- 1School of Earth and Environmental Sciences/Research Institute of Oceanography, Seoul National University, Seoul, Republic of Korea
- 2East Sea Fisheries Research Institute, National Institute of Fisheries Science, Gangneung, Republic of Korea
- 3Department of Marine Bioscience, Gangneung-Wonju National University, Gangneung, Republic of Korea
- 4Department of Mathematics, Pusan National University, Busan, Republic of Korea
- 5Ocean Research Division, Korea Hydrographic and Oceanographic Agency, Busan, Republic of Korea
Walleye pollock (Gadus chalcogramma) caught in the Korean fishing area dramatically decreased in the late 1980s. To investigate the potential impact of the late 1980s climate regime shift on the collapse of the pollock catch, we developed a three-dimensional hydrodynamic model with data assimilation and a particle tracking model. Data-assimilated reanalysis showed that sea surface temperature increased by approximately 2°C in the spawning area of pollock in the late 1980s. The suitable spawning area in the East Korean Bay decreased due to warming in the late 1980s. Spawned eggs of walleye pollock were tracked using a particle tracking model for 30 days in January and February during 1983–1992. The number of individuals transported to the nursery within the Korean fishing area from the spawning area was reduced by 74% in the late 1980s. The intensified East Korean Warm Current (EKWC) could be responsible for the decreased number of individuals transported to the southern area in the late 1980s. Warming in the Korean fishing area could also cause a decrease in pollock. These oceanic changes might be linked to climate regime shifts in the late 1980s. The warming regime with positive Arctic Oscillation and weakened monsoon intensified the northward flow of the EKWC and accelerated the warming of the spawning and fishing areas in the late 1980s.
Introduction
Walleye pollock, Gadus chalcogramma, which is a semi-demersal cold water species, is distributed in the coastal areas of the North Pacific Ocean from the East Japan Sea (EJS) to the central California coast (Bailey et al., 1999a; Makino et al., 2014; Bang et al., 2018; Park et al., 2018). The annual catch of walleye pollock in 2018 was 3.4 million metric tons, making it the second-ranked species in the global catches of fishes (FAO, 2020).
Walleye pollock, which maintained high biomass in the EJS until the 1980s, constituted the most important fish species culturally and economically in Korea as well as in the countries around the EJS (Beamish and McFarlane, 1995; Kang, 2009; Lee et al., 2019). It has been known that there are four major walleye pollock spawning grounds in the EJS: the Tartar Strait and Peter the Great Gulf off Russia, western coastal areas, off Hokkaido and Honshu, Japan, and the East Korean Bay (EKB), Korea (Figure 1A) (Kim and Kang, 1998; Kang and Kim, 2015; Seol et al., 2020). The EKB has been reported as a spawning ground for the walleye pollock living in the Korean fishing area (Kang et al., 2013; Seol et al., 2020). Although the catch of walleye pollock in Japan and Russia is relatively stable, the catch of walleye pollock in Korea, which varies depending on the number of eggs and larvae transported from the EKB, varies significantly (Kim and Kang, 1998). The yearly catch of walleye pollock in the Korean fishing area shows a dramatic decrease in the late 1980s (Figure 2). Juvenile pollock (<300 mm) catch peaked at 67 thousand metric tons (TMT) in 1984. However, catches of adult (>300 mm) and juvenile walleye pollocks suddenly collapsed in the late 1980s. The average catch of juveniles during 83–87 was 41 TMT; however, it was only 9 TMT during 88–92. Adult catches showed a similar change in the late 1980s. It was 36 TMT during 83–87 and 12 TMT during 88–92. Owing to its importance in commercial fisheries, pollock stock collapse has been a crucial issue in the management of Korean fishing, and not much effort has been directed at determining the possible causes of the collapse. Overfishing and the physical environmental changes in habitats due to climate change have been suggested as possible causes of changes in the walleye pollock stock (Jung et al., 2017; Funamoto, 2018).
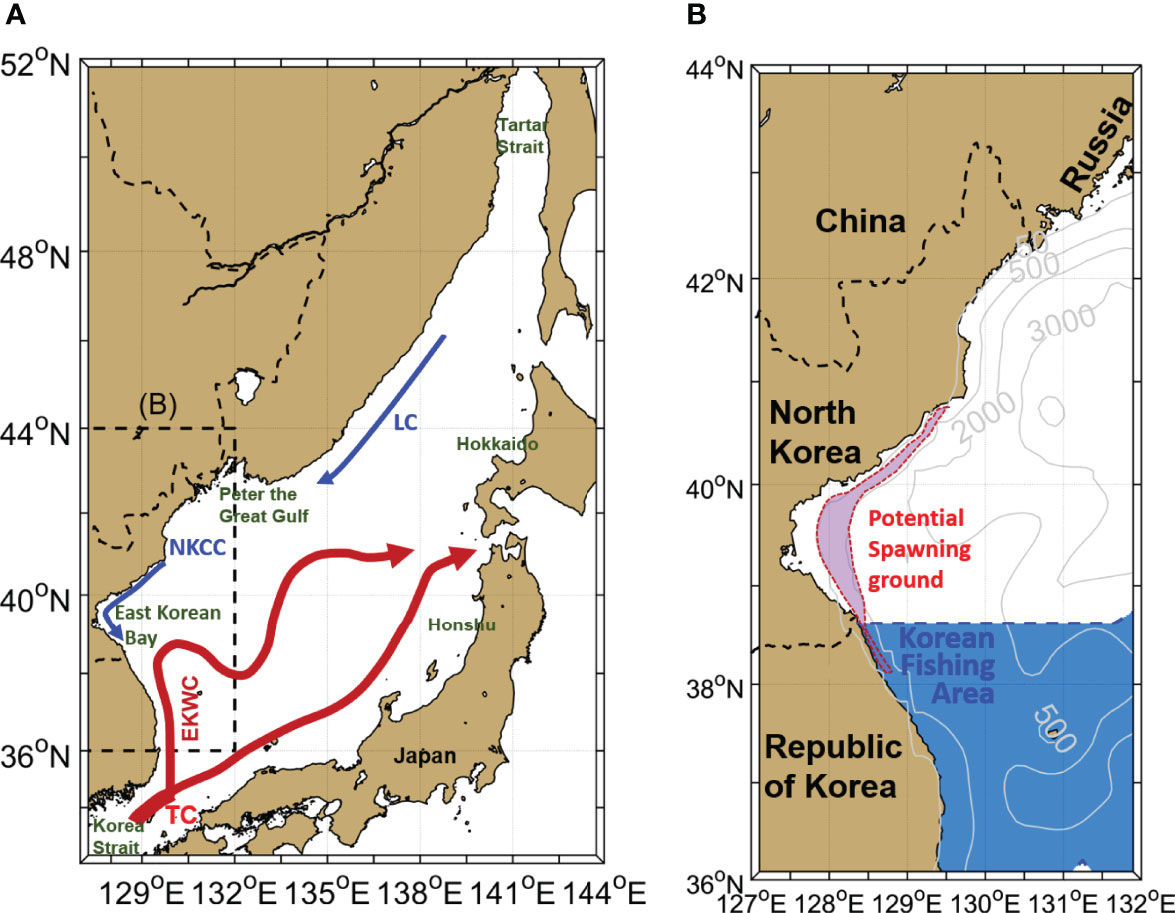
Figure 1 (A) Major currents in the East/Japan Sea (EJS). The TC, EKWC, NKCC and LC represent the Tsushima Current, East Korean Warm Current, North Korean Cold Current and Liman Current, respectively. (B) Study area indicating the spawning ground (red shading) of walleye pollock in the East Korean Bay (EKB) and Korean fishing area (blue shading).
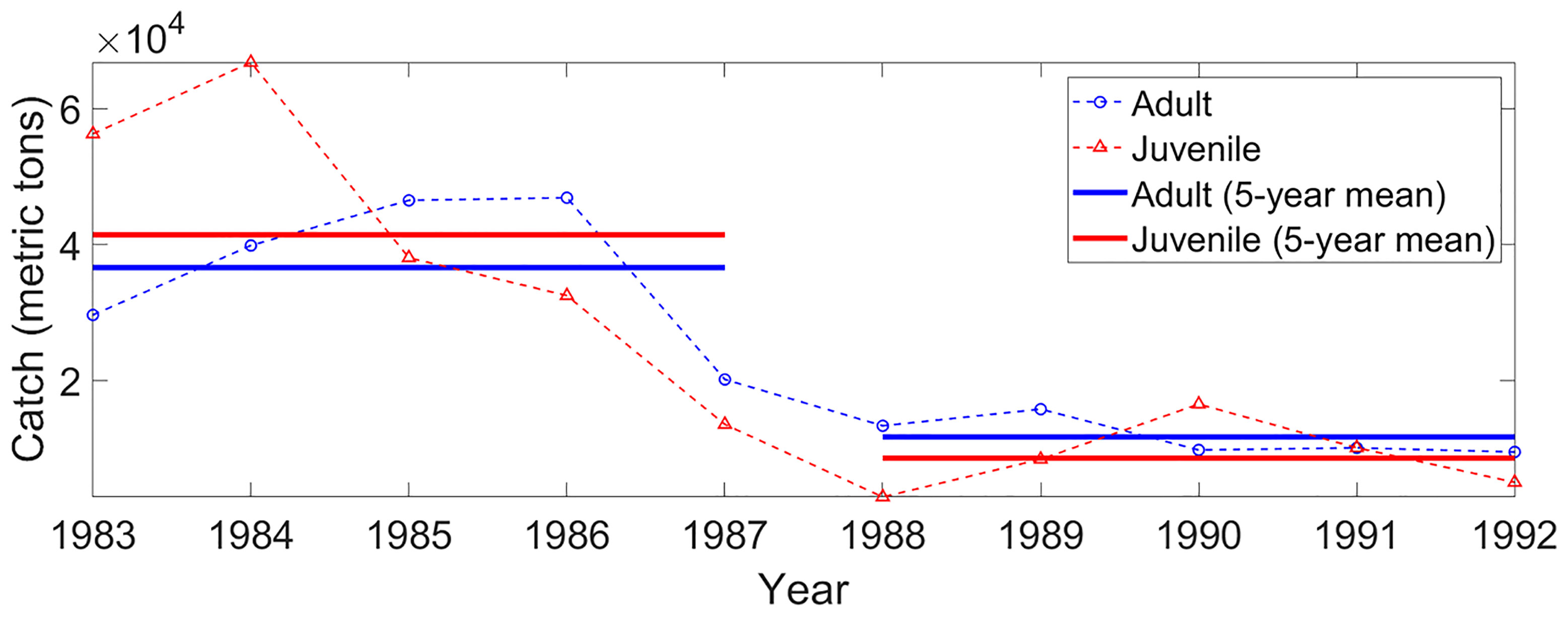
Figure 2 Annual mean catches of walleye pollock in the Korean fishing area during 1983–1992. Red triangles and blue circles denote juvenile and adult walleye pollocks, respectively. Red and blue solid lines indicate the 5-year mean catches of juvenile and adult walleye pollocks, respectively. Catch data for walleye pollock was obtained from KOSIS (Korean Statistical Information Service; https://kosis.kr/statHtml/statHtml.do?orgId=101&tblId=DT_1EW0004&conn_path=I2).
In the late 1980s, a climate regime shift (CRS) occurred as indicated by the weakened monsoon, intensified Arctic Oscillation, and weakened Siberian High and Aleutian Low-pressure systems (Jung et al., 2017; Ma et al., 2020). The responses of the marine environment due to the CRS vary around the subpolar front (Yasunaka and Hanawa, 2002). The subpolar front region of the EJS was warmed after the CRS, which had a considerable effect on the marine ecosystems (Minobe et al., 2004; Seo et al., 2006; Kim et al., 2007; Tian et al., 2008). In the southwestern EJS, seawater temperature shifted from cold to warm (8.7 to 10.3°C in February) after the late 1980s due to the alternating North Pacific High-pressure system (Kang et al., 2002; Zhang et al., 2007). This change influenced the structure and function of the western EJS ecosystem (Zhang et al., 2000).
The early life stage is the most vulnerable period in the life cycle of a fish. Walleye pollock eggs rise to the surface due to buoyancy as they develop and drift toward nursery grounds on the continental shelf near the coast due to ocean currents; therefore, the early life stage is susceptible to changes in the physical environment (Huang et al., 2021). The water temperatures and transport processes of the eggs and larvae from the spawning grounds to nursery areas affect their survival (Nakatani and Maeda, 1984; Canino, 1994). If the eggs and larvae are transported to the open ocean where feeding conditions are poor, their mortality rate increases (Kim and Kendall, 1989; Bailey et al., 1997; Bailey et al., 1999b; Kim et al., 2015; Huang et al., 2021). The transportation process around the spawning ground and changes in the environment due to the CRS are the factors that determine the recruitment success (Kasai et al., 1992; Kuroda et al., 2014; Kim et al., 2015). Particle tracking experiments based on ocean circulation models are effective attempts to simulate the transport process of eggs and larvae (Kasai et al., 1992; Bartsch and Coombs, 1997; Kim et al., 2015; Takeshige et al., 2015; Huang et al., 2021). Walleye pollock transport processes have also been investigated by several studies using particle tracking models (Hermann et al., 1996; Shimizu and Isoda, 1998; Kuroda et al., 2014; Petrik et al., 2015). Despite various studies on walleye pollock eggs and larvae transport process in many regions, numerical models on these aspects for the western EJS region are unavailable (Kang et al., 2013; Kang and Kim, 2015; Lee et al., 2019). In particular, the potential impact of CRS on egg and larval transport, which may affect the collapse of walleye pollock stock in the EKB, has not yet been revealed (Kim and Kang, 1998; Kim et al., 2007; Kang and Kim, 2015; Lee et al., 2018; Lee et al., 2019; Seol et al., 2020).
We developed a three-dimensional hydrodynamic model with data assimilation to produce reliable data to investigate the egg and larval transport process and its effect on the collapse of the walleye pollock catch in the late 1980s. Then, we simulated the egg and larval transport process using a particle tracking model. The hydrodynamic and particle tracking model configurations are introduced in Section 2. Section 3 presents the results of the particle tracking experiment. The changes to the oceanic environment and the potential effect of CRS on the collapse of the walleye pollock stock in the EKB is discussed in Section 4. Conclusions are presented in Section 5.
Model Setup and Methodology
Study Area
The EJS is a semi-enclosed deep marginal sea surrounded by Korea, Japan, and Russia. Average depth and total surface area are about 1,600m and 1.0 x 106 km2 (Chang et al., 2004). A long and narrow continental shelf and slope area exist along the eastern coast of Korea.
The Tsushima Current (TC) through the Korea Strait provides warm and saline water into the EJS (Figure 1A). The East Korean Warm Current (EKWC), which separates from the TC, flows northward along the Korean coast. In the northern basin of the EJS, the Liman Current and North Korean Cold Current (NKCC) flow southward along the Russian and Korean coasts, respectively. The EKWC encounters the NKCC and forms a subpolar front in the EKB (Lee et al., 2018). Fish community and biomass in the EJS are greatly affected by both subtropical and subarctic climates (Tian et al., 2006).
Hydrodynamic Model
We employed the Regional Ocean Modeling System (ROMS) to reproduce the physical environment (Shchepetkin and McWilliams, 2005). ROMS is a free-surface, terrain-following ocean model governed by a hydrostatic primitive equation with Boussinesq approximation. The ocean model is discretized on an Arakawa-C staggered grid in the horizontal direction. The circulation and hydrography in the EJS depend on the transport and water mass through the straits, which connect to the Northwest Pacific. The transport and water mass in the straits are determined by the interaction between the EJS and the Northwest Pacific. Therefore, our Northwest Pacific model domain covered not only the EJS but also the Northwest Pacific (15–52° N, 115–162° E; Supplementary Figure 1) to reflect the interaction through the straits. The domain had a horizontal grid size of 1/10° and 40 vertical layers. Vertical resolution varied according to the topography and was enhanced in shallow marginal seas. The model was initialized using temperature and salinity data from the World Ocean Atlas 1998 (Antonov et al., 1998; Boyer et al., 1998). The model included a spin-up period of 10 years, with surface and lateral boundary forcing for the year 1982. We used the daily mean ERA-Interim variables as the atmospheric surface forcing. ERA-Interim is a global atmospheric reanalysis produced by the European Center for Medium-Range Weather Forecasts. Mean sea level pressure, 10 m winds, 2 m air temperature, specific humidity, and shortwave radiation were used for surface forcing. A bulk formula was employed to calculate the surface heat flux (Fairall et al., 2003). Tides were included at the ocean lateral boundary by using the 10 tidal components (M2, S2, N2, K2, K1, O1, P1, Q1, Mf, and Mm) provided by the TPXO7 ocean tide model (Egbert and Erofeeva, 2002). Monthly mean discharge data from 1983 to 1992 for the Yangtze River were used. For the other 11 rivers around the Yellow Sea and Bohai Sea, we used the climate monthly mean data obtained from the Global River Discharge Database (Vörösmarty et al., 1996). Topography data were obtained from the Earth Topography 1 arc minute (ETOPO1) dataset and interpolated into the model grid points (Amante and Eakins, 2009). The model integrated every 90 seconds and produced daily output.
Data Assimilation based on the Ensemble Optimal Interpolation
The model simulation may have prediction errors caused by model uncertainty. Since the model projections are based on the initial values, errors gradually increase as the model is integrated. Data assimilation can reduce the error in a statistically optimal manner (Dee, 2005). Sea surface temperature (SST) was assimilated with the ensemble optimal interpolation (EnOI) in the Northwest Pacific ocean circulation model to reduce model error. Evensen (2003) first introduced the EnOI, which was designed to reduce the enormous number of computations used in the Ensemble Kalman Filter. The EnOI uses an ensemble from the results of long historical model runs. The EnOI analysis was applied using the following equation:
where ψa and ψf are the analysis and forecast model state vectors, respectively. As the state vector includes all variables, such as water temperature, salinity, sea surface height anomaly, u-velocity, and v-velocity, the assimilation effect can be expected for all variables. is the background covariance matrix of the static ensemble for the EnOI. The α, which adjusts the error covariance of the EnOI, is 1 in this study. H indicates the observation operator. The measurement error covariance, Re prevents the ensemble covariance from becoming too small.where ϵ indicates the observational error which is estimated by the data provider and d is the observed value.
The EnOI consisted of 37 ensemble members formed by a subsampling field from the historical model data. The historical model results of the same date for 36 years, from 1983 to 2018, was sub-sampled. The assimilation was conducted every 7 days. We adopted the horizontal and vertical decorrelation length scales of 35 km and 100 m, respectively, from Seo et al. (2010). A horizontal decorrelation length scale was set to avoid interference between each observation point. The observational data used for the assimilation were the satellite SST data from the Optimum Interpolation Sea Surface Temperature (OISST) data (Reynolds et al., 2007). Horizontal subsampling intervals were 2°, 1°, and 0.5° in the northwestern Pacific, EJS, and southwestern EJS, respectively. The observation errors provided by the OISST dataset were spatially different. The total number of observations used in each assimilation step was 541. In section 3, the reanalysis was validated by comparing the mean SSTs (from January to February) from the reanalysis and that from the OISST averaged over 1983–1987 (hereafter, 83–87) and 1988–1992 (hereafter, 88–92), which represented the periods before and after the pollock collapse, respectively (Supplementary Figure 2).
Particle Tracking Experiment
Particle tracking experiments were conducted using reanalysis data to understand the transport process of walleye pollock individuals (eggs and larvae). We used the Larval TRANSport Lagrangian model (LTRANS) configured for ROMS output (Schlag and North, 2012).
Most walleye pollock eggs spawn in January and February and stay in the surface layer (Kim et al., 2007; Kang and Kim, 2015). Therefore, the walleye pollock eggs were set to the surface grid according to the suitable spawning temperature range during the initial stage of the experiment. The suitable temperature range for spawning grounds in the model was set at 2–5°C, considering the known optimal spawning temperature (Nakatani and Maeda, 1984) and low mortality (Bailey and Stehr, 1986; Yoo et al., 2015; Seol et al., 2020). The spawning ground was located in the EKB between 36°N and 41°N (Figure 1B). Grids with a surface temperature of 2–5°C and a bottom depth of 50–500 m were selected as suitable spawning areas considering the habitat conditions (Lee et al., 2019). Suitable spawning areas during 83–87 and 88–92 were compared. We counted the suitable spawning days at grids with a temperature of 2–5°C and a bottom depth of 50–500 m in the EKB. The suitable spawning period ratio in each grid cell was defined as the ratio between suitable spawning days and total releasing days (592 days) in January and February during 83–87 and 88–92 (Figure 3).
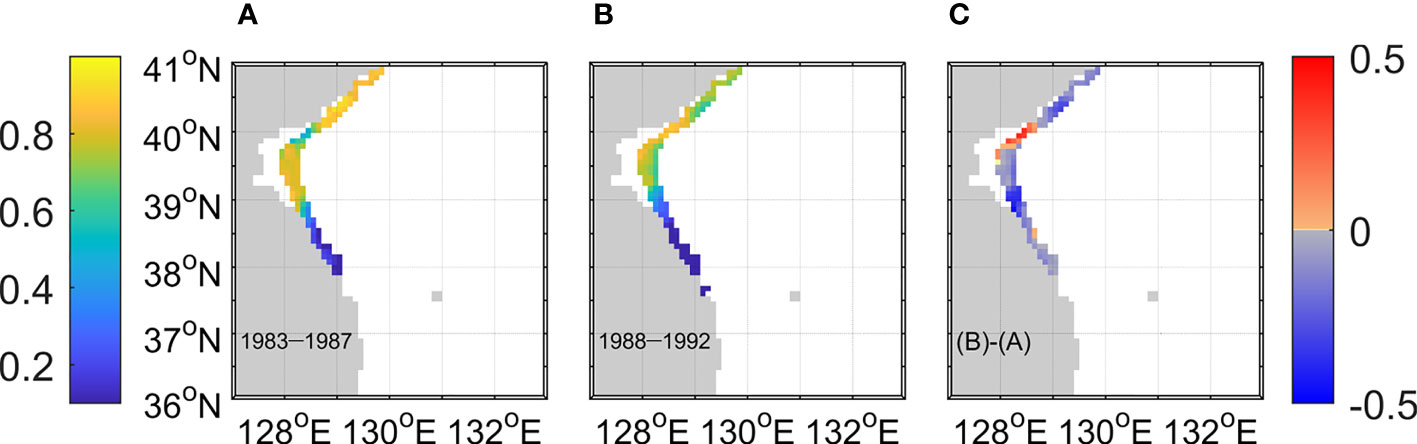
Figure 3 Suitable Spawning Period Ratio at each grid in the EKB. It was calculated as suitable days for spawning divided by total days from January to February during (A) 83–87 and (B) 88–92. (C) Difference between the two periods.
The time interval of particle movement (internal time step) was an hour, and the output frequency of the reanalysis data (external time step) was one day in the particle tracking model. A shorter time step in the particle tracking model might reduce unexpected movement of particles and improve the consistencies between projections of the model and reanalysis. Therefore, daily reanalysis data were interpolated to hourly data for the internal calculations of movements of particles. To simulate the appropriate vertical movement, it is necessary to obtain biological parameters, such as temporal changes in the density, size, and shape of the eggs and larvae as well as the vertical swimming ability and temperature selectivity of the larvae (Kuroda et al., 2014). However, there are large uncertainties associated with these biological parameters of the walleye pollock in the western EJS (Bang et al., 2018). In addition, models applying passive behavior of individuals performed better than those considering diel vertical migration (Petrik et al., 2015). Therefore, to simplify the problem, we did not consider the vertical movement of walleye pollock eggs and larvae. Random displacement was not applied and particle movement was determined considering horizontal advection at the surface. Walleye pollock individuals were tracked for 30 days from 1st January to 28th (or 29th) February during 1983–1992 (Supplementary Figure 3A). We released one egg at each suitable spawning grid corresponding to the suitable spawning conditions every day. On an average 69 eggs were released each day throughout the study and in total 40,900 eggs were released (Supplementary Figure 3B). The transport of individuals depends on the currents in the spawning area. Walleye pollock eggs take approximately 2 weeks to hatch (Blood, 1994; Bailey et al., 2012), and hatched larvae acquire swimming abilities at least 5 weeks after hatching (Stabeno et al., 1996). Therefore, the total number of individuals at each grid, 15 and 30 days after spawning during 83–87 and 88–92, were compared (Figure 4). We analyzed the abundance of individual walleye pollock 15 and 30 days after spawning to focus on egg drift and the earliest part of the larval period, when swimming abilities can be ignored.
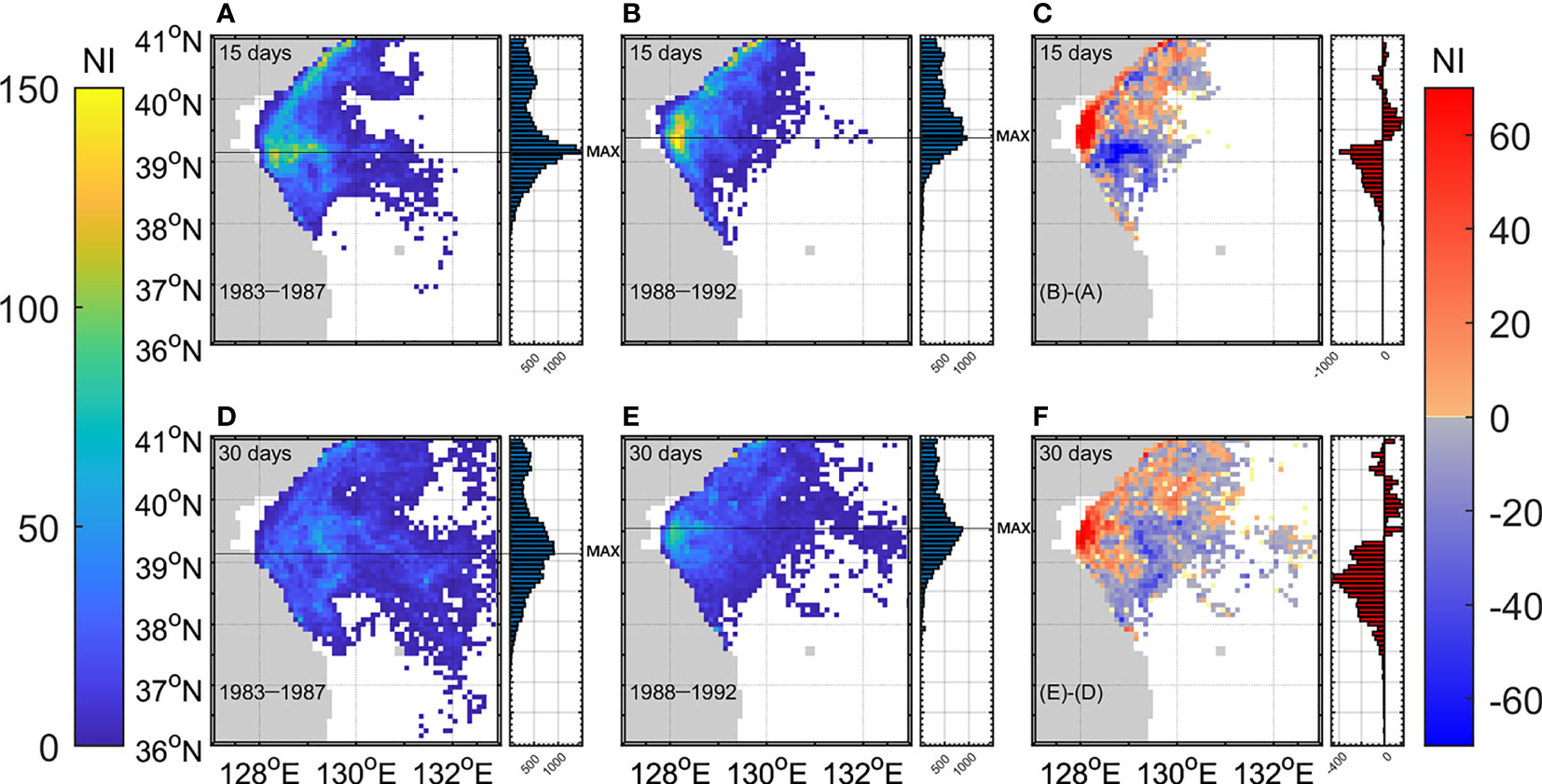
Figure 4 Horizontal distribution of individual abundance at each grid 15 days after spawning for (A) 83–87 and (B) 88–92. (C) Difference between the two periods. (D-F) are the same except at 30 days after spawning. Meridional distributions of individual abundances are denoted next to the horizontal maps.
Furthermore, we compared the mean SSTs, surface velocities, and wind velocities during 83–87 and 88–92 to investigate the environment changes around the EKB (Supplementary Figures 4–6). Temporal variations in the mean meridional travel distances of the total individuals from the spawning grounds were compared with the climate indices (Supplementary Figure 7).
Results
Reproducibility of the Reanalysis
Reproduction of surface temperature in the EJS is essential as suitable spawning grounds for walleye pollock are determined by surface temperature in this study. The latitudes of the 5 and 10°C isothermal lines were comparable in both the SSTs, although the 10°C isotherm in the reanalysis was located further north than that of the OISST near the coast (Supplementary Figure 2). The differences between the SSTs averaged over the EJS during 83–87 and 88–92 were 0.62°C in the reanalysis and 0.78°C in the observation.
The spatial mean of the root-mean-square error between the reanalysis and the OISST were 1.19 and 1.22°C during 83–87 and 88–92, respectively. Pattern correlation coefficients between the reanalysis SST and OISST were 0.98 in both periods. There were some differences between the high temperature (>13°C) in the path of the EKWC and the low temperature (<2°C) in the EKB. This difference might be due to the large uncertainty of satellite derived SST in coastal seas (Kwak et al., 2015).
Suitable Spawning Areas
Walleye pollock inhabits the cold waters along the continental shelf and slope regions (Kim and Kang, 1998; Kang and Kim, 2015). The EKB of North Korea is the most representative spawning ground in the Korean waters in winter (Kang et al., 2013). The mean suitable spawning period ratio during 83–87 was 0.64 (Figure 3A). A high ratio (>0.8) was obtained for the northern EKB during 83–87. A suitable spawning period ratio of 0.8 indicated that the egg particles were released on 474 days which was 80% of the total particle tracking experiment days (592 days). The ratio for the southern EKB was lower than 0.3. The mean ratio decreased to 0.53 during 88–92 (Figure 3B) despite a marginal increase around 40°N. The decrease in mean ratio was 0.11 during 83–87 and 88–92 (Figure 3C).
Transport of Individuals
The individuals were widely dispersed over time. The abundance of individual walleye pollock after 15 days were higher in the central area of the EKB than in other areas during both periods (Figures 4A, B). Nevertheless, a considerable number of individuals were advected offshore by the current. Individuals were even more concentrated in the central coast of the EKB and the number of individuals increased by approximately 60, because of the decreased southward dispersion of individuals (Figure 4C). The individuals followed similar patterns 30 days after spawning during both periods (Figures 4D, E), although they were dispersed further widely from spawning grounds. The difference in the number of individuals also showed a similar pattern as that after 15 days. More individuals were found in the northern EKB than in the southern EKB during 88–92 (Figure 4F).
In addition, meridional distributions of the abundance of individuals are represented in Figure 4. The individual abundance pattern with latitude showed an asymmetric distribution with more individuals in the north during both periods. The degree of asymmetry was high during 88–92. The maximum abundance of individuals after both 15 and 30 days appeared at 39.1°N during 83–87 (Figures 4A, D), but it moved north to 39.4°N after 15 days and 39.5°N after 30 days during 88–92 (Figures 4B, E). Significant differences were found between the mean of the total number of individuals between the two periods (t-test, p < 0.01). The differences were noticeable after 15 and 30 days in the southern EKB. The abundance of individuals in the southern EKB during 88–92 was less than half of that during 83–87 (Figures 4C, F).
Additionally, zonal distributions of the abundance of individuals from 36°N to 38.6°N were examined to compare the individuals in the nursery ground of the Korean fishing area between the two periods. Figure 5 shows the individual abundance according to the distance from the coast in the Korean fishing area. The individuals decreased over the entire area during 88–92 due to the intensified EKWC. The decrease in the number of individuals was more prominent in the nursery ground on the continental shelf and slope near the coast (<100 km). The individual abundance decreased by 71% after 15 days and by 74% after 30 days during 88–92 (Figures 5C, F).
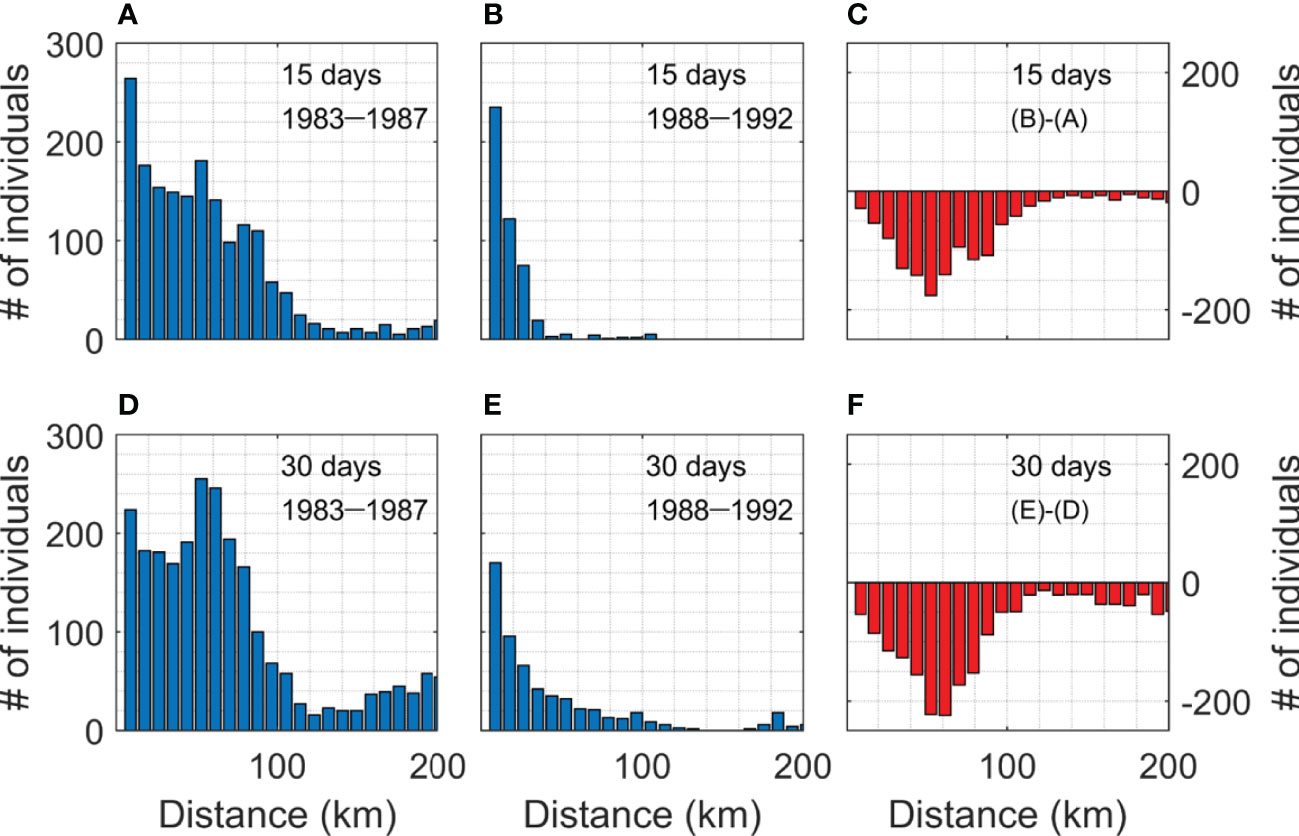
Figure 5 Zonal distribution of individual abundances from 36°N to 38.6°N according to the distance from the coast 15 days after spawning for (A) 83–87 and (B) 88–92. (C) Difference between the two periods. (D-F) are the same, except at 30 days after spawning in the nursery within the Korean fishing area.
Discussion
Decrease of Walleye Pollock and Change in Oceanic Environment
The spawning grounds of walleye pollock are mainly located in the continental shelf area (Bailey et al., 1997; Kang and Kim, 2015). Floating eggs of walleye pollock are mainly distributed in the surface layer of the ocean owing to their buoyancy (Oh et al., 2004). As a result, environmental changes in the surface layer may influence the spawning of walleye pollock eggs and the survival of larvae (Funamoto, 2007). In particular, the water temperature of the upper layer directly affects the mortality of the floating eggs and indirectly influences the recruitment and population of walleye pollock (Hamatsu et al., 2004; Oh et al., 2004; Tian et al., 2008; Yoo et al., 2015). The SST difference during 83–87 and 88–92 shows remarkable warming in the EKB, known as the spawning ground of walleye pollock (Supplementary Figure 4C). The maximum increase in the SST was >3°C. This warming may have reduced the suitable spawning area. Warming in the Korean fishing area may also influence the juveniles and adults. Change in temperature is an important factor that may alter the physiological functioning, behavior, and demographic traits of organisms. Temperature changes lead to shifts in the size structure, spatial range, and seasonal abundance of fish populations (Doney et al., 2012). Intolerable temperature conditions promote migration or local extinction if adaptation is impossible (Parmesan, 2006). Warming in the Korean fishing area prevented the formation of suitable habitats. Reduced habitats may accelerate the collapse of walleye pollock stocks. In the southern EKB (~39°N), the difference in the mean suitable spawning period ratio was 0.17 due to the warming (Figure 3C). Reduced spawning grounds might be the cause for the decrease in walleye pollock catch in the late 1980s (Seol et al., 2020). The mean SST increased by 0.8 °C in the EKB and the Korean fishing area during 83–87 and 88–92, whereas the global mean SST increased by approximately 0.4 °C from the 1950s to the 2000s (Levitus et al., 2009). The warming in the EKB and the Korean fishing area was linked to the intensification of the EKWC.
Horizontal advection has a significant role in the ecosystem variability, especially in the strong boundary current regions where the currents transport temperature anomalies and change nutrient availability (Miller et al., 2004). Current patterns linked to subarctic front systems usually change due to climate variation in the EJS (Kim et al., 2007). The EKWC, which transports large amounts of heat to the EKB, is a dominant flow along the Korean coast during both periods. The SST in the EKB is affected by the path of the EKWC. Previous studies have reported that the EKWC generally separates from the coast at approximately 38°N and flows eastward, based on observations (Cho and Kim, 1996; Chang et al., 2004). Most model-based experiments have had difficulty resolving the exact path of the EKWC (Seung, 1993; Hogan and Hurlburt, 2000). However, we were able to depict the path of the EKWC more accurately using data assimilation. There was a significant difference between the paths of the EKWC during the two periods (Supplementary Figure 5C). The EKWC flowed further north during 88–92 than during 83–87.
In the late 1980s, increased temperature and intensified northward flow around the spawning ground in the southern EKB might negatively influence the early life stages of walleye pollock in the EJS. A warmed spawning ground decreased the spawning rate. The walleye pollock that remained in the southern EKB decreased as the intensified northward current transported the eggs and larvae further north. Walleye pollock might change the spawning ground and habitat to adjust to the warmed spawning condition and transport northward to fulfil its life cycle. This change in habitat might shift the spawning migration route further north. The northward shifting of spawning ground, habitat, and migration route might eventually lead to the collapse of walleye pollock in the Korean fishing area. The proportion of walleye pollock catch in the Korean fishery dropped after the late 1980s regime shift (Kang and Kim, 2015). Decreased walleye pollock catch in the late 1980s continued until the 2020s. In addition to the environmental changes, there was high fishing pressure on the juvenile pollock from the 1970s to 1990s as the catch of adult walleye Pollock decreased (Kang and Kim, 2015; Bang et al., 2018).
Climate Regime Shift in the Late 1980s
The path and speed of the ocean current might be affected by northwesterly winds, which are dominant in the EJS during winter (Seager et al., 2001). The northwesterly wind was predominant during both periods (Supplementary Figures 6A, B). The northwesterly wind can generate a southward surface Ekman current suppressing the northward EKWC. It drags water southeastward in the ocean, and the Coriolis force acts southwestward. The balance between the drag and Coriolis force eventually moves water southward at the surface. The difference in wind velocity between the two periods shows that the northwesterly wind was weakened, which resulted in a more northward flow of the EKWC and heat transport during 88–92 (Supplementary Figure 6C). Our model result suggested that the intensified northward flow and increased heat transport resulted in warm conditions and decreased the walleye pollock eggs and larvae in the southern EKB. The decreased walleye pollock eggs and larvae may be linked to the collapse of walleye pollock catch in the Korean fishing area.
Changes in spawning and transport of walleye pollock eggs are attributed to ocean temperature and currents, which are determined by atmospheric conditions. Reanalysis in this study was forced by atmospheric boundary conditions, which reflect realistic atmospheric climate change in the North Pacific. The change in wind velocity might result from the difference in atmospheric pressure, which is practically quantified by climate indices.
Typical climate indices in the North Pacific are the Arctic Oscillation Index (AOI) and the East Asian Winter Monsoon Index (EAWMI) in winter (Rebstock and Kang, 2003; Tian et al., 2006; Kim et al., 2007; Tian et al., 2008; Jung et al., 2017). It is known that oceanic changes in the EJS are also highly associated with variations in the AOI and EAWMI (Thompson and Wallace, 1998; Jung et al., 2017). Supplementary Figures 7A, B indicate the temporal variation of AOI and EAWMI during the study period. The AOI is defined by surface atmospheric pressure patterns (Thompson and Wallace, 1998). The EAWMI is defined as the difference in the area-averaged zonal wind speed at the 300-hPa level, and it represents the intensity of the winter monsoon (Jhun and Lee, 2004). We filtered the high-frequency signals for less than 10 years from the long-period climate indices (1970–2019). Both climate indices have suddenly changed in the late 1980s, and this change has been reported as the CRS in many previous studies (Tian et al., 2006; Tian et al., 2008; Nagato and Tanaka, 2012; Jung et al., 2017; Ma et al., 2020). The AOI shifted from a negative phase to a positive phase in the late 1980s. The Arctic wind vortex strengthened and hindered the southward intrusion of cold air to mid-latitudes during the positive period of the AOI. The positive phase led to warm conditions in the walleye pollock spawning grounds and Korean fishing area. The warm conditions in the Korean fishing area might result in adverse oceanic conditions for the adult walleye pollock due to the altered ecosystem, related to competition with other species (Kang et al., 2000). The CRS with a weakened monsoon and intensified northward warm current might have provided an unfavorable condition for the walleye pollock in the late 1980s.
Most of the mean distances of individuals were >20 km southward for 30 days during 83–87, but >30 km northward during 88–92 (Supplementary Figure 7C). The increase in northward individuals resulted in a decrease in the number of individuals transported to the nursery in the Korean fishing area (Figure 5). The fewer eggs and larvae transported to the nursery might cause a decrease in the walleye pollock catch in the Korean fishing area. The transport of individuals to the nursery area is closely linked to the survival rates of the larvae and juveniles, which is critical for the abundance of walleye pollock (Funamoto, 2018). A comparison of temporal variation between the climate indices and travel distances of the individuals suggests that the CRS might be responsible for changes in spawning rates, transport of the individuals, mortality, and abundance of walleye pollock in the Korean fishing area.
Conclusions
The catch of walleye pollock in the Korean fishing area rapidly decreased in the late 1980s. The lag correlation between the juvenile and adult walleye pollock catches implies that the walleye pollock stock depends on the survival in early life stages. The survival of walleye pollock in its early life stage is closely related to the water temperature and the transport process in the spawning area. The cause of catch collapse has not yet been clarified because of the lack of reliable environmental data. The regional hydrodynamic model results with data assimilation provided more reliable reanalysis data. The particle tracking model experiment enabled us to investigate the potential impact of environmental changes on the transport of early life individuals, such as eggs and larvae.
Based on the reanalysis data, the area of estimated suitable spawning grounds decreased due to warming in the late 1980s. Moreover, the southward bound individuals from the spawning grounds to the nurseries in the Korean fishing area decreased due to the intensification of the northward EKWC during 88–92. Reduction of suitable spawning ground and individuals transported southward to the nursery could have resulted in a decrease in walleye pollock juveniles in the Korean fishing area. Unfavorable oceanic conditions for the spawning and nursery areas might have influenced not only the survival of larvae but also the recruitment and year-class strength of the population of walleye pollock. Furthermore, increased water temperature might negatively affect walleye pollock adults living in the Korean fishing area (Kotwicki et al., 2005). These ecological changes in the life history of walleye pollock might have influenced its collapse in the late 1980s.
Oceanic environmental changes in the late 1980s were associated with fluctuations in atmospheric conditions in the northern hemisphere such as the Arctic Oscillation (AO) and East Asian Winter Monsoon. The Korean fishing area was warmed, and the northwesterly suppression of the northward EKWC was weakened in the late 1980s. The CRS with a positive AO is responsible for weakening the northwesterly and the increase of SST in January and February. Therefore, the CRS, which induced atmospheric and oceanic changes in the late 1980s, might have impacted the collapse of the walleye pollock catch in the Korean fishing area.
Both CRS and overfishing could cause the collapse of the walleye pollock catch. Heavy fishing activities on juveniles and spawners in the EKB might accelerate the decrease in the walleye pollock catch (Kim et al., 2014). Although several studies have been conducted on walleye pollock in the EJS, its recruitment mechanisms, prey-predator relationship, and food availability under changing environments, and ecology in the western EJS still remain to be understood comprehensively. We defined the spawning grounds based on physical parameters, such as temperature and bottom depth. However, spawning grounds might also be influenced by other biotic and abiotic factors. Fluctuation in female spawning biomass over previous years changed the production of eggs and the availability of hatched larvae (Smart et al., 2012). In addition, walleye pollock adults spawn eggs to areas where suitable larval food is abundant (Kendall and Nakatani, 1992). These factors may interact in various spatial and temporal scales and affect the production, abundance, and distribution patterns of eggs (Brodeur et al., 1996). Biotic factors influencing the spawning conditions need to be considered in the future study. In addition, a profound understanding of the walleye pollock ecology in the EJS, considering egg size, mortality, year-class, recruitment, vertical migration, and swimming ability, will help in the development of an individual-based model.
Data Availability Statement
The raw data supporting the conclusions of this article will be made available by the authors, without undue reservation.
Author Contributions
Conceptualization: Y-YK and Y-KC. Methodology: Y-YK, S-TL and HJ. Writing draft: Y-YK. Review and editing: SK and CL. Visualization: Y-YK and Y-KK. Supervision: Y-KC. Project administration: K-YJ and D-SB. All authors contributed to the article and approved the submitted version.
Funding
This research was a part of the project titled “Analysis and Projection of Long-term Sea Level Change in Response to Climate Change around Korean Peninsula (2021)” funded by the Korea Hydrographic and Oceanographic Agency (KHOA) and Ministry of Education of the Republic of Korea and the National Research Foundation of Korea (NRF-2021R1A2B5B03087097).
Conflict of Interest
The authors declare that the research was conducted in the absence of any commercial or financial relationships that could be construed as a potential conflict of interest.
Publisher’s Note
All claims expressed in this article are solely those of the authors and do not necessarily represent those of their affiliated organizations, or those of the publisher, the editors and the reviewers. Any product that may be evaluated in this article, or claim that may be made by its manufacturer, is not guaranteed or endorsed by the publisher.
Supplementary Material
The Supplementary Material for this article can be found online at: https://www.frontiersin.org/articles/10.3389/fmars.2022.802748/full#supplementary-material
References
Amante C., Eakins B. W. (2009). “ETOPO1 1 Arc-Minute Global Relief Model: Procedures, Data Sources and Analysis,” in Technical Memorandum NESDIS NGDC-24 (Washington: NOAA), 19.
Antonov J., Levitus S., Boyer T., Conkright M., O'Brien T., Stephens C. (1998). World Ocean Atlas 1998 Vol. 2: Temperature of the Pacific Ocean, NOAA Atlas NESDIS 28 (Washington, DC: US Government Printing Office).
Bailey K. M., Bond N. A., Stabeno P. J. (1999b). Anomalous Transport of Walleye Pollock Larvae Linked to Ocean and Atmospheric Patterns in May 1996. Fish. Oceanogr. 8, 264–273. doi: 10.1046/j.1365-2419.1999.00113.x
Bailey K., Quinn T. II, Bentzen R., Grant W. S. (1999a). Population Structure and Dynamics of Walleye Pollock, Theragra Chalcogramma. Adv. Mar. Biol. 37, 179–255. doi: 10.1016/S0065-2881(08)60429-0
Bailey K., Stabeno P., Powers D. (1997). The Role of Larval Retention and Transport Features in Mortality and Potential Gene Flow of Walleye Pollock. J. Fish. Biol. 51, 135–154. doi: 10.1111/j.1095-8649.1997.tb06097.x
Bailey K., Stehr C. L. (1986). Laboratory Studies on the Early Life History of the Walleye Pollock, Theragra Chalcogramma (Pallas). J. Exp. Mar. Biol. Ecol. 99, 233–246. doi: 10.1016/0022-0981(86)90225-X
Bailey K. M., Zhang T., Chan K., Porter S. M., Dougherty A. B. (2012). Near Real-Time Forecasting of Recruitment From Larval Surveys: Application to Alaska Pollock. Mar. Ecol.-Prog. Ser. 452, 205–217. doi: 10.3354/meps09614
Bang M., Kang S., Kim S., Jang C. J. (2018). Changes in the Biological Characteristics of Walleye Pollock Related to Demographic Changes in the East Sea During the Late 20th Century. Mar. Coast. Fish. 10, 91–99. doi: 10.1002/mcf2.10004
Bartsch J., Coombs S. (1997). A Numerical Model of the Dispersion of Blue Whiting Larvae, Micromesistiuspoutassou (Risso), in the Eastern North Atlantic. Fish. Oceanogr. 6, 141–154. doi: 10.1046/j.1365-2419.1997.00036.x
Beamish R., McFarlane G. A. (1995). “A Discussion of the Importance of Aging Errors, and an Application to Walleye Pollock: The World’s Largest Fishery,” in Recent Developments in Fish Otolith Research (Columbia: University of South Carolina Press), 545–565.
Blood D. (1994). Embryonic Development of Walleye Pollock, Theragra Chalcogramma, From Shelikof Strait, Gulf of Alaska. Fish. Bull. 92, 207–222. doi: 10.1111/j.1365-2419.1996.tb00080.x
Boyer T., Levitus S., Antonov J., Conkright M., O’Brien T., Stephens C. (1998). World Ocean Atlas 1998 Vol. 5: Salinity of the Pacific Ocean, NOAA Atlas NESDIS 31 (Washington, DC: US Government Printing Office).
Brodeur R. D., Picquelle S. J., Blood D. M., Merati N. (1996). Walleye Pollock Egg Distribution and Mortality in the Western Gulf of Alaska. Fish. Oceanogr. 5, 92–111. doi: 10.1111/j.1365-2419.1996.tb00085.x
Canino M. F. (1994). Effects of Temperature and Food Availability on Growth and RNA/DNA Ratios of Walleye Pollock Theragra Chalcogramma (Pallas) Eggs and Larvae. J. Exp. Mar. Biol. Ecol. 175, 1–16. doi: 10.1016/0022-0981(94)90173-2
Chang K.-I., Teague W., Lyu S., Perkins H., Lee D.-K., Watts D., et al. (2004). Circulation and Currents in the Southwestern East/Japan Sea: Overview and Review. Prog. Oceanogr. 61, 105–156. doi: 10.1016/j.pocean.2004.06.005
Cho Y.-K., Kim K. (1996). Seasonal Variation of the East Korea Warm Current and Its Relation With the Cold Water. La. Mer. 34, 172–182.
Dee D. P. (2005). Bias and Data Assimilation. Q. J. R. Meteorol. Soc. 131, 3323–3343. doi: 10.1256/qj.05.137
Doney S. C., Ruckelshaus M., Emmett Duffy J., Barry J. P., Chan F., English C. A., et al. (2012). Climate Change Impacts on Marine Ecosystems. Ann. Rev. Mar. Sci. 4, 11–37. doi: 10.1146/annurev-marine-041911-111611
Egbert G. D., Erofeeva S. Y. (2002). Efficient Inverse Modeling of Barotropic Ocean Tides. J. Atmos. Ocean. Tech. 19, 183–204. doi: 10.1175/1520-0426(2002)019<0183:EIMOBO>2.0.CO;2
Evensen G. (2003). The Ensemble Kalman Filter: Theoretical Formulation and Practical Implementation. Ocean. Dyn. 53, 343–367. doi: 10.1007/s10236-003-0036-9
Fairall C., Bradley E. F., Hare J., Grachev A., Edson J. (2003). Bulk Parameterization of Air–Sea Fluxes: Updates and Verification for the COARE Algorithm. J. Clim. 16, 571–591. doi: 10.1175/1520-0442(2003)016<0571:BPOASF>2.0.CO;2
FAO (2020). “The State of World Fisheries and Aquaculture 2020” in Sustainability in Action (Rome: Food and Agriculture Organization).
Funamoto T. (2007). Temperature-Dependent Stock-Recruitment Model for Walleye Pollock (Theragra Chalcogramma) Around Northern Japan. Fish. Oceanogr. 16, 515–525. doi: 10.1111/j.1365-2419.2007.00454.x
Funamoto T. (2018). Population Dynamics of Demersal Fish Focusing on Walleye Pollock (Gadus Chalcogrammus). Fish. Population Dynam. Monitor. Manage. 51–75. doi: 10.1007/978-4-431-56621-2_4
Hamatsu T., Yabuki K., Watanabe K. (2004). Decadal Changes in Reproduction of Walleye Pollock (Theragra Chalcogramma) Off the Pacific Coast of Northern Japan. Fish. Oceanogr. 13, 74–83. doi: 10.1111/j.1365-2419.2004.00311.x
Hermann A. J., Rugen W. C., Stabeno P. J., Bond N. A. (1996). Physical Transport of Young Pollock Larvae (Theragra Chalcogramma) Near Shelikof Strait as Inferred From a Hydrodynamic Model. Fish. Oceanogr. 5, 58–70. doi: 10.1111/j.1365-2419.1996.tb00082.x
Hogan P. J., Hurlburt H. E. (2000). Impact of Upper Ocean–Topographical Coupling and Isopycnal Outcropping in Japan/East Sea Models With 1/8˚ to 1/64˚ Resolution. J. Phys. Oceanogr. 30, 2535–2561. doi: 10.1175/1520-0485(2000)030<2535:IOUOTC>2.0.CO;2
Huang S., Deng Z., Tang G., Li H., Yu T. (2021). Numerical Study on Blue Mackerel Larval Transport in East China Sea. J. Mar. Syst. 217, 103515. doi: 10.1016/j.jmarsys.2021.103515
Jhun J.-G., Lee E.-J. (2004). A New East Asian Winter Monsoon Index and Associated Characteristics of the Winter Monsoon. J. Clim. 17, 711–726. doi: 10.1175/1520-0442(2004)017<0711:ANEAWM>2.0.CO;2
Jung H. K., Rahman S. M., Kang C.-K., Park S.-Y., Lee S. H., Park H. J., et al. (2017). The Influence of Climate Regime Shifts on the Marine Environment and Ecosystems in the East Asian Marginal Seas and Their Mechanisms. Deep. Sea. Res. Part II. 143, 110–120. doi: 10.1016/j.dsr2.2017.06.010
Kang J.-s. (2009). The Dispute Over the Pollock Fishing Spot in Hamkyeongnamdo Under Japanese Imperialism: A Controversy About Motor Boat. Daegu. Hist. Rev. 96, 85–115.
Kang S., Kim S. (2015). What Caused the Collapse of Walleye Pollock Population in Korean Waters? KMI. Int. J. Marit. Aff. Fish. 7, 43–58. doi: 10.54007/ijmaf.2015.7.1.43
Kang S., Kim S., Bae S.-W. (2000). Changes in Ecosystem Components Induced by Climate Variability Off the Eastern Coast of the Korean Peninsula During 1960–1990. Prog. Oceanogr. 47, 205–222. doi: 10.1016/S0079-6611(00)00043-4
Kang Y. S., Kim J. Y., Kim H. G., Park J. H. (2002). Long-Term Changes in Zooplankton and Its Relationship With Squid, Todarodes Pacificus, Catch in Japan/East Sea. Fish. Oceanogr. 11, 337–346. doi: 10.1046/j.1365-2419.2002.00211.x
Kang S., Park J. H., Kim S. (2013). Size-Class Estimation of the Number of Walleye Pollock Theragra Chalcogramma Caught in the Southwestern East Sea During the 1970s-1990s. Kor. J. Fisher. Aquat. Sci. 46, 445–453. doi: 10.5657/KFAS.2013.0445
Kasai A., Kishi M. J., Sugimoto T. (1992). Modeling the Transport and Survival of Japanese Sardine Larvae in and Around the Kuroshio Current. Fish. Oceanogr. 1, 1–10. doi: 10.1111/j.1365-2419.1992.tb00020.x
Kendall A., Nakatani T. (1992). Comparisons of Early-Life-History Characteristics of Walleye Pollock Theragra Chalcogramma in Shelikof Strait, Gulf of Alaska, and Funka Bay, Hokkaido, Japan. Fish. Bull. 90, 129–138.
Kim S., Kang S. (1998). The Status and Research Direction for Fishery Resources in the East Sea/Sea of Japan. Kor. Soc. Fish. Res. 1, 44–58.
Kim D.-H., Kang S., Kim S. (2014). Market and Consumption of Walleye Pollock in Korea. Fisher. Sci. 80, 213–218. doi: 10.1007/s12562-014-0725-y
Kim S., Kendall J. A. W. (1989). Distribution and Transport of Larval Walleye Pollock (Theragra Chalcogramma) in Shelikof Strait, Gulf of Alaska, in Relation to Water Movement. Conseil. Int. Pour l’Exploration. La. Mer. 191, 127–136.
Kim J. J., Stockhausen W., Kim S., Cho Y.-K., Seo G.-H., Lee J.-S. (2015). Understanding Interannual Variability in the Distribution of, and Transport Processes Affecting, the Early Life Stages of Todarodes Pacificus Using Behavioral-Hydrodynamic Modeling Approaches. Prog. Oceanogr. 138, 571–583. doi: 10.1016/j.pocean.2015.04.003
Kim S., Zhang C.-I., Kim J.-Y., Oh J.-H., Kang S., Lee J. B. (2007). Climate Variability and Its Effects on Major Fisheries in Korea. Ocean. Sci. J. 42, 179–192. doi: 10.1007/BF03020922
Kotwicki S., Buckley T. W., Honkalehto T., Walters G. (2005). Variation in the Distribution of Walleye Pollock (Theragra Chalcogramma) With Temperature and Implications for Seasonal Migration. Fish. Bull. 103, 574–587.
Kuroda H., Takahashi D., Mitsudera H., Azumaya T., Setou T. (2014). A Preliminary Study to Understand the Transport Process for the Eggs and Larvae of Japanese Pacific Walleye Pollock Theragra Chalcogramma Using Particle-Tracking Experiments Based on a High-Resolution Ocean Model. Fisher. Sci. 80, 127–138. doi: 10.1007/s12562-014-0717-y
Kwak M.-T., Seo G.-H., Cho Y.-K., Kim B.-G., You S. H., Seo J.-W. (2015). Long-Term Comparison of Satellite and In-Situ Sea Surface Temperatures Around the Korean Peninsula. Ocean. Sci. J. 50, 109–117. doi: 10.1007/s12601-015-0009-1
Lee C. I., Han M. H., Jung H. K., Park H. J., Park J. M. (2019). Spawning Season, and Factors Influencing Allometric Growth Pattern and Body Condition of Walleye Pollock Gadus Chalcogrammus in the Middle East Sea, Korea. Kor. J. Ichthyol. 31, 141–149. doi: 10.35399/ISK.31.3.3
Lee C. I., Rahman S. M., Jung H. K., Kang C.-K., Park H. J. (2018). Oceanic and Fisheries Response in the Northwest Pacific Marginal Seas With Climate Variability. Environ. Manage. Mar. Ecosys. 99, 99–115. doi: 10.1201/9781315153933-4
Levitus S., Antonov J. I., Boyer T. P., Locarnini R. A., Garcia H. E., Mishonov A. V. (2009). Global Ocean Heat Content 1955–2008 in Light of Recently Revealed Instrumentation Problems. Geophys. Res. Lett. 36, L07608. doi: 10.1029/2008GL037155
Makino M., Kim S., Velikanov A., Criddle K., Funamoto T., Hirota M., et al. (2014). Introduction: From the Birth to the Table of Walleye Pollock Theragra Chalcogramma. Fisher. Sci. 80, 103–107. doi: 10.1007/s12562-014-0728-8
Ma S., Tian Y., Li J., Yu H., Cheng J., Sun P., et al. (2020). Climate Variability Patterns and Their Ecological Effects on Ecosystems in the Northwestern North Pacific. Front. Mar. Sci. 7. doi: 10.3389/fmars.2020.546882
Miller A. J., Chai F., Chiba S., Moisan J. R., Neilson D. J. (2004). Decadal-Scale Climate and Ecosystem Interactions in the North Pacific Ocean. J. Oceanogr. 60, 163–188. doi: 10.1023/B:JOCE.0000038325.36306.95
Minobe S., Sako A., Nakamura M. (2004). Interannual to Interdecadal Variability in the Japan Sea Based on a New Gridded Upper Water Temperature Dataset. J. Phys. Oceanogr. 34, 2382–2397. doi: 10.1175/JPO2627.1
Nagato Y., Tanaka H. (2012). Global Warming Trend Without the Contributions From Decadal Variability of the Arctic Oscillation. Polar. Sci. 6, 15–22. doi: 10.1016/j.polar.2012.02.001
Nakatani T., Maeda T. (1984). Thermal Effect on the Development of Walleye Pollock Eggs and Their Upward Speed to the Surface. Bull. Japan. Soc. Sci. Fisher. 50, 937–942.
Oh T., Sakuramoto K., Lee S. (2004). The Relationship Between Spawning Area Water Temperature and Catch Fluctuation of Walleye Pollock in the East Sea/Sea of Japan. Kor. Soc. Fish. Res. 6, 1–13.
Park H. J., Park T. H., Lee C.-I., Kang C.-K. (2018). Ontogenetic Shifts in Diet and Trophic Position of Walleye Pollock, Theragra Chalcogramma, in the Western East Sea (Japan Sea) Revealed by Stable Isotope and Stomach Content Analyses. Fish. Res. 204, 297–304. doi: 10.1016/j.fishres.2018.03.006
Parmesan C. (2006). Ecological and Evolutionary Responses to Recent Climate Change. Annu. Rev. Ecol. Evol. Syst. 37, 637–669. doi: 10.1146/annurev.ecolsys.37.091305.110100
Petrik C. M., Duffy-Anderson J. T., Mueter F., Hedstrom K., Curchitser E. N. (2015). Biophysical Transport Model Suggests Climate Variability Determines Distribution of Walleye Pollock Early Life Stages in the Eastern Bering Sea Through Effects on Spawning. Prog. Oceanogr. 138, 459–474. doi: 10.1016/j.pocean.2014.06.004
Rebstock G. A., Kang Y. S. (2003). A Comparison of Three Marine Ecosystems Surrounding the Korean Peninsula: Responses to Climate Change. Prog. Oceanogr. 59, 357–379. doi: 10.1016/j.pocean.2003.10.002
Reynolds R. W., Smith T. M., Liu C., Chelton D. B., Casey K. S., Schlax M. G. (2007). Daily High-Resolution-Blended Analyses for Sea Surface Temperature. J. Clim. 20, 5473–5496. doi: 10.1175/2007JCLI1824.1
Schlag Z., North E. (2012). Lagrangian TRANSport Model (LTRANS V. 2) Users Guide (Cambridge, MD: Cambridge).
Seager R., Kushnir Y., Naik N. H., Cane M. A., Miller J. (2001). Wind-Driven Shifts in the Latitude of the Kuroshio–Oyashio Extension and Generation of SST Anomalies on Decadal Timescales. J. Clim. 14, 4249–4265. doi: 10.1175/1520-0442(2001)014<4249:WDSITL>2.0.CO;2
Seo G.-H., Choi B.-J., Cho Y.-K., Kim Y. H., Kim S. (2010). Assimilation of Sea Surface Temperature in the Northwest Pacific Ocean and Its Marginal Seas Using the Ensemble Kalman Filter. Ocean. Sci. J. 45, 225–242. doi: 10.1007/s12601-010-0021-4
Seo H., Kim S., Seong K., Kang S. (2006). Variability in Scale Growth Rates of Chum Salmon (Oncorhynchus Keta) in Relation to Climate Changes in the Late 1980s. Prog. Oceanogr. 68, 205–216. doi: 10.1016/j.pocean.2006.02.003
Seol K., Lee C.-I., Jung H.-K. (2020). Long Term Changes in Sea Surface Temperature Around Habitat Ground of Walleye Pollock (Gadus Chalcogrammus) in the East Sea. J. Kor. Soc. Mar. Environ. Saf. 26, 195–205. doi: 10.7837/kosomes.2020.26.2.195
Seung Y. H. K. (1993). A Numerical Modeling of the East Sea Circulation. J. Oceanol. Soc Kor. 28, 292–304.
Shchepetkin A. F., McWilliams J. C. (2005). The Regional Oceanic Modeling System (ROMS): A Split-Explicit, Free-Surface, Topography-Following-Coordinate Oceanic Model. Ocean. Model. 9, 347–404. doi: 10.1016/j.ocemod.2004.08.002
Shimizu M., Isoda Y. (1998). 10. Numerical Simulations of the Transport Process of Walleye Pollock Eggs Into Funka Bay. Memoir. Faculty. Fisher. Hokkaido. Univ. 45, 56–59.
Smart T. I., Duffy-Anderson J. T., Horne J. K., Farley E. V., Wilson C. D., Napp J. M. (2012). Influence of Environment on Walleye Pollock Eggs, Larvae, and Juveniles in the Southeastern Bering Sea. Deep. Sea. Res. Part II. 65, 196–207. doi: 10.1016/j.dsr2.2012.02.018
Stabeno P. J., Schumacher J. D., Bailey K. M., Brodeur R. D., Cokelet E. D. (1996). Observed Patches of Walleye Pollock Eggs and Larvae in Shelikof Strait, Alaska: Their Characteristics, Formation and Persistence. Fish. Oceanogr. 5, 81–91. doi: 10.1111/j.1365-2419.1996.tb00084.x
Takeshige A., Miyake Y., Nakata H., Kitagawa T., Kimura S. (2015). Simulation of the Impact of Climate Change on the Egg and Larval Transport of Japanese Anchovy (Engraulis Japonicus) Off Kyushu Island, the Western Coast of Japan. Fish. Oceanogr. 24, 445–462. doi: 10.1111/fog.12121
Thompson D. W., Wallace J. M. (1998). The Arctic Oscillation Signature in the Wintertime Geopotential Height and Temperature Fields. Geophys. Res. Lett. 25, 1297–1300. doi: 10.1029/98GL00950
Tian Y., Kidokoro H., Watanabe T. (2006). Long-Term Changes in the Fish Community Structure From the Tsushima Warm Current Region of the Japan/East Sea With an Emphasis on the Impacts of Fishing and Climate Regime Shift Over the Last Four Decades. Prog. Oceanogr. 68, 217–237. doi: 10.1016/j.pocean.2006.02.009
Tian Y., Kidokoro H., Watanabe T., Iguchi N. (2008). The Late 1980s Regime Shift in the Ecosystem of Tsushima Warm Current in the Japan/East Sea: Evidence From Historical Data and Possible Mechanisms. Prog. Oceanogr. 77, 127–145. doi: 10.1016/j.pocean.2008.03.007
Vörösmarty C., Fekete B., Tucker B. (1996). Global River Discharge Database, Version 1.0 (RivDIS V1. 0), A Contribution to IHP-V Theme 1 (Paris: UNESCO Press).
Yasunaka S., Hanawa K. (2002). Regime Shifts Found in the Northern Hemisphere SST Field. J. Meteorol. Soc. Japan. Ser. II. 80, 119–135. doi: 10.2151/jmsj.80.119
Yoo H.-K., Byun S.-G., Yamamoto J., Sakurai Y. (2015). The Effect of Warmer Water Temperature of Walleye Pollock (Gadus Chalcogrammus) Larvae. J. Kor. Soc. Mar. Environ. Saf. 21, 339–346. doi: 10.7837/kosomes.2015.21.4.339
Zhang C. I., Lee J. B., Kim S., Oh J.-H. (2000). Climatic Regime Shifts and Their Impacts on Marine Ecosystem and Fisheries Resources in Korean Waters. Prog. Oceanogr. 47, 171–190. doi: 10.1016/S0079-6611(00)00035-5
Keywords: Walleye pollock, East/Japan Sea, climate regime shift, larval transport, numerical model, data assimilation, particle tracking model
Citation: Kim Y-Y, Kang Y-K, Lee S-T, Jung HK, Lee CI, Kim S, Jeong KY, Byun D-S and Cho Y-K (2022) Potential Impact of Late 1980s Regime Shift on the Collapse of Walleye Pollock Catch in the Western East/Japan Sea. Front. Mar. Sci. 9:802748. doi: 10.3389/fmars.2022.802748
Received: 27 October 2021; Accepted: 28 March 2022;
Published: 03 May 2022.
Edited by:
Ying Wu, East China Normal University, ChinaReviewed by:
Kisei R. Tanaka, Pacific Islands Fisheries Science Center (NOAA), United StatesChristina Maria Hernandez, Massachusetts Institute of Technology, United States
Copyright © 2022 Kim, Kang, Lee, Jung, Lee, Kim, Jeong, Byun and Cho. This is an open-access article distributed under the terms of the Creative Commons Attribution License (CC BY). The use, distribution or reproduction in other forums is permitted, provided the original author(s) and the copyright owner(s) are credited and that the original publication in this journal is cited, in accordance with accepted academic practice. No use, distribution or reproduction is permitted which does not comply with these terms.
*Correspondence: Yang-Ki Cho, Y2hveWtAc251LmFjLmty