- 1Department of Biology, The Pennsylvania State University, University Park, PA, United States
- 2Unidad Académica de Sistemas Arrecifales Puerto Morelos, Instituto de Ciencias del Mar y Limnología, Universidad Nacional Autónoma de México, Puerto Morelos, Mexico
Mass coral bleaching compromises the long-term persistence of coral reefs, yet our current understanding of the different cellular mechanisms leading to the development of a bleached coral is still limited. In this perspective, we mapped the cascade of cellular events and physiological responses of symbiotic corals triggered by thermal stress. Based on existing knowledge, we created an integrated model that describes phenotypic changes induced by sensing mechanisms. Cellular responses are mapped in the context of reactive oxygen species (ROS) production in the algal symbiont chloroplast, followed by signaling to the nucleus and subsequent “leak” to the coral host cell. The starting point is set by ROS production and signaling, which is a day-to-day mechanism by which symbiotic corals maintain homeostasis and acclimate to environmental variation. As stress and acclimation are intimately linked, our model maps coral responses from the initial stimulus in the chloroplast to the complex cascade of events leading to seasonal phenotypic changes (i.e., seasonal acclimation), and if stress progresses, to the downstream coral bleached phenotype (i.e., when the coral’s capacity to acclimate is overwhelmed by heat stress). Placing acclimation, heat stress and bleaching responses in a common ground is a critical step to reduce the source of uncertainty in understanding the coral response to climate change, fundamental for the development of predictive climate models.
Introduction
Mass coral bleaching is a widespread and conspicuous aspect of climate change in coral reefs. Thermal anomalies (+ 1.5–2°C above the long-term average of the warmest month) impose heat stress levels above the limits of tolerance of the symbiotic relationship, inducing the collapse of the algal and coral functional association (Hoegh-Guldberg, 1999). As a result, coral reefs are projected to decline by a further 70–90% (> 99% at 2°C) (Hoegh-Guldberg, 1999; IPCC, 2019). Driven by the association between coral bleaching and coral reef persistence, intense research efforts have been directed to understand coral responses to heat-stress, with a large body of evidence directing to the impairment of algal symbiont photosynthesis and subsequent host programmed cell death (PCD) [reviewed in Weis (2008)]. However, understanding the cascade of events that leads to the development of the bleached coral phenotype is still very limited.
Advances in our understanding of the inter-partner communication during thermal stress responses place reactive oxygen species (ROS) at the center of the physiological disturbance (Weis, 2008; Baird et al., 2009). In homeostasis, chloroplasts, peroxisomes, and mitochondria are continuously producing ROS, maintaining radicals at relatively low levels (Chan et al., 2010). When thermal stress is under the limits of tolerance for the symbiosis, increased ROS levels are non-deleterious, and corals adjust to alternative homeostatic states depending on the overall cellular quantity of ROS. When thermal stress is outside the limits of tolerance, deleterious oxidative stress triggers coral bleaching, suggested by the strong correlation between induction of ROS and the accumulation of oxidative damage products in both the algal symbiont and host (Brown et al., 2002; Downs et al., 2002; Dias et al., 2019). Long-standing attributions of “oxidative-damage” associated with ROS are challenged by the realization that they are secondary intracellular messengers, essential for the homeostasis of organisms (Chan et al., 2010; Acín-Pérez et al., 2014). This results in the difficult task of separating coral light acclimation and thermal acclimation with the development of the bleached phenotype.
Homeostasis is accomplished through a complex network of interacting genes and biochemical pathways, where different stressors can affect common sets of genes or gene families, which is consistent with the observation that certain overlapped pathways are involved in coral physiological responses to various stressors. Although key phenotypic descriptions may solve this limitation, there no agreement on the definition of the bleached coral phenotype. Large losses of pigments and/or symbionts are insufficient in identifying the bleached condition, and alternative descriptors such as the cessation of photosynthetic activity and large alterations to optical traits have been found to be robust proxies (Scheufen et al., 2017a,b). In this perspective, we used the optical trait a*Chla (holobiont light absorption efficiency) to quantify the seasonal variability of the acclimatory coral condition and to identify the bleached phenotype (Scheufen et al., 2017b). We conceptually delineate the possible sequence of events linking early sensing of thermal stress in the algal symbiont chloroplast, with host cascade responses leading to the bleached phenotype.
Limits of Seasonal Acclimation
Solar radiation is the main source of energy for all photosynthetic primary producers, as well as for symbiotic corals, an evolutionary consequence of a mutualistic association with intracellular photosynthetic algae. Translocation of algal photosynthates satisfies the majority of the carbon metabolic requirements of the coral host and is also responsible for the high rates of calcification that allow for the creation of tropical coral reefs. A prominent emergent property of this mutualism is the optimization of light absorption with a minimum investment of resources, making symbiotic corals one of the most efficient light collectors currently known (Enríquez et al., 2005, 2017; Terán et al., 2010). Given the metabolic integration of host and symbiont, acclimation to light or photoacclimation is a time-dependent process that occur in all cellular compartments. At the symbiont chloroplast level, adjustments in the photosynthetic apparatus (Iglesias-Prieto and Trench, 1997; Gorbunov et al., 2001) operate on the scale of seconds to days. At the coral colony level, changes in tissue pigmentation (Fitt et al., 2000; Scheufen et al., 2017a), relative abundance of different algal symbiont species (Rowan and Knowlton, 1995; Kemp et al., 2015; Hoadley et al., 2019) and morphological and skeletal changes (Gladfelter, 1984; Todd, 2008; Malik et al., 2021) operate over periods of weeks to months. Overall, responses involve changes in host metabolism (Lohr et al., 2019) and gene expression (Malik et al., 2021). Distinctly, a main feature of symbiotic coral photoacclimation to light is to ensure optimal photosynthetic activity that guarantees enough translocation of photosynthates to the host. This places the photosynthetic activity of symbionts in hospite at the center of cellular processes that regulate coral phenotypes (Scheufen et al., 2017a).
Seasonal changes in photosynthetic active radiation (PAR) and sea surface temperature (SST) drive seasonal acclimation in symbiotic corals, resulting in winter and summer phenotypes (Brown et al., 1999; Fagoonee et al., 1999; Carricart-Ganivet et al., 2000; Fitt et al., 2000; Warner et al., 2002; Scheufen et al., 2017a). Winter phenotypes are generally characterized by higher pigmentation and quantum yield of Chlorophyll a (Chla) fluorescence in hospite (Fv/Fm). In contrast, summer phenotypes are more efficient light collectors (Figure 1A), characterized by decreased symbiont densities, Chla, Fv/Fm, and increased photosynthetic and calcification rates. With the overall decrease of Chla density in the tissue, corals increase exponentially the absorption efficiency of their pigments (a*Chla) due to multiple scattering processes in the coral skeleton (Enríquez et al., 2005). This results in a distinguishable winter-to-summer shift of the light absorption efficiency of the holobiont (a*Chla) (Figure 1A). Multiple scattering of light on the coral skeleton produces diffuse light in the tissue, reducing pigment self-shading and increasing the probability of light absorption for the symbionts in hospite (Enríquez et al., 2005). In summer phenotypes, these processes allow significant enhancements of the local irradiance within the tissue for a smaller symbiont population, setting the limits of the seasonal variation with this reduction (Enríquez et al., 2005) (Figure 1A).
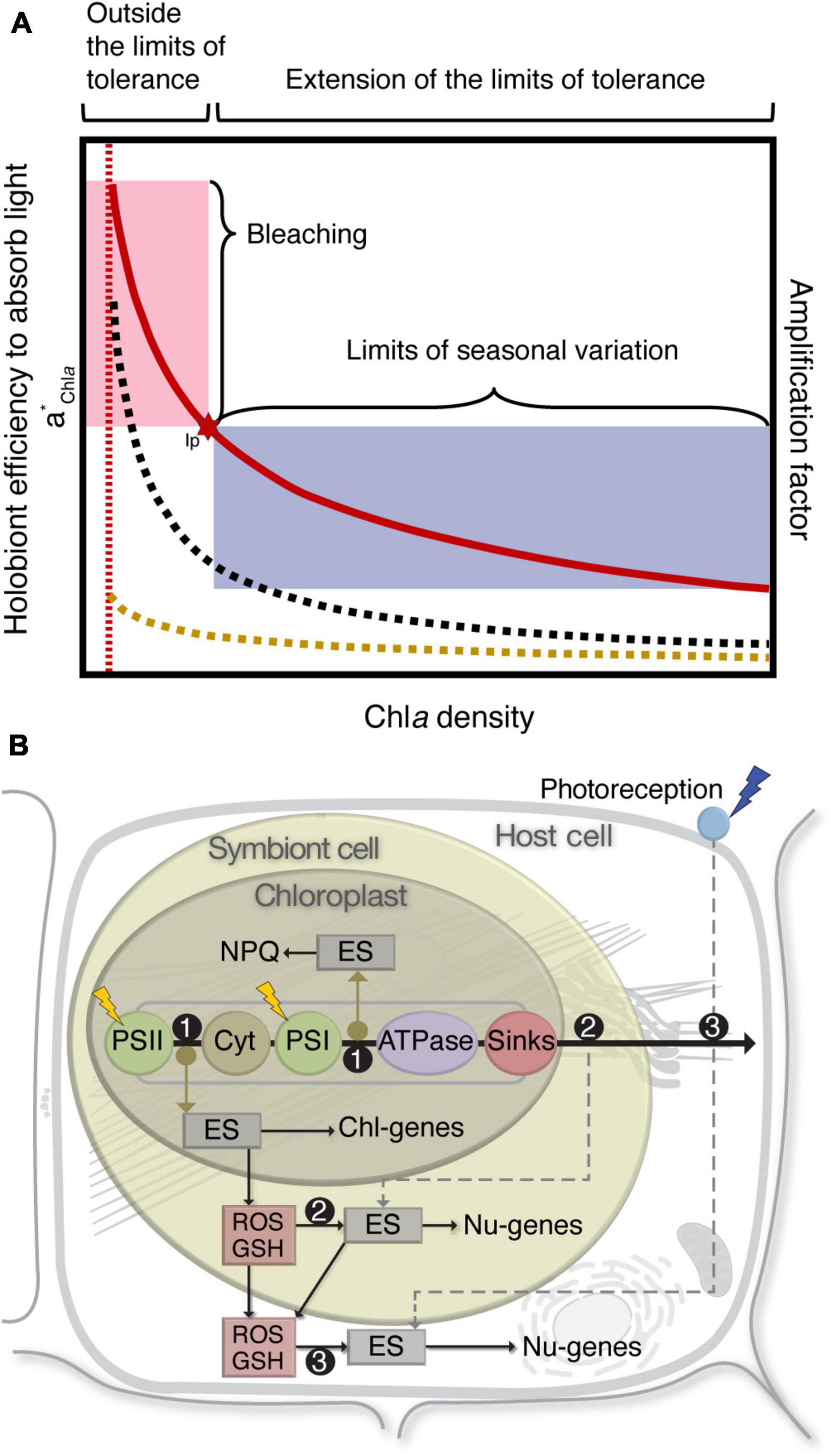
Figure 1. Seasonal phenotypic variation and cellular communication in symbiotic corals. (A) Seasonal changes in holobiont light absorption efficiency (quantified by means of the specific absorption coefficient of Chlorophyll a, a*Chla) as a function of pigment (Chla) density in corals. Lines show the comparative increase in light absorption efficiency of freshly isolated symbiont cells (yellow dashed line), absorption by intact coral laminae (black dashed line), and the estimated enhanced factor of light absorption resulting from the comparison of both optical measurements (red line). This amplification or “enhancement factor” is the consequence of the capacity of the coral skeleton to induce multiple scattering of light. Inflection point (Ip) of a*Chla sets a limit for the variation of the coral photoacclimatory response (shaded blue area) and the development of the bleached phenotype (shaded red area) [modified from Enríquez et al. (2005)]. (B) Responses in the different cellular compartments of the holosymbiont: (1) Chloroplasts perceive the environmental change as “light stress,” the increase in electron transfer produces over-reduction in the PQ-pool and a ΔpH + (with associated NPQ) across the thylakoid membrane; the net result is the decrease in Fv/Fm and the generation of an alternative homeostatic state. (2) Symbiont cells perceive the change as “oxidative stress,” energy sensors (ES) activate changes in gene expression; the net result is the decrease in symbiont pigmentation and an alternative homeostatic state. (3) Host cells perceive the change as “thermal stress,” energy sensors activate changes in gene expression, and immune responses facilitate symbiont trafficking to adjust symbiont cell density in the host tissue; the net result is an alternative homeostatic state that sets the limits of acclimation. (Chl-genes) Chloroplast genes, (Nu-genes) Nuclear genes, (GSH) Glutathione.
The well-balanced winter-to-summer oscillation is disrupted during thermal anomalies (Hoegh-Guldberg, 1999). Under these conditions, the symbiosis falls into a positive feed-back loop, with the loss of symbiont cells enhancing the local irradiance within the tissue, inducing further losses of symbiont cells. Increase in magnitude of the environmental stress accelerates this symbiont loss and bleaching (Swain et al., 2016), which is evident in an a*Chla inflection point (Figure 1A). This pushes the symbiosis outside the limits of tolerance, compromising homeostasis, and crosses the “tipping point” toward the bleached phenotype. The severe loss of the symbiont population results in a no longer functional symbiosis. If the deleterious stress is removed in time, the symbiosis may be functionally re-established, but if the stress persists, a further “tipping point” will lead to mass mortality accompanied with an ecosystem collapse.
Cascade of Cellular Events
Homeostasis establishment associated with a new acclimatory state is not the result of a single physiological process, but rather the result of many biological processes that the coral integrates over time. Compartmentalization of the eukaryotic cell into different organelles presents paradigms in the regulation of homeostasis compared to prokaryotes, where all the main cellular processes happen within the same compartment (Chan et al., 2010). Compartmentalization is especially relevant for symbiotic corals, where additional cellular compartments are present (chloroplasts, algal symbiont cells). Descriptions of changes in coral metabolic activity over the past few decades has led to the general agreement that crosstalk between the chloroplast, the symbiont cell and the host occur to coordinate holosymbiont homeostasis [reviewed in Davy et al. (2012)]. In this context, ROS is the key player as a secondary intracellular messenger among the different cellular compartments and its production is required for cellular signaling to induce different cellular adjustments (Cruz De Carvalho, 2008). Reactive oxygen species levels are kept under control by an orchestrated enzymatic and antioxidant efficient system that sets the redox-status of the cell. Its signaling to the nucleus turns on such defense mechanisms to minimize its deleterious effects (Erickson et al., 2015). Moreover, extra-mitochondrial source of ROS can trigger metabolic cellular adjustments in the mitochondria (Acín-Pérez et al., 2014), fundamental in any acclimatory response to temperature variation.
The starting point for the transition to a summer phenotype is in the chloroplast (Figure 1B). The documented coral response suggests that this environmental change is perceived as “light stress” resulting in increased light absorption and decreased Fv/Fm. The increased electron transport via a series of electron carriers produces an over reduction of the plastoquinone pool (PQ-pool) and expands the pH gradient (ΔpH +) across the membrane, producing more adenosine triphosphate (ATP). ΔpH + influences thermal dissipation via non-photochemical quenching (NPQ), which provides a mechanism that protects the photosynthetic apparatus from excess excitation energy (EEE), and has been shown to be associated with the conversion of diadinoxanthin to diatoxanthin (xanthophyll cycling) (Brown, 1997). With the change in electron transfer, ROS can fluctuate in specific concentrations due to phase transitions of thylakoid membrane lipids (Iglesias-Prieto et al., 1992; Tchernov et al., 2004), thermal inactivation of both photosystem II (PSII) (Iglesias-Prieto, 1996; Warner et al., 1996, 1999; Iglesias-Prieto and Trench, 1997) and Ribulose-1,5-bisphosphate carboxylase-oxygenase (RuBisCO) (Jones et al., 1998), damage of repair mechanisms in PSII (Warner et al., 1999; Takahashi et al., 2004), and changes in inorganic carbon concentrations (sink limitation) (Buxton et al., 2009). This alters chloroplast gene expression and ROS “leak” to the symbiont cytoplasm, functioning as components of environmental-signaling pathways (Chan et al., 2010). The modulation of this response can be considered as the signal for corals to establish a new homeostatic state, which is reversible upon removal of the external stress.
As SST and PAR continue to increase toward the summer, symbiont cells perceive in hospite the environmental change as increasing levels of “oxidative stress” and increases in excitation pressure in their photosynthetic apparatus. Under these conditions, an energy sensor from the cytochrome b6f (Cytb6f) activates changes in nuclear gene expression to modify light-harvesting antenna size (Figure 1B) (Gierz et al., 2016, 2017). Light and thermal stress are associated with the loss and inhibition of de novo synthesis of the major light-harvesting complexes (Dove et al., 2006; Takahashi et al., 2008). Moreover, NPQ-associated xanthophyll cycling decreases photon flux to PSII reaction centers, thus preventing over reduction of the PQ-pool by reducing the functional absorption cross-section of PSII reaction centers (σPSII). This new homeostatic state with decreased symbiont pigmentation is reversible upon removal of the external stress.
Later in the summer, with continuation of the environmental change, levels of oxidative stress continue to increase, and ROS can activate changes in nuclear gene expression to induce PCD in the host cell (and/or symbiont cell), reducing the number of symbionts in the host tissue (Figure 1B). Although the exact mechanism by which symbiont cell numbers are controlled is not known, immune responses play a key role in symbiont trafficking (Davy et al., 2012). Moreover, it is recognized that cellular mechanisms of symbiont loss involve symbiont degradation, exocytosis, host cell detachment (with the intact symbiont), host cell apoptosis, autophagy, and/or necrosis (Gates et al., 1992; Dunn et al., 2002, 2007; Weis, 2008; Downs et al., 2009; Paxton et al., 2013). The decrease of overall Chla density in the host tissue, via reduction in Chla per symbiont cell as well as symbiont cell loss, results in the development of the summer phenotype at the end of the warmest period. This new homeostatic state with decreased Fv/Fm, symbiont densities, and host tissue pigmentation is the result of ROS-mediated changes in the different cellular compartments of a mechanism tightly controlled by oxidant scavengers providing the flexibility to acclimate to PAR and SST. The fact that the summer phenotype presents higher coral performance (photosynthesis and calcification rates) (Scheufen et al., 2017a), demonstrates a successful acclimation through a cascade of cellular events previously described. This state is reversible when environmental conditions change, and the coral returns to the previous homeostatic state. If temperature continues to increase into a prolonged thermal anomaly over the symbiosis limits of tolerance, all these homeostatic mechanisms can be overwhelmed, and a bleached coral phenotype is developed.
The Bleached Coral Phenotype
Prolonged thermal anomalies (+ 1.5–2°C above the long-term average of the warmest month) drive the symbiosis outside its limits of tolerance. The thermal stress is exacerbated by the enhanced local illumination of the symbionts within the tissue, a physical change that explains the severe loss of symbionts during thermal stress. The extreme environmental condition accelerates the production of ROS, eventually overwhelming antioxidant responses. This extremely high reactivity facilitates extensive cellular damage, including cellular membrane lipid peroxidation (LPO), DNA degradation, and protein denaturation (Weis, 2008; Roth, 2014). Although carbon fixation may continue via the Calvin cycle, net translocation is significantly reduced (Hillyer et al., 2017).
In this view, a plausible mechanistic road map recognizes acclimatory states before the development of a bleached coral phenotype. When exposed to thermal anomalies above the limits of tolerance, several attempts to acclimate will take place within the chloroplast, the symbiont cell (by oxidative stress), and the host cell (with PCD), with subsequent failure to maintain a functional symbiosis. With the accumulation of deleterious oxidative damage, most symbiotic corals will eventually develop a bleached phenotype through the same acclimatory attempts. However, differences in coral susceptibility arise when we disentangle the acclimatory state prior to the exposure to stress and the susceptibility of the resident symbiont species. Understanding these differences will allow for the determination of a starting point in the road map that will determine the steps involved in developing the bleached phenotype.
The last few decades have seen many new and exciting findings paving the way to a better understanding of coral stress responses [reviewed in Putnam (2021)]. Some of the major areas of focus include genomics, symbiont responses in cultured conditions, acclimation in depth gradients, and coral bleaching. These conditions are associated with the need to partition coral light acclimation, thermal acclimation, and the definition of a bleached coral phenotype. However, there are scarce approaches to delineate these differences, even when environmental stress is a major factor changing homeostatic states and coral acclimation. ROS are produced during normal cell metabolism and their regulation is a common cellular event accompanied with oxidative damage (Dat et al., 2000). This mechanism triggers different homeostatic states in the coral (Figure 2), with initial stimuli in the chloroplast, evident in the variation of excitation pressure and Fv/Fm of symbionts in hospite. If acclimation is not successful, the next step may be controlled by ROS levels regulating expression of the light-harvesting complexes (Gierz et al., 2016, 2017). If acclimation is still not successful and the production of ROS continues, it may trigger the regulation of symbiont cell densities in the host tissue, similar to seasonal variation (Figure 2). In this context, a continuous reduction in symbiont cell numbers may lead to the development of the bleached coral phenotype.
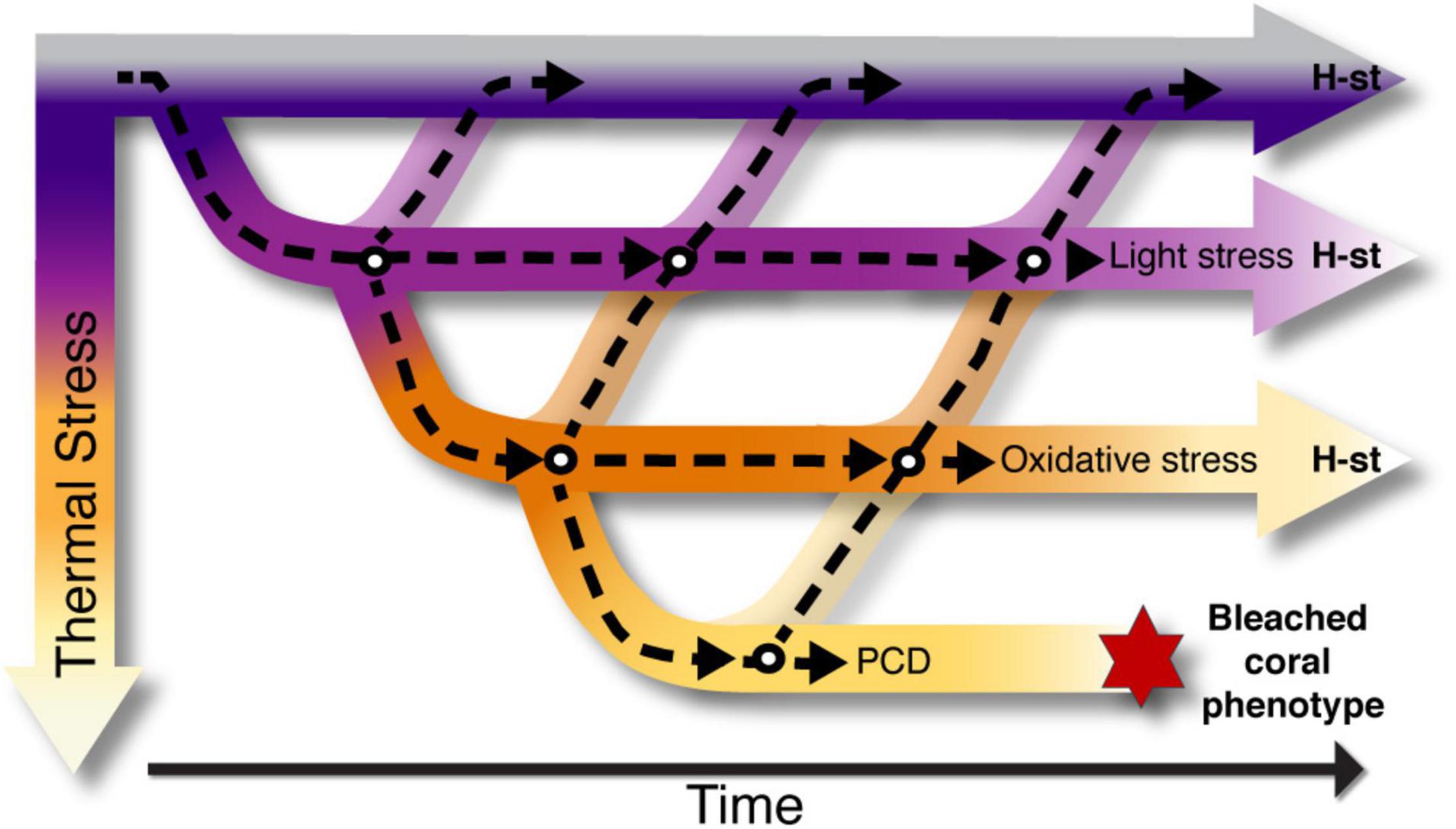
Figure 2. Road map of homeostatic states leading to the bleached coral phenotype. Light and thermal responses triggered by ROS as a secondary cellular messenger, are considered as the signal for corals to initiate processes required for the establishment of new homeostatic states (H-st). Within the road map, several phenotypic steps take place (white circles) to achieve the new H-st. These states are time dependent and take place in the different compartments of the cell: in the chloroplast (light stress), symbiont cell (oxidative stress) and host cell (oxidative stress and Program Cell Death). All H-st are reversible when environmental conditions change, and the coral may return to previous homeostatic states. When thermal stress increases above the limits of tolerance of the symbiosis, the net accumulation of oxidative damage is deleterious and corals are unable to adjust by establishing a new homeostatic state, developing the bleached phenotype.
The different roles of ROS, both in acclimation and in the development of a bleached phenotype, results in a duality that drives distinct processes in the symbiosis. Ecological studies may track seasonal and bleaching dynamics at the ecosystem level with non-invasive techniques. However, to disentangle bleaching mechanisms, experimental approaches are more suitable. In this case, commonly used parameters to quantify coral bleaching such as, decrease in Fv/Fm, decrease in pigmentation (merely chla density and color charts), increase in ROS abundance, are insufficient to identify a bleached phenotype and fully describe the physiological condition of the coral. It is imperative to account for the loss of symbiont cells in the host tissue, to distinguish between acclimatory conditions (limits of acclimation) and bleached coral conditions. This, to reduce the source of uncertainties in the understanding of coral responses to climate change, fundamental for the development of predictive climate models.
Data Availability Statement
The original contributions presented in the study are included in the article/supplementary material, further inquiries can be directed to the corresponding authors.
Author Contributions
KG-C and RI-P wrote the manuscript. SE critical conceptual inputs. All authors contributed to manuscript revision, read, and approved the submitted version.
Funding
Projects to complement gaps in knowledge has received funding from The Pennsylvania State University Start-up awarded to RI-P.
Conflict of Interest
The authors declare that the research was conducted in the absence of any commercial or financial relationships that could be construed as a potential conflict of interest.
Publisher’s Note
All claims expressed in this article are solely those of the authors and do not necessarily represent those of their affiliated organizations, or those of the publisher, the editors and the reviewers. Any product that may be evaluated in this article, or claim that may be made by its manufacturer, is not guaranteed or endorsed by the publisher.
References
Acín-Pérez, R., Carrascoso, I., Baixauli, F., Roche-Molina, M., Latorre-Pellicer, A., Fernández-Silva, P., et al. (2014). ROS-triggered phosphorylation of complex II by Fgr kinase regulates cellular adaptation to fuel use. Cell Metab. 19, 1020–1033. doi: 10.1016/j.cmet.2014.04.015
Baird, A. H., Bhagooli, R., Ralph, P. J., and Takahashi, S. (2009). Coral bleaching: the role of the host. Trends Ecol. Evol. 24, 16–20.
Brown, B. E., Downs, C. A., Dunne, R. P., and Gibb, S. W. (2002). Exploring the basis of thermotolerance in the reef coral Goniastrea aspera. Mar. Ecol. Prog. Ser. 242, 119–129.
Brown, B. E., Dunne, R. P., Ambarsari, I., and Tissier, M. D. A. Le, and Satapoomin, U. (1999). Seasonal fluctuations in environmental factors and variations in symbiotic algae and chlorophyll pigments in four Indo-Pacific coral species. Mar. Ecol. Prog. Ser. 191, 53–69. doi: 10.3354/meps191053
Buxton, L., Badger, M., and Ralph, P. (2009). Effects of moderate heat stress and dissolved inorganic carbon concentration on photosynthesis and respiration of symbiodinium sp. (dinophyceae) in culture and in symbiosis. J. Phycol. 45, 357–365. doi: 10.1111/j.1529-8817.2009.00659.x
Carricart-Ganivet, J. P., Beltrán-Torres, A. U., Merino, M., and Ruiz-Zárate, M. A. (2000). Skeletal extension, density and calcification rate of the reef building coral Montastraea annularis (Ellis and Solander) in the Mexican Caribbean. Bull. Mar. Sci. 66, 215–224.
Chan, K. X., Crisp, P. A., Estavillo, G. M., and Pogson, B. J. (2010). Chloroplast-to-nucleus communication: Current knowledge, experimental strategies and relationship to drought stress signaling. Plant Signal. Behav. 5, 1575–1582. doi: 10.4161/psb.5.12.13758
Cruz De Carvalho, M. H. (2008). Drought stress and reactive oxygen species: Production, scavenging and signaling. Plant Signal. Behav. 3, 156–165. doi: 10.4161/psb.3.3.5536
Dat, J., Vandenabeele, S., Vranová, E., Van Montagu, M., Inzé, D., and Van Breusegem, F. (2000). Dual action of the active oxygen species during plant stress responses. Cell. Mol. Life Sci. 57, 779–795. doi: 10.1007/s000180050041
Davy, S. K., Allemand, D., and Weis, V. M. (2012). Cell Biology of Cnidarian-Dinoflagellate Symbiosis. Microbiol. Mol. Biol. Rev. 76, 229–261. doi: 10.1128/MMBR.05014-11
Dias, M., Ferreira, A., Gouveia, R., Madeira, C., Jogee, N., Cabral, H., et al. (2019). Long-term exposure to increasing temperatures on scleractinian coral fragments reveals oxidative stress. Mar. Environ. Res. 150:104758. doi: 10.1016/j.marenvres.2019.104758
Dove, S., Ortiz, J. C., Enríquez, S., Fine, M., Fisher, P., Iglesias-Prieto, R., et al. (2006). Response of holosymbiont pigments from the scleractinian coral Montipora monasteriata to short-term heat stress. Limnol. Oceanogr. 51, 1149–1158.
Downs, C. A., Fauth, J. E., Halas, J. C., Dustan, P., Bemiss, J., and Woodley, C. M. (2002). Oxidative stress and seasonal coral bleaching. Free Radic. Biol. Med. 33, 533–543. doi: 10.1016/s0891-5849(02)00907-3
Downs, C. A., Kramarsky-Winter, E., Martinez, J., Kushmaro, A., Woodley, C. M., Loya, Y., et al. (2009). Symbiophagy as a cellular mechanism for coral bleaching. Autophagy. 5, 211–216. doi: 10.4161/auto.5.2.7405
Dunn, S. R., Bythell, J. C., Le Tissier, M. D. A., Burnett, W. J., and Thomason, J. C. (2002). Programmed cell death and cell necrosis activity during hyperthermic stress-induced bleaching of the symbiotic sea anemone Aiptasia sp. J. Exp. Mar. Bio. Ecol. 272, 29–53. doi: 10.1016/s0022-0981(02)00036-9
Dunn, S. R., Schnitzler, C. E., and Weis, V. M. (2007). Apoptosis and autophagy as mechanisms of dinoflagellate symbiont release during cnidarian bleaching: Every which way you lose. Proc. R. Soc. B Biol. Sci. 274, 3079–3085. doi: 10.1098/rspb.2007.0711
Enríquez, S., Méndez, E. R., Hoegh-Guldberg, O., and Iglesias-Prieto, R. (2017). Key functional role of the optical properties of coral skeletons in coral ecology and evolution. Proc. R. Soc. B Biol. Sci. 284:20161667. doi: 10.1098/rspb.2016.1667
Enríquez, S., Méndez, E. R., and Iglesias-Prieto, R. (2005). Multiple scattering on coral skeletons enhances light absorption by symbiotic algae. Limnol. Oceanogr. 50, 1025–1032. doi: 10.1364/AO.49.005032
Erickson, E., Wakao, S., and Niyogi, K. K. (2015). Light stress and photoprotection in Chlamydomonas reinhardtii. Plant J. 82, 449–465. doi: 10.1111/tpj.12825
Fagoonee, I., Wilson, H. B., Hassell, M. P., and Turner, J. R. (1999). The Dynamics of Zooxanthellae Populations: A Long-Term Study in the Field. New Ser. 283, 843–845. doi: 10.1126/science.283.5403.843
Fitt, W. K., McFarland, F. K., Warner, M. E., and Chilcoat, G. C. (2000). Seasonal patterns of tissue biomass and densities of symbiotic dinoflagellates in reef corals and relation to coral bleaching. Limnol. Oceanogr. 45, 677–685. doi: 10.1371/journal.pone.0029535
Gates, R. D., Baghdasarian, G., and Muscatine, L. (1992). Temperature stress causes host cell detachment in symbiotic cnidarians: implications for coral bleaching. Biol. Bull. 182, 324–332. doi: 10.2307/1542252
Gierz, S. L., Forêt, S., and Leggat, W. (2017). Transcriptomic analysis of thermally stressed Symbiodinium reveals differential expression of stress and metabolism genes. Front. Plant Sci 8:271. doi: 10.3389/fpls.2017.00271
Gierz, S. L., Gordon, B. R., and Leggat, W. (2016). Integral Light-Harvesting Complex Expression in Symbiodinium Within the Coral Acropora aspera under Thermal Stress. Sci. Rep. 6:25081. doi: 10.1038/srep25081
Gladfelter, E. H. (1984). Skeletal development in Acropora cervicornis - III. A comparison of monthly rates of linear extension and calcium carbonate accretion measured over a year. Coral Reefs. 3, 51–57. doi: 10.1007/bf00306140
Gorbunov, M. Y., Kolber, Z. S., and Lesser, M. P. (2001). Photosynthesis and photoprotection in symbiotic corals. Limnol. Oceanogr. 46, 75–85. doi: 10.4319/lo.2001.46.1.0075
Hillyer, K. E., Dias, D. A., Lutz, A., Roessner, U., and Davy, S. K. (2017). Mapping carbon fate during bleaching in a model cnidarian symbiosis: the application of 13C metabolomics. New Phytol. 214, 1551–1562. doi: 10.1111/nph.14515
Hoadley, K. D., Lewis, A. M., Wham, D. C., Pettay, D. T., Grasso, C., Smith, R., et al. (2019). Host–symbiont combinations dictate the photo-physiological response of reef-building corals to thermal stress. Sci. Rep. 9, 1–15. doi: 10.1038/s41598-019-46412-4
Hoegh-Guldberg, O. (1999). Climate change, coral bleaching and the future of the world’s coral reefs. Mar. Freshw. Res. 50, 839–866.
Iglesias-Prieto, R. (1996). Temperature-dependent inactivation of photosystem II in symbiotic dinoflagellates. Proc 8th Int Coral Reef Symp. Panama 2, 1313–1318.
Iglesias-Prieto, R., Matta, J. L., Robins, W. A., and Trench, R. K. (1992). Photosynthetic response to elevated temperature in the symbiotic dinoflagellate Symbiodinium microadriaticum in culture. Proc. Natl. Acad. Sci. U.S.A. 89, 10302–10305. doi: 10.1073/pnas.89.21.10302
Iglesias-Prieto, R., and Trench, R. K. (1997). Acclimation and adaptation to irradiance in symbiotic dinoflagellates. II. Response of chlorophyll-protein complexes to different photon-flux densities. Mar. Biol. 130, 23–33. doi: 10.1007/s002270050221
IPCC. (2019). “Summary for Policymakers,” in IPCC Special Report on the Ocean and Cryosphere in a Changing Climate, eds H.-O. Pörtner, D. C. Roberts, and V. Masson (Switzerland: IPCC).
Jones, R. J., Hoegh-Guldberg, O., Larkum, A. W. D., and Schreiber, U. (1998). Temperature-induced bleaching of corals begins with impairment of the CO2 fixation mechanism in zooxanthellae. Plant Cell Environ. 21, 1219–1230. doi: 10.1046/j.1365-3040.1998.00345.x
Kemp, D. W., Thornhill, D. J., Rotjan, R. D., Iglesias-Prieto, R., Fitt, W. K., and Schmidt, G. W. (2015). Spatially distinct and regionally endemic Symbiodinium assemblages in the threatened Caribbean reef-building coral Orbicella faveolata. Coral Reefs. 34, 535–547. doi: 10.1007/s00338-015-1277-z
Lohr, K. E., Camp, E. F., Kuzhiumparambil, U., Lutz, A., Leggat, W., Patterson, J. T., et al. (2019). Resolving coral photoacclimation dynamics through coupled photophysiological and metabolomic profiling. J. Exp. Biol. 222:jeb195982. doi: 10.1242/jeb.195982
Malik, A., Einbinder, S., Martinez, S., Tchernov, D., Haviv, S., Almuly, R., et al. (2021). Molecular and skeletal fingerprints of scleractinian coral biomineralization: From the sea surface to mesophotic depths. Acta Biomater. 120, 263–276. doi: 10.1016/j.actbio.2020.01.010
Paxton, C. W., Davy, S. K., and Weis, V. M. (2013). Stress and death of cnidarian host cells play a role in cnidarian bleaching. J. Exp. Biol. 216, 2813–2820. doi: 10.1242/jeb.087858
Putnam, H. M. (2021). Avenues of reef-building coral acclimatization in response to rapid environmental change. J. Exp. Biol. 224:jeb239319. doi: 10.1242/jeb.239319
Roth, M. S. (2014). The engine of the reef: Photobiology of the coral-algal symbiosis. Front. Microbiol. 5:422. doi: 10.3389/fmicb.2014.00422
Rowan, B., and Knowlton, N. (1995). Intraspecific diversity and ecological zonation in coral-algal symbiosis. Proc. Natl. Acad. Sci.U.S.A. 92, 2850–2853. doi: 10.1073/pnas.92.7.2850
Scheufen, T., Iglesias-prieto, R., and Enríquez, S. (2017a). Changes in the Number of Symbionts and Symbiodinium Cell Pigmentation Modulate Differentially Coral Light Absorption and Photosynthetic Performance. Front. Mar. Sci. 4:309. doi: 10.3389/fmars.2017.00309/full
Scheufen, T., Kraemer, W. E., Iglesias-Prieto, R., and Enriquez, S. (2017b). Seasonal variation modulates coral sensibility to heat-stress and explains annual changes in coral productivity. Sci. Rep 7:4937. doi: 10.1038/s41598-017-04927-8
Swain, T. D., DuBois, E., Gomes, A., Stoyneva, V. P., Radosevich, A. J., Henss, J., et al. (2016). Skeletal light-scattering accelerates bleaching response in reef-building corals. BMC Ecol 16:10. doi: 10.1186/s12898-016-0061-4
Takahashi, S., Nakamura, T., Sakamizu, M., Van Woesik, R., and Yamasaki, H. (2004). Repair Machinery of Symbiotic Photosynthesis as the Primary Target of Heat Stress for Reef-Building Corals. Plant Cell Physiol. 45, 251–255. doi: 10.1093/pcp/pch028
Takahashi, S., Whitney, S., Itoh, S., Maruyama, T., and Badger, M. (2008). Heat stress causes inhibition of the de novo synthesis of antenna proteins and photobleaching in cultured Symbiodinium. Proc. Natl. Acad. Sci.U.S.A. 105, 4203–4208. doi: 10.1073/pnas.0708554105
Tchernov, D., Gorbunov, M. Y., Vargas, C. De, Yadav, S. N., Milligant, A. J., Häggblom, M., et al. (2004). Membrane lipids of symbiotic algae are diagnostic of sensitivity to thermal bleaching in corals. Proc. Natl. Acad. Sci.U.S.A. 101, 13531–13535. doi: 10.1073/pnas.0402907101
Terán, E., Méndez, E. R., Enríquez, S., and Iglesias-Prieto, R. (2010). Multiple light scattering and absorption in reef-building corals. Appl. Opt. 49, 5032–5042.
Warner, M. E., Chilcoat, G. C., McFarland, F. K., and Fitt, W. K. (2002). Seasonal fluctuations in the photosynthetic capacity of photosystem II in symbiotic dinoflagellates in the Caribbean reef-building coral Montastraea. Mar. Biol. 141, 31–38. doi: 10.1007/s00227-002-0807-8
Warner, M. E., Fitt, W. K., and Schmidt, G. W. (1999). Damage to photosystem II in symbiotic dinoflagellates: a determinant of coral bleaching. Proc. Natl. Acad. Sci.U.S.A. 96, 8007–8012. doi: 10.1073/pnas.96.14.8007
Warner, W. K., Fitt, W. K., and Schmidt, G. W. (1996). The effects of elevated temperature on the photosynthetic efficiency of zooxantheliae in hospiteUom four different species of reef coral: a novel approach. Plant Cell Environ. 19, 291–299.
Keywords: oxidative stress, seasonal phenotype, coral bleaching, ROS, bleached coral phenotype
Citation: Gómez-Campo K, Enríquez S and Iglesias-Prieto R (2022) A Road Map for the Development of the Bleached Coral Phenotype. Front. Mar. Sci. 9:806491. doi: 10.3389/fmars.2022.806491
Received: 31 October 2021; Accepted: 25 January 2022;
Published: 16 March 2022.
Edited by:
Davide Seveso, University of Milano-Bicocca, ItalyReviewed by:
Joshua Patterson, University of Florida, United StatesGretchen Goodbody-Gringley, Central Caribbean Marine Institute, Cayman Islands
Copyright © 2022 Gómez-Campo, Enríquez and Iglesias-Prieto. This is an open-access article distributed under the terms of the Creative Commons Attribution License (CC BY). The use, distribution or reproduction in other forums is permitted, provided the original author(s) and the copyright owner(s) are credited and that the original publication in this journal is cited, in accordance with accepted academic practice. No use, distribution or reproduction is permitted which does not comply with these terms.
*Correspondence: Kelly Gómez-Campo, a2pnMjdAcHN1LmVkdQ==; Roberto Iglesias-Prieto, cnppM0Bwc3UuZWR1