita for 2- and 4-plate-day conditions.
Species-by-Species Optimization
The use of 8-d sampling duration was optimal for the greatest proportion of taxa in terms of earliest detection (64% of taxa), detection of the highest recruitment density (41%), and detection of the highest recruitment rate (27%; Figure 5). The use of the 16-d sampling duration was optimal for having the greatest proportion of colonized replicate plates, a proxy for the least spatially patchy (77%; Figure 5). The four criteria used to evaluate optimal sampling duration were all significantly different from one another (χ2 = 130; df = 15, p < 0.001) but, overall, the intermediate durations (i.e., 8- and 16-d) were considered as optimal.
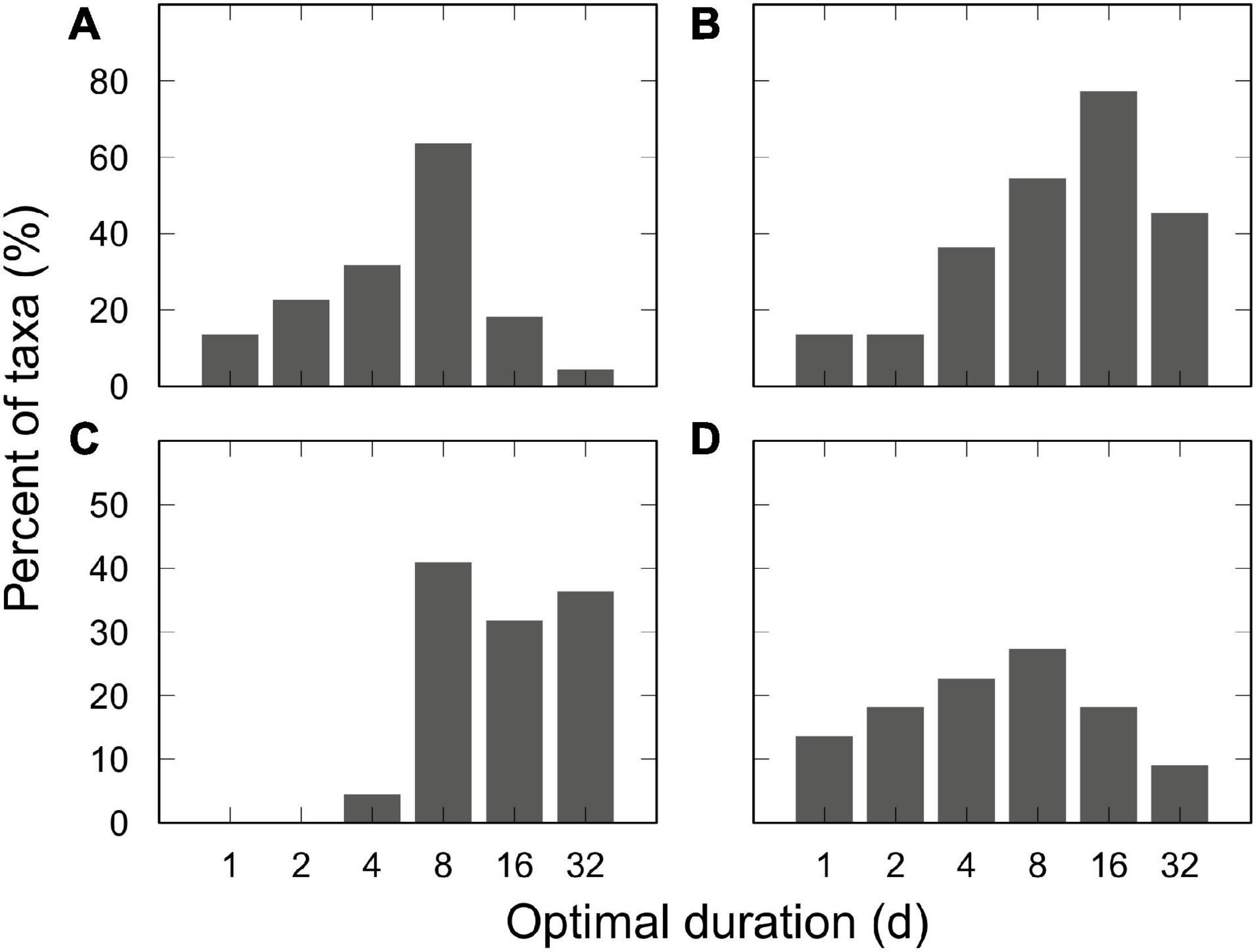
Figure 5. Distribution of optimal sampling durations for an assemblage of 22 benthic taxa under low propagule supply evaluated using four criteria. Criteria: (A) earliest day of species detection; (B) greatest proportion of colonized replicate plates; (C) highest recruitment density; and (D) highest recruitment rate. For each criterion, percentages do not add up to 100 due to instances (of a given taxa) where multiple durations were tied for the optimal position.
Assemblage-Level Optimization
The number of taxa accumulated during the 32-d experiment varied significantly among sampling durations and sampling frequencies but not as a function of the interaction between these two variables (Table 1). Overall, longer sampling durations of a given sampling frequency were more likely to detect more species by the end of the experiment than were shorter durations (Figure 6A and Supplementary Figure 2). Likewise, higher sampling frequencies of a given sampling duration detected more species by the end of the experiment than did lower frequencies (Figure 6A). However, within the range of durations and frequencies considered in this study, more species were detected by increasing sampling duration (up to ca. 500% more taxa) than by increasing sampling frequency (only up to ca. 160%; Figure 6A).
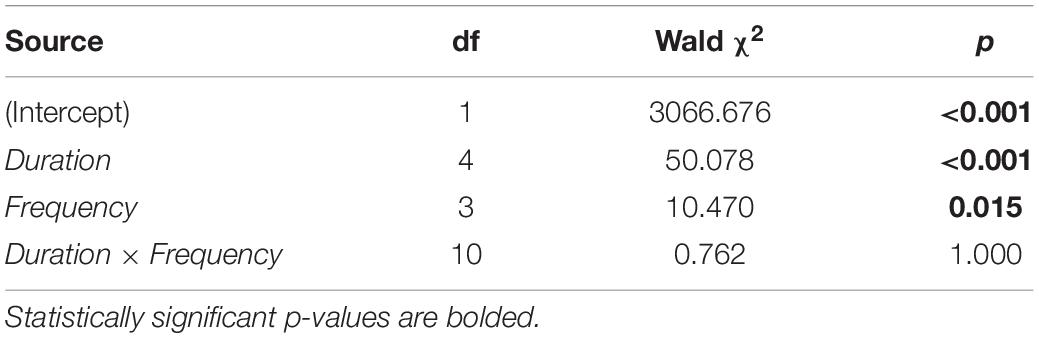
Table 1. Results of an analysis of variance (ANOVA, GLM procedure with Poisson error distribution) testing the effects of Duration, Frequency, and Duration × Frequency on the number of species accumulated during the 32-d experiment.
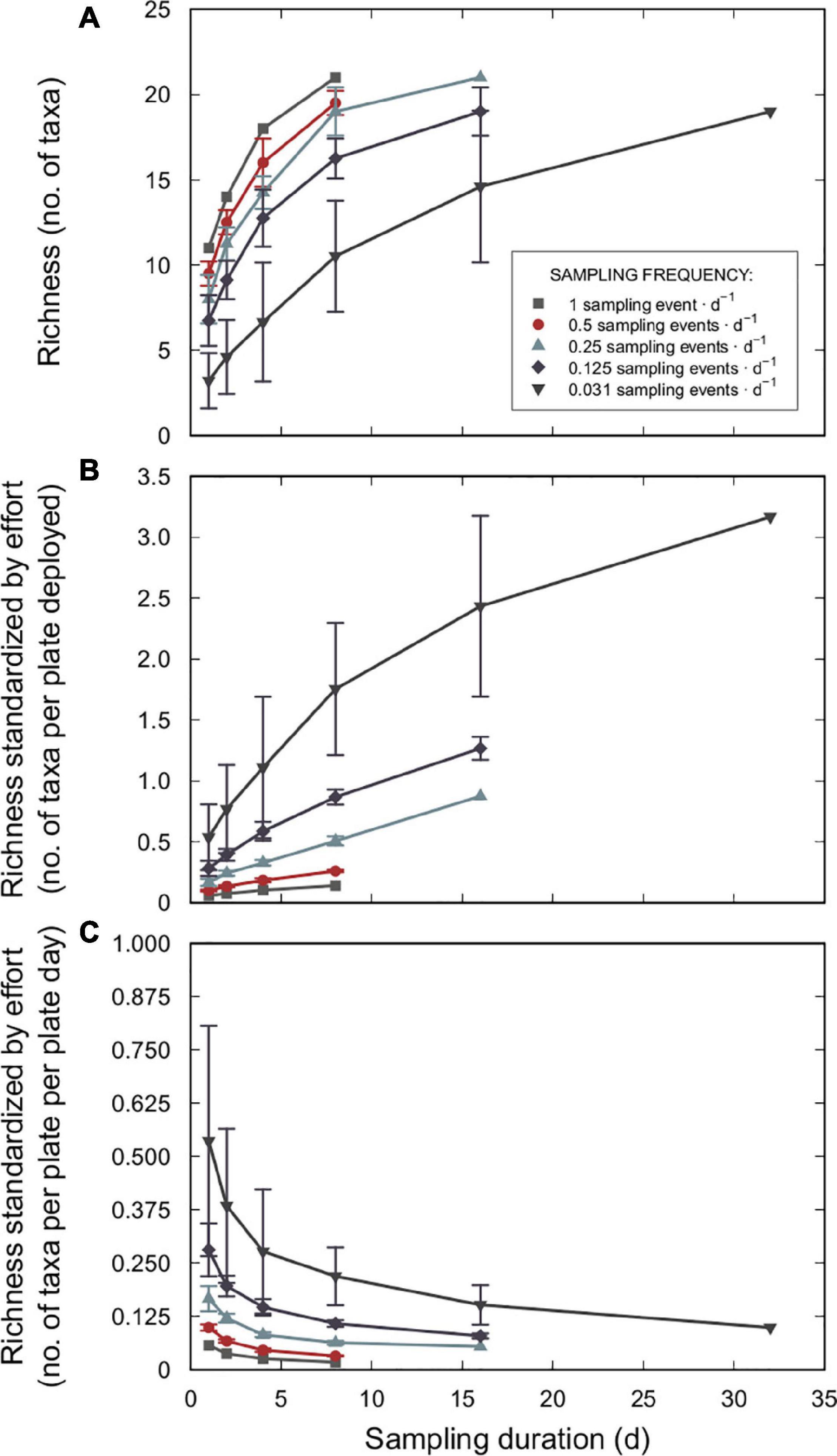
Figure 6. (A) Mean taxonomic richness accumulated by the final day of the 32-d experiment as a function of sampling duration (plate submergence time); see Supplementary Figure 1 for the original data used to calculate each data point. (B) Mean taxonomic richness standardized by sampling effort (number of plates deployed) accumulated by the final day of the 32-d experiment as a function of sampling duration. (C) Mean taxonomic richness standardized by sampling effort (number of plates deployed per plate day) accumulated by the final day of the 32-d experiment as a function of sampling duration. Sampling frequency refers to the rate of deploying a new set of replicate plates over the course of the experiment (sampling frequencies: 1 [daily], 0.5 [every second day], 0.25 [every fourth day], 0.125 [every eighth day], and 0.031 [once during the study period] sampling events ⋅ d–1). Each data point is the mean value for each sampling strategy. Bars represent ± one standard deviation. No standard deviation associated with the 1 sampling event ⋅ d–1 frequency because values are based on one accumulation curve.
Species detection (richness) as a function of duration was influenced by the species-specific recruitment patterns to the six different duration treatments. In particular, (a) two taxa (Actinaria and A. gracilis) did not recruit onto 1-d duration plates, (b) three taxa (A. rubens, C. turrita, and Littorina sp.) onto 1- or 2-d duration plates, (c) two taxa (Nereididae and Spionidae) onto 1-, 2-, or 4-d duration plates, and (d) one taxon (T. fuscata) onto 1-, 2-, 4-, or 8-d duration plates. In contrast, three taxa (A. gardineri, C. fornicata, and S. psammicola) did not recruit onto the longest duration plates (32-d). Strikingly, C. fornicata also did not recruit onto two shortest duration plates (1- and 2-d). Peculiarly, in addition to the 32-d plates, S. psammicola also did not recruit onto 1-, 4-, or 16-d duration plates.
For each sampling strategy (i.e., duration-frequency combination), species accumulated over time but no strategy detected all 22 taxa observed in the study (Supplementary Figure 1; no analysis could be made for any one-time deployment strategies because they consisted of a single value, i.e., 19 taxa accumulated by the end of the 32-days study period). However, two strategies detected 95% of the taxa: (1) 8-d duration at 1 d–1 sampling frequency and (2) 16-d duration at 0.25 d–1 frequency (Supplementary Figure 1). However, the sampling effort required for these two strategies differed substantially (i.e., 150 vs. 30 plates, which corresponds to 1,200 vs. 480 plate days). Almost all regressions (17 out 18 models) showed a logarithmic relationship between time elapsed and the cumulative number of species detected (Supplementary Table 3 and Supplementary Figure 2).
When sampling effort from the perspective of the human effort needed to deploy and retrieve plates was accounted for, the mean taxonomic richness per plate deployed was substantially diminished for increasingly higher sampling frequency strategies, such that one-time deployments were more efficient and resulted in greater richness per plate deployed (Figure 6B). However, when standardized for human effort and time until plate retrieval (i.e., delay), the mean richness per plate per day decreased markedly with increasingly longer sampling duration and higher sampling frequency strategies (Figure 6C). Correspondingly, shorter durations (e.g., 1 day) and lower frequencies (e.g., one-time deployment) were more efficient and were associated with greater richness per plate per day.
Discussion
Species-Specific Optimization
Ideally, temporal sampling strategies, such as duration and frequency, should be tested regularly over time (since conditions may continually change), but based on the four criteria used in this study, our results clearly show that an intermediate sampling duration between 1 and 2 weeks can be optimal for species detection when propagule supply is low (i.e., <1 recruit ⋅ plate–1 ⋅ d–1 for 92% of the plates retrieved in this study). Intriguingly, there was a general agreement among the diverse criteria used in this study, which indicates that the optimal deployment duration was indeed an intermediate one of 1–2 weeks. This also suggests that optimization based on other criteria, such as the highest recruitment densities or rates, are appropriate methods to determine optimal conditions for sampling. Further, enhanced species detection via intermediate duration has practical implications for both the design of ecological studies and monitoring for rare species using passive sampling tools, such as settlement plates. In such studies, settlement plates could be used to quantify recruitment rates of targeted fouling species or to monitor the development of fouling assemblages. However, in earlier studies, typical deployment durations were from several weeks to several months (e.g., Floerl et al., 2010; Ma et al., 2017; Marraffini et al., 2017; Tait et al., 2018). Thus, a shorter sampling duration (e.g., Stachowicz et al., 2002) might be better for species detection, such as during seasonal onset of recruitment as well as determining the end of the reproductive season, both situations when propagule supply would be expected to be low.
Additionally, the biological response (recruitment) using the targeted species’ optimal sampling duration would also be associated with the least amount of temporal variation in environmental conditions when compared to unoptimized, longer durations (e.g., Sephton et al., 2011). Monitoring for rare species (e.g., endangered species, nascent populations of non-indigenous species; Espinosa et al., 2011; Collin et al., 2013) using settlement plates can be achieved by intensive sampling at small spatial scales; however, optimizing for sampling duration could yield the desired outcome with less sampling effort (assuming that the optimal seasonal window for sampling is known, which may vary by species). The context-specific (e.g., depending on target species and sites) identification of the optimal sampling duration has important implications for early detection of non-indigenous species, since any time saved by using a shorter sampling duration would directly contribute to even earlier species detection and, thus, allow for a more rapid response (Harvey et al., 2009). And, if early detection at the timescales of days and weeks truly matters, then shorter durations and, therefore, earlier plate retrieval relative to longer durations will potentially translate to an earlier time of (a) species identification (which is possible for certain taxa; e.g., Stachowicz et al., 2002) and (b) reporting new records of non-indigenous species. The enhanced sensitivity of shorter durations to detect rare species when sampling with settlement plates raises the possibility of applying this passive sampling tool for early detection and monitoring in remote areas and for rapid assessment surveys (including bio-blitzes) when the temporal window for sampling is limited. Thus, if a sampling window of 1–2 weeks is logistically feasible during the seasonal recruitment period, then settlement plates can be utilized for both survey scenarios.
Optimal strategies for species detection may actually entail a sampling design with different combinations of sampling duration, i.e., using long and short durations concurrently. Notably, ecological studies and monitoring using settlement plates may not necessarily use equal sampling duration throughout the entire study period due to unforeseen circumstances such as extreme weather events or logistical constraints (Ma et al., 2017; Sokolowski et al., 2017). Recruitment data can be standardized as a rate value as part of the data processing and analysis. However, this process of standardization assumes that any biological or physical process acting upon settlement plates and fouling organisms is constant over time, which may be problematic for data interpretation if sampling duration (and, similarly, frequency) vary substantially within or among studies. Our results reveal that it is conceptually possible to recalibrate recruitment rates among different short-term sampling durations (e.g., shown simplistically in this study as the relationship between additive and cumulative recruitment). We recommend due care in the implementation of a sampling strategy to be taken to properly standardize biological response derived from different sampling durations, for instance, for meta-analyses. Moreover, the general 1:1 relationship between additive and cumulative recruitment over the same time period reveals that encounter rate of competent larvae shapes recruitment rates on different sampling durations over the timescales investigated in this study.
Assemblage-Level Optimization
Despite arguments for both long and short durations, increasing sampling duration increased the number of species detected over time. Unlike species-specific optimization, no intermediate sampling duration was found, but the slopes of the species accumulation curves appeared to begin to plateau over the short timescales used in this study (logarithmic growth). Although there was a tendency of upward parallel shift with each increase in sampling frequency, this was likely a function of sampling effort with respect to the number of plates deployed. This logarithmic growth pattern suggests that there is a diminishing return on maximizing taxonomic richness with longer sampling duration, which was evident even after standardizing for sampling effort with respect to material resources (i.e., per plate deployed). This was, however, not the case after standardizing for effort with respect to both time and material resources (i.e., per plate per day) and, instead of logarithmic growth, exhibited a pattern of exponential decay. Although longer durations, nevertheless, tend to detect more species than do shorter durations, some species (i.e., recruits of A. gardineri, C. fornicata, and S. psammicola) were not detected on the longest sampling durations. There could be several explanations for this. First, some species may settle on the plates and then leave the substratum. This case is plausible for mobile species such as sea stars, which may fall off the plate once they grow to a larger size. Second, observer or visibility bias may make it difficult to detect small and rare species that can take advantage of the three-dimensional structure of the fouling community (e.g., the presence of erect bryozoans and hydroids even after several weeks) and evade detection. In this study, this is a plausible scenario for the free-living flatworms (Platyhelminthes: Turbellaria), which their detection on shorter duration plates was made easier when the human observer can see them moving against the background of the bare settlement plate. Third, the net effect of interspecific interactions, such as the order or timing of species arrival (i.e., priority effects; Fukami, 2015) and competition for space, may eliminate or substantially reduce the abundance of certain species over time; however, this scenario is unlikely for the timescales and community examined in this study because substantial space was available, even after 32 days of exposure.
Although this study demonstrates that increasing sampling frequency can increase the number of species detected, a much greater effort was required for gains in species detection over the short timescales examined over the study period. Nevertheless, increased sampling frequency can improve the detection of rare species by simply spreading high sampling effort over time and providing finer temporal coverage between sampling time points, although this improvement in species detection came at a great cost after standardizing for sampling effort (both in time and material resources). Furthermore, the combination of high sampling frequency and long sampling duration allows for multiple trajectories of ecological succession to be sampled, each with a potentially different end point (depending on the sampling duration) despite trending toward convergence in the composition and structure in fouling assemblage over the long term and culminating in a climax community (Vance, 1988). The structure of fouling assemblages depends on seasonal timing and, to a lesser extent, year of plate deployment because different species recruit at different times and these initial recruits inevitably shape community development over the long term (Sutherland, 1974; Otsuka and Dauer, 1982). This outcome—shaped by seasonality (and shifts in seasonality associated with human-mediated climate change; Stachowicz et al., 2002) and biotic interactions (e.g., competition for space)—is consistent with our result that a sampling strategy involving longer durations and high frequencies can maximize taxonomic richness. For instance, the sharp decline in C. tenuissimum recruitment on 16-d duration plates was likely a result of being outcompeted by other species that recruited during the latter half of the study period (Figure 3). The main drawback of increasing sampling frequency is the increased cost of producing and handling more settlement plates, including the transport of plates to the site (i.e., cost of increased visits to sites). In practice, increasing sampling frequency may not be worth the extra effort, especially given that lengthening durations have greater returns in species detection than increasing frequencies, albeit over short timescales and that altering sampling duration does not have this same drawback in cost. Further studies to experimentally test the effect of sampling frequency would require separate series of plates for each frequency, although this would require a substantial number of plates to achieve independence of samples.
Temporal Sampling Strategies to Detect Rare Species
Temporal variation in propagule supply (and thus settlement) ultimately affects how sampling should be designed to optimize the detection of rare species. Although the timing of this study targeted the seasonal onset of reproduction of multiple species when propagule supply was expected to be low, our findings provide general guidance on how to optimize temporal aspects of sampling. Four temporal sampling scenarios concerning sampling duration and sampling frequency were conceptualized and introduced under different supply patterns (Figure 1). When viewed from a coarse timescale, supply and settlement rates exhibit seasonal variability such that the onset (and end) of the reproductive season is associated with low propagule supply and settlement rates. At finer scales, pulsed supply and local settlement patterns could be resolved for some species if the right durations and frequencies are used in the sampling design to detect these patterns. In this study, we observed the seasonal onset of settlement onto plates and, for some species, evidence of pulsed recruitment over short temporal periods (e.g., C. tenuissimum and H. arctica; Figure 3), which corresponds to the scenario shown in Figure 1C. The results from both the species-specific and assemblage-level optimization analyses indicate that short sampling durations are not optimal under conditions of low propagule supply (i.e., rarity). Interestingly, the optimal sampling duration for a majority of species, when analyzed species-by-species, was an intermediate duration (in the order of weeks). However, when analyzed at the assemblage-level, the optimal duration was the longer one of about 1 month. In this study, we only analyzed the effect of sampling frequency on species detection at the assemblage-level and found that the optimal frequency was the higher frequency of daily sampling. This finding was, however, undoubtedly due to the sheer increase in sampling effort, which, in turn, captures a greater amount of temporal variability related to variations in propagule supply and diel variations in propagule releases (e.g., larvae; Hurlbut, 1992; Bourque et al., 2007; Manríquez et al., 2016; Ma et al., 2020). Moreover, the combination of short sampling durations and low sampling frequencies, despite efficiencies in effort, appears to be the least optimal strategy for the detection of rare species. Indeed, after controlling for sampling effort with respect to material resources, the strategy with the lowest frequency examined in the study (0.031 sampling events ⋅ d–1), coupled with the longest duration (32 d), was the most efficient in detecting species under low propagule densities, at least on an assemblage-wide basis. If, however, time resource (i.e., time elapsed between deploying and retrieving plates) is also an important consideration in addition to material resources, then the strategy associated with the lowest frequency (0.031 sampling events ⋅ d–1) and the shortest duration (1 day), was the most efficient in detecting species under low propagule densities. Despite inherent inefficiencies in effort, these findings indicate that increasingly piecemeal gains in species detection associated with longer sampling durations and higher sampling frequencies would require further decision making with regards to resource allocation.
Conclusion
Initial settlement patterns and rare species are difficult to observe in nature because of the inherent challenges in designing sampling strategies that are optimized to detect their presence (MacKenzie et al., 2005; Tait et al., 2016). In many ways, different sampling durations represent different sampling methods; therefore, an investigator should take appropriate steps to calibrate their data when comparing recruitment rates determined from plates with different immersion times (e.g., comparing of rates within a study or among studies). Such calibrations may be possible by using the relationship between additive and cumulative recruitment that we investigated for several fouling invertebrate species. Moreover, our unique experimental design allowed for a better comparison of in situ sampling duration. In practice, comparing data among sampling durations is complex and difficult because the investigator cannot control for both sampling start and end dates, which confounds results interpretation. Arguments for long sampling durations for species detection seem to outweigh arguments for short durations. However, our findings support the use of an intermediate sampling duration over a chosen temporal sampling window to detect individual rare species within month-long timescales. Further experiments are needed to determine if this generalization can be scaled up and if similar temporal optimization procedures can be applied to other ecological and conservation contexts (i.e., optimal duration will probably depend on taxa and geography), and we have proposed a conceptual framework to optimize temporal sampling strategies (a combination of duration and frequency) to different scenarios of propagule supply. Our results were consistent with the expectation that a sampling strategy involving longer sampling duration and higher sampling frequency would maximize detection (e.g., taxonomic richness) under a temporally varied (e.g., pulsed) low propagule supply scenario, which best represents the reality of this study (seasonal onset of reproduction).
Data Availability Statement
The raw data supporting the conclusions of this article will be made available by the authors, without undue reservation.
Author Contributions
KCKM contributed to conception and design of the study, field collections, sample processing, data curation and analyses, and writing of the manuscript. CWM and LEJ contributed to writing of the manuscript, interpretation of the data, and funding. All authors contributed to manuscript revisions, read, and approved the submitted version.
Funding
Financial and in-kind supported from the Natural Sciences and Engineering Research Council (NSERC), Fisheries and Oceans Canada, Québec-Océan, and Université Laval for the Canadian Aquatic Invasive Species Network II (CAISN II) within which this research was carried out.
Conflict of Interest
The authors declare that the research was conducted in the absence of any commercial or financial relationships that could be construed as a potential conflict of interest.
Publisher’s Note
All claims expressed in this article are solely those of the authors and do not necessarily represent those of their affiliated organizations, or those of the publisher, the editors and the reviewers. Any product that may be evaluated in this article, or claim that may be made by its manufacturer, is not guaranteed or endorsed by the publisher.
Acknowledgments
We thank Caroline Potvin for field assistance, the management and users of Ben Eoin Marina for permitting the use of their floating docks and facilities, and the two reviewers for their comments and suggestions on an earlier version of this manuscript.
Supplementary Material
The Supplementary Material for this article can be found online at: https://www.frontiersin.org/articles/10.3389/fmars.2022.809327/full#supplementary-material
References
Allen, F. E., and Ferguson Wood, E. J. (1950). Investigation on underwater fouling. II. The biology of fouling in Australia: results of a year’s research. Aust. J. Mar. Freshw. Res. 1, 92–105. doi: 10.1071/MF9500092
Arenas, F., Bishop, J. D. D., Carlton, J. T., Dyrynda, P. J., Farnham, W. F., Gonzalez, D. J., et al. (2006). Alien species and other notable records from a rapid assessment survey of marinas on the south coast of England. J. Mar. Biol. Assoc. UK 86, 1329–1337. doi: 10.1017/S0025315406014354
Bishop, J. D. D., Wood, C. A., Lévêque, L., Yunnie, A. L. E., and Viard, F. (2015). Repeated rapid assessment surveys reveal contrasting trends in occupancy of marinas by non-indigenous species on opposite sides of the western English Channel. Mar. Pollut. Bull. 95, 699–706. doi: 10.1016/j.marpolbul.2014.11.043
Bourque, D., Davidson, J., MacNair, N. G., Arsenault, G., LeBlanc, A. R., and Miron, G. (2007). Reproduction and early life history of an invasive ascidian Styela clava Herdman in Prince Edward Island, Canada. J. Exp. Mar. Biol. Ecol. 342, 78–84. doi: 10.1016/j.jembe.2006.10.017
Bullard, S. G., and Whitlatch, R. B. (2004). A Guide to the Larval and Juvenile Stages of Common Long Island Sound Ascidians and Bryozoans. Groton: University of Connecticut.
Canning-Clode, J., Fowler, A. E., Byers, J. E., Carlton, J. T., and Ruiz, G. M. (2011). ‘Caribbean Creep’ chills outs: climate change and marine invasive species. PLoS One 6:e29657. doi: 10.1371/journal.pone.0029657
Cannizzo, Z. J., and Griffen, B. D. (2019). An artificial habitat facilitates a climate-mediated range expansion into a suboptimal novel ecosystem. PLoS One 14:e0211638. doi: 10.1371/journal.pone.0211638
Chalmer, P. N. (1982). Settlement patterns of species in a marine fouling community and some mechanisms of succession. J. Exp. Mar. Biol. Ecol. 58, 73–85. doi: 10.1016/0022-0981(82)90098-3
Chang, A. L., Blakeslee, A. M., Miller, A. W., and Ruiz, G. M. (2011). Establishment failure in biological invasions: a case history of Littorina littorea in California, USA. PLoS One 6:e16035. doi: 10.1371/journal.pone.0016035
Collin, S. B., Edwards, P. K., Leung, B., and Johnson, L. E. (2013). Optimizing early detection of non-indigenous species: estimating the scale of dispersal of a nascent population of the invasive tunicate Ciona intestinalis (L.). Mar. Pollut. Bull. 73, 64–69. doi: 10.1016/j.marpolbul.2013.05.040
Collin, S. B., and Johnson, L. E. (2014). Invasive species contribute to biotic resistance: negative effect of Caprellid amphipods on an invasive tunicate. Biol. Invasions 16, 2209–2219. doi: 10.1007/s10530-014-0659-4
Collin, S. B., Tweddle, J. F., and Shucksmith, R. J. (2015). Rapid Assessment of marine non-native species in the Shetland Islands, Scotland. BioInvasions Rec. 4, 147–155. doi: 10.3391/bir.2015.4.3.01
Dafforn, K. A., Johnston, E. L., and Glasby, T. M. (2009). Shallow moving structures promote marine invader dominance. Biofouling 25, 277–287. doi: 10.1080/08927010802710618
Espinosa, F., Rivera-Ingraham, G., and García-Gómez, J. C. (2011). Influence of habitat structure and nature of substratum on limpet recruitment: conservation implications for endangered species. Estuar. Coast Shelf Sci. 94, 164–171. doi: 10.1016/j.ecss.2011.06.005
Fletcher, L. M., Atalah, J., and Forrest, B. M. (2018). Effect of substrate deployment timing and reproductive strategy on patterns in invasiveness of the colonial ascidian Didemnum vexillum. Mar. Environ. Res. 141, 109–118. doi: 10.1016/j.marenvres.2018.08.006
Floerl, O., Inglis, G., Peacock, L., and Plew, D. (2012). The Efficacy of Settlement Plate Arrays for Marine Surveillance. Wellington: Ministry of Primary Industries.
Floerl, O., Wilkens, S., and Woods, C. (2010). Temporal Development of Biofouling Assemblages. Auckland: National Institute of Water and Atmospheric Research Ltd.
Fukami, T. (2015). Historical contingency in community assembly: integrating niches, species pools, and priority effects. Annu. Rev. Ecol. Syst. 46, 1–23. doi: 10.1146/annurev-ecolsys-110411-160340
Gaines, S., Brown, S., and Roughgarden, J. (1985). Spatial variation in larval concentrations as a cause of spatial variation in settlement for the barnacle, Balanus glandula. Oecologia 2, 267–272. doi: 10.1007/BF00384297
Gartner, H. N., Clarke Murray, C., Frey, M. A., Nelson, J. C., Larson, K. L., Ruiz, G. M., et al. (2016). Non-indigenous invertebrate species in the marine fouling communities of British Columbia, Canada. BioInvasions Rec. 5, 205–212. doi: 10.3391/bir.2016.5.4.03
Grosberg, R. K. (1981). Competitive ability influences habitat choice in marine invertebrates. Nature 290, 700–702. doi: 10.1038/290700a0
Harvey, C. T., Qureshi, S. A., andMacIsaac, H. J. (2009). Detection of a colonizing, aquatic, non-indigenous species. Divers. Distrib. 15, 429–437. doi: 10.1111/j.1472-4642.2008.00550.x
Hayes, K. R., Cannon, R., Neil, K., and Inglis, G. (2005). Sensitivity and cost considerations for the detection and eradication of marine pests in ports. Mar. Pollut. Bull. 50, 823–834. doi: 10.1016/j.marpolbul.2005.02.032
Howes, S., Herbinger, C. M., Darnell, P., and Vercaemer, B. (2007). Spatial and temporal patterns of recruitment of the tunicate Ciona intestinalis on a mussel farm in Nova Scotia, Canada. J. Exp. Mar. Biol. Ecol. 342, 85–92. doi: 10.1016/j.jembe.2006.10.018
Hunt, H. L., and Scheibling, R. E. (1997). Role of early post-settlement mortality in recruitment of benthic marine invertebrates. Mar. Ecol. Prog. Ser. 155, 269–301. doi: 10.3354/meps155269
Hurlbert, S. H. (1984). Pseudoreplication and the design of ecological field experiments. Ecol. Monogr. 54, 187–211. doi: 10.2307/1942661
Hurlbut, C. J. (1991). The effects of larval abundance, settlement and juvenile mortality on the depth distribution of a colonial ascidian. J. Exp. Mar. Biol. Ecol. 150, 183–202. doi: 10.1016/0022-0981(91)90067-7
Hurlbut, C. J. (1992). Larval release and supply predict temporal variation in settlement of a colonial ascidian. Mar. Ecol. Prog. Ser. 80, 215–219. doi: 10.3354/meps080215
Johnson, C. R., Lewis, T. E., Nichols, D. S., and Degnan, B. M. (1997). “Bacterial induction of settlement and metamorphosis in marine invertebrates,” in Proceedings of the 8th International Coral Reef Symposium, eds H. A. Lessios and I. G. Macintyre (Balboa: Smithsonian Tropical Research Institute), 1219–1224.
Johnson, L. E., and Strathmann, R. R. (1989). Settling barnacle larvae avoid substrata previously occupied by a mobile predator. J. Exp. Mar. Biol. Ecol. 128, 87–103. doi: 10.1016/0022-0981(89)90094-4
Kakkonen, J. E., Worsfold, T. M., Ashelby, C. W., Taylor, A., and Beaton, K. (2019). The value of regular monitoring and diverse sampling techniques to assess aquatic non-native species: a case study from Orkney. Manag. Biol. Invasions 10, 46–79. doi: 10.3391/mbi.2019.10.1.04
Karanth, K. U., Nichols, J. D., Kumar, N. S., and Hines, J. E. (2006). Assessing tiger population dynamics using photographic capture-recapture sampling. Ecology 87, 2925–2937. doi: 10.1890/0012-9658(2006)87[2925:atpdup]2.0.co;2
Keough, M. J., and Downes, B. J. (1982). Recruitment of marine invertebrates: the role of active larval choices and early mortality. Oecologia 54, 348–353. doi: 10.1007/BF00380003
Keough, M. J., and Raimondi, P. T. (1995). Responses of settling invertebrate larvae to bioorganic films: effects of different types of films. J. Exp. Mar. Biol. Ecol. 185, 235–253. doi: 10.1016/0022-0981(94)00154-6
Keough, M. J., and Raimondi, P. T. (1996). Responses of settling invertebrate larvae to bioorganic films: effects of large-scale variation in films. J. Exp. Mar. Biol. Ecol. 207, 59–78. doi: 10.1016/S0022-0981(96)02632-9
Kinlan, B. P., and Gaines, S. D. (2003). Propagule dispersal in marine and terrestrial environments: a community perspective. Ecology 84, 2007–2020. doi: 10.1890/01-0622
Lambert, G. (2007). Invasive sea squirts: a growing global problem. J. Exp. Mar. Biol. Ecol. 342, 3–4. doi: 10.1016/j.jembe.2006.10.009
Langhans, S. D., and Tockner, K. (2006). The role of timing, duration, and frequency of inundation in controlling leaf litter decomposition in a river-floodplain ecosystem (Tagliamento, northeastern Italy). Oecologia 147, 501–509. doi: 10.1007/s00442-005-0282-2
Ma, K. C. K. (2020). Rare Species Detection and Benthic Recruitment across Multiple Scales of Space and Time with Implications for Early Detection of Marine Invasive Species. Ph.D. thesis. Québec: Université Laval.
Ma, K. C. K., Deibel, D., Lowen, J. B., and McKenzie, C. H. (2017). Spatio-temporal dynamics of ascidian larval recruitment and colony abundance in a non-indigenous Newfoundland population. Mar. Ecol. Prog. Ser. 585, 99–112. doi: 10.3354/meps12437
Ma, K. C. K., McKindsey, C. W., and Johnson, L. E. (2020). Larval supply is a limited determinant of settlement at mesoscales across an anthropogenic seascape. Hydrobiologia 847, 4015–4029. doi: 10.1007/s10750-020-04391-y
Ma, K. C. K., Simard, N., Stewart-Clark, S. E., Bernier, R. Y., Nadeau, M., and Willis, J. (2016). Early detection of the non-indigenous colonial ascidian Diplosoma listerianum in eastern Canada and its implications for monitoring. Manag. Biol. Invasions 7, 365–374. doi: 10.3391/mbi.2016.7.4.06
MacKenzie, D. I., Nichols, J. D., Sutton, N., Kawanishi, K., and Bailey, L. L. (2005). Improving inferences in population studies of rare species that are detected imperfectly. Ecology 86, 1101–1113. doi: 10.1890/04-1060
Manríquez, P. H., Castilla, J. C., Ortiz, V., and Jara, M. E. (2016). Empirical evidence for large-scale human impact on intertidal aggregations, larval supply and recruitment of Pyura praeputialis around the Bay of Antofagasta, Chile. Austral Ecol. 41, 701–714. doi: 10.1111/aec.12359
Marraffini, M. L., Ashton, G. V., Brown, C. W., Chang, A. L., and Ruiz, G. M. (2017). Settlement plates as monitoring devices for non-indigenous species in marine fouling communities. Manag. Biol. Invasions 8, 559–566. doi: 10.3391/mbi.2017.8.4.11
McCune, B., and Grace, J. B. (2002). “Data transformations,” in Analysis of Ecological Communities, eds B. McCune, J. B. Grace, and D. L. Urban (Gleneden Beach: MjM Software Design), 67–89.
Mehta, S. V., Haight, R. G., Homans, F. R., Polasky, S., and Venette, R. C. (2007). Optimal detection and control strategies for invasive species management. Ecol. Econ. 61, 237–245. doi: 10.1016/j.ecolecon.2006.10.024
Minchin, D. (2007). Rapid coastal survey for targeted alien species associated with floating pontoons in Ireland. Aquat. Invasions 2, 63–70. doi: 10.3391/ai.2007.2.1.8
Minchinton, T. E., and Scheibling, R. E. (1991). The influence of larval supply and settlement on the population structure of barnacles. Ecology 72, 1867–1879. doi: 10.2307/1940984
Mineur, F., Cook, E. J., Minchin, D., Bohn, K., MacLeod, A., and Maggs, C. A. (2012). Changing coasts: marine aliens and artificial structures. Oceanogr. Mar. Biol. Ann. Rev. 50, 189–234. doi: 10.1201/b12157
Miron, G., Boudreau, B., and Bourget, E. (1995). Use of larval supply in benthic ecology: testing correlations between larval supply and larval settlement. Mar. Ecol. Prog. Ser. 124, 301–305. doi: 10.3354/meps124301
Moore, A. M., Vercaemer, B., DiBacco, C., Sephton, D., and Ma, K. C. K. (2014). Invading Nova Scotia: first records of Didemnum vexillum Kott, 2002 and four more non-indigenous invertebrates in 2012 and 2013. BioInvasions Rec. 3, 225–234. doi: 10.3391/bir.2014.3.4.03
Myers, J. H., Simberloff, D., Kuris, A. M., and Carey, J. R. (2000). Eradication revisited: dealing with exotic species. Trends Ecol. Evol. 15, 316–320. doi: 10.1016/S0169-5347(00)01914-5
Otsuka, C. M., and Dauer, D. M. (1982). Fouling community dynamics in Lynnhaven Bay, Virginia. Estuaries 5, 10–22. doi: 10.2307/1352212
Pelletier-Rousseau, M., Bernier, R., Clarke Murray, C., Drolet, D., Lacoursière-Roussel, A., Locke, A., et al. (2019). Assessment of recreational boating as a vector for marine non-indigenous species on the Atlantic coast of Canada. Biol. Invasions 21, 2447–2470. doi: 10.1007/s10530-019-01991-1
Pineda, J., Hare, J. A., and Sponaugle, S. (2007). Larval transport and dispersal in the coastal ocean and consequences for population connectivity. Oceanography 20, 22–39. doi: 10.5670/oceanog.2007.27
Pineda, J., Porri, F., Starczak, V., and Blythe, J. (2010). Causes of decoupling between larval supply and settlement and consequences for understanding recruitment and population connectivity. J. Exp. Mar. Biol. Ecol. 392, 9–21. doi: 10.1016/j.jembe.2010.04.008
Pollock, L. W. (1998). A Practical Guide to the Marine Animals of Northeastern North America. New Brunswick: Rutgers University Press.
R Core Team (2019). R: A Language and Environment for Statistical Computing. Vienna: R Foundation for Statistical Computing.
Rejmánek, M., and Pitcairn, M. J. (2002). “When is eradication of exotic pest plants a realistic goal?” in Turning the Tide: The Eradication of Invasive Species, eds C. R. Veitch and M. N. Clout (Gland: IUCN/SSC Invasive Species Specialist Group), 249–253.
Richmond, M. D., and Seed, R. (1991). A review of marine macrofouling communities with special reference to animal fouling. Biofouling 3, 151–168. doi: 10.1080/08927019109378169
Robertson, M. P., Cumming, G. S., and Erasmus, B. F. N. (2010). Getting the most of atlas data. Divers. Distrib. 16, 363–375. doi: 10.1111/j.1472-4642.2010.00639.x
Roughgarden, J., Gaines, S., and Possingham, H. (1988). Recruitment dynamics in complex life cycles. Science 241, 1460–1466. doi: 10.1126/science.11538249
Sephton, D., Vercaemer, B., Nicolas, J. M., and Keays, K. (2011). Monitoring for invasive tunicates in Nova Scotia, Canada (2006-2009). Aquat. Invasions 6, 391–403. doi: 10.3391/ai.2011.6.4.04
Simkanin, C., Davidson, I. C., Therriault, T. W., Jamieson, G., and Dower, J. F. (2017). Manipulating propagule pressure to test the invasibility of subtidal marine habitats. Biol. Invasions 19, 1565–1575. doi: 10.1007/s10530-017-1379-3
Sokolowski, A., Ziółkowska, M., Balazy, P., Kukliński, P., and Plichta, I. (2017). Seasonal and multi-annual patterns of colonisation and growth of sessile benthic fauna on artificial substrates in the brackish low-diversity system of the Baltic Sea. Hydrobiologia 790, 183–200. doi: 10.1007/s10750-016-3043-9
Stachowicz, J. J., Terwin, J. R., Whitlatch, R. B., and Osman, R. W. (2002). Linking climate change and biological invasions: ocean warming facilitates nonindigenous species invasions. Proc. Natl. Acad. Sci. U. S. A. 24, 15497–15500. doi: 10.1073/pnas.242437499
Sutherland, J. P. (1974). Multiple stable points in natural communities. Am. Nat. 108, 859–873. doi: 10.1086/282961
Tait, L., Inglis, G., and Seaward, K. (2018). Enhancing passive sampling tools for detecting marine bioinvasions. Mar. Pollut. Bull. 128, 41–50. doi: 10.1016/j.marpolbul.2018.01.015
Tait, L., Inglis, G., Seaward, K., Spong, K., and Wilkens, S. (2016). Optimising Settlement Arrays for Surveillance of Non-Indigenous Biofouling Species: Results and Recommendations Following Settlement Array Field Trials. Wellington: Ministry of Primary Industries.
Thompson, J. C., Letissier, M. D. A., Thomason, P. O., and Field, S. N. (2002). Optimising settlement tiles: the effects of surface texture and energy, orientation and deployment duration upon the fouling community. Biofouling 18, 293–304. doi: 10.1080/0892701021000034409
Tilman, D. (1989). “Ecological experimentation: strengths and conceptual problems,” in Long-Term Studies in Ecology, ed. G. E. Likens (New York: Springer), 136–157. doi: 10.1007/978-1-4615-7358-6_6
Todd, C. D., Phelan, P. J. C., Weinmann, B. E., Gude, A. R., Andrews, C., Paterson, D. M., et al. (2006). Improvements to a passive trap for quantifying barnacle larval supply to semi-exposed rocky shores. J. Exp. Mar. Biol. Ecol. 332, 135–150. doi: 10.1016/j.jembe.2005.11.013
Underwood, A. J. (1994). On beyond BACI: sampling designs that might reliably detect environmental disturbances. Ecol. Appl. 4, 3–15. doi: 10.2307/1942110
Underwood, A. J., Chapman, M. G., and Connell, S. D. (2000). Observations in ecology: you can’t make progress on processes without understanding the patterns. J. Exp. Mar. Biol. Ecol. 250, 97–115. doi: 10.1016/S0022-0981(00)00181-7
Underwood, E. C., and Fisher, B. L. (2006). The role of ants in conservation monitoring; If, when, and how. Biol. Conserv. 132, 166–182. doi: 10.1016/j.biocon.2006.03.022
Vance, R. R. (1988). Ecological succession and the climax community on a marine subtidal rock wall. Mar. Ecol. Prog. Ser. 48, 125–136. doi: 10.3354/meps048125
Vaz-Pinto, F., Torrontegi, O., Prestes, A. C., Álvaro, N. V., Neto, A. I., andMartins, G. M. (2014). Invasion success and development of benthic assemblages: effect of timing, duration of submersion and substrate type. Mar. Environ. Res. 94, 72–79. doi: 10.1016/j.marenvres.2013.12.007
Wahl, M. (1989). Marine epibiosis. I. Fouling and antifouling: some basic aspects. Mar. Ecol. Prog. Ser. 58, 175–189. doi: 10.3354/meps058175
Walters, L. J., Miron, G., and Bourget, E. (1999). Endoscopic observations of invertebrate larval substratum exploration and settlement. Mar. Ecol. Prog. Ser. 182, 95–108. doi: 10.3354/meps182095
Wieczorek, S. K., and Todd, C. D. (1997). Inhibition and facilitation of bryozoan and ascidian settlement by natural multi-species biofilms: effects of film age and the roles of active and passive larval attachment. Mar. Biol. 128, 463–473. doi: 10.1007/s002270050113
World Register of Introduced Marine Species [WRiMS] (2021). World Register of Introduced Marine Species. Available Online at: http://www.marinespecies.org/introduced (accessed December 18, 2021).
Yund, P. O., Collins, S., and Johnson, S. L. (2015). Evidence of a native Northwest Atlantic COI haplotype clade in the cryptogenic colonial ascidian Botryllus schlosseri. Biol. Bull. 228, 201–216. doi: 10.1086/BBLv228n3p201
Keywords: early detection, recruitment, temporal patterns, biofouling, accumulation curves, aquatic species, settlement plates
Citation: Ma KCK, McKindsey CW and Johnson LE (2022) Detecting Rare Species With Passive Sampling Tools: Optimizing the Duration and Frequency of Sampling for Benthic Taxa. Front. Mar. Sci. 9:809327. doi: 10.3389/fmars.2022.809327
Received: 04 November 2021; Accepted: 04 February 2022;
Published: 16 March 2022.
Edited by:
Cataldo Pierri, University of Bari Aldo Moro, ItalyReviewed by:
Francisco Barros, Federal University of Bahia, BrazilMarc Rius, Centre for Advanced Studies of Blanes, Spain
Copyright © 2022 Ma, McKindsey and Johnson. This is an open-access article distributed under the terms of the Creative Commons Attribution License (CC BY). The use, distribution or reproduction in other forums is permitted, provided the original author(s) and the copyright owner(s) are credited and that the original publication in this journal is cited, in accordance with accepted academic practice. No use, distribution or reproduction is permitted which does not comply with these terms.
*Correspondence: Kevin C. K. Ma, ay5tYUBydS5hYy56YQ==, a2V2aW5ja21hQGdtYWlsLmNvbQ==
†ORCID: Kevin C. K. Ma, orcid.org/0000-0003-1678-077X; Christopher W. McKindsey, orcid.org/0000-0002-3026-6454; Ladd E. Johnson, orcid.org/0000-0002-0521-1830