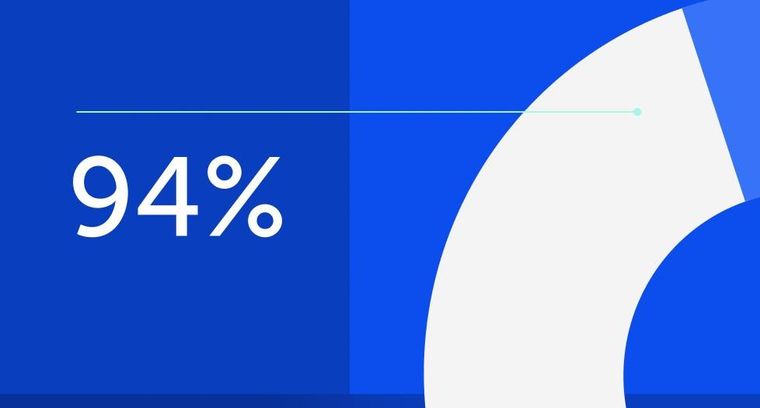
94% of researchers rate our articles as excellent or good
Learn more about the work of our research integrity team to safeguard the quality of each article we publish.
Find out more
REVIEW article
Front. Mar. Sci., 30 June 2022
Sec. Deep-Sea Environments and Ecology
Volume 9 - 2022 | https://doi.org/10.3389/fmars.2022.799191
This article is part of the Research TopicRecent and Emerging Innovations in Deep-Sea Taxonomy to Enhance Biodiversity Assessment and ConservationView all 15 articles
As one of the oldest branches of biology, taxonomy deals with the identification, classification and naming of living organisms, using a variety of tools to explore traits at the morphological and molecular level. In the deep sea, particular challenges are posed to the taxonomic differentiation of species. Relatively limited sampling effort coupled with apparent high diversity, compared to many other marine environments, means that many species sampled are undescribed, and few specimens are available for each putative species. The resulting scarce knowledge of intraspecific variation makes it difficult to recognize species boundaries and thus to assess the actual diversity and distribution of species. In this review article, we highlight some of these challenges in deep-sea taxonomy using the example of peracarid crustaceans. Specifically, we offer a detailed overview of traditional as well as modern methods that are used in the taxonomic analysis of deep-sea Peracarida. Furthermore, methods are presented that have not yet been used in peracarid taxonomy, but have potential for the analysis of internal and external structures in the future. The focus of this compilation is on morphological methods for the identification, delimitation and description of species, with references to molecular analysis included where relevant, as these methods are an indispensable part of an integrative taxonomic approach. The taxonomic impediment, i.e. the shortage of taxonomists in view of a high undescribed biodiversity, is discussed in the context of the existing large taxonomic knowledge gaps in connection with the increasing threat to deep-sea ecosystems. Whilst peracarid crustaceans are used here as an exemplary taxon, the methodology described has broad relevance to many other deep-sea taxa, and thus will support broader research into deep-sea biodiversity and ecology more widely.
The dichotomy in deep-sea biodiversity research consisting of a gap between the sheer scale of the deep sea and our incomplete knowledge of what actually lives there, is immense; areas away from the shelf edge making up the deep sea cover more than two-thirds of the Earth’s global surface, but only a tiny portion of this has been examined by scientists (Ramirez-Llodra et al., 2010; Costello and Chaudhary, 2017). It is in part because of this limited knowledge that estimates of how many metazoan species to expect in the deep sea vary widely, ranging between 0.5 to more than 10 Mio. species (May, 1992; Grassle and Maciolek, 1992; Poore and Wilson, 1993; Lambshead and Boucher, 2003; Appeltans et al., 2012). There are currently > 26,000 named species catalogued in the World Register of Deep-Sea Species (WoRDSS; Glover et al., 2021), but certainly many more are to be discovered, especially among the inconspicuous, small-size and short-ranged fractions (Mora et al., 2011).
The discovery and description of the first species from the deep sea, the sea pen Umbellula encrinus (Linnaeus, 1758), heralded the beginning of the taxonomic study of deep-sea organisms. Remarkably, this coincided with the revision of the previous classification system and the birth of modern taxonomy as introduced by Linnaeus (1735) Systema Naturae. Our knowledge of deep-sea species has been thereby closely linked, on the one hand, with the ever-improving technology and logistics for taking samples from the deep sea and, on the other hand, with methodological advances to make external and internal parts of organisms visible. Here, the invention of the first compound microscopes towards the end of the 16th century had pushed taxonomic work forward considerably since it allowed to study the smaller size fractions and thus greatly increased the number of known species (Rosenthal, 2009; Manktelow, 2010). Regarded today as art, the detailed scientific illustrations of taxonomists at the earliest time such as Carl Linnaeus (1707–1778), Alexander von Humboldt (1769–1859), Ernst Haeckel (1834–1919), or Georg Ossian Sars (1837–1927) were indispensable in the absence of the photographic imaging techniques available today (Figures 1A–G). Isolated deep-sea samples had already been collected prior, but it was only 150 years ago that a global collection as part of the HMS Challenger Expedition (1872–1876) could refute the thesis that the deep sea is devoid of life (Murray and Renard, 1891). Research into deep-sea biodiversity has gradually shifted from a more exploratory focus that involved a mere inventory of species to a more systematic approach that addresses issues such as how deep-sea diversity is structured. Likewise, taxonomy, as a legacy of Charles Darwin (1809–1882), Ernst Haeckel and more recently the German systematist Willi Hennig (1913–1976), has made a transition from classifying taxa based on their morphological appearance (phenetics) to using homologous characters to illuminate phylogenetic relationships (cladistics).
Figure 1 Scientific illustrations of peracarids as complement of taxa description from past to present. (A) Isopod genus Astacilla Cordiner, 1793 illustrated by Cordiner (1793). (B) Mesopodopsis slabberi (van Beneden, 1861), the earliest illustrated mysid by Slabber (1778). (C) Diastylis scorpioides (Lepechin, 1780), the earliest published illustration of a cumacean as Oniscus scorpioides Lepechin, 1780 (see Holthuis, 1964). (D) Diastylis scorpioides (Lepechin, 1780), illustrated by G.O. Sars more than one century later (G.O. Sars, 1900). (E) The amphipod Pardalisca abyssi Boeck, 1871 illustrated after the voyage of H.M.S. Challenger during the years 1873–76 (Stebbing, 1888). (F) Original hand inked drawing made by Roger Bamber for the description of the tanaid Zeuxo holdichi Bamber, 1990. (G) Original plate outline with the drawings made by Édouard Chevreux for the amphipod description Pontogeneia minuta Chevreux, 1908 (Crustacean collection MNHN). (H) Compound microscope equipped with camera lucida to draw specimens for taxonomical purposes (photo I Frutos). (I) Preparing a plate by hand inking from previously made pencil drawings (photo I Frutos). (J) Electronical inking of drawings using a drawing tablet and computer (photo I Frutos).
To date, referring to morphological features is still the means of choice when delimiting, identifying and describing deep-sea species. This is likely because it seems easy to apply, and others, such as the biological species concept sensu Mayr (1942; “Species are groups of interbred natural populations reproductively isolated from other such groups”) cannot readily be applied due to the difficulty to obtain data on reproduction of deep-sea species (see also Brandt et al., 2012). With the advent of molecular approaches in taxonomy in general and deep-sea taxonomy in particular, however, many complications are associated with the phenotypic data, including evidence of sexually dimorphic or polymorphic species, convergence, and phenotypic plasticity (Raupach and Wägele, 2006; Vrijenhoek, 2009; Błażewicz-Paszkowycz et al., 2012; Brandt et al., 2012; Riehl et al., 2012; Błażewicz-Paszkowycz et al., 2014; Brandt et al., 2014; Mohrbeck et al., 2021). While molecular techniques have certainly helped expedite species identification and delimitation, phylogenetic relationships and biodiversity assessment, also on the background of intensifying anthropogenic impacts on deep-sea ecosystems, the description and naming of species remains pivotal to understanding their ecological function and evolution. Traditional taxonomy, however, in general cannot keep up with automated, high-throughput molecular methods that generate large amounts of data at a rapid pace, resulting in a large number of unnamed species on taxonomists’ shelves, which remain unavailable for conservation purposes (Pante et al., 2015; Gellert et al., 2022). Moreover, for many (and not only) biologists, species identification also reduced to the pragmatic ability to distinguish between species remains far from a satisfactory solution. The simple curiosity to know and understand biodiversity in every detail at different levels of life organization, as well as the search for answers to how and why, goes beyond rapid and precise species identification (Will et al., 2005; Wheeler, 2018; Dupérré, 2020).
In that regard, morphological techniques used in deep-sea taxonomy did not stand still, but are constantly being further developed or have been introduced as new applications. For example, Confocal Laser Scanning Microscopy (CLSM) was originally developed in the 1950s to map the anatomy of the human nervous system and is now increasingly being used for the taxonomic analysis of microscopic invertebrates in the deep sea (Michels and Büntzow, 2010; Brandt et al., 2014; Meißner et al., 2017; Martínez Arbizu and Petrunina, 2018; Jennings et al., 2018; Kaiser et al., 2018; Błażewicz et al., 2019; Chim and Tong, 2020; Kaiser et al., 2021; Demidov et al., 2021). 3-D visualizations of internal structures are reconstructed from histological sections (Neusser et al., 2016; Bober et al., 2018; Gooday et al., 2018). Underwater Hyperspectral Imagery has been employed to aid identification of deep-sea megafaunal species owing to their specific spectral profiles alongside automated tools for the annotation of benthic fauna from video or still imagery (Langenkämper et al., 2017; Dumke et al., 2018; Kakui and Fujiwara, 2020; Singh and Mumbarekar, 2021).
The remit of this review article is to compile and evaluate available traditional and modern tools and techniques in morphology-based taxonomy with a focus on peracarid crustaceans. With more than 21,000 described species, the malacostracan superorder Peracarida is a highly diverse group containing about a third of the total richness of crustaceans (Appeltans et al., 2012; Wilson and Ahyong, 2015). Common to all peracarids is brood care, whereby embryos are carried around in a ventral brood pouch formed by coxal oostegites until juveniles are released. Peracarids occur in all aquatic habitats, including caves, freshwater, stygobiont and marine environments, but only the oniscidean isopods contain truly terrestrial species. Besides extant species, they have occurrences in the fossil record, including deep-sea areas (Secrétan and Riou, 1986; Selden et al., 2016; San Vicente and Cartanyà, 2017; Luque and Gerken, 2019). Spanning different size classes, from meio- to megafauna, the highest diversity of peracarids is likely to be found within the macrofauna, where they represent one of the most diverse groups in the deep sea (Hessler and Jumars, 1974; Sanders et al., 1985; Frutos et al., 2017a; Brandt et al., 2019; Washburn et al., 2021). Peracarids are the main component of suprabenthos, which includes all swimming bottom-dependent animals performing, with varying amplitude, intensity, and regularity, seasonal or daily vertical migrations above the seafloor (Brunel et al., 1978; Frutos et al., 2017a; Ashford et al., 2018). Most species of deep-sea peracarids are benthic, with tanaidaceans and some isopod taxa living mostly infaunally, whilst many amphipods, isopods and cumaceans are known as good swimmers (Błażewicz-Paszkowycz et al., 2012; Poore and Bruce, 2012). Shrimp-like mysids and lophogastrids similarly have good swimming capacities, representing members of suprabenthic (mysids) and pelagic (lophogastrids) communities (San Vicente et al., 2014a). Although the variety of lifestyles, morphologies and functions of deep-sea peracarids is large, with some exceptions, a general suite of taxonomic working methods can be applied to their study (including the study of some fossil specimens).
This review is intended to describe the entire process required for the morphological examination of deep-sea peracarids, from deep-sea sampling to long-term storage in historical collections. The focus is on fixation and conservation for microscopy as well as the selection and application of imaging techniques. Although this compilation is dedicated to the morphological analysis, recommendations for sample preparation are also given with regard to genetic/omic studies as part of an integrative workflow. Given the great diversity of peracarids in the deep sea, we hope that this overview will find broad application and importance in exploring the cornerstone of any biological research there, the species.
Deep-sea science is indisputably expensive and logistically difficult. Study areas are usually far away from the coast, sampling itself takes long hours, and apart from vents or seeps, faunal densities are typically low. Moreover, the ship-time costs, the effort and number of people involved to get a sample, with all the physical difficulties to successfully work at great ocean depth, make deep-sea material very precious. While this is common sense, prior to sampling consideration should therefore be given to how best to sample, process and fix samples simultaneously for various purposes (e.g., morphological, molecular, ecological and biochemical) in order to get the most out of the material. At the same time, media and methods for long-term storage need to be evaluated so that the vouchers and slides are retained for future work. A full representation of the described workflow of sample collection and processing is shown in Figure 2.
Figure 2 Workflow to illustrate all steps that are required for the taxonomic investigation of the deep-sea peracarid fauna under the cold chain regime (Riehl et al., 2014) - from sampling, morphological taxonomic investigation, molecular and biogeographic analysis to the final storage of samples and data. Links to: OBIS, Ocean Biogeographic Information System1; GBIF, Global Biodiversity Information Facility2; DeepData, Deep Seabed and Ocean Database of the International Seabed Authority3; WoRMS, World Register of Marine Species4; WoRDSS, World Register of Deep-Sea Species5; BoLD, Barcode of Life Data System6; and Genbank7. (1https://obis.org/; 2https://www.gbif.org/; 3https://data.isa.org.jm/isa/map/; 4http://www.marinespecies.org/; 5http://www.marinespecies.org/deepsea/; 6https://www.boldsystems.org/; 7https://www.ncbi.nlm.nih.gov/genbank/).
Basically, two ways of collecting data are common: 1) still or video imagery in situ, and 2) direct sampling (Schiaparelli et al., 2016). Identification to the species level using images is difficult or even impossible for the megafauna (Hanafi-Portier et al., 2021; Horton et al., 2021), so that ex-situ examinations are required or even mandatory for the mostly much smaller Peracarida. The majority of deep-sea peracarids are sediment-bound, i.e. living in, on or just above the seabed (suprabenthic lifestyle). Depending on lifestyle and mobility of the target organisms, a variety of benthic sampling devices are used in deep-sea research. On soft bottoms, in general, coring devices, including box corer, multi- and megacorer, collect epi- and infaunal species; towed apparatus (trawls, sledges and dredges) is used for the epi- and supra-fauna; as well as baited and sediment traps, for the collections of more mobile and/or pelagic species. Manned submersibles or remotely operated vehicles (ROV) can help in the sample collection by means of push-corer, suction pump, small nets or picking up larger structures on hard substrata (for sampling specificities see Jamieson, 2016; Kaiser and Brenke, 2016; Kelley et al., 2016; Narayanaswamy et al., 2016; Frutos et al., 2017a). In water column studies, pelagic peracarid species are collected by means of mid-water trawls or plankton nets (Kürten et al., 2013; MacIsaac et al., 2014; Papiol et al., 2019); the latter are also suitable as collector of benthic peracarids if they are used as additional sampler attached to trawling devices such as otter or beam trawls (Nouvel and Lagardère, 1976; Lagardère, 1977). In addition, peracarids can also be sampled indirectly by examining the gut content of decapod or the fish stomach content, because they are their food source (Sorbe, 1981; Carrasón and Matallanas, 2001; Preciado et al., 2017). The advantages or disadvantages for the use of the aforementioned types of sampling devices are summarized in Table 1; however, an optimal choice is the combination of different equipment types to sample (Taylor et al., 2021; Ríos et al., 2022), which also provides complementary information on species behavior (Frutos and Sorbe, 2010; San Vicente et al., 2014b).
The choice of sampling devices depends on the target taxon (with regard to size class and lifestyle), seafloor topography, substrate type and depth, as well as data requirements (qualitative vs. quantitative). Benthic sledges are useful, for instance, to collect specimens with high swimming capacities (i.e. mysids and lophogastrids; Frutos, 2006), as well as relatively high specimen numbers, and thereby enable more coherent morphological and genetic assessment. Although sledges provide large numbers of peracarid fauna, additional equipment (such as opening/closing system of nets, flowmeters or pingers in the sledge frame) is required to better express abundances as densities (Brunel et al., 1978; Sorbe, 1983; Cartes et al., 1994; Dauvin et al, 1995; Frutos, 2006; Frutos et al., 2017a). Corers, by contrast, only provide low faunal densities, but offer quantitative insights when collecting undisturbed sediment surfaces (Jóźwiak et al., 2020; Lins and Brandt, 2020).
In all cases, minimizing mechanical damage to the specimens during sampling and processing to avoid loss of taxonomic information, and considering different preservation options for the same sample are important considerations. On the one hand, this includes careful handling during sampling and sample processing (washing and sieving), but also swift storage of the samples, especially if genetic or biochemical analyses are to be carried out. For example, precautions should be taken for trawled devices prior to sampling to avoid hard substrate entering the nets and grinding individuals (Kaiser and Brenke, 2016). Since sediment is part of the sample, it is important to remove it by sieving to maximize fixative concentration and thus improve sample preservation. As crustaceans can easily lose their legs and antennae, which is often essential for taxonomic identification, sediment samples should therefore be carefully sieved, if necessary with prior elutriation of the sediment samples in seawater.
Processing the samples for different purposes needs specimens to be removed from the sediment as soon as possible after the arrival of the sample on deck. Here, the maintenance in high ethanol content may arguably be even more crucial for genetic analysis (see 3.2.1 Light Microscopy) than to maintain a cold chain protocol. The latter has been thought to be essential for molecular work on deep-sea isopods (Riehl et al., 2014). For sampling under tropical climatic conditions, however, it is strongly recommended that the samples are transferred to a cold environment as soon as possible. A disadvantage of fixing the entire sample in ethanol, however, is that the tegument/cuticle of the peracarids becomes hard and stiff and could impede further morphological examination (e.g. of subcuticular elements), while the setae required for morphological determination, become brittle and can break off. Furthermore, some morphological features can only be observed in live (unfixed) specimens. For example, in deep-sea amphipods, optical structures often can only be visualized in live animals: Leucothoe cathalaa is showing the whitish pigmentation of the rounded eye before storage in preservative medium (Figure 3E, while its eyes are hardly visible in preserved specimens, even under light microscope (Frutos and Sorbe, 2013). Equally, samples that are to be frozen, e.g. for biochemistry studies, should be identified as accurate as possible and pictured before being preserved. Thus, live sorting should be considered, whenever possible, whereby the respective individuals are selected directly from the sample and individually identified, photographed and fixed (Brix et al., 2020; Ahyong et al., 2022).
Figure 3 Peracarida specimens visualized applying different modern imaging techniques to complement the taxonomical description of species. (A–G) Digital still camera on stereomicroscope. (H, I) Still camera. (J, K) dissected specimen under Light microscope. (L–N) Scanning Electron Microscope. (O–R) Confocal Laser Scanning Microscope. (S) Microcomputed tomograph. (A) The paranarthrurellid tanaidacean Armatognathia swing Błażewicz and Jóźwiak, 2019 from Błażewicz et al. (2019) under Creative Commons license. (B) The mysid Paramblyops rostratus (Holt and Tattersall, 1905) from Frutos (2017). (C) The ischnomesid isopod Cornuamesus longiramus (Kavanagh and Sorbe, 2006), and (D) the diastylid cumacean Campylaspis vitrea Calman, 1906 from Frutos et al. (2017a). (E) The leucothoid amphipod Leucothoe cathalaa Frutos and Sorbe, 2013 from Frutos and Sorbe (2013). (F) The first asellote isopod from the fossil record Fornicaris calligarisi Wilson and Selden, 2016 from Selden et al. (2016),© The Crustacean Society, reprinted with permission of Oxford University Press on benhalf of The Crustacean Society. (G) The oldest crown cumacean Eobodotria muisca Luque and Gerken, 2019 from Luque and Gerken (2019), reprinted with permission of Royal Society Publishing. (H) The oldest known fossil mysid Aviamysis pinetellensis San Vicente and Cartanyà, 2017 from San Vicente and Cartanyà (2017), reprinted with permission of Cambridge University Press. (I) Two fossil lophogastrids of family Lophogastridae from Secrétan and Riou (1986), reprinted with permission of Annales of Paléontologie. (J) The eusirid amphipod Dorotea papuana Corbari, Frutos and Sorbe, 2019 from Corbari et al. (2019). (K) The paranthurid isopod Paranthura santiparrai Frutos, Sorbe and Junoy, 2011 from Frutos et al. (2011). (L) The nannoniscid isopod Austroniscus obscurus Kaiser and Brandt, 2007 from Kaiser and Brandt (2007). (M) The paramunnid isopod Pentaceration bifficlyro Kaiser and Marner, 2012 from Kaiser and Marner (2012). (N) The paranthurid isopod Paranthura santiparrai Frutos, Sorbe and Junoy, 2011 from Frutos et al. (2010). (O) The oedicerotid amphipod Oedicerina teresae Jażdżewska, 2021 from Jażdżewska et al. (2022) under Creative Commons license. (P) The nannoniscid isopod Thaumastosoma platycarpus Hessler, 1970 from Kaiser et al. (2018). (Q) The nannoniscid isopod Nannoniscus magdae Kaiser, Brix and Jennings, 2021 from Kaiser et al. (2021). (R) The paranthrurellid tanaidacean Paranarthrurella arctophylax (Norman and Stebbing, 1886), from Błażewicz et al. (2019) under Creative Commons license. (S) Fossil lophogastrid specimen showing internal anatomy after microcomputed tomography, from Jauvion (2020).
Fixation of specimens in taxonomic studies aims to prevent the spontaneous deterioration of taxonomically important features of the collected animals and thus its methods should be selected and applied with a thorough regard for the subsequently planned discovery pipeline of methods. The two main threats to morphological and genetic features of marine crustaceans that have to be prevented by fixation are dead cell/tissue autolysis by endogenous enzymes and destruction of biological material by microbial (bacterial/fungal) contaminants. An optimal fixative should aim to prevent both threats at the same time. Specimen fixation is of paramount importance if a significant time lapse occurs between collection and analysis, which is usually the case for marine samples, especially deep-sea ones, collected on board of research vessels and later analyzed in research institutions on dry land. In fact, the current average shelf life of new species between discovery and description is about 21 years (Fontaine et al., 2012). Furthermore, good preservation is also extremely important for material of taxonomic significance, especially type material that has to be available for subsequent re-analysis in museum collections. While the term “preservation” is usually used for application of fixatives for prolonged storage of museum specimens, both underlying principles and specific compounds used are analogous to fixation for general purposes and will be discussed together here.
Fixation inevitably changes the physico-chemical properties of the specimen, so it has to be performed in a way that is compatible with downstream taxonomic techniques, both with regard to imaging morphology for identification purposes and to analyzing genetic and biochemical make-up of the specimen. Thus, selection of proper fixative is always a trade-off between efficiency and durability of preservation on one hand and lack of significant interference with taxonomically important features of the specimen (Eltoum et al., 2001). Among the properties that need to be considered are i.e.: crude shape changes which may result from physico-chemical processes (drying, osmotic swelling); delicate morphological elements that may be damaged during the fixation process itself; physical features that may deteriorate upon chemical reactions with the fixative, especially upon prolonged exposure (color, transparency, flexibility, malleability etc.); biochemical composition (e.g. lipid or carbohydrate content of specific tissues); integrity of nucleic acids and their accessibility to isolation; antigenic properties and/or enzymatic activity of proteins (Barbosa et al., 2014). With regard to deep-sea biological investigations, another consideration that has to be taken into account is the availability of fixative at the collection site: this includes questions of logistics (ease of transport, security), legal issues, shelf life of the fixative itself etc. Sometimes, a two-tier fixation protocol may be adopted, with simpler fixative applied on board the collection vessel for short-term preservation and subsequent exchange for museum-grade fixative during preparation for long-term storage in a biological collection. Of course, taxonomists are often confronted by the fact that the specimens to be examined have not been collected and preserved by themselves, so they no longer have a choice of fixation method, but some fixatives can be exchanged for others (e.g. ethanol can be replaced with formaldehyde and vice versa) prior to analysis if interference is expected (Pereira et al., 2019). As the published literature is contradictory about the compatibility of some fixation protocols with subsequent taxonomic analysis (especially by nucleic acid isolation, PCR and/or next generation sequencing) and anecdotal evidence for the suitability of individual protocols prevails, taxonomists are recommended to understand the physico-chemical principles of fixation and of genetic methods, so that an informed decision may be made. A classification of the fixatives most commonly used in the Peracarida taxonomic community and short description of their main advantages and disadvantages is included in Table 2.
Table 2 The most common types of fixatives used by peracarid taxonomists with their advantages and disadvantages summarized.
In some cases, taxonomic studies are performed not on specimens from extant taxa collected while still alive, but on subfossil or fossil material which is already naturally “fixed” or transformed into a relatively permanent, physico-chemically stable form. Morphology of preserved tissues may be studied in such samples using the same imaging techniques as described below for extant material – optical microscopy, electron microscopy or microcomputed tomography (Sánchez-García et al., 2016; Nagler et al., 2017; Jauvion, 2020; Luque et al., 2021; Robin et al., 2021), but the physical preparation of the sample lacks the fixation step, instead involving mechanical preparation (slicing, milling, polishing). For some taxa of deep-sea Peracarida, morphological studies of fossils using recently available imaging techniques led to taxonomic corrections and reclassification of whole groups of specimens: a decapod tail described as amphipod (McMenamin et al., 2013; Starr et al., 2016); samples that upon close investigation contained not amphipods but previously unknown genera and species of tanaids (Vonk and Schram, 2007); a new mysid genus (Cartanyà, 1991; San Vicente and Cartanyà, 2017) or a new lophogastrid taxon (Secrétan and Riou, 1986; Jauvion, 2020).
The most common fixative types in aquatic zoology can be classified into two groups: those relying on quick dehydration and those relying on molecular cross-linking of biochemical components. Both aim to quickly and efficiently inhibit the activity of enzymes (endogenous or microbial ones) which could destroy the biological macromolecules that the specimen consists of: proteases for proteins, nucleases for nucleic acids or glycosidases for carbohydrates. Dehydration withdraws the main reaction substrate for hydrolytic reactions and inactivates enzymes by coagulation-mediated denaturation. Cross-linking prevents enzyme-substrate interactions by stopping diffusion as well as by preventing conformational changes of the enzyme molecule that are crucial for its activity. Some fixation methods aim also to inhibit major lytic enzyme groups by specific biochemical interactions with their co-substrates or active sites, or to target microbial life with antibiotic toxins (Table 2).
The most universal and frequently used fixatives based on the dehydration principle are aliphatic alcohols, especially ethanol. Ethanol works by quickly mixing with water, penetrating the specimen, and removing the solvation shells from proteins and other molecules. The most efficient and rapid-acting concentration of 95–96% is considered the optimal fixative both for field fixation and long-term storage when preservation of tissue structure, biochemical composition and DNA for genetic analysis are important (Palero et al., 2010; Wetzer, 2015; Martin, 2016; Beninde et al., 2020).
While 70% ethanol is also historically used for long-term storage in museum collections due to its superior anti-microbial activity, numerous studies have shown that the increased water content and insufficient lytic enzyme inhibition leads to detectable levels of DNA degradation, correlating with storage time and therefore making subsequent genetic studies on material stored in the manner more difficult – especially for taxonomically valuable material (e.g. type specimens) (Marquina et al., 2021); moreover, the high-water content and lowered pH of 70% ethanol may lead to cuticle decalcification upon long-term storage, which is important especially for those peracarids that have taxonomically important calcium carbonate deposits in different forms (amorphous, calcite, aragonite) in the exoskeleton, e.g. isopods. On the other hand, rapid and complete dehydration by concentrated ethanol has the disadvantage of making arthropod exoskeletons stiff and brittle, as their natural elasticity depends to a large extent on extracellular matrix proteins which lose their properties when denatured/coagulated by water loss, leading to mechanical damage in transport or during dissection (Costa et al., 2021). The fragility of tegument is especially problematic in the case of some deep-sea Peracarida where delicate appendages and armament are often essential for taxonomic identification – therefore, an addition of up to 5% glycerol (by volume) during fixation and preservation would be strongly recommended as it softens the exoskeleton and makes it less fragile. In some cases, the tegument may also become opaque due to coagulated protein precipitation, hampering internal observation (e.g., of musculature or gut content), and taxonomically important pigmentation may be partially or totally dissolved, e.g. making eyes difficult to notice visually (Frutos and Sorbe, 2013; Campean and Coleman, 2018). Therefore, while 95% ethanol remains the optimal concentration for on-site fixation and long-term storage, it may be preferably exchanged for 70% ethanol in sample transit and before laboratory manipulations. Absolute (~100%) ethanol is much more expensive than 95% ethanol and may sometimes introduce microscopic morphological artefacts due to its extreme hygroscopy.
Methanol, while used in histological fixation, is ineffective for long-term storage of specimens for taxonomic purposes and should be avoided since its dehydration power is relatively weak, leading to insufficient protein coagulation and residual lytic activities. Isopropanol is as efficient in protein coagulation as ethanol and does not stiffen carbohydrate structures (carapaces) as much, but this advantage is offset by its relatively high price and slow diffusion into larger biological structures, leading to potential loss of fine details or DNA contained in internal structures (King and Porter, 2004).
Despite prevailing misconceptions in literature about ethanol with additives that make it unsuitable for human consumption (so-called denatured alcohol), these additives (e.g. methanol, ether or acetone) have no discernible effect on the fixation process, long-term preservation and downstream applications (when nucleic acids are isolated for genetic analysis, these additives are removed together with ethanol itself, and they are present in far too low concentrations to impact downstream processes anyway). The same is true for traces of benzene or its derivatives present in absolute ethanol. The misplaced recommendations against using denatured alcohol for specimen preservation for genetic analysis stem from faulty interpretation of several studies where “pure ethanol” at 95% was compared to “denatured alcohol” at 70% (as this is the concentration readily available commercially in many countries), and the above-mentioned inferior performance of the latter in DNA preservation was mistakenly ascribed to the denaturing additives (Wall et al., 2014). If denatured 95% ethanol is available, it may be used for fixing deep-sea Peracarida equally to pure 95% ethanol. The main advantages of ethanol as a fixative for taxonomy of deep-sea Peracarida include: low cost, fast action, potential for long-term storage, good preservation of DNA and proteins (including linear antigenic determinants). The main disadvantages include: high volatility (and therefore potential for evaporation from non-hermetic storage containers), flammability, legal issues (especially with transport to the collection site), need for time-consuming removal for some downstream applications (especially involving nucleic acid isolation), potential for morphological distortion by rapid water removal from small specimens with delicate exoskeletons, as well as fragility of dehydrated specimens.
The most frequently used cross-linking fixative is formaldehyde which reacts with proteins, nucleic acids as well as some lipids and carbohydrates to form a durable network of covalently linked macromolecules. For long-term storage, formaldehyde is usually used at concentration of 4% (or sometimes higher). The working solution is obtained by diluting so-called formalin (stabilized concentrated solution of ca. 36%) or by de-polymerizing the solid polymer paraformaldehyde. Formaldehyde penetrates tissues quickly and preserves structures efficiently, while not dehydrating the specimen at the molecular level, leading to full preservation of flexibility of appendages and tegument, making dissection easy. Since aquatic solutions of formaldehyde are acidic (due to hydrolysis and forming of geminal methanediol), it is crucial that this fixative is buffered to neutral or slightly basic pH (7.5–8.5) when used on marine crustaceans if biochemical integrity of the tegument is to be preserved, to prevent dissolution of calcium carbonate in their exoskeleton. The most frequently used buffering agents for this task are sodium borate (borax), sodium phosphate, sodium bicarbonate and hexamethylenetetramine (urotropin) (Presnell and Schreibman, 1997; Martin, 2016). On the other hand, decalcification in acidic formaldehyde solutions makes some tegument more transparent, allowing for easier microscopic observation of internal structures. For small aquatic animals with shells or carapaces, formaldehyde is sometimes combined with compounds that accelerate protein coagulation during the initial specimen soaking (picric and acetic acids) - this fixative is called Bouin’s solution and may be recommended where careful preservation of deep tissue morphology is of importance. An alternative for formaldehyde is the higher molecular weight bifunctional molecule, glutaraldehyde, which forms more stable and durable crosslinks, but is much more expensive, makes tissues hard and difficult to dissect and prevents any subsequent molecular analysis. The advantages of formaldehyde for peracarid taxonomy, especially used in commercial and monitoring studies, include: low cost, fast action, capacity for long-term storage (low volatility). The main disadvantages are: high toxicity (which necessitates careful handling, especially during transport), strong biochemical changes which are sometimes irreversible (DNA and RNA may be isolated from formaldehyde-fixed specimens after de-crosslinking, but it is of significantly lower quality; while some proteins retain antigenic properties, some do not), deterioration of some physical features of the specimen (tissue hardening, “tanning” - generation of secondary pigments), deformation of microscopic features by spontaneously precipitating paraformaldehyde crystals. It has been demonstrated that formaldehyde-crosslinked nucleic acids are more labile to hydrolysis, which is why they yield worse quality sequencing data; de-crosslinking is most efficient at 70°C in dilute buffer at pH=8.0 (Evers et al., 2011).
A historically common preservation technique for short-term maintenance of collected specimens until the availability of more efficient fixative is refrigeration or freezing of sample in the seawater in which it was collected. Refrigeration does not stop degradation processes, it only slows them down, while freezing (e.g., flash-freezing in liquid nitrogen) strongly disrupts microscopic morphology owing to generation of ice crystals within tissues, so these methods are recommended only when the main purpose of material collection is biochemical analysis in the near future.
While ethanol works by dehydration at the molecular level, water may be removed from the specimen also physically by drying (spontaneous, heat-induced or using hygroscopic materials such as silica gel). While common as a preservation procedure in terrestrial arthropods, this method is of highly limited applicability for marine peracarids: morphology is strongly disturbed by the drying process itself and by marine water salts, dry specimens are extremely delicate with regard to mechanical damage, inhibition of lytic enzymes and microbial growth is inefficient, nucleic acid chains tend to break. The only exception is preparation of specimens for SEM where liquid needs to be removed while preserving micromorphology – freeze-drying (lyophilisation) or critical point drying in liquid carbon dioxide are the fixation methods of choice here.
Organic solvent-based dehydrating fixatives, which are commonly used in histology, are also sometimes applied for preservation of marine crustaceans, although this is mainly of historical significance and should be discouraged for modern taxonomic analysis. Specifically, acetone or Carnoy’s solution (ethanol with chloroform and acetic acid) dissolve and wash out hydrophobic components of the specimen, including biological membranes and lipid pigments, much more strongly than ethanol, preserving only the crude external structures (e.g. the exoskeleton), which is not acceptable for museum-quality preservation.
A group of less frequently used fixatives are inorganic salt coagulants involving heavy metals that act on negatively charged groups in proteins and lipids. Osmium tetroxide is an efficient fixative for lipid-rich tissues, but its application for crustaceans is mostly limited to concurrent fixation and staining for electron microscopy (see below). Similarly, in some histological work on marine crustaceans, Zenker’s fixative is used. This solution contains highly toxic mercuric chloride acting as coagulant and providing excellent tissue fixation for detailed histological analysis. Its usage nowadays is limited, since it has to be handled with extreme care and produces hazardous waste that requires costly disposal.
Sometimes, antimicrobial additives (amphothericin, thimerosal, azide etc.) are used to prevent microbial contamination and degradation of the sample, but as they usually have a relatively narrow spectrum of action and do not influence the spontaneous degradation of dead tissue by endogenous enzymes, they can have an auxiliary function at best.
Several specialized fixatives have been developed for specimens destined for subsequent nucleic acid isolation and genetic analysis. While RNA is both inherently unstable and subject to degradation by ubiquitous and abundant RNAses, DNA (a more common object of genetic analysis for taxonomic purposes) is chemically very stable, degrading only under specific conditions, and its deterioration in unfixed specimens is mostly due to action of microbial digestive enzymes because tissues of marine invertebrates are very poor in endogenous nucleases. Thus, while commercial fixatives like RNAlater™ and other chaotropic salt-based protein denaturants aimed at rapid and efficient elimination of RNAse activity are crucial to any transcriptomic (RNA-based) analysis, they are very expensive and simpler fixatives (like ethanol) are just as efficient in DNAse inhibition if only DNA-based analysis is foreseen. Alternatives to ethanol as a fixative for DNA-based studies have been proposed (e.g. propylene glycol-containing antifreeze solution (Robinson et al., 2021) or solutions containing metal chelators that deprive DNAses of cofactors mixed with detergents (Pokluda et al., 2014) or polar solvents (Lins et al., 2021) and they facilitate subsequent DNA isolation, but they are not efficient in preserving morphology or in long-term prevention of microbial contamination, so they should be used only in targeted taxonomic studies (e.g. barcoding or metabarcoding). When selecting the fixative for a specimen that will (or may) be subjected to genetic analysis by DNA sequencing, it is important to take into account the specific technique to be used: some techniques (e.g. Illumina) sequence short fragments and thus may be efficiently used even on DNA of low quality, e.g. isolated from formaldehyde-fixed specimens; some techniques (e.g. nanopore) need long DNA molecules and thus should be applied only for material fixed with ethanol or DNA-specific fixatives. Importantly, both freeze-thaw cycles and drying-rehydration cycles contribute to DNA strand breakage and should be avoided if longer DNA is required.
Body length of peracarids rarely exceeds several millimeters. For this reason, the morphological identification of the peracarids involves observation of the details of head/cephalothorax, thorax, and abdomen appendages as well as additional components such as labrum, labium or epignath. The dissection of microscopic size requires experience, “surgical” dexterity, and precise tools. The needles used for the preparation of larger crustaceans are much too large for working with small crustaceans, while thin entomological needles are too flexible for dissection of the crustaceans. Tungsten needles, with tips although extremely fine, remain rigid and inelastic, are an ideal solution for peracarid dissection. Nowadays there are many companies on the market that offer tungsten needles, but sharpening can also be done in the lab, using solution of KOH, as copper as cathode and a low electric voltage.
Scientific drawings are the pillar of taxonomic research. Drawing practiced with the support of a camera lucida microscope enable future researchers to recognize named species (Figure 1H). In the early Linnean days of taxonomy, it was essential to prepare drawings to visualize features, but recently they are increasingly being replaced by other (e.g., photographic) techniques (Wilson, 2003; Anderson, 2014; d’Udekem d’Acoz and Verheye, 2017; Lörz and Horton, 2021), that are also being applied to fossilised specimens (Selden et al., 2016; Jauvion, 2020). There have been fierce debates over photographs or microscopic images to become substitutes for drawings or even types (cf. Zhang et al., 2017). Although changes to the International Code for Zoological Nomenclature now have a certain consistency with regard to the type problem (Zhang et al., 2017), the idea of describing species purely based on imagery or molecular taxonomic units (MOTUs) (Jörger and Schrödl, 2013; Sharkey et al., 2021) still remains the exception for peracarids.
Drawings provide an interpretation often in a rather schematic way. The traditional scientific drawing workflow is clearly a lengthy one, starting with pencil drawings, followed by inking, scanning, as well as editing and arranging plates (Figures 1G–J). Yet, pencil and ink drawings, on the one hand, aid in-depth examination of the morphology and, on the other hand, distracting details may be omitted if they are systematically uninformative. Besides, drawing habitus of poorly calcified specimens enables us to visualize the morphological characters which cannot be well pictured by camera because of low contrast. Images, on the other hand, ideally give a precise representation of the morphological structures (also with regard to coloration and patterns, see amphipod example above), even more so with the development of high-resolution imaging techniques (Kaiser et al., 2018; Błażewicz et al., 2019; Jażdżewska et al., 2022). In addition, photography is far less subjective than creating drawings, but despite these advantages has so far rarely found its way into peracarid taxonomy.
The preparation of drawings presenting details of morphological structure has been historically/traditionally carried out by means of a camera lucida attached to the microscope. This device is a simple system of mirrors (Wollaston, 1807) which makes it possible to reproduce an object (body habitus or appendages) on a sheet of paper placed next to the microscope (Figure 1H). Despite the simplicity of its design, the camera is a relatively expensive piece of optical microscope equipment: only few optical companies manufacture them, and they are not usually exchangeable between different models of microscopes. In addition to the traditional use of camera lucida, focus-stacked microphotographs can be the baseline for drawings (Coleman, 2006) or even substitute for pencil drawings (d’Udeckem d’Acoz and Verheye, 2017; Wilson and Humphrey, 2020). Nevertheless, both camera lucida and stacked microphotographs techniques can also be applied together for producing drawings of fossils (Selden et al., 2016). The appropriate camera and acquisition software to equip the microscope are also expensive.
Microscopic images are useful to complement scientific drawings when studying rare (singleton or unique) species. While this is a general phenomenon in the description of species (Lim et al., 2012; Wells et al., 2019), it becomes particularly evident in the morphological analyses of deep-sea species including peracarids (Brandt et al., 2012; Higgs and Attrill, 2015). Drawings without dissecting parts of the specimen are often sought not to sacrifice the holotype, but it is thanks to the use of imaging techniques, chiefly non-destructive methods (such as CLSM, see below), it is possible to fill in missing gaps of morphological information. However, it is clear that not always taxonomist have access to all facilities to use such as useful techniques and methods.
So far, however, no efforts to refrain from drawings in peracarid taxonomy have been taken but, on the contrary to bring together as much information as possible (including molecular, ecological, and biogeographic) as part of an integrative process (Brix et al., 2015; Malyutina et al., 2018; Kaiser et al., 2018; Schnurr et al., 2018; Błażewicz et al., 2019; Jakiel et al., 2019; Riehl and De Smet, 2020; Kaiser et al., 2021). Above all, the use of digital drawing techniques and the corresponding software (something expensive as well) has made a significant contribution to reducing the time required for, and improving the quality of species illustrations (Coleman, 2003; Coleman, 2009; Bober and Riehl, 2014; Montesanto, 2015). However, much greater advances appear to have been achieved in the development of 3D reconstruction and imaging techniques.
Morphology (i.e., shape of the organisms and its parts) is still the most important taxonomic characteristic and thus methods of its recording and analysis – imaging methods – are crucial tools in the armory of a taxonomist of deep-sea Peracarida (Figure 3). Concentrating on imaging for taxonomic purposes, we need to differentiate the imaging of overall morphology (habitus) which may be performed without any previous zoological knowledge (Figures 3A–I), and imaging of specialized, taxonomically important features, the choice of which must be informed by accumulated knowledge and expertise. For deep-sea peracarids, where specimens are difficult to obtain (complicated logistics), available in limited numbers and thus are highly valuable, an important consideration is the distinction between imaging taxonomically important morphological features in situ (in intact specimens) versus imaging of prepared or isolated body parts (ex situ, after dissection and/or sectioning, Figures 3J, K), which may be sometimes necessary even for type specimens. When selecting imaging techniques, some thought must be also paid to the location of taxonomically distinctive features within the body of the crustacean – some techniques are exclusively suited to imaging external morphology (e.g. SEM, Figures 3L–N, or CLSM, Figures 3O–R), while others were developed specifically for imaging internal organs and hidden features (e.g. microCT, Figure 3S). Finally, a modern taxonomist must bear in mind that imaging can be used not only for purely morphological (shape-related) analysis, but specific contrast techniques are available to draw conclusions about biochemical composition of tissue elements as well as course of physiological processes which may be helpful as additional taxonomic characteristics and form an additional level of analysis (apart from morphological and genetic ones). Table 3 includes recent examples of application of specific imaging techniques which will be reviewed below to Peracarida and other crustaceans.
Table 3 Selected examples of literature references where different imaging techniques were used to study the taxonomy of peracarids and other crustaceans or were applied to visualize peracarids for non-taxonomic purposes.
While bright field light microscopy is the original method in taxonomy of any small organisms, its applicability to deep-sea Peracarida is limited by the relative lack of inherent contrast in their bodies. Light microscopy relies mainly on absorption, refraction, and dispersion of incident rays in the specimen, and marine crustaceans tend to be colorless (low absorption) and with optical refringence that is uniform and similar to surrounding seawater. While habitus imaging may be performed on whole specimens by reflected light stereomicroscopy in air (Hegna, 2010), the resulting images are poor in details and thus of low usefulness in taxonomy.
Most commonly, zoological specimens are prepared in a procedure called mounting, where the animal is placed on a glass slide in a drop of liquid and covered with another flat piece of glass (the thickness of this cover glass is adapted to the working distance of the microscope objective to be applied). Mounting has two main purposes: to prevent the desiccation-related destruction of specimen, and to provide an environment with uniform refraction properties in order to minimize image blurring due to photon scattering on phase borders. Therefore, the mounting medium for marine crustaceans must mix well and rapidly with seawater, and its refractive index should be as close as possible to that of glass (1.52). While animals can be mounted in water itself for short-term observation (e.g. on board), it evaporates quickly and a different mounting medium is needed if the specimen is to be stored as microscope slide. The most important decision in the choice of mounting medium is related to the desired permanence of the slide: specimens in non-permanent (liquid- or gel-based) media may be manipulated, moved around, remounted, or even removed from the slide for other type of analysis; permanent (solidifying) medium preserves the slide permanently in the same attitude of the specimen. Sometimes, the mounting medium includes components that have additional functions with regard to the specimen itself: clearing (optical homogenization by removal of light-scattering inclusions) and/or maceration (chemical removal of unwanted tissue, e.g. muscles inside the tegument). These components are usually acids (e.g. lactic acid) or bases (e.g. potassium hydroxide), and care must be taken not to exceed the necessary dosage and, if possible, to remove the agent before final mounting, as they may progressively destroy taxonomically important features or even the whole specimen during prolonged storage. Table 4 lists the commonly used mounting media for microscopic imaging of Peracarida with their main advantages and disadvantages.
Table 4 Advantages and disadvantages of mounting media commonly used for light-microscopy studies of peracarids.
The most common components of non-permanent mounting media used for taxonomic imaging of small marine arthropods include: glycerol (higher refractive index than water and negligible evaporation; sometimes mixed with 10% saline to facilitate mixing during slide preparation), gelatin (less recommendable as it is prone to desiccation and cracking), polyvinyl alcohol (included in the popular commercial mounting medium Mowiol and in the complex self-made medium polyvinyl lactophenol), and chloral hydrate (included together with glycerol in popularly used Hoyer’s medium, where it contributes to its high refractive index). They are often used in personally formulated mixtures based on experience and anecdotal evidence on performance – it is possible that some are more suitable for certain systematic groups of Peracarida than others, but systematic studies are lacking and it seems that subjective personal preference remains the main argument for mounting medium choice. Oil-based mounting media are also available, but rarely used for invertebrate taxonomy as they do not perform well with carbohydrate exoskeletons. Permanent (solidifying/hardening, either by physical curing or by chemical polymerisation) mounting media are also often used for museum specimen storage, but this practice prevents any further manipulation of the specimen (including potential new molecular discrimination techniques) and should be discouraged for rare type material where methodological developments in molecular studies may warrant the need for access to relatively unchanged biological material in distant future. However, permanent mounting may be recommended for long-term storage of dissected parts (e.g. appendages) which are of purely morphological value. While some resin-based solidifying media are marketed as reversible (they may be liquefied by heating with an excess of solvent), both morphological structure and biochemical composition is usually compromised by such treatment and all solidifying mounting media should be treated as permanent. The most common base ingredients of solidifying mounting media used in taxonomy of Peracarida include natural resins (Canada balsam, Euparal and others that solidify by gradual solvent evaporation and vitrification), synthetic resins (included in such preparations as DPX or Permount) and formaldehyde-based polymers (mainly dimethylhydantoin formaldehyde – DMHF – which is recognized as superior to resins due to much less cracking and bubbling artefacts; Bameul, 1990).
If the entire or dissected specimen is to be preserved in long-term storage in the form of microscope slide mounted in liquid medium, this slide must be also sealed using impermeant sealants that isolate the specimen from external moisture and oxygen (numerous commercial products are available, e.g. based on linseed oil, plant resins, paraffin or acrylic glue; even simple nail varnish may be used for this purpose, but care must be taken that its components do not interfere with any staining that was applied) (Allington-Jones and Sherlock, 2007). When considering long-term storage in non-permanent mounting media, the question of microbial contamination potential must be also taken into account: glycerol-based media are most resistant to contamination, while microbes grow most easily in those containing gelatin. Since the function of the mounting medium requires the compounds involved to thoroughly permeate the specimen, it needs to be extensively washed if it is required at some later point to release it from the slide after microscopy for some other (e.g. genetic) analysis. Common liquid mounting media (e.g. glycerol-based) do not damage nucleic acids and can be removed by washing, but polymerizing permanent mounting makes isolating DNA from the sample impossible.
For transmitted light imaging, the standard procedure is to stain the specimen with light-absorbing dyes to create contrast. In current practice for taxonomic purposes, researchers aim to use non-selective stains to visualize most tissue types and structures (in crustaceans, the most important element being usually the exoskeleton and its outgrowths, especially on the appendages). The most commonly used dyes are hematoxylin (which stains nucleic acids – and thus living tissue – dark blue) (Hegna, 2010) and eosin (which stains most biological macromolecules, including those in the extracellular matrix and exoskeleton, pink), most often combining these two as counterstains (Žnidaršič et al., 2018). Other, more selective dyes can also be used to stain crustaceans, including azure II (stains polysaccharides, including cuticle components), alizarin red (stains calcium deposits in calcified carapace), chlorazol black (basic dye that stains anionic macromolecules, mainly nucleic acids), alcian blue (basic dye for acidic glycans in connective tissue), toluidine blue, lignin pink (both glycan-selective stains with differing affinity) or even the non-selective India ink that stains by physical interactions. Specimens stained using these techniques are usually mounted by immobilization on standard microscope slides, but sectioned or dissected samples may be also prepared after staining. Image is recorded by photographic cameras attached to standard light microscopes or even simply by drawing (see Preparing Drawings). If a specimen stained with a cationic dye is to be subsequently used for DNA isolation, an additional washing step may be included to remove the bound dye which might impact downstream reaction efficiency. Some fluorescent DNA-binding (intercalating) dyes (see below) are virtually impossible to remove from DNA during isolation, but there are few reports (from experiments on tissues of vertebrates) finding them interfering even in complex genetic procedures (e.g. next generation sequencing), so this should not be a critical issue in invertebrate taxonomy.
The indisputable advantage of bright field light microscopy imaging is the common availability of cheap instrumentation which requires little specialist training on the part of the researcher. Light microscopes are usually available, even on board research vessels, and can be used for imaging of freshly collected specimens before fixation. When combined with staining, this technique can provide convincing basis for quantitative measurements and rudimentary conclusions with regard to biochemical composition of some structures (e.g. carapace calcification). The central disadvantage is the relatively poor contrast, both against the background and internally within the imaged specimen, leading to potential obfuscation of taxonomically important morphological differences and features. Standard light microscopy (both in reflected and transmitted light) is poor in rendering internal structures of the body and requires extensive dissection to image complex elements (like appendages). Efficiently imaging three-dimensional structures is not possible, even though they may be observed by stereomicroscopy (attempts have been made to construct and publish 3D images of Amphipoda to be viewed through red-cyan glasses, with limited success (Haug et al., 2011a). Nevertheless, taxonomical descriptions relying on bright field images of unstained or stained Peracarida continue to be routinely published, e.g. new amphipod species imaged after lignin pink staining (Hadjab et al., 2020) or new isopod species stained with chlorazol black (Pereira et al., 2019).
The contrast problem has led to the application of some specialized variants of optical contrast light microscopy (which all require technical add-on enhancements to the microscope itself which are relatively rare in zoological laboratories). One technique which has found use in taxonomically useful imaging of arthropods is dark field microscopy, where incident light is directed at the specimen in such a way that it does not pass into the objective unless deflected (reflected, refracted or scattered) by the specimen, leading to improved contrast against background and higher salience of delicate surface structures (Haug et al., 2011b). Another applicable method is polarization contrast that can underline differences in thickness and density of thicker homogenous structures formed by the cuticle (Fernández del Río et al., 2016; Melzer et al., 2021). Finally, interference contrast (also known as Nomarski contrast) is a powerful technique enabling the visualization of fine ultrastructural details. It has hitherto found application in deep-sea isopod and amphipod species taxonomy (Bruce, 1995; Bruce, 1997; Just, 2001; Tomikawa and Mawatari, 2006; Storey and Poore, 2009) but also in coastal and freshwater species (Shimomura and Mawatari, 1999; Shimomura and Mawatari, 2000; Tanaka, 2004; Jaume and Queinneck, 2007), demonstrating its power in imaging fine morphological structure of appendages (Maruzzo et al., 2007).
The most common solution to the contrast problem in biological microscopy is to make use of fluorescence, the physical phenomenon where some compounds (called fluorophores) absorb light of higher energy (lower wavelength) and subsequently emit light of lower energy (higher wavelength). This difference in wavelength, called Stokes shift, makes it possible to design microscopes which separate the incident (illumination) light from the light emanating from the sample, and thus obtain an image exclusively of the fluorescent elements within the sample. For most biological specimens, fluorescence microscopy requires staining with fluorescent dyes (fluorophore-containing compounds which bind to specific structures in the sample). Crustaceans (and arthropods in general), however, usually display relatively strong fluorescence of endogenous compounds (so-called autofluorescence) in intact specimens, allowing for easy fluorescence microscopy imaging and accounting for the widespread use of this technique in taxonomy. While biochemical studies of compounds responsible for autofluorescence in crustaceans are still too few and this field needs further intensive research, most parts of crustacean exoskeleton exhibit a broad-spectrum, near UV-excited autofluorescence that is a consequence of its highly cross-linked structure with glycan and protein components both contributing to the resulting fluorophores. Serendipitously, formaldehyde fixation tends to strengthen this broad-spectrum fluorescence component, making it even easier to image specimens fixed in this way (Hughes and Ahyong, 2016). Another source of autofluorescence is the elastomeric protein resilin, abundant in sites that are under strong mechanical stress such as tegument joints or mouthpart appendages, which contains dityrosine crosslinks that generate autofluorescence. Finally, some metabolic compounds (flavins, pterins, porphyrins, etc.) present in tissues also have fluorescent properties, enhancing the potential for fluorescent imaging of unstained specimens (Riehl and De Smet, 2020). Some arthropods have evolved dedicated autofluorescent compounds, probably important for ecological interactions, such as in some hoplocarid mantis shrimps with markings containing a yellow fluorescent fluorophore that are important in visual recognition or in shallow water copepods which contain dedicated fluorescent proteins similar to the more well-known ones from cnidarians. This ecologically motivated autofluorescence is even more common in terrestrial arthropods such as scorpions (which produce coumarin pigments) or millipedes (which rely on pterins). However, in crustaceans from the aphotic zone these dedicated fluorophores have not been detected yet and the observed autofluorescence seems to be a side effect of the biochemical structure of tissues and tegument (Glenn et al., 2013). Fluorescent properties may be used to enhance the visual signal generated by bioluminescence in deep-sea Peracarida that display this property, thus being ecologically important for visual communication within the species or between different species. Examples include, the lanceolid amphipod Megalanceola stephenseni (Chevreux, 1920) and amphipods from the families Pronoidae, Scinidae and Lysianassidae (Herring, 1981; Zeidler, 2009), mysids from family Mysidae (Herring, 1981) and the lophogastrid Neognathophausia ingens (Dohrn, 1870) (Frank et al., 1984), the fluorescence of which seems not to originate from the species itself, but rather to be dependent upon components of its food (Wittmann et al., 2014). This topic needs further studies on living specimens, preferably in situ (Macel et al., 2020). In any case, the presence of autofluorescence does not preclude the use of additional staining of specific structures in the crustacean body with fluorescent dyes for taxonomic purposes, but its continued presence needs to be taken into account for potential spectral overlap when selecting imaging channels.
When using fluorescence microscopy for taxonomic purposes, specimens are often stained with fluorescent dyes to further enhance contrast and facilitate the imaging of structures with defined biochemical composition. With regard to their mode of action, these dyes can be divided into four groups:
1) Broad specificity acidic dyes, which bind mainly to carbohydrates in the tegument. They are useful in detailed imaging of appendages, exoskeleton protrusions etc., while staining virtually the whole body of the animal to a different extent. The most commonly used dyes from this group are acid fuchsin (Riehl and De Smet, 2020; Kaiser et al., 2021) and Congo red (Michels and Büntzow, 2010; Kihara and Martinez Arbizu, 2012). An interesting example is rose bengal, a halogenated fluorescein derivative which has the capacity to bind cellular components as well, but in the presence of abundant extracellular carbohydrate material binds mostly to it. In the taxonomy of deep-sea Peracarida, its main use is for transient staining of (usually formaldehyde-fixed) mixed material to aid in visual sorting (due to its strong color) (Hegna, 2010), but its fluorescent properties allow it also to be used in whole-body fluorescence microscopy (Chim and Tong, 2020).
2) Carbohydrate-specific dyes, mostly taken over from the textile industry. They are i.a. Blankophor/Calcofluor (Brooker et al., 2012b), Shirlastain or aniline blue, which bind mainly to chitin in the exoskeleton (Riehl and De Smet, 2020).
3) Calcium binding stains, that are useful to identify calcified parts of the skeleton, such as calcein or alizarin red (Haug et al., 2011a).
4) Cationic dyes, which mainly bind to nucleic acids and stain living tissues more or less uniformly. They are safranin, eosin, DAPI or Hoechst family dyes (Kakui and Hiruta, 2017).
More specialized fluorescent probes binding to cellular or subcellular elements with restricted distribution may also be used, e.g. cytoskeleton-specific binders such as phalloidin or fluorescent antibodies (this technique is called immunofluorescence), but this is of limited usefulness in taxonomy and more commonly found in physiological or embryological studies. Both autofluorescence and probe/dye fluorescence is subject to a phenomenon called photobleaching, where long-term illumination causes a chemical reaction that destroys fluorophore molecules, leading to decreased image brightness. This can be slowed down by including so-called anti-fade components in the mounting medium, but this is rarely necessary with the bright and stable fluorophores used for taxonomically relevant imaging of crustaceans.
With regard to instrumentation, the simplest application of the fluorescence microscopy principle is the widefield fluorescence microscope which uses the same optical principle as a bright field microscope, but separates optical paths of excitation and emission light using filters and dichroic mirrors. Images generated in a widefield microscope can be viewed directly through the eyepiece or recorded using photographic or motion cameras. They can also be overlaid in-microscope with bright field images, pinpointing the location of fluorescent structures within the whole body of the animal. Widefield image quality is restricted by the so-called out-of-focus blur, i.e. light emitted from above and below the focal plane which enters the objective and decreases the image sharpness. This can be strongly limiting in the imaging of small taxonomically important elements within a larger structure. Therefore, an increasing number of taxonomic studies make use of another fluorescence imaging modality, so-called confocal microscopy. A confocal microscope retains only the objective lens from a standard optical microscope setup and images only a single point within the sample (so-called confocal volume), using regulated apertures (here called pinholes) to cut off out-of-focus illumination from both excitation and emission light paths. Therefore, a confocal microscope does not generate an image, but measures the fluorescence intensity in a spatially defined point within the sample. An image is subsequently reconstructed digitally by dedicated computer software from data collected from various confocal volumes, as the illumination is scanned across the sample. The scan may be effected in two ways: either by using optically deflected laser beams (laser-scanning confocal microscopy, in zoology usually known under the less logical name confocal laser scanning microscopy or CLSM) or by using spinning discs (Nipkow discs) with multiple pinholes (spinning disc confocal microscopy). While spinning disc confocal microscopy generates images much faster and with higher inherent brightness, these advantages are mostly important in imaging live specimens, which is rare for deep-sea taxonomical purposes. The relative rarity and costliness of spinning disc microscopes combined with their lack of versatility make them a niche tool for crustacean taxonomy when compared to laser-scanning microscopes (Haug et al., 2011b).
A confocal image is not “recorded” in a way that a camera records a widefield image, but is reconstructed from individual pixels in silico, so the native form of this image is already digital and with no loss of quality upon digitization. Since the confocal volume can be moved across the sample in all directions, a confocal microscope can be used to record three-dimensional images of specimens, making it especially useful in crustacean taxonomy where many important features such as appendage structure are inherently three-dimensional (Figures 3O–R). Properties of light, however, restrict the image resolution in the Z axis (parallel to the long axis of the objective) to ca. 2–3 times less than lateral resolution, so confocal images are never truly 3D-isomorphic. If isomorphism is absolutely necessary for taxonomic purposes, several images with different specimen orientation must be recorded. Since laser scanning confocal microscopy involves moving a small confocal volume around a large specimen, it is notoriously slow, with a good resolution image of an average-sized deep-sea crustacean taking more than 10 hours to record. Moreover, because for good resolution it is necessary to use medium-magnification objectives which usually do not allow the whole animal to fit in a single field of view, sophisticated software must be used to reconstruct the whole image from several adjacent scans in a procedure called tiling – its success (the lack of visible artifacts on scan joints) depends largely on the quality of the objective (spherical aberration correction). When recording 3D confocal images and using them for taxonomy, the way that they will be presented and disseminated in the literature must be considered, because the original files are usually too large to include even as supplementary information in published articles. A number of 2D projections (most common being maximum intensity projection and surface projection) have been developed to help present 3D data.
Electron microscopy is a group of imaging techniques which use physical effects which happen when the sample is illuminated with a stream of high-energy electrons: usually, transmitted, scattered or secondary electrons are detected. The main advantage of electron microscopy in biological imaging is the potential to generate images of much higher inherent resolution than light microscopy, since the electron beam is equivalent to radiation with a very short wavelength compared to visible light. However, for purposes of taxonomy of macroscopic invertebrates, this aspect rarely comes into play, since subcellular features (and generally features of submicrometric size) are not often used as taxonomically defining. The variant of this technique that is most often used by taxonomists is scanning electron microscopy (SEM), where the sample is illuminated by a narrow electron beam which moves across its surface and secondary electrons emitted from every spot on the way (only from the surface since they have too low energies to escape from lower layers of material) are measured using an array of detectors, recreating in real time a spatial map of the surface relief. The main advantages of this method which make it so attractive for imaging for taxonomic purposes are: high sensitivity to small changes in surface geometry which makes it possible to efficiently image surface texture and generate high-resolution images of delicate and complex structures such as those abounding on crustacean exoskeletons and appendages; high depth of field which retains in focus structures that are far away from each other along the z axis, generating a realistic and sharp image of the whole macroscopic specimen while retaining sub-microscopic resolution; the ability to modify magnification in a wide range (from several-fold to tens of thousands-fold) in a contiguous, real-time manner while conducting observations; and the possibility to easily reconstruct three-dimensional measurements from images or generate true 3D images of the specimen by recording images from two different angles. However, the method has also significant disadvantages, mostly related to the onerous and highly invasive sample preparation required for imaging in a typical SEM instrument: since both the high-energy illumination electrons and the low-energy secondary electrons that are being imaged can be deflected by interactions with air molecules, low-pressure vacuum environment is needed around the sample, which means it cannot contain water (so, biological samples must be dehydrated before imaging); since atoms contained in organic compounds do not interact with high-energy electrons efficiently and do not generate many secondary electrons, it is often necessary to coat the specimen surface with a layer of higher atomic number atoms which will produce a brighter image; the absorption of electrons by the specimen generates a high static electrical charge which would quickly lead to scanning artifacts, discharges and specimen destruction if not removed, thus the specimen must be electrically conductive or coated with a material which conducts electricity. For these reasons, SEM is a destructive technique and specimens of Peracarida prepared for SEM imaging cannot usually be used subsequently for any further preparation or analysis using other methods. The sample preparation process for deep-sea crustaceans for SEM imaging has several important steps at which different approaches may be taken depending on specific needs of the researcher. Due to the high energies and harsh treatment involved, the specimen needs to be fixed in a strong fixating agent, usually glutaraldehyde or a mixture of glutaraldehyde and formaldehyde. Dehydration cannot be achieved by air drying as this would destroy delicate surface structures, so water is first replaced by an organic solvent (e.g. ethanol or acetone), and this solvent with higher vapor pressure may be either evaporated directly with less damage to the specimen or it may be replaced with liquid carbon dioxide which then evaporates in conditions around its phase transition critical point (where gas and liquid densities are equal, removing the damaging surface tension - so-called critical point drying). For imaging, the specimen may then be coated with a thin layer of metal (such as gold, platinum, palladium or their mixtures) which provides both better secondary electron emission and electrical conductance, or with a layer of powdered carbon (graphite) which only increases conductance. Another useful metal with unique properties is osmium – its tetroxide is an efficient fixative due to the ability to bind lipids (see the chapter on fixation), coating with osmium itself provides conductivity, and both treatments strongly increase contrast due to efficient secondary electron generation.
Apart from standard SEM, other electron microscopy techniques have been used to image aquatic crustaceans, including deep-sea Peracarida. Environmental SEM (ESEM) is a variation of SEM where differential pumping and pressure-limiting apertures allow the placement of the specimen in a gaseous environment. While this still requires a low-pressure environment, water vapor pressure may be kept at saturation levels, allowing the imaging of water-containing (non-dehydrated) specimens (Drumm, 2005). This is of high importance for potential taxonomic usage as imaging is thus non-destructive and the specimen may be re-used in studies using other methods (however, the pressures used in ESEM are usually low enough to cause the sample to freeze, and the freeze-thaw cycle may break up longer DNA molecules, so the sample may be no longer ideal for e.g. nanopore sequencing). The gaseous environment requires low electron beam energies and specialized detectors, which has both practical advantages (most importantly there is no need to coat the sample in conductive material as there is no static electricity build-up, confirming the non-destructive characteristics of this methodology) and disadvantages (the depth of field is severely limited, making low magnification imaging of large specimens difficult). While for terrestrial arthropods, this has allowed the imaging of even live individuals, the applicability of ESEM for aquatic animals is less apparent due to imaging artifacts from liquid droplets at fine structures, but the technological advances in recent years will probably remove this impediment. While SEM is usually used to image the specimen surface, it can be modified for three-dimensional imaging of deeper tissue layers, which is of special interest for crustacean taxonomists as it allows to recreate high-resolution images of small appendages with complex structure (e.g. mouthparts). One such modification is serial block-face SEM (SBF-SEM) where the animal is stained with heavy metals (osmium, gold, uranium or lead), embedded in a block of epoxy resin and placed in the imaging chamber of a SEM microscope. The top layers of the block are subsequently serially removed with an ultramicrotome which is contained within the imaging chamber itself, and SEM images of the surface at each cutting depth are combined into a 3D image (Kaji et al., 2016). Alternatively, top layers of biological material (e.g. exoskeleton) may be removed by so-called ion beam milling (abrasion by bombardment with a focused stream of high-energy ions) in a technique called focused ion beam SEM (FIB-SEM) (Haug et al., 2011a). The advantage of FIB-SEM in comparison to SBF-SEM is that location of in-depth imaging is determined by the researcher, the 3D image resolution is uniform in all dimensions and the sample does not require embedding, while SBF-SEM is significantly faster (and having the resolution in the vertical dimension limited by the thickness of ultramicrotome slice is usually not a problem for taxonomically relevant features of crustacean bodies). Finally, traditional transmission electron microscopy (TEM), which involves preparing ultra-thin slices of the sample and treating them with heavy metal stains or probes, has also been applied in proof-of-concept studies to image the fine structure of tissue of some peracarids, but its applicability in taxonomy is not related to morphological studies, but limited to determination of differences in molecular composition of proteins, e.g. by immunogold staining, or carbohydrates, e.g. by lectin-gold staining (this potential has not yet been practically applied for taxonomic purposes in Peracarida).
Computed tomography refers to any technique that allows three-dimensional imaging of internal structure by techniques that do not require physical dissection/slicing of the specimen (such as magnetic resonance imaging or positron emission tomography). However, in practical usage in zoology, this term (and the subordinate term microcomputed tomography, or µCT, when applied to microscopic objects) is understood exclusively as applying to imaging via X-ray illumination and multi-point detection of transmitted and scattered X-rays (more properly known as X-ray tomography). The principle is the three-dimensional analogue of standard medical X-ray imaging of tissue, with pixel size in the micrometric range. This allows the non-destructive imaging of internal structure of zoological specimens and has become one of mainstays of morphology studies of deep-sea crustaceans for taxonomic purposes (Gutiérrez et al., 2018) and specially for treatment of fossil records (Jauvion et al, 2016; Jauvion, 2020; see Figure 3S). Specimen preparation is simple: while the samples may be unfixed (e.g. flash-frozen), it is usual to use specimens fixed in the standard manner (since µCT allows for subsequent use of the same specimen in any other analysis or imaging protocol). Both ethanol and formaldehyde fixatives work fine, with some studies recommending the use of acidic coagulants (in the form of Bouin’s fixative) to yield higher image contrast (this is, however, not necessary for crustacean taxonomy in most cases, as the inherent contrast between soft and hard tissue is sufficient anyway) (Wirkner and Richter, 2004). Image quality may be enhanced by stains (in this technique idiosyncratically called “contrast agents”), with the most common ones (providing superior X-ray scattering capabilities) containing atoms of iodine (e.g. Lugol’s solution) or osmium (e.g. osmium tetroxide) (Grams and Richter, 2021). This staining helps especially to differentiate between soft tissues with different fat content, but it has not hitherto been shown to be important for crustacean taxonomy, with enough endogenous contrast present in virtually all cases. While the specimens do not need to be dry for imaging itself to be successful, the lengthy scanning process often leads to spontaneous evaporation (air-drying) and consequential morphological artifacts, which makes many researchers opt for specimen dehydration (usually by critical point drying) before µCT imaging. It must be reminded that this makes the sample unsuitable for some potential downstream analysis, including some optical microscopy methods (e.g. immunofluorescence) or nucleic acid isolation for long-chain sequencing. In some studies where precise discrimination between small internal features was necessary (e.g. in neuroanatomy of arthropods), higher energies of X-ray illumination (derived from a large device known as a synchrotron) have been used (Betz et al., 2007). However, for taxonomically important morphological features laboratory-scale µCT (which uses fully shielded bench-size X-ray sources) is fully sufficient. The main advantages of µCT for imaging morphological features of deep-sea Peracarida is the non-destructive character of imaging (thus, it can be used even for the most valuable type samples), the ease of sample preparation and the isomorphic resolution of three-dimensional images (allowing reliable measurement of spatial features). Disadvantages are limited to low access to relevant equipment in some academic centers (although this is currently changing with increasing affordability of µCT equipment) and lack of obvious links between physico-chemical composition of biological tissues and structures and contrast features of the image (which is, however, usually not important for taxonomists).
The naming of species according to defined standards serves to link new information with existing knowledge. The purpose of formal species descriptions is therefore to show how a species is characterized, how it differs from other known species, and ultimately to make the name available for biogeographical, conservational, or phylogenetic studies amongst others. In the past, species descriptions consisted only of the name and diagnosis of the most important segregating features, later detailed descriptions followed, which are extensive, time consuming and (arguably) not necessary (Riedel et al., 2013; Renner, 2016).
A detailed morphological description clearly contradicts ongoing efforts to accelerate taxonomic work. New methodologies and integrative approaches also do not contribute much to the goal of making taxonomy faster, on the contrary, they tend to increase complexity. This is also due to the fact that with increasing use of molecular tools in taxonomy, often more (new) species are discovered than being described (Pante et al., 2015), a condition that is also observed in studies of deep-sea peracarids (Jennings et al., 2018; Brix et al., 2020; Kaiser et al., 2021; Mohrbeck et al., 2021). Reasons for this gap are manifold: for instance, definitive (morphological and molecular) evidence of a new species is absent, the authors lack taxonomic expertise or there is not enough time to describe all the species in the duration of a (post doc) project (Pante et al., 2015; Brix et al., 2020; Malyutina et al., 2020; Kaiser et al., 2021).
From a peracarid study point of view, there arguably has hardly been any progress in deviating from the so-called taxonomic impediment, i.e. the description of the many, especially small-sized taxa by declining number of taxonomists (Convention on Biological Diversity [CBD], 2010; Mora et al., 2011; Coleman, 2015; Engel et al., 2021). Over the past decades, novel tools have been introduced to put taxonomy into the fast lane, from automated species descriptions (e.g., using DELTA - DEscriptive Language for TAxonomy, Dallwitz et al., 2000), turbo- (Riedel et al., 2013) and cybertaxonomy (Zhang, 2008), to descriptions based exclusively on DNA sequences as diagnostic characters (Jörger and Schrödl, 2013). Turbotaxonomy, for example, describes the approach of linking molecular sequences, morphological descriptions, and high-resolution digital imaging to enable the rapid formal description of a relatively large number of new species (Riedel et al., 2013). While the appropriateness of some new approaches is certainly controversial (e.g., DNA sequences as diagnostic characters, Meier et al., 2022), so far only a few of the modern endeavors mentioned above have been translated into the description of new deep-sea peracarid species (e.g., Lowry and Myers, 2012; Sittrop et al., 2015).
The task of describing all peracarid species from the deep sea is enormous. Hundreds of species are already known within the Peracarida from there, especially within the Isopoda and Tanaidacea (Brandt et al., 2012; Błażewicz-Paszkowycz et al., 2012). Yet, the number of undescribed species is probably much larger, although robust estimates are scarce (Wilson, 2017). From the central abyssal Pacific, for example, 187 and 98 supposedly new species within Isopoda and Tanaidacea, respectively, could be identified from a single sample campaign (Błażewicz et al., 2019; Brix et al., 2020). Add to this, the need of taxonomic revisions and redescriptions of earlier works, which is crucial, but also leads to a step backwards in the description and assessment of deep-sea peracarid biodiversity (Brandt et al., 2012).
For the hypothetical case that around 10,000 deep-sea species within the Isopoda and Tanaidacea still have to be described, existing taxonomists would need around 1,000-2,500 years with a current average rate of 4–9 descriptions per year (Figure 4). However, this also requires that sufficient taxonomic expertise remains available and that its number do not decrease any further. Therefore, taxonomic intercalibration exercises in the form of the exchange of sketches and informal taxonomic information were encouraged in order to compare undescribed biodiversity between different regions (International Seabed Authority ISA, 2020; Lins et al., 2021; Washburn et al., 2021). Furthermore, lengthy morphometric investigations and descriptions of new species have already been replaced by proteomic profiles (Yeom et al., 2021). In addition, molecular methods such as e-DNA metabarcoding approaches are propagated, which record biodiversity in a certain area by circumventing formal species descriptions (Dell’Anno et al., 2015; Pawlowski et al., 2018).
Figure 4 Rate of deep-sea species descriptions within Isopoda (top) and Tanaidacea (below); the line indicates the cumulative number of species (corresponding left y-axis), and the scatter plot indicates the actual number of species described (right y-axis) per year. The average description rate in the last ten years was ~4 and 9 species for tanaidaceans and isopods respectively. Data retrieved from WoRDSS (Glover et al., 2021) and updated through WoRMS (WoRMS Editorial Board, 2021). According to WoRDSS (Glover et al., 2021) only species described from below 500 m are included.
Despite the urgency to describe deep-sea fauna in the wake of augmented human impacts, we believe that species should still be formally named and described. Furthermore, descriptions should adhere to common standards, such as according to the International Code of Zoological Nomenclature (ICZN). Species descriptions take time to be accurate and robust, but they could become standardized and more automated (e.g., using programs such as DELTA or MANTIS; Dallwitz et al., 2000; Naskrecki, 2008; Brown, 2013). In addition, experts for a specific group could agree on the lowest common denominator of diagnostic features necessary for the delineation and identification of species, while supplementary microscopic images (such as CLSM see above) provide further taxonomically important information, as well as biogeography, environmental parameters, or DNA barcoding. Overall, we agree with Glover et al. (2018) that only through a comprehensive study of deep-sea species can we gain a better understanding of their function and value for the for deep-sea ecosystems.
Undoubtedly, peracarids are an integral part of deep-sea benthic ecosystems (Hessler and Wilson, 1983; Błażewicz-Paszkowycz et al., 2012; Frutos et al., 2017a). Within the particularly species-rich groups, isopods and tanaidaceans, so far around 2,000 species have been described (Figure 4), and that should be only a fraction of what is actually present. While well-established traditional methods are often still in use to describe and classify deep-sea Peracarida, new methodologies, notably molecular and microscopic imaging tools, have taken their taxonomic analysis to a new (integrative) level. Specifically, these methods have helped solve some common issues in peracarid taxonomy, including, but not limited to, the delineation of morphologically the same or similar species (Havermans et al., 2013; Brandt et al., 2014; Brix et al., 2015; Jakiel et al., 2020; Kaiser et al., 2021), those with strong sexual or ontogenetic dimorphism (Riehl et al., 2012; Błażewicz-Paszkowycz et al., 2014; Riehl and Kühn, 2020), polymorphism (Larsen, 2001) or incomplete, damaged specimens (Kaiser et al., 2018). The latter is more the rule than the exception. In particular, fragile peracarid crustaceans are damaged when taking samples from greater depths or during sample processing. In addition, fixatives, especially ethanol, although the latter being still first choice, also make the specimens brittle, so they tend to lose their legs or antennae (even if the latter may be mitigated by using small amounts of glycerol (Wilson and Humphrey, 2020). The ability to identify damaged specimens is therefore certainly an advantage of molecular methods over traditional morphological identification (Mohrbeck et al., 2015).
While the methodologies considered here are focused on deep-sea peracarids, they can be applied, in the same way, to the study of other benthic small-sized crustaceans, i.e. ostracods and copepods. With special requirements for efficient sampling (<300 μm mesh-size nets or multi-corer; see Narayanaswamy et al., 2016), the identification of specimens of meiofaunal harpacticoid copepods often demands the dissection of their smallest appendages (Kihara and Martinez Arbizu, 2012; Rossel and Martínez Arbizu, 2018). They are studied in a similar workflow using modern imaging tools under an integrative approach for species identification (Easton and Thistle, 2016; Khodami et al., 2020), however, special techniques adapted to their tiny size (i.e. mass spectrometry) are also suitable for their identification (Rossel and Martínez Arbizu, 2018; Rossel and Martinez Arbizu, 2019).
Overall, the introduction of new taxonomic methods for application to deep-sea specimens seems to be delayed compared to those in shallow waters or on land. ‘Omic’ approaches, for instance, are increasingly being utilized for classification and identification of species (Bourlat et al., 2013; Raupach et al., 2016; Rossel and Martinez Arbizu, 2019). Whole-genome data, that are already used to separate prokaryote strains, may also be applied to eukaryote taxonomy in the future (Raupach et al., 2016). Yet, in the marine realm and even more so in the deep sea, the application of genomics is still in its infancy. In recent years, genomes have been published for a number of marine species (Wilson et al., 2005; Ritchie et al., 2017; Li et al., 2019), here in particular for amphipods, but also genomes from a number of tanaidacean species have been now analyzed (Kakui and Kano, 2021). For deep-sea isopods and tanaidaceans this is still pending though. Another promising approach is proteomic fingerprinting, which has already been used successfully in the identification of deep-sea isopods (Paulus et al., 2021; Kürzel et al., 2022). The advantages are the faster and cheaper application of proteomics, for example compared to the molecular genetic approach. Yet, it requires a library of protein mass spectra and, overall, the technology is not yet mature enough to reliably delineate species from an unknown deep-sea sample from one another and thus needs further evaluation (Kürzel et al., 2022).
The ‘hesitation’ in testing new methods is probably partly due to the challenges of deep-sea sampling itself, as fauna densities are typically low especially at greater depths (Frutos and Sorbe, 2014; Wilson, 2017; Malyutina et al., 2018) and therefore the number of organisms usually needed for any kind of molecular analysis may not be achieved. In addition, most deep-sea peracarids, with the exception of a few giant isopods and amphipods, are small, and often only a few millimetres in size, which makes it difficult to extract DNA from these specimens while keeping a whole animal as a voucher. Finally, many of these methods come at a price, require special facilities and equipment as well as expertise (e.g. Pinu et al., 2019, but see Le et al., 2021). Yet, there is no question that now is the time to look more closely than ever before into describing deep-sea biodiversity, which also means to delve deeper into these new approaches, but also to critically evaluate those that have been applied so far (e.g. with respect to long-term preservation of samples and slides, Table 4). The deep-sea environment could be used to a greater extent for its resources in the future and is already affected by deep-sea fisheries (e.g. Clark et al., 2016), environmental pollution (Chiba et al., 2018) and climate change (Sweetman et al., 2017). So, time is of the essence to describe more species rather quickly in order to better understand these impacts and their consequences for deep-sea ecosystems.
Despite all the advances, taxonomy has probably never been as challenging as it is today. It starts with the fact that the importance of taxonomic research is not recognized and in turn not well promoted or funded (e.g. Wägele et al., 2011; Saunders, 2020; Britz et al., 2020). In part, this is because the quality of scientific progress is measured by the Impact Factor of journals, with taxonomic journals often falling behind (Wägele et al., 2011). Chairs with a purely taxonomic focus have become a rarity, and taxonomy has become often only a sub-area of otherwise molecular or ecological subjects (e.g., Lester et al., 2014). Since taxonomic research appears to have no future, only a few young scientists can get enthusiastic about the topic, and there is already a shortage of well-trained taxonomists evident today. This taxonomic impediment mentioned above, in which a decreasing number of taxonomists are faced with a high undescribed diversity, is also noticeable among (deep-sea) peracarid taxonomists (Błażewicz-Paszkowycz et al., 2012). Within the last ten years there have been seven and 16 active taxonomists (only first authors counted), who have described deep-sea species within the Tanaidacea and Isopoda, respectively, but only few of them holding a permanent position (Glover et al., 2021). For amphipods, Coleman (2015) counted nine active taxonomists, although these include the entire diversity of this speciose group - from freshwater to marine. Yet, with regard to the methods and techniques presented in this review, we show how diverse and demanding the taxonomic work is, which not only includes the time-consuming work of describing new species, but often also dealing with unsolved phylogenetic histories including species’ redescription and assessment of museum’s type material. Among other things, this not only requires a taxonomist to have profound theoretical knowledge of species concepts and phylogenetic analytical methods but also methodical skills, for example in the application of various microscopy techniques or imaging processes as well as relevant molecular methods, while at the same time having to keep up with the pace of how the latter are developing.
It is not a new topic that taxonomic work is highly underrated, and at the same time it is not an individual problem that taxonomists do not get recognition for their work, but that is placed in a broader context and ultimately linked to how society values biodiversity and nature. In our opinion, this is exactly where we have to start, namely to convey taxonomic research and thus the diversity of life to other scientists, but also the wider public. New methods can play a special role here, because the application of the new imaging processes opens up a new world not only to taxonomists, but also to other scientists. SEM let us recognize surfaces that were previously invisible and provides information about the hardness of the tegument; CLSM or computed tomography help to recognize internal structures and thus contribute to the understanding of the functional morphology, embryology or even to the recognition of the material quality that defines the respective structures. All of this not only gives us the opportunity to learn what type of animal we are seeing, but also how it is constructed and how it functions. Thus, these new techniques (including imaging), which are primarily geared towards taxonomy, are an important link to other sciences thus making taxonomy a highly integrative field of science. For laypeople, of course, this only plays a subordinate role; instead, ethical and aesthetic reasons to value or reject something are often in the foreground (cf. Jamieson et al., 2021). Analogous to Haeckel’s drawings, the art factor (microscopic images) could be used to reach the public and convince them of the beauty of deep-sea life, and thus also to raise their awareness of how biodiverse the deep sea is and that this diversity is threatened.
Learning more about the deep sea and its inhabitants is an urgent need, and taxonomy will play an important role in this endeavor. Therefore, changes must be addressed here too, in order to describe deep-sea species and thus biodiversity more quickly and at the same time to ensure high-quality taxonomic work. Although great advances have been made in microscopy and imaging tools, it has been shown that relying on morphology alone to describe species poses a number of pitfalls. Therefore, integrative taxonomy in describing deep-sea species is the way forward, as it provides multiple lines of evidence to reliably differentiate species from one another. So, whenever possible, both morphological and molecular (if fixation allows), as well as possibly a description of the environment among others should be sought when describing species. In this paper we have also discussed a number of methods that have not yet or only rarely been so far used in peracarid taxonomy, but that may become more important in the future. Here, particular mention should be made of (non-destructive) microscopic techniques such as CLSM, ESEM or µCT or ‘omic’ approaches including genomics and proteomics. Above all, however, taxonomic work is to be recognized as what it is, i.e. a multidisciplinary science that makes an essential part of research into deep-sea biodiversity and thus a significant contribution to its conservation.
IF and MB developed the idea; IF and SK produced the figures. All authors contributed to drafting and editing the text, read and approved the submitted version.
SK acknowledges a grant by the Polish National Agency for Academic Exchange (NAWA) under the ULAM program (PPN/ULM/2019/1/00169); IF acknowledges PPN/BFR/2019/1/00031/U/00001 PHC-Polonium; MB and IF acknowledge – NCN OPUS 2018/31/B/NZ8/03198 and NCN OPUS 2016/13/B/NZ8/02495.
The authors declare that the research was conducted in the absence of any commercial or financial relationships that could be construed as a potential conflict of interest.
All claims expressed in this article are solely those of the authors and do not necessarily represent those of their affiliated organizations, or those of the publisher, the editors and the reviewers. Any product that may be evaluated in this article, or claim that may be made by its manufacturer, is not guaranteed or endorsed by the publisher.
Preparation of this manuscript began during the Covid-19 pandemic, which did not allow co-authors to meet in person until restrictions were relaxed. The authors are therefore indebted to the online technology that made the advancement of this article possible. We would like to thank Laure Corbari and Paula Martín-Lefevre, Musée national d’Histoire Naturelle in Paris, who kindly provided the scan of the original plate outline of Chevreux; and two reviewers for their comments that substantially improved this manuscript.
Ahyong S. T., Chan B. K. K., Chan T.-Y., Corbari L., Ďuriš Z., Frutos I, et al. (2022). “Crustacean Diversity and Discovery,” in The Marine Fauna and Flora of the Bismarck Sea. Patrimoines Naturels. Eds. Bouchet P., Payri C., Sabroux R., Samadi S. Paris:Muséum national d'Histoire Naturelle
Allington-Jones L., Sherlock E. (2007). Choosing a Microscope Slide Sealant: A Review of Aging Characteristics and the Development of a New Test, Using Low Oxygen Environments. NatSCA News 12, 4–14.
Almeida M., Frutos I., Company J. B., Martin D., Romano C., Cunha M. R. (2017). Biodiversity of Suprabenthic Peracarid Assemblages From the Blanes Canyon Region (NW Mediterranean Sea) in Relation to Natural Disturbance and Trawling Pressure. Deep Sea Res. Pt. II Top. Stud. Oceanogr. 137, 390–403. doi: 10.1016/j.dsr2.2016.06.019
Anderson G. (2014). Endangered: A Study of Morphological Drawing in Zoological Taxonomy. Leonardo 47 (3), 232–240. doi: 10.1162/LEON_a_00675
Appeltans W., Ahyong S. T., Anderson G., Angel M. V., Artois T., Bailly N., et al. (2012). The Magnitude of Global Marine Species Diversity. Curr. Biol. 22 (23), 2189–2202. doi: 10.1016/j.cub.2012.09.036
Ashford O. S., Kenny A. J., Barrio Frojan C. R. S., Bonsall M. B., Horton T., Brandt A., et al. (2018). Phylogenetic and Functional Evidence Suggests That Deep-Ocean Ecosystems are Highly Sensitive to Environmental Change and Direct Human Disturbance. Proc. R. Soc B. 285, 20180923. doi: 10.1098/rspb.2018.0923
Bamber R. N. (2007). New Apseudomorph Tanaidaceans (Crustacea, Peracarida, Tanaidacea) From the Bathyal Slope Off New Caledonia. Zoosystema 29 (1), 51–81.
Bameul F. (1990). Le DMHF: Un Excellent Milieu De Montage En Entomologie. L’Entomologiste 46 (5), 233–239.
Barbosa P., Berry D. L., Kary C. S. (2014). Insect Histology: Practical Laboratory Techniques (John Wiley and Sons, Ltd). doi: 10.1002/9781118876114
Barnard J. L., Ingram C. L. (1990). Lysianassoid Amphipoda (Crustacea) From Deep-Sea Thermal Vents. Smithson Contrib. Zool. 449, 1–80. doi: 10.5479/si.00810282.499
Bellan-Santini D., Thurston M. H. (1996). Amphipoda of the Hydrothermal Vents Along the Mid-Atlantic Ridge. J. Nat. Hist. 30, 685–702. doi: 10.1080/00222939600770381
Beninde J., Möst M., Meyer A. (2020). Optimized and Affordable High-Throughput Sequencing Workflow for Preserved and Nonpreserved Small Zooplankton Specimens. Mol. Ecol. Resour. 20, 1632–1646. doi: 10.1111/1755-0998.13228
Betz O., Wegst U., Weide D., Heethoff M., Helfen L., Lee W.-K., et al. (2007). Imaging Applications of Synchrotron X-Ray Phase-Contrast Microtomography in Biological Morphology and Biomaterials Science. I. General Aspects of the Technique and Its Advantages in the Analysis of Millimetre-Sized Arthropod Structure. J. Microsc. 227, 51–71. doi: 10.1111/j.1365-2818.2007.01785.x
Błażewicz M., Jóźwiak P., Jennings R. M., Studzian M., Frutos I. (2019). Integrative Systematics and Ecology of a New Deep-Sea Family of Tanaidacean Crustaceans. Sci. Rep. 9 (1), 1–70. doi: 10.1038/s41598-019-53446-1
Błażewicz-Paszkowycz M., Bamber R., Anderson G. (2012). Diversity of Tanaidacea (Crustacea: Peracarida) in the World’s Oceans–How Far Have We Come? PloS One 7 (4), e33068. doi: 10.1371/journal.pone.0033068
Błażewicz-Paszkowycz M., Bamber R. N., Cunha M. R. (2011). New Tanaidomorph Tanaidacea (Crustacea: Peracarida) From Submarine Mud-Volcanoes in the Gulf of Cadiz (North-East Atlantic). Zootaxa 2769 (1), 1–53. doi: 10.11646/zootaxa.2769.1.1
Błażewicz-Paszkowycz M., Jennings R. M., Jeskulke K., Brix S. (2014). Discovery of Swimming Males of Paratanaoidea (Tanaidacea). Pol. Polar. Res. 35 (2), 415–453. doi: 10.2478/popore-2014-0022
Bober S., Riehl T. (2014). Adding Depth to Line Artwork by Digital Stippling – A Step-by-Step Guide to the Method. Org. Divers. Evol. 14 (3), 327–337. doi: 10.1007/s13127-014-0173-7
Bober S., Riehl T., Brandt A. (2018). An Organ of Equilibrium in Deep-Sea Isopods Revealed: The Statocyst of Macrostylidae (Crustacea, Peracarida, Janiroidea). Zoomorph 137 (1), 71–82. doi: 10.1007/s00435-017-0376-5
Bourlat S. J., Borja A., Gilbert J., Taylor M. I., Davies N., Weisberg S. B., et al. (2013). Genomics in Marine Monitoring: New Opportunities for Assessing Marine Health Status. Mar. Poll. Bull. 74 (1), 19–31. doi: 10.1016/j.marpolbul.2013.05.042
Bourque D. A., Morey K. C., Bradley D. L., Fost B., Daley J. M., Jacobson N., et al. (2020). Dimethyldimethyl Hydantoin: An Alternative Fluid for Morphological and Genetic Preservation. Biopreserv. Biobank. 18, 283–289. doi: 10.1089/bio.2020.0001
Boxshall G. A., Kihara T. C., Huys R. (2016). Collecting and Processing Non-Planktonic Copepods. J. Crust. Biol. 36, 576–583. doi: 10.1163/1937240X-00002438
Brandt A., Alalykina I., Brix S., Brenke N., Błażewicz M., Golovan O. A., et al. (2019). Depth Zonation of Northwest Pacific Deep-Sea Macrofauna. Prog. Oceanogr. 176, 102131. doi: 10.1016/j.pocean.2019.102131
Brandt A., Błażewicz-Paszkowycz M., Bamber R. N., Mühlenhardt-Siegel U., Malyutina M. V., Kaiser S., et al. (2012). Are There Widespread Peracarid Species in the Deep Sea (Crustacea: Malacostraca)? Pol. Polar. Res. 33 (2), 139–162. doi: 10.2478/v10183-012-0012-5
Brandt A., Brix S., Held C., Kihara T. C. (2014). Molecular Differentiation in Sympatry Despite Morphological Stasis: Deep-Sea Atlantoserolis Wägele 1994 and Glabroserolis Menzies 1962 From the South-West Atlantic (Crustacea: Isopoda: Serolidae). Zool. J. Linn. Soc 172 (2), 318–359. doi: 10.1111/zoj.12178
Britz R., Hundsdörfer A., Fritz U. (2020). Funding, Training, Permits—the Three Big Challenges of Taxonomy. Megataxa 1 (1), 49–52. doi: 10.11646/megataxa.1.1.10
Brix S., Leese F., Riehl T., Kihara T. C. (2015). A New Genus and New Species of Desmosomatidae Sars 1897 (Isopoda) From the Eastern South Atlantic Abyss Described by Means of Integrative Taxonomy. Mar. Biodiv. 45, 7–61. doi: 10.1007/s12526-014-0218-3
Brix S., Osborn K. J., Kaiser S., Truskey S. B., Schnurr S. M., Brenke N., et al. (2020). Adult Life Strategy Affects Distribution Patterns in Abyssal Isopods – Implications for Conservation in Pacific Nodule Areas. Biogeosciences 17, 6163–6184. doi: 10.5194/bg-17-6163-2020
Brökeland W., Guðmundsson G., Svavarsson J. (2010). Diet of Four Species of Deep-Sea Isopods (Crustacea: Malacostraca: Peracarida) in the South Atlantic and the Southern Ocean. Mar. Biol. 157, 177–187. doi: 10.1007/s00227-009-1308-9
Brooker A., Bron J., Shinn A. (2012a). Description of the Free-Swimming Juvenile Stages of Lernaeocera Branchialis (Pennellidae), Using Traditional Light and Confocal Microscopy Methods. Aquat. Biol. 14, 153–163. doi: 10.3354/ab00388
Brooker A., Shinn A., Bron J. (2012b). Use of Laser Scanning Confocal Microscopy for Morphological Taxonomy and the Potential for Digital Type Specimens (E-Types). Aquat. Biol. 14, 165–173. doi: 10.3354/ab00389
Brown B. V. (2013). Automating the” Material Examined” Section of Taxonomic Papers to Speed Up Species Descriptions. Zootaxa 3683 (3), 297–299. doi: 10.11646/zootaxa.3683.3.8
Bruce N. L. (1995). The Taxonomy and Phylogeny of Tube-Tailed Sphaeromatid Isopods (Crustacea) With Descriptions of New Species and a New Genus From Southern Australia. Ophelia 43 (2), 127–180. doi: 10.1080/00785326.1995.10429829
Bruce N. L. (1997). A New Genus of Marine Isopod (Crustacea: Flabellifera: Sphaeromatidae) From Australia and the Indo- Pacific Region. Mem. Mus. Vic. 56, 145–234. doi: 10.24199/j.mmv.1997.56.08
Bruce N. L. (2005). Two New Species of the Mesopelagic Isopod Genus Syscenus Harger 1880 (Crustacea: Isopoda: Aegidae) From the Southwestern Pacific. Zootaxa 1070, 31–42. doi: 10.11646/zootaxa.1070.1.2
Bruce H. S., Patel N. H. (2020). Knockout of Crustacean Leg Patterning Genes Suggests That Insect Wings and Body Walls Evolved From Ancient Leg Segments. Nat. Ecol. Evol. 4, 1703–1712. doi: 10.1038/s41559-020-01349-0
Brunel P., Besner M., Messier D., Poirier L., Granger D., Weinstein M. (1978). Le Traîneau Macer-GIROQ: Appareil Amélioré Pour L’échantillonnage Quantitatif De La Petite Faune Nageuse Au Voisinage Du Fond. Int. Rev. Ges Hydrobiol. 63 (6), 815–829. doi: 10.1002/iroh.19780630612
Buhl-Jensen L. (1986). The Benthic Amphipod Fauna of the West-Norwegian Continental Shelf Compared With the Fauna of Five Adjacent Fjords. Sarsia 71, 193–208. doi: 10.1080/00364827.1986.10419690
Campean A. J., Coleman C. O. (2018). A New Species of Sicafodia Just 2004 (Crustacea, Amphipoda, Sicafodiidae) From the North Atlantic. Mar. Biodiver. 48, 939–948. doi: 10.1007/s12526-017-0635-1
Carrasón M., Matallanas J. (2001). Feeding Ecology of the Mediterranean Spiderfish, Bathypterois Mediterraneus (Pisces: Chlorophthalmidae), on the Western Mediterranean Slope. Fish. Bull. 99 (2), 266–274.
Cartes J. E., Sorbe J. C., Sardà F. (1994). Spatial Distribution of Deep-Sea Decapods and Euphausiids Near the Bottom in the Northwestern Mediterranean. J. Exp. Mar. Biol. Ecol. 179, 131–144. doi: 10.1016/0022-0981(94)90021-3
CBD, Secretariat of the Convention on Biological Diversity (2010). Guide to the Global Taxonomy Initiative. CBD Tech. Ser. 30 (1–195), i–viii.
Chardy P. (1979). Structure of Deep Sea Asellota Assemblages in the Bay of Biscay; Relationships With the Abyssal Environment. Ambio Special Rep. 6, 79–82.
Chiba S., Saito H., Fletcher R., Yogi T., Kayo M., Miyagi S., et al. (2018). Human Footprint in the Abyss: 30 Year Records of Deep-Sea Plastic Debris. Mar. Pol. 96, 204–212. doi: 10.1016/j.marpol.2018.03.022
Chim C. K., Tong S. J. (2020). Two New Species of Paratanaoid Tanaidaceans of the Family Incertae Sedis (Crustacea: Peracarida) From Polymetallic Nodule Fields in the Eastern Clarion-Clipperton Fracture Zone. Zootaxa 4758 (3), 461–485.
Clark M. R., Althaus F., Schlacher T. A., Williams A., Bowden D. A., Rowden A. A. (2016). The Impacts of Deep-Sea Fisheries on Benthic Communities: A Review. ICES J. Mar. Sci. 73 (1), i51–i69. doi: 10.1093/icesjms/fsv123
Coleman C. O. (2003). “Digital Inking”: How to Make Perfect Line Drawings on Computers. Org. Divers. Evol. 14, 1–14. doi: 10.1078/1439-6092-00081
Coleman C. O. (2006). Substituting Time-Consuming Pencil Drawings in Arthropod Taxonomy Using Stacks of Digital Photographs. Zootaxa 1360, 61–68. doi: 10.11646/zootaxa.1360.1.4
Coleman C. O. (2009). Drawing Setae the Digital Way. Zoosyst. Evol. 85 (2), 305–310. doi: 10.1002/zoos.200900008
Coleman C. O. (2015). Taxonomy in Times of the Taxonomic Impediment–Examples From the Community of Experts on Amphipod Crustaceans. J. Crust. Biol. 35 (6), 729–740. doi: 10.1163/1937240X-00002381
Corbari L., Frutos I., Sorbe J. C. (2019). Dorotea Gen. Nov., a New Bathyal Genus (Amphipoda, Eusiridae) From the Solomon Sea (Papua New Guinea). Zootaxa 4568, 69−80. doi: 10.11646/zootaxa.4568.1.4
Corbera J. (2006). “Arthropoda Cumacea,” in Handbook of Deep-Sea Hydrothermal Vent Fauna. Denisia, vol. 18. Eds. Desbruyères D. I., Segonzac M., Bright M., 370–371.
Corbera J., Martín D. (2002). Two New Cumacean Species (Crustacea: Peracarida) From Shallow Waters Off Thailand. Sci. Mar. 66, 407–415. doi: 10.3989/scimar.2002.66n4407
Corbera J., Segonzac M., Cunha M. R. (2008). A New Deep-Sea Genus of Nannastacidae (Crustacea, Cumacea) From the Lucky Strike Hydrothermal Vent Field (Azores Triple Junction, Mid-Atlantic Ridge). Mar. Biol. Res. 4 (3), 180–192. doi: 10.1080/17451000801898576
Cordiner C. (1793). Remarkable ruins, and romantic prospects, of North Britain. With ancient monuments, and singular subjects of natural history. Peter Mazell, London. 96 plates with letterpress.
Costa A. B., Silva M.B.da, Fraga R. E., Rocha A.A.da, Nishiyama P. B., Anjos M.S.d., et al. (2021). Evaluation of an Alternative Technique for Preserving Crustaceans in Dry Conditions With Joint Mobility: A Proposal for Didactic Purposes. Acta Sci. Biol. Sci. 43, e53450. doi: 10.4025/actascibiolsci.v43i1.53450
Costello M. J., Chaudhary C. (2017). Marine Biodiversity, Biogeography, Deep-Sea Gradients, and Conservation. Curr. Biol. 27 (11), R511–R527. doi: 10.1016/j.cub.2017.06.015
Curatolo T., Calvaruso C., Galil B. S., Lo Brutto S. (2013). Geometric Morphometry Supports a Taxonomic Revision of the Mediterranean Bathyporeia Guilliamsoniana (Spence Bate 1857) (Amphipoda, Bathyporeiidae). Crustaceana 86, 820–828. doi: 10.1163/15685403-00003217
Dallwitz M. J., Paine T. A., Zurcher E. J. (2000) Principles of Interactive Keys. Available at: http://delta-intkey.com (Accessed 5 July 2012).
Dauvin J. C., Sorbe J. C., Lorgeré J. C. (1995). The Benthic Boundary Layer Macrofauna From the Upper Continental Slope and the Cap-Ferret Canyon (Bay of Biscay). Oceanol. Acta 18, 113–122.
Dell’Anno A., Carugati L., Corinaldesi C., Riccioni G., Danovaro R. (2015). Unveiling the Biodiversity of Deep-Sea Nematodes Through Metabarcoding: Are We Ready to Bypass the Classical Taxonomy? PloS One 10 (12), e0144928. doi: 10.1371/journal.pone.0144928
Demidov O., Kihara T. C., Martinez Arbizu P., Clark P. F. (2021). The Megalopal Stage of the Hydrothermal Vent Crab Austinograea Rodriguezensis Tsuchida and Hashimoto 2002 (Decapoda: Bythograeidae): A Morphological Description Based on CLSM Images. Zootaxa 5040 (3), 365–387. doi: 10.11646/zootaxa.5040.3.3
Drumm D. T. (2005). Comparative Morphology of the Mouthparts, Chelipeds and Foregut of Two Kalliapseudid Apseudomorphans (Crustacea: Tanaidacea). P. Acad. Nat. Sci. Phil. 154, 137–147. doi: 10.1635/0097-3157(2004)154[0137:CMOTMC]2.0.CO;2
d’Udekem d’Acoz C., Verheye M. H. (2017). Epimeria of the Southern Ocean With Notes on Their Relatives (Crustacea, Amphipoda, Eusiroidea). Eur. J. Taxon. 359, 1–553. doi: 10.5852/ejt.2017.359
Dumke I., Purser A., Marcon Y., Nornes S. M., Johnsen G., Ludvigsen M., et al. (2018). Underwater Hyperspectral Imaging as an in Situ Taxonomic Tool for Deep-Sea Megafauna. Sci. Rep. 8 (1), 1–11. doi: 10.1038/s41598-018-31261-4
Dupérré N. (2020). Old and New Challenges in Taxonomy: What Are Taxonomists Up Against? Megataxa 1, 59–62. doi: 10.11646/megataxa.1.1.12
Easton E. E., Thiestle D. (2016). Do Some Deep-Sea, Sediment-Dwelling Species of Harpacicoid Copepods Have 1000-km-Scale Range Sizes? Mol. Ecol. 25, 4301–4318. doi: 10.1111/mec.13744
Eiler S. M., Haug C., Haug J. T. (2016). Detailed Description of a Giant Polychelidan Eryoneicus-Type Larva With Modern Imaging Techniques. Spixiana 39, 22–60.
Eltoum I., Fredenburgh J., Myers R. B., Grizzle W. E. (2001). Introduction to the Theory and Practice of Fixation of Tissues. J. Histotechnol. 24, 173–190. doi: 10.1179/his.2001.24.3.173
Engel M. S., Ceríaco L. M. P., Daniel G. M., Dellapé P. M., Löbl I., Marinov M., et al. (2021). The Taxonomic Impediment: A Shortage of Taxonomists, Not the Lack of Technical Approaches. Zool. J. Linn. Soc 193, 381–387. doi: 10.1093/zoolinnean/zlab072
Esquete P., Wilson G. D. F., Troncoso J. S. (2014). Ecology and Systematics of a New Species of Uromunna (Crustacea: Isopoda) From Spanish Eelgrass Beds. Helgol. Mar. Res. 68 (2), 329–339. doi: 10.1007/s10152-014-0393-4
Evers D. L., Fowler C. B., Cunningham B. R., Mason J. T., O’Leary T. J. (2011). The Effect of Formaldehyde Fixation on RNA: Optimization of Formaldehyde Adduct Removal. J. Mol. Diagn. 13 (3), 282–288. doi: 10.1016/j.jmoldx.2011.01.010
Fernández del Río L., Arwin H., Järrendahl K. (2016). Polarizing Properties and Structure of the Cuticle of Scarab Beetles From the Chrysina Genus. Phys. Rev. E 94, 12409. doi: 10.1103/PhysRevE.94.012409
Fontaine B., Perrard A., Bouchet P. (2012). Twenty-One Years of Shelf Life Between Discovery and Description of New Species. Curr. Biol. 22, 943–944. doi: 10.1016/j.cub.2012.10.029
Frank T. M., Widder E. A., Latz M. I., Case J. F. (1984). Dietary Maintenance of Bioluminescence in a Deep-Sea Mysid. J. Exp. Biol. 109, 385–389. doi: 10.1242/jeb.109.1.385
Frutos I. (2006). Estudio De Las Comunidades Suprabentónicas Submareales De La Ría De La Coruña Y Plataforma Continental Adyacente (NW Península Ibérica: Universidad de Alcalá).
Frutos I. (2017). “Mysida,” in Inventario De La Biodiversidad Marina De Galicia: Proyecto LEMGAL(Santiago de Compostela:Consellería do Mar, Xunta de Galicia), 443−448 pp.
Frutos I., Brandt A., Sorbe J. C., Orejas Saco del Valle C. (2017a). “Deep-Sea Suprabenthic Communities: The Forgotten Biodiversity,” in Marine Animal Forests. The Ecology of Benthos Biodiversity Hotspots. Eds. Rossi S., Bramanti L., Gori A. (Springer), 475–503 pp. doi: 10.1007/978-3-319-21012-4_21
Frutos I., Corbari L., Sorbe J. C. (2017b). Diversity of Deep-Sea Amphipoda From Papua New Guinea (SW Pacific Ocean). Biodivers. J. 8 (2), 505–506.
Frutos I., Sorbe J. C. (2010). Politolana Sanchezi Sp. Nov. (Crustacea: Isopoda: Cirolanidae), a New Benthic Bioturbating Scavenger From Bathyal Soft-Bottoms of the Southern Bay of Biscay Northeastern Atlantic Ocean. Zootaxa (Alicante, Spain:XVI SIEBM Abstract book) 2640, 20–34. doi: 10.11646/zootaxa.2640.1.2
Frutos I., Sorbe J. C. (2013). Leucothoe Cathalaa Sp. Nov. (Crustacea: Amphipoda: Leucothoidae) a New Bathyal Benthic Species From the Le Danois Bank (“El Cachucho” Spanish MPA), Southern Bay of Biscay. J. Mar. Biol. Assoc. UK 93 (3), 659–666.
Frutos I., Sorbe J. C. (2014). Bathyal Suprabenthic Assemblages From the Southern Margin of the Capbreton Canyon (“Kostarrenkala” Area), SE Bay of Biscay. Deep Sea Res. Pt. II Top. Stud. Oceanogr. 104, 291–309. doi: 10.1016/j.dsr2.2013.09.010
Frutos I., Sorbe J. C., Junoy J. (2010). “La Primera Especie Ciega Del Género Paranthura (Crustacea: Isopoda: Anthuridea) Habita En El Mar Cantábrico (N España),” in XVI Simposio Ibérico de Estudios de Biología Marina, Alicante (España, Septiembre 2010, Vol. 6–10.
Frutos I., Sorbe J. C., Junoy J. (2011). The First Blind Paranthura Species (Crustacea, Isopoda, Paranthuridae) From the “El Cachucho” Marine Protected Area (Le Danois Bank, Southern Bay of Biscay). Zootaxa 2971, 17–32. doi: 10.11646/zootaxa.2971.1.2
Fryer G. (1968). Evolution and Adaptive Radiation in the Chydoridae (Crustacea: Cladocera): A Study in Comparative Functional Morphology and Ecology. Phil. T. R. Soc London Ser. B. 254, 221–382+384. doi: 10.1098/rstb.1968.0017
Galassi D. M. P., De Laurentiis P., Giammatteo M. (1998). Integumental Morphology in Copepods: Assessment by Confocal Laser Scanning Microscopy (CLSM). Frag. Entomol. 30, 79–92.
Geiselbrecht H., Melzer R. R. (2013a). How do Mandibles Sense? – The Sensory Apparatus of Larval Mandibles in Palaemon Elegans Rathke 1837 (Decapoda, Palaemonidae). Arthropod. Struct. Dev. 42, 1–16. doi: 10.1016/j.asd.2012.09.001
Geiselbrecht H., Melzer R. R. (2013b). Nervous Systems in 3D: A Comparison of Caridean, Anomuran, and Brachyuran Zoea-I (Decapoda). J. Exp. Zool. B.: Mol. Dev. Evol. 320, 511–524. doi: 10.1002/jez.b.22528
Geiselbrecht H., Melzer R. R. (2014). Fine Structure and Ecdysis of Mandibular Sensilla Associated With the Lacinia Mobilis in Neomysis Integer (Leach 1814) (Crustacea, Malacostraca, Peracarida). Arthropod. Struct. Dev. 43, 221–230. doi: 10.1016/j.asd.2014.01.002
Gellert M., Bird G. J., Stępień A., Studzian M., Błażewicz M. (2022). A Hidden Diversity in the Atlantic and the SE Pacific: Hamatipedidae N. Fam. Front. Mar. Sci. 8, 773437. doi: 10.3389/fmars.2021.773437
Giurginca A., Šustr V., Tajovsky K., Giurginca M., Matei I. (2015). Spectroscopic Parameters of the Cuticle and Ethanol Extracts of the Fluorescent Cave Isopod Mesoniscus Graniger (Isopoda, Oniscidea). ZooKeys 515, 111–125. doi: 10.3897/zookeys.515.9395
Glenn D., Caldwell R. L., Pakes M. J. (2013). Fluorescence in Arthropoda Informs Ecological Studies in Anchialine Crustaceans, Remipedia, and Atyidae. J. Crust. Biol. 33, 620–626. doi: 10.1163/1937240X-00002170
Glover A. G., Higgs N., Horton T. (2021). World Register of Deep-Sea Species (WoRDSS). doi: 10.14284/352
Glover A. G., Wiklund H., Chen C., Dahlgren T. G. (2018). Point of View: Managing a Sustainable Deep-Sea ‘Blue Economy’requires Knowledge of What Actually Lives There. Elife 7, e41319. doi: 10.7554/eLife.41319.005
Gooday A. J., Sykes D., Góral T., Zubkov M. V., Glover A. G. (2018). Micro-CT 3D Imaging Reveals the Internal Structure of Three Abyssal Xenophyophore Species (Protista, Foraminifera) From the Eastern Equatorial Pacific Ocean. Sci. Rep. 8 (1), 1–12. doi: 10.1038/s41598-018-30186-2
Göpel T., Wirkner C. S. (2018). Morphological Description, Character Conceptualization and the Reconstruction of Ancestral States Exemplified by the Evolution of Arthropod Hearts. PloS One 13, e0201702. doi: 10.1371/journal.pone.0201702
Grams M., Richter S. (2021). Locomotion in Anaspides (Anaspidacea, Malacostraca) – Insights From a Morpho-Functional Study of Thoracopods With Some Observations on Swimming and Walking. Zoology 144, 125883. doi: 10.1016/j.zool.2020.125883
Grassle J. F., Maciolek N. J. (1992). Deep-Sea Species Richness: Regional and Local Diversity Estimates From Quantitative Bottom Samples. Am. Nat. 139 (2), 313–341. doi: 10.1086/285329
Guidi-Guilvard L. D., Thistle D., Khripounoff A. (2007). Two-Year Temporal Variability of Small Hyperbenthos Collected 4 M Above the Bottom in the Deep, (2347 M) NW Mediterranean. ICES 05, 5.
Gutiérrez Y., Ott D., Töpperwien M., Salditt T., Scherber C. (2018). X-Ray Computed Tomography and its Potential in Ecological Research: A Review of Studies and Optimization of Specimen Preparation. Ecol. Evol. 8, 7717–7732. doi: 10.1002/ece3.4149
Hadjab R., Ayati K., Piscart C. (2020). A New Species of Freshwater Amphipods Echinogammarus (Amphipoda, Gammaridae) From Algeria. Taxonomy 1, 36–47. doi: 10.3390/taxonomy1010005
Hanafi-Portier M., Corbari L., Chan T.-Y., Chen W.-J., Chen J.-N., Lee M.-Y., et al. (2021). When Imagery and Physical Sampling Work Together: Towards an Integrative Methodology of Image-Based Megafauna Identification. Front. Mar. Sci. 8, 749078. doi: 10.3389/fmars.2021.749078
Haug J. T., Haug C., Kutschera V., Mayer G., Maas A., Liebau S., et al. (2011b). Autofluorescence Imaging, an Excellent Tool for Comparative Morphology: Autofluorescence Imaging. J. Microsc. Oxford 244, 259–272. doi: 10.1111/j.1365-2818.2011.03534.x
Haug C., Mayer G., Kutschera V., Waloszek D., Maas A., Haug J. T. (2011a). Imaging and Documenting Gammarideans. Int. J. Zool. doi: 10.1155/2011/380829
Havermans C., Sonet G., d’Udekem d’Acoz C., Nagy Z. T., Martin P., Brix S., et al. (2013). Genetic and Morphological Divergences in the Cosmopolitan Deep-Sea Amphipod Eurythenes Gryllus Reveal a Diverse Abyss and a Bipolar Species. PloS One 8, e74218. doi: 10.1371/journal.pone.0074218
Hegna T. A. (2010). Photography of Soft-Bodied Crustaceans via Drying, Whitening, and Splicing. J. Crust. Biol. 30, 351–356. doi: 10.1651/09-3253.1
Herring P. J. (1981). Studies on Bioluminescent Marine Amphipods. J. Mar. Biol. Assoc. U.K. 61 (1), 161–176. doi: 10.1017/S0025315400045999
Hessler R. R., Jumars P. A. (1974). Abyssal Community Analysis From Replicate Cores in the Central North Pacific. Deep Sea Res. Oceanogr. Abstr. 21 (3), 185–209. doi: 10.1016/0011-7471(74)90058-8
Hessler R. R., Sanders H. L. (1967). Faunal Diversity in the Deep-Sea. Deep Sea Res. Part I Oceanogr. Res. 14(1), 65–78. doi: 10.1016/0011-7471(67)90029
Hessler R. R., Wilson G. D. F. (1983). “The Origin and Biogeography of Malacostracan Crustaceans in the Deep Sea,” in Evolution, Time, and Space: The Emergence of the Biosphere, vol. 23 . Eds. Sims R. W., Price J. H., Whalley P. E. S. (Whalley: Systematics Association, Special Publication), 227–254.
Higgs N. D., Attrill M. (2015). Biases in Biodiversity: Wide-Ranging Species Are Discovered First in the Deep Sea. Front. Mar. Sci. 2. doi: 10.3389/fmars.2015.00061
Holthuis L. B. (1964). The Earliest Published Record of a Cumacean. Crustaceana 7, 317–318. doi: 10.1163/156854064X00533
Horton T., Marsh L., Bett B. J., Gates A. R., Jones D. O. B., Benoist N. M. A., et al. (2021). Recommendations for the Standardisation of Open Taxonomic Nomenclature for Image-Based Identifications. Front. Mar. Sci. 8. doi: 10.3389/fmars.2021.620702
Horton T., Thurston M. H., Vlierboom R., Gutteridge Z., Pebody C. A., Gates A. R., et al. (2020). Are Abyssal Scavenging Amphipod Assemblages Linked to Climate Cycles? Prog. Oceanogr. 184, 102318. doi: 10.1016/j.pocean.2020.102318
Hughes L. E., Ahyong S. T. (2016). Collecting and Processing Amphipods. J. Crust. Biol. 36, 584–588. doi: 10.1163/1937240X-00002450
Hughes L. E., Kaji T. (2016). Description of a New Species of Quadrivisio Stebbing 1907, From Songkhla Lake, Thailand (Crustacea: Peracarida: Amphipoda: Maeridae). Raffles B. Zool. 9 (64), 351–359.
ISA (2020). Workshop on Deep-Sea Taxonomic Standardization: Strategic Approaches for Collaboration. ISA Workshop, 15–16.
Jażdżewska A. M., Brandt A., Arbizu P. M., Vink A. (2021). Exploring the Diversity of the Deep Sea — Four New Species of the Amphipod Genus Oedicerina Described Using Morphological and Molecular Methods. Zool. J. Linn. Soc 194(1), 181–225. doi: 10.1093/zoolinnean/zlab032 .
Jakiel A., Palero F., Błażewicz M. (2019). Deep Ocean Seascape and Pseudotanaidae (Crustacea: Tanaidacea) Diversity at the Clarion-Clipperton Fracture Zone. Sci. Rep. 9, 17305. doi: 10.1038/s41598-019-51434-z
Jakiel A., Palero F., Błażewicz M. (2020). Secrets From the Deep: Pseudotanaidae (Crustacea: Tanaidacea) Diversity From the Kuril-Kamchatka Trench. Prog. Oceanogr. 183, 102288. doi: 10.1016/j.pocean.2020.102288
Jakiel A., Stępień A., Błażewicz M. (2018). A Tip of the Iceberg – Pseudotanaidae (Tanaidacea) Diversity in the North Atlantic. Mar. Biodiv. 48, 859–895. doi: 10.1007/s12526-018-0881-x
Jamieson A. J. (2016). “Landers: Baited Cameras and Traps,” in Biological Sampling in the Deep Sea. Eds. Clark M. R., Consalvey M., Rowden A. A. (John Wiley and Sons Ltd), 228–259 pp.
Jamieson A. J., Singleman G., Linley T. D., Casey S. (2021). Fear and Loathing of the Deep Ocean: Why Don’t People Care About the Deep Sea? ICES J. Mar. Sci. 78 (3), 797–809. doi: 10.1093/icesjms/fsaa234
Jaume D., Queinneck E. (2007). A New Species of Freshwater Isopod (Sphaeromatidea: Sphaeromatidae) From an Inland Karstic Stream on Espíritu Santo Island, Vanuatu, Southwestern Pacific. Zootaxa 1653, 41–55. doi: 10.11646/zootaxa.1653.1.3
Jauvion C. (2020). De La Vie a La Pierre: Préservation Exceptionnelle D’arthropodes Marins Fossils (MNHN Paris: Museum national d’histoire naturelle), 429 pp.
Jauvion C., Audo D., Charbonnier S., Vannier J. (2016). Virtual Dissection and Lifestyle of a 165 - Million-Year-Old Female Polychelidan Lobster. Arthropod. Struct. Dev. 45, 122–132. doi: 10.1016/j.asd.2015.10.004
Jennings R. M., Brix S., Bober S., Svavarsson J., Driskell A. (2018). More Diverse Than Expected: Distributional Patterns of Oecidiobranchus Hessler 1970 (Isopoda, Asellota) on the Greenland-Iceland-Faeroe Ridge Based on Molecular Markers. Mar. Biodiv. 48 (2), 845–857. doi: 10.1007/s12526-018-0857-x
Jersabek C. D. (2005). The ‘Frank J. Myers Rotifera Collection’ at the Academy of Natural Sciences of Philadelphia. Hydrobiol 546, 137–140. doi: 10.1007/s10750-005-4110-9
Jirikowski G., Richter S., Wolff C. (2013). Myogenesis of Malacostraca — the “Egg-Nauplius” Conceptrevisited. Front. Zool. 10, 76. doi: 10.1186/1742-9994-10-76
Jirikowski G., Wolff C., Richter S. (2015). Evolution of Eumalacostracan Development — New Insights Into Loss and Reacquisition of Larval Stages Revealed by Heterochrony Analysis. Evo. Devo 6, 4. doi: 10.1186/2041-9139-6-4
Jörger K. M., Schrödl M. (2013). How to Describe a Cryptic Species? Practical Challenges of Molecular Taxonomy. Front. Zool. 10 (1), 1–27. doi: 10.1186/1742-9994-10-59
Jóźwiak P., Pabis K., Brandt A., Błażewicz M. (2020). Epibenthic Sled Versus Giant Box Corer – Comparison of Sampling Gears for Tanaidacean Species Richness Assessment in the Abyssal Benthic Ecosystem. Prog. Oceanogr. 181. doi: 10.1016/j.pocean.2019.102255
Just J. (2001). Bathyal Joeropsididae (Isopoda: Asellota) From South-Eastern Australia, With Description of Two New Genera. Mem. Mus. Vic. 58 (2), 297–333. doi: 10.24199/j.mmv.2001.58.16
Kaiser S., Brandt A. (2007). Two New Species of the Genus Austroniscus Vanhoeffen 1914 (Isopoda: Asellota: Nannoniscidae) From the Antarctic Shelf. Zootaxa 1394, 47–68. doi: 10.11646/zootaxa.1394.1.3
Kaiser S., Brenke N. (2016). “Epibenthic Sledges,” in Biological Sampling in the Deep Sea. Eds. Clark M. R., Consalvey M., Rowden A. A. (John Wiley and Sons Ltd), 184–206 pp.
Kaiser S., Brix S., Kihara T. C., Janssen A., Jennings R. M. (2018). Integrative Species Delimitation in the Deep-Sea Genus Thaumastosoma Hessler 1970 (Isopoda, Asellota, Nannoniscidae) Reveals a New Genus and Species From the Atlantic and Central Pacific Abyss. Deep Sea Res. Pt. II Top. Stud. Oceanogr. 148, 151–179. doi: 10.1016/j.dsr2.2017.05.006
Kaiser S., Kihara T. C., Brix S., Mohrbeck I., Janssen A., Jennings R. M. (2021). Species Boundaries and Phylogeographic Patterns in New Species of Nannoniscus (Janiroidea: Nannoniscidae) From the Equatorial Pacific Nodule Province Inferred From mtDNA and Morphology. Zool. J. Linn. Soc, 193(3), 1020–1071. doi: 10.1093/zoolinnean/zlaa174
Kaiser S., Marner M. (2012). A New Species of Pentaceration Just 2009 (Isopoda, Asellota, Paramunnidae) From the Challenger Plateau, New Zealand (Tasman Sea). Zoosystem Evol. 88, 171–184. doi: 10.1002/zoos.201200015
Kaji T., Fritsch M., Schwentner M., Olesen J., Richter S. (2014). Male Claspers in Clam Shrimps (Crustacea, Branchiopoda) in the Light of Evolution: A Case Study on Homology Versus Analogy: Male Claspers in Clam Shrimps. J. Exp. Zool. (Mol. Dev. Evol.) 322, 269–280. doi: 10.1002/jez.b.22574
Kaji T., Kakui K., Miyazaki N., Murata K., Palmer A. R. (2016). Mesoscale Morphology at Nanoscale Resolution: Serial Block-Face Scanning Electron Microscopy Reveals Fine 3D Detail of a Novel Silk Spinneret System in a Tube-Building Tanaid Crustacean. Front. Zool. 13, 14. doi: 10.1186/s12983-016-0146-0
Kakui K. (2014). A Novel Transmission Pathway: First Report of a Larval Trematode in a Tanaidacean Crustacean. Fauna Ryukyuana 17, 13–22.
Kakui K., Fujiwara Y. (2020). First In Situ Observations of Behavior in Deep-Sea Tanaidacean Crustaceans. Zool. Sci. 37, 1–4. doi: 10.2108/zs200028
Kakui K., Hiruta C. (2017). Tube Construction by a Tanaidacean Crustacean Using a Novel Mucus Secretion System Involving the Anal Opening. Zool. Lett. 3, 20. doi: 10.1186/s40851-017-0082-7
Kakui K., Kano Y. (2021). First Complete Mitochondrial Genome of a Tanaidacean Crustacean (Arctotanais Alascensis). Zool. Sci. 38 (3), 267–272. doi: 10.2108/zs200167
Kamanli S. A., Kihara T. C., Ball A. D., Morritt D., Clark P. F. (2017). A 3D Imaging and Visualization Workflow, Using Confocal Microscopy and Advanced Image Processing for Brachyuran Crab Larvae: 3D Imaging of Crab Larvae Using CLSM. J. Microsc. Oxford 266, 307–323. doi: 10.1111/jmi.12540
Kelley C., Kerby T., Sarradin P.-M., Sarrazin J., Lindsay D. J. (2016). “Submersibles and Remotely Operated Vehicles,” in Biological Sampling in the Deep Sea. Eds. Clark M. R., Consalvey M., Rowden A. A. (John Wiley and Sons Ltd), 285–305 pp.
Kenning M., Harzsch S. (2013). Brain Anatomy of the Marine Isopod Saduria Entomon Linnaeus 1758 (Valvifera, Isopoda) With Special Emphasis on the Olfactory Pathway. Front. Neuroanat. 7. doi: 10.3389/fnana.2013.00032
Kensley B. (1989). Marine Isopod Crustaceans From the St. Paul and Amsterdam Islands, Southern Indian Océan. Bull. Mus. Natn. Hist. Nat. 4° Sér. 11 (1), 147–164.
Khodami S., Mercado-Salas N. F., Martinez Arbizu P. (2020). Genus Level Molecular Phylogeny of Aegisthidae Gisbrecht 1893 (Copepoda: Harpacticoida) Reveals Morphological Adaptations to Deep-Sea and Plagic Habitats. BMC Evol. Biol. 20, 36. doi: 10.1186/s12862-020-1594-x
Kihara T. C., Martinez Arbizu P. (2012). Three New Species of Cerviniella Smirnov 1946 (Copepoda: Harpacticoida) From the Arctic. Zootaxa 3345, 1–33. doi: 10.11646/zootaxa.3345.1.1
King J. R., Porter S. D. (2004). Recommendations on the Use of Alcohols for Preservation of Ant Specimens (Hymenoptera, Formicidae). Insect. Soc. 51, 197–202. doi: 10.1007/s00040-003-0709-x
Kodama M., Kawamura T. (2019). A New Species of Bemlos Shoemaker 1925 (Amphipoda: Aoridae) From Deep Water Off Tanabe Bay, Japan, With a Review of the Deep-Sea Aorids and Their Adaptations to the Deep Sea. J. Crust. Biol. 39, 54–61. doi: 10.1093/jcbiol/ruy098
Koomen P., von Vaupel Klein J. C. (1995). The Suitability of Various Mounting Media for Permanent Mounts of Small Chitinous Crustaceans, With Special Reference to the Observation of Integumental Organs. Crustaceana 68, 428–437. doi: 10.1163/156854095X01583
Kottmann J., Kihara T. C., Glatzel T., Veit-Köhler G. (2013). A New Species of Wellsopsyllus (Copepoda, Harpacticoida, Paramesochridae) From the Deep Southern Ocean and Remarks on its Biogeography. Helgol. Mar. Res. 67, 33–48. doi: 10.1007/s10152-012-0302-7
Kraft A., Bauerfeind E., Nöthig E.-M., Klages M., Beszczynska-Möller A., Bathmann U. V. (2013). Amphipods in Sediment Traps of the Eastern Fram Strait With Focus on the Life-History of the Lysianassoid Cyclocaris Guilelmi. Deep Sea Res. Part I. Oceanogr. Res. 73, 62–72. doi: 10.1016/j.dsr.2012.11.012
Kreissl S., Uber A., Harzsch S. (2008). Muscle Precursor Cells in the Developing Limbs of Two Isopods (Crustacea, Peracarida): An Immunohistochemical Study Using a Novel Monoclonal Antibody Against Myosin Heavy Chain. Dev. Genes. Evol. 218, 253–265. doi: 10.1007/s00427-008-0216-1
Kürten B., Frutos I., Struck U., Painting S. J., Polunin N. V. C., Middelburg J. J. (2013). Trophodynamics and Functional Feeding Groups of North Sea Fauna: A Combined Stable Isotope and Fatty Acid Approach. Biogeochemistry 113, 189–212. doi: 10.1007/s10533-012-9701-8
Kürzel K., Kaiser S., Lörz A. N., Rossel S., Paulus E., Peters J., et al. (2022). Correct Species Identification and Its Implications for Conservation Using Haploniscidae (Crustacea, Isopoda) in Icelandic Waters as a Surrogate. Front. Mar. Sci. 8, 795196. doi: 10.3389/fmars.2021.795196
Lagardère J. P. (1977). Recherches Sur La Distribution Vertical Et Sur L’alimentation Des Crustacés Décapodes Benthiques De La Pente Continentale Du Golfe De Gascogne. Bull. Cent. Etud. Rech. Sci. Biarritz 11 (4), 367–440.
Lambshead P. J. D., Boucher G. (2003). Marine Nematode Deep-Sea Biodiversity–Hyperdiverse or Hype? J. Biogeogr. 30 (4), 475–485. doi: 10.1046/j.1365-2699.2003.00843.x
Landschoff J., Komai T., du Plessis A., Gouws G., Griffiths C. L. (2018). MicroCT Imaging Applied to Description of a New Species of Pagurus Fabricius 1775 (Crustacea: Decapoda: Anomura: Paguridae), With Selection of Three-Dimensional Type Data. PloS One 13, e0203107. doi: 10.1371/journal.pone.0203107
Langenkämper D., Zurowietz M., Schoening T., Nattkemper T. W. (2017). Biigle 2.0-Browsing and Annotating Large Marine Image Collections. Front. Mar. Sci. 4, 83. doi: 10.3389/fmars.2017.00083
Larsen K. (2001). Morphological and Molecular Investigation of Polymorphism and Cryptic Species in Tanaid Crustaceans: Implications for Tanaid Systematics and Biodiversity Estimates. Zool. J. Linn. Soc 131, 353–379. doi: 10.1111/j.1096-3642.2001.tb02241.x
Lee S., Brown R. L., Monroe W. (2009). Use of Confocal Laser Scanning Microscopy in Systematics of Insects With a Comparison of Fluorescence From Different Stains. Syst. Entomol. 34, 10–14. doi: 10.1111/j.1365-3113.2008.00451.x
Le J. T., Levin L. A., Lejzerowicz F., Cordier T., Gooday A. J., Pawlowski J. (2021). Scientific and Budgetary Tradeoffs Between Morphological and Molecular Methods for Deep-Sea Biodiversity Assessment. Integr. Environ. Assess. Manage 18(3), 665–663. doi: 10.1002/ieam.4466
Lepechin I. (1780). Tres Oniscorum Species Descriptae. Acta Acad. Sci. Imperialis Petropolitanae 1778, 248–249.
Lester P. J., Brown S. D. J., Edwards E. D., Holwell G. I., Pawson S. M., Ward D. F., et al. (2014). Critical Issues Facing New Zealand Entomology. N. Z. Entomol. 37 (1), 1–13. doi: 10.1080/00779962.2014.861789
Lim G. S., Balke M., Meier R. (2012). Determining Species Boundaries in a World Full of Rarity: Singletons, Species Delimitation Methods. Syst. Biol. 61 (1), 165–169. doi: 10.1093/sysbio/syr030
Linnaeus C. (1735). Systema Naturae; Sive, Regna Tria Naturae: Systematice Proposita Per Classes, Ordines, Genera and Species. Haak.
Lins L., Brandt A. (2020). Comparability Between Box-Corer and Epibenthic-Sledge Data on Higher Taxon Level: A Case Study Based on Deep-Sea Samples From the NW Pacific. Prog. Oceanogr. 182, 102273. doi: 10.1016/j.pocean.2020.102273
Lins L., Zeppilli D., Menot L., Michel L. N., Bonifácio P., Brandt M., et al. (2021). Toward a Reliable Assessment of Potential Ecological Impacts of Deep-Sea Polymetallic Nodule Mining on Abyssal Infauna. Limnol. Oceanogr. Meth. 19, 626–650. doi: 10.1002/lom3.10448
Li J. Y., Song Z. L., Yan G. Y., He L. S. (2019). The Complete Mitochondrial Genome of the Largest Amphipod, Alicella Gigantea: Insight Into Its Phylogenetic Relationships and Deep Sea Adaptive Characters. Int. J. Biol. Macromol. 141, 570–577. doi: 10.1016/j.ijbiomac.2019.09.050
Lörz A.-N., Horton T. (2021). Investigation of the Amathillopsidae (Amphipoda, Crustacea), Including the Description of a New Species, Reveals a Clinging Lifestyle in the Deep Sea Worldwide. ZooKeys 1031, 19–39. doi: 10.3897/zookeys.1031.62391
Lowry J. K., Myers A. A. (2012). Podosiridae, a New Family of North Atlantic Deep Sea Amphipod (Crustacea, Amphipoda). Zootaxa 3546 (1), 81–84. doi: 10.11646/zootaxa.3546.1.6
Luque J., Gerken S. (2019). Exceptional Preservation of Comma Shrimp From a Mid-Cretaceous Lagerstätte of Colombia, and the Origins of Crown Cumacea. Proc. R. Soc. B. 286, 20191863. doi: 10.1098/rspb.2019.1863
Luque J., Xing L., Briggs D. E. G., Clark E. G., Duque A., Hui J., et al. (2021). Crab in Amber Reveals an Early Colonization of Nonmarine Environments During the Cretaceous. Sci. Adv. 7 (43), eabj5689. doi: 10.1126/sciadv.abj5689
Macel M.-L., Ristoratore F., Locascio A., Spagnuolo A., Sordino P., D’Aniello S. (2020). Sea as a Color Palette: The Ecology and Evolution of Fluorescence. Zool. Lett. 6, 9. doi: 10.1186/s40851-020-00161-9
MacIsaac K. G., Kenchington T. J., Kenchington E. L. R., Best M. (2014). The Summer Assemblage of Large Pelagic Crustacea in the Gully Submarine Canyon: Major Patterns. Deep Sea Res. Pt. II Top. Stud. Oceanogr. 104, 51–66. doi: 10.1016/j.dsr2.2013.08.017
Maeno A., Kohtsuka H., Takatani K., Nakano H. (2019). Microfocus X-Ray CT (microCT) Imaging of Actinia Equina (Cnidaria), Harmothoe Sp. (Annelida), and Xenoturbella Japonica (Xenacoelomorpha). J. Vis. Exp., 150 e59161. doi: 10.3791/59161
Malyutina M., Frutos I., Brandt A. (2018). Diversity and Distribution of the Deep-Sea Atlantic Acanthocope (Crustacea, Isopoda, Munnopsidae), With Description of Two New Species. Deep Sea Res. Pt. II Top. Stud. Oceanogr. 148, 130−150. doi: 10.1016/j.dsr2.2017.11.003
Malyutina M. V., Kihara T. C., Brix S. (2020). A New Genus of Munnopsidae Lilljeborg 1864 (Crustacea, Isopoda), With Descriptions of Two Abyssal New Species From the Clarion Clipperton Fracture Zone, North-Eastern Tropical Pacific. Mar. Biodiv. 50, 42. doi: 10.1007/s12526-020-01061-z
Manktelow M. (2010). “History of Taxonomy,” in Lecture From Dept. Of Systematic Biology (Uppsala University).
Marek P. (2017). Ultraviolet-Induced Fluorescent Imaging for Millipede Taxonomy. Res. Ideas Outcomes 3, e14850. doi: 10.3897/rio.3.e14850
Marquina D., Buczek M., Ronquist F., Łukasik P. (2021). The Effect of Ethanol Concentration on the Morphological and Molecular Preservation of Insects for Biodiversity Studies. Peer J 9, e10799 2020. doi: 10.7717/peerj.10799
Martin J. W. (2016). Collecting and Processing Crustaceans: An Introduction. J. Crust. Biol. 36, 393–395. doi: 10.1163/1937240X-00002436
Martínez Arbizu P., Petrunina (2018). Two New Species of Tantulocarida From the Atlantic Deep Sea With First CLSM Pictures of Tantulus Larva. Mar. Biodiv. 48 (1), 231–237. doi: 10.1007/s12526-016-0627-6
Martin J. W., France S. C., Van Dover C. L. (1993). Halice Hesmonectes, a New Species of Pardaliscid Amphipod (Crustacea, Peracarida) From Hydrothermal Vents in the Eastern Pacific. Can. J. Zool. 71, 1724–1732. doi: 10.1139/z93-244
Maruzzo D., Minelli A., Ronco M., Fusco G. (2007). Growth and Regeneration of the Second Antennae of Asellus Aquaticus (Isopoda) in the Context of Arthropod Antennal Segmentation. J. Crust. Biol. 27, 184–196. doi: 10.1651/S-2756.1
Maybury C., Morrison L., Stewart V. (1991). The Search for a Reliable Mounting Medium for Recent ‘Live’ Foraminifera. J. Micropalaeontol. 9, 172–172. doi: 10.1144/jm.9.2.172
Mayr E. (1942). Systematics and the Origin of Species (New York: Columbia University Press), 315 pp.
McMenamin M. A. S., Zapata L. P., Hussey M. C. (2013). A Triassic Giant Amphipod From Nevada, USA. J. Crust. Biol. 33 (6), 751–759. doi: 10.1163/1937240X-00002192
Meißner K., Bick A., Götting M. (2017). Arctic Pholoe (Polychaeta: Pholoidae): When Integrative Taxonomy Helps to Sort Out Barcodes. Zool. J. Linn. Soc 179 (2), 237–262. doi: 10.1111/zoj.12468
Meier R., Blaimerb B. B., Buenaventura E., Hartopb E., vonRintelen T., Srivathsana A., et al. (2022). A Re-Analysis of the Data in Sharkey Et Al.’s, (2021) Minimalist Revision Reveals That BINs do Not Deserve Names, But BOLD Systems Needs a Stronger Commitment to Open Science. Cladistics, 38, 264–275. doi: 10.1111/cla.12489
Melzer R. R., Spitzner F., Šargač Z., Hörnig M. K., Krieger J., Haug C., et al. (2021). Methods to Study Organogenesis in Decapod Crustacean Larvae II: Analysing Cells and Tissues. Helgol. Mar. Res. 75, 2. doi: 10.1186/s10152-021-00547-y
Menzel L. (2011). First Descriptions of Copepodid Stages, Sexual Dimorphism and Intraspecific Variability of Mesocletodes Sars 1909 (Copepoda, Harpacticoida, Argestidae), Including the Description of a New Species With Broad Abyssal Distribution. ZooKeys 96, 39–80. doi: 10.3897/zookeys.96.1496
Michels J. (2007). Confocal Laser Scanning Microscopy: Using Cuticular Autofluorescence for High Resolution Morphological Imaging in Small Crustaceans. J. Microsc. Oxford 227, 1–7. doi: 10.1111/j.1365-2818.2007.01787.x
Michels J., Büntzow M. (2010). Assessment of Congo Red as a Fluorescence Marker for the Exoskeleton of Small Crustaceans and the Cuticle of Polychaetes. J. Microsc. 238 (2), 95–101. doi: 10.1111/j.1365-2818.2009.03360.x
Mohrbeck I., Horton T., Jażdżewska A. M., Arbizu P. M. (2021). DNA Barcoding and Cryptic Diversity of Deep-Sea Scavenging Amphipods in the Clarion-Clipperton Zone (Eastern Equatorial Pacific). Mar. Biodiv. 51 (2), 1–15. doi: 10.1007/s12526-021-01170-3
Mohrbeck I., Raupach M. J., Martínez Arbizu P., Knebelsberger T., Laakmann S. (2015). High-Throughput Sequencing—The Key to Rapid Biodiversity Assessment of Marine Metazoa? PloS One 10 (10), e0140342. doi: 10.1371/journal.pone.0140342
Montesanto G. (2015). A Fast GNU Method to Draw Accurate Scientific Illustrations for Taxonomy. ZooKeys 515, 191–206. doi: 10.3897/zookeys.515.9459
Mora C., Tittensor D. P., Adl S., Simpson A. G., Worm B. (2011). How Many Species Are There on Earth and in the Ocean? PloS Biol. 9 (8), e1001127.
Moreira P. S. (1973). Especies De Isopoda (Crustacea, Peracarida). Programa Rio Grande Do Sul II. Parte I, Publicaçao Especial do Instituto Oceanografico. 3(2), 213–229.
Mrak P., Žnidaršič N., Štrus J. (2013). Alizarin Red S Staining of the Crustacean Cuticle: Implementation in the Study of Porcellio Scaber Larvae. ABS 56, 51–62.
Mrak P., Žnidaršič N., Tušek-Žnidarič M., Klepal W., Gruber D., Strus J. (2012). Egg Envelopes and Cuticle Renewal in Porcellio Embryos and Marsupial Mancas. ZooKeys 176, 55–72. doi: 10.3897/zookeys.176.2418
Murray J., Renard A. F. (1891). Report on Deep-Sea Deposits Based on the Specimens Collected During the Voyage of HMS Challenger in the Years 1872 to 1876 (HM Stationery Office).
Nagler C., Haug J. T. (2016). Functional Morphology of Parasitic Isopods: Understanding Morphological Adaptations of Attachment and Feeding Structures in Nerocila as a Pre-Requisite for Reconstructing the Evolution of Cymothoidae. PeerJ 4, e2188. doi: 10.7717/peerj.2188
Nagler C., Hyžný M., Haug J. T. (2017). 168 Million Years Old “Marine Lice” and the Evolution of Parasitism Within Isopods. BMC Evol. Biol. 17, 76. doi: 10.1186/s12862-017-0915-1
Narayanaswamy B. E., Bett B. J., Lamont P. A., Rowden A. A., Bell E. M., Menot L. (2016). “Corers and Grabs,” in Biological Sampling in the Deep Sea. Eds. Clark M. R., Consalvey M., Rowden A. A. (John Wiley and Sons Ltd), 207–227 pp.
Naskrecki P. (2008) Mantis V. 2.0 - A Manager of Taxonomic Information and Specimens. Available at: http://insects.oeb.harvard.edu/mantis (Accessed 30 July 2012).
Neuhaus B., Schmid T., Riedel J. (2017). Collection Management and Study of Microscope Slides: Storage, Profiling, Deterioration, Restoration Procedures, and General Recommendations. Zootaxa 4322, 1–173. doi: 10.11646/zootaxa.4322.1.1
Neusser T. P., Jörger K. M., Lodde-Bensch E., Strong E. E., Schrödl M. (2016). The Unique Deep Sea—Land Connection: Interactive 3D Visualization and Molecular Phylogeny of Bathyhedyle Boucheti N. Sp. (Bathyhedylidae N. Fam.)—The First Panpulmonate Slug From Bathyal Zones. PeerJ 4, e2738. doi: 10.7717/peerj.2738
Nouvel H., Lagardère J. P. (1976). Les Mysidacés Du Talus Continental Du Golfe De Gascogne I. Tribu Des Erythropini (Genre Erythrops Excepté). Bull. Mus. Natn. Hist. Nat. 414 (291), 1243–1324.
Palero F., Hall S., Clark P. F., Johnston D., Mackenzie-Dodds J., Thatje S. (2010). DNA Extraction From Formalin-Fixed Tissue: New Light From the Deep Sea. Sci. Mar. 74, 465–470. doi: 10.3989/scimar.2010.74n3465
Pante E., Schoelinck C., Puillandre N. (2015). From Integrative Taxonomy to Species Description: One Step Beyond. Syst. Biol. 64, 152–160. doi: 10.1093/sysbio/syu083
Papiol V., Cartes J. E., Vélez-Belchí P., Martín-Sosa P. (2019). Near-Bottom Zooplankton Over Three Seamounts in the East Canary Islands: Influence of Environmental Variables on Distribution and Composition. Deep Sea Res. Part I. Oceanogr. Res. 149, 1030025. doi: 10.1016/j.dsr.2019.04.003
Paulus E., Brix S., Siebert A., Arbizu P. M., Rossel S., Peters J., et al. (2021). Recent Speciation and Hybridization In Icelandic Deep-Sea Isopods: An Integrative Approach Using Genomics and Proteomics. Authorea Preprints.
Pawlowski J., Kelly-Quinn M., Altermatt F., Apothéloz-Perret-Gentil L., Beja P., Boggero A., et al. (2018). The Future of Biotic Indices in the Ecogenomic Era: Integrating (E) DNA Metabarcoding in Biological Assessment of Aquatic Ecosystems. Sci. Tot. Environ. 637, 1295–1310. doi: 10.1016/j.scitotenv.2018.05.002
Pereira E., Roccatagliata D., Doti B. L. (2019). Xiphoarcturus – a New Genus and Two New Species of the Family Antarcturidae (Isopoda: Valvifera) From the Mar Del Plata Submarine Canyon and its Phylogenetic Relationships. Arthropod. Syst. Phyl. 77, 303–323. doi: 10.26049/ASP77-2-2019-07
Pinu F. R., Beale D. J., Paten A. M., Kouremenos K., Swarup S., Schirra H. J., et al. (2019). Systems Biology and Multi-Omics Integration: Viewpoints From the Metabolomics Research Community. Metabolites 9 (4), 76. doi: 10.3390/metabo9040076
Pokluda P., Čížek L., Stříbrná E., Drag L., Lukeš J., Novotný V. (2014). A Goodbye Letter to Alcohol: An Alternative Method for Field Preservation of Arthropod Specimens and DNA Suitable for Mass Collecting Methods. Eur. J. Entomol. 111, 175–179. doi: 10.14411/eje.2014.024
Poore G. C. B., Bruce N. L. (2012). Global Diversity of Marine Isopods (Except Asellota and Crustacean Symbionts). PloS One 7, e43529. doi: 10.1371/journal.pone.0043529
Porter M. L. (2016). Collecting and Processing Mysids, Stygiomysids, and Lophogastrids. J. Crust. Biol. 36, 592–595. doi: 10.1163/1937240X-00002443
Preciado I., Cartes J. E., Punzón A., Frutos I., López-López L., Serrano A. (2017). Food Web Functioning of the Benthopelagic Community in a Deep-Sea Seamount Based on Diet and Stable Isotope Analyses. Deep Sea Res. Pt. II Top. Stud. Oceanogr. 137, 56–68. doi: 10.1016/j.dsr2.2016.07.013
Presnell J. K., Schreibman M. P. (1997). Humason’s Animal Tissue Techniques. 5th ed (Baltimore (Md: Johns Hopkins University press).
Ramirez-Llodra E., Brandt A., Danovaro R., Mol B. D., Escobar E., German C. R., et al. (2010). Deep, Diverse and Definitely Different: Unique Attributes of the World’s Largest Ecosystem. Biogeosciences 7 (9), 2851–2899. doi: 10.5194/bg-7-2851-2010
Raupach M. J., Amann R., Wheeler Q. R., Roos R. (2016). The Application of “–Omics” Technologies for the Classificationand Identification of Animals. Org. Divers. Evol. 16, 1–12. doi: 10.1007/s13127-015-0234-6
Raupach M. J., Wägele J. W. (2006). Distinguishing Cryptic Species in Antarctic Asellota (Crustacea: Isopoda)-A Preliminary Study of Mitochondrial DNA in Acanthaspidia Drygalskii. Antarc. Sci. 18 (2), 191. doi: 10.1017/S0954102006000228
Renner S. S. (2016). A Return to Linnaeus’s Focus on Diagnosis, Not Description: The Use of DNA Characters in the Formal Naming of Species. Syst. Biol. 65 (6), 1085–1095. doi: 10.1093/sysbio/syw032
Riedel A., Sagata K., Suhardjono Y. R., Tänzler R., Balke M. (2013). Integrative Taxonomy on the Fast Track-Towards More Sustainability in Biodiversity Research. Front. Zool. 10 (1), 1–9. doi: 10.1186/1742-9994-10-15
Riehl T., Brenke N., Brix S., Driskell A., Kaiser S., Brandt A. (2014). Field and Laboratory Methods for DNA Studies on Deep-Sea Isopod Crustaceans. Pol. Polar. Res., 35(2) 203–224. doi: 10.2478/popore-2014-0018
Riehl T., De Smet B. (2020). Macrostylis Metallicola Spec. Nov. — An Isopod With Geographically Clustered Genetic Variability From a Polymetallic-Nodule Area in the Clarion-Clipperton Fracture Zone. PeerJ 8, e8621. doi: 10.7717/peerj.8621
Riehl T., Kühn M. A. L. (2020). Uniting What Belongs Together—Reevaluation of the Isopod Species Macrostylis Grandis and M. Ovata Using Ontogenetic, Morphological and Genetic Evidence. Prog. Oceanogr. 181, 102238. doi: 10.1016/j.pocean.2019.102238
Riehl T., Wilson G. D., Hessler R. R. (2012). New Macrostylidae Hansen 1916 (Crustacea: Isopoda) From the Gay Head-Bermuda Transect With Special Consideration of Sexual Dimorphism. Zootaxa 3277 (1), 1–26. doi: 10.11646/zootaxa.3277.1.1
Ríos P., Cristobo J., Altuna A., Frutos I., Manjón-Cabeza E., García Guillén, et al. (2022). Avilés Canyon System: Increasing the Benthic Biodiversity Knowledge. Estuar. Coast. Shelf Sci. 274, 107924. doi: 10.1016/j.ecss.2022.107924
Ritchie H., Jamieson A. J., Piertney S. B. (2017). Genome Size Variation in Deep-Sea Amphipods. R. Soc Open Sci. 4 (9), 170862. doi: 10.1098/rsos.170862
Robin N., Gueriau P., Luque J., Jarvis D., Daley A. C., Vonk R. (2021). The Oldest Peracarid Crustacean Reveals a Late Devonian Freshwater Colonization by Isopod Relatives. Biol. Lett. 17, 20210226. doi: 10.1098/rsbl.2021.0226
Robinson C. V., Porter T. M., Wright M. T. G., Hajibabaei M. (2021). Propylene Glycol-Based Antifreeze Is an Effective Preservative for DNA Metabarcoding of Benthic Arthropods. Freshw. Sci. 40, 77–87. doi: 10.1086/712232
Rodríguez J. G., Garmendia J. M., Muxika I., Gómez-Ballesteros M., Quincoces I., Díez I., et al. (2021). Macrofaunal Variability in the Continental Shelf and Canyons in the Southeastern Bay of Biscay. Reg. Stud. Mar. Sci. 48, 102012. doi: 10.1016/j.rsma.2021.102012
Rosli N., Leduc D., Rowden A. A., Clark M. R., Probert P. K., Berkenbusch K., et al. (2016). Differences in Meiofauna Communities With Sediment Depth Are Greater Than Habitat Effects on the New Zealand Continental Margin: Implications for Vulnerability to Anthropogenic Disturbance. PeerJ 4, e2154. doi: 10.7717/peerj.2154
Rossel S., Martínez Arbizu P. (2018). Automatic Specimen Identification of Harpacticoids (Crustacea : Copepoda) Using Random Forest and MALDI-TOF Mass Spectra, Including a Post Hoc Test for False Positive Discovery. Methods Ecol. Evol. 9, 1421–1434. doi: 10.1111/2041-210X.13000
Rossel S., Martinez Arbizu P. (2019). Revealing Higher Than Expected Diversity of Harpacticoida (Crustacea: Copepoda) in the North Sea Using MALDI-TOF MS and Molecular Barcoding. Sci. Rep. 9 (1), 1–14. doi: 10.1038/s41598-019-45718-7
Sánchez-García A., Peálver E., Bird G. J., Perrichot V., Delclòs X. (2016). Palaeobiology of Tanaidaceans (Crustacea: Peracarida) From Cretaceous Ambers: Extending the Scarce Fossil Record of a Diverse Peracarid Group. Zool. J. Linn. Soc. 178, 492–522. doi: 10.1111/zoj.12427
Sánchez F., Serrano A., Parra S., Cartes J. E. (2008). Habitat Characteristics as Determinant of the Structure and Spatial Distribution of Epibenthic and Demersal Communities of Le Danois Bank (Cantabrian Sea, N Spain). J. Mar. Syst. 72, 64–86. doi: 10.1016/j.jmarsys.2007.04.008
Sanders H. L., Hessler R. R., Garner S. P. (1985). Hirsutia Bathyalis, a New Unusual Deep-Sea Benthic Peracaridan Crustacean From the Tropical Atlantic. J. Crust. Biol. 5 (1), 30–57. doi: 10.2307/1548219
San Vicente C., Cartanyà J. (2017). A New Mysid (Crustacea, Mysida) From the Ladinian Stage (Middle Triassic) of Conca De Barberà (Catalonia, NE Iberian Peninsula). J. Paleontol. 91 (5), 968–980. doi: 10.1017/jpa.2017.24
San Vicente C., Frutos I., Cartes J. E. (2014b). Petalophthalmus Papilloculatus Sp. Nov. (Crustacea: Mysida: Petalophthalmidae), A New Bathyal Suprabenthic Mysid From the Galicia Bank (NE Atlantic Ocean). Zootaxa 3765 (1), 77–91. doi: 10.11646/zootaxa.3765.1.5
San Vicente C., Guerao G., Olensen J. (2014a). “Lophogastrida and Mysida,” in Atlas of Crustacean Larvae. Eds. Martin J. W., Olensen J., Høeg J. T. (Baltimore: Johns Hopkins University Press), 199–205.
Sars G. O. (1900). An account of the Crustacea of Norway. Vol III. Cumacea. Part V and VI Diastylidae. Bergen. pp. 41–68, plates XXXIII–XLVIII.
Saunders T. E. (2020). Taxonomy at a Crossroads: Communicating Value, Building Capability, and Seizing Opportunities for the Future. Megataxa 1 (1), 63–66. doi: 10.11646/megataxa.1.1.13
Schiaparelli S., Schnabel K. E., Richer de Forges B., Chan T.-Y. (2016). “Sorting, Recording, Preservation and Storage of Biological Samples,” in Biological Sampling in the Deep Sea. Eds. Clark M. R., Consalvey M., Rowden A. A. (John Wiley and Sons).
Schmidt C., Escobar Wolf K., Lins L., Martínez Arbizu P., Brandt A. (2018). Meiofauna Abundance and Community Patterns Along a Transatlantic Transect in the Vema Fracture Zone and in the Hadal Zone of the Puerto Rico Trench. Deep Sea Res. Pt. II Top. Stud. Oceanogr. 148, 223–235. doi: 10.1016/j.dsr2.2017.12.021
Schmidt C., Martínez Arbizu P. (2015). Unexpectedly Higher Metazoan Meiofauna Abundances in the Kuril-Kamchatka Trench Compared to the Adjacent Abyssal Plains. Deep Sea Res. Pt. II Top. Stud. Oceanogr. 111, 60–75. doi: 10.1016/j.dsr2.2014.08.019
Schnurr S., Osborn K. J., Malyutina M., Jennings R., Brix S., Driskell A., et al. (2018). Hidden Diversity in Two Species Complexes of Munnopsid Isopods (Crustacea) at the Transition Between the Northernmost North Atlantic and the Nordic Seas. Mar. Biodiv. 48, 813–843. doi: 10.1007/s12526-018-0877-6
Secrétan S., Riou B. (1986). Les Mysidacés (Crustacea, Peracarida) Du Callovien De La Voulte-Sur-Rhône. Ann. Paleontol. 72 (4), 295–323.
Selden P., Wilson G. D. F., Simonetto L., Dalla Vecchia F. (2016). First Fossil Asellote (Isopoda: Asellota), From the Upper Triassic (Norian) of the Carnic Prealps (Friuli, Northeastern Italy). J. Crust. Biol. 36 (1), 68–86. doi: 10.1163/1937240X-00002387
Serrano A., Cartes J. E., Papiol V., Punzón A., García-Alegre A., Arronte J. C., et al. (2017). Epibenthic Communities of Sedimentary Habitats in a NE Atlantic Deep Seamount (Galicia Bank). J. Sea Res. 130, 154–165. doi: 10.1016/j.seares.2017.03.004
Serrano A., Sánchez F., Punzón A., Velasco F., Olaso I. (2011). Deep Sea Megafaunal Assemblages Off the Northern Iberian Slope Related to Environmental Factors. Sci. Mar. 75 (3), 425–437. doi: 10.3989/scimar.2011.75n3425
Sharkey M. J., Janzen D. H., Hallwachs W., Chapman E. G., Smith M. A., Dapkey T., et al. (2021). Minimalist Revision and Description of 403 New Species in 11 Subfamilies of Costa Rican Braconid Parasitoid Wasps, Including Host Records for 219 Species. ZooKeys 1013, 1–665. doi: 10.3897/zookeys.1013.55600
Shaw P. (1989). New Amphipods From Geothermal Vent Sites Off the West Coast of Vancouver Island, British Columbia, With a Reappraisal of the Amphipod Family Sebidae. Can. J. Zool. 67 (8), 1882–1890. doi: 10.1139/z89-269
Shimomura M., Mawatari S. F. (1999). Paramunna Rhipis, a New Species of Asellote Isopod (Paramunnidae) From Japan. Crust. Res. 28, 153–159. doi: 10.18353/crustacea.28.0_153
Shimomura M., Mawatari S. F. (2000). Santia Katoi Sp. Nov., a New Isopod Crustacean From Shirahama, Japan. Publ. Seto Mar. Bioi. Lab. 39 (1), 29–34. doi: 10.5134/176292
Shimomura M., Ohtsuka S. (2005). “Deep-Sea Asellote Isopods (Crustacea Peracarida) of Nansei Islands, Southwestern Japan,” in Deep-Sea Fauna and Pollutans in Nansei Islands, vol. 29 . Eds. Hasegawa K., Shinohara G., Takeda M. (National Science Museum Monographs), 249–259.
Singh R., Mumbarekar V. (2021). Neural Network Model Approach for Automated Benthic Animal Identification (ICT Express).
Sittrop D. J., Serejo C. S., Souza-Filho J. F., Senna A. R. (2015). New Genera and Species of Urothoidae (Amphipoda) From the Brazilian Deep Sea, With the Re-Assignment of Pseudurothoe and Urothopsis to Phoxocephalopsidae. J. Nat. Hist. 49 (9–10), 527–552. doi: 10.1080/00222933.2014.953227
Slabber M. (1769–1778a). Natuurkundige Verlustigingen 15, Tweede Waarneeming van een Steur-garnael met trompetswyze oogen, pp. 136–139, pls. 15, figs 3–4. Haarlem.
Sorbe J. C. (1981). Rôle Du Benthos Dans Le Régime Alimentaire Des Poissons Démersaux Du Secteur Sud Gascogne. Kieler Meeresforsch. Sonderh. 5, 479–489.
Sorbe J. C. (1983). Description D’un Traîneau Destiné a L’échantillonnage Quantitatif Etagé De La Faune Suprabenthique Néritique. Ann. Inst. Océanogr. 59 (2), 117–126.
Starr H. W., Hegna T. A., McMenamin M. A. S. (2016). ). Epilogue to the Tale of the Triassic Amphipod: Rosagammarus McMenamin, Zapata and Hussey 2013 is a Decapod Tail (Luning Formation, Nevada, USA). J. Crust. Biol. 36 (4), 525–529. doi: 10.1163/1937240X-00002444
Stebbing T. R. R. (1888). Report on the Amphipoda Collected by H.M.S. Challenger During the Years 1873–76. Report on the Scientific Results of the Voyage of H.M.S. Challenger During the Years 1873–1876. Zoology 29, xxiv + 1737.
Steedman H. F. (1958). Dimethyl Hydantoin Formaldehyde: A New Water-Soluble Resin for Use as a Mounting Medium. J. Cell Sci. s3–99, 451–452. doi: 10.1242/jcs.s3-99.48.451
Stegner M. E., Stemme T., Iliffe T. M., Richter S., Wirkner C. S. (2015). The Brain in Three Crustaceans From Cavernous Darkness. BMC Neurosci. 16, 19. doi: 10.1186/s12868-015-0138-6
Stemme T., Eickhoff R., Bicker G. (2014). Olfactory Projection Neuron Pathways in Two Species of Marine Isopoda (Peracarida, Malacostraca, Crustacea). Tissue Cell 46, 260–263. doi: 10.1016/j.tice.2014.05.010
Storey M., Poore G. C. B. (2009). New Species of Brucerolis (Crustacea: Isopoda: Serolidae) From Seas Around New Zealand and Australia. Mem. Mus. Vic. 66, 147–173. doi: 10.24199/j.mmv.2009.66.15
Štrus J., Tušek-Žnidarič M., Repnik U., Blejec A., Summers A. (2019). Microscopy of Crustacean Cuticle: Formation of a Flexible Extracellular Matrix in Moulting Sea Slaters Ligia Pallasii. J. Mar. Biol. Ass. 99, 857–865. doi: 10.1017/S0025315418001017
Sweetman A. K., Thurber A. R., Smith C. R., Levin L. A., Mora C., Wei C. L., et al. (2017). Major Impacts of Climate Change on Deep-Sea Benthic Ecosystems. Elem. Sci. Anth. 5(4).
Tanaka K. (2004). A New Species of Gnathia (Isopoda: Cymothoida: Gnathiidae) From Ishigaki Island, the Ryukyus, Southwestern Japan. Crustacean Res. 33, 51–60. doi: 10.18353/crustacea.33.0_51
Tandberg A. H., Tore Rapp H., Schander C., Vader W., Sweetman A. K., Berge J. (2012). Exitomelita Sigynae Gen. Et Sp. Nov.: A New Amphipod From the Arctic Loki Castle Vent Field With Potential Gill Ectosymbionts. Polar. Biol. 35, 705–716. doi: 10.1007/s00300-011-1115-x
Taylor J., Devey C., Le Saout M., Petersen S., Frutos I., Linse K., et al. (2021). The Discovery and Preliminary Geological and Faunal Descriptions of Three New Steinahóll Vent Sites, Reykjanes Ridge, Iceland. Front. Mar. Sci. 8. doi: 10.3389/fmars.2021.520713
Tomikawa K., Mawatari S. F. (2006). A New Species of the Genus Amathillopsis (Crustacea: Amphipoda: Amathillopsidae) From Japan. Species Div. 11 (3), 199–207. doi: 10.12782/specdiv.11.199
Turner L. M., Ricevuto E., Massa Gallucci A., Lorenti M., Gambi M.-C., Calosi P. (2016). Metabolic Responses to High Pco2 Conditions at a CO2 Vent Site in Juveniles of a Marine Isopod Species Assemblage. Mar. Biol. 163, 211. doi: 10.1007/s00227-016-2984-x
Valdecasas A. G., Abad A. (2011). Morphological Confocal Microscopy in Arthropods and the Enhancement of Autofluorescence After Proteinase K Extraction. Microsc. Microanal. 17, 109–113. doi: 10.1017/S1431927610094213
Vonk R., Schram F. (2007). Three New Tanaid Species (Crustacea, Peracarida, Tanaidacea) From the Lower Cretaceous Álava Amber in Northern Spain. J. Paleont. 81 (6), 1502–1509. doi: 10.1666/05-020.1
Vrijenhoek R. C. (2009). Cryptic Species, Phenotypic Plasticity, and Complex Life Histories: Assessing Deep-Sea Faunal Diversity With Molecular Markers. Deep Sea Res. Pt. II Top. Stud. Oceanogr. 56 (19–20), 1713–1723. doi: 10.1016/j.dsr2.2009.05.016
Wägele H., Klussmann-Kolb A., Kuhlmann M., Haszprunar G., Lindberg D., Koch A., et al. (2011). The Taxonomist-an Endangered Race. A Practical Proposal for its Survival. Front. Zool. 8 (1), 1–7. doi: 10.1186/1742-9994-8-25
Wall A., Campo D., Wetzer R. (2014). Genetic Utility of Natural History Museum Specimens: Endangered Fairy Shrimp (Branchiopoda, Anostraca). ZooKeys 457, 1–14. doi: 10.3897/zookeys.457.6822
Washburn T. W., Menot L., Bonifácio P., Pape E., Błażewicz M., Bribiesca-Contreras G., et al. (2021). Patterns of Macrofaunal Biodiversity Across the Clarion-Clipperton Zone: An Area Targeted for Seabed Mining. Front. Mar. Sci. 8. doi: 10.3389/fmars.2021.626571
Wells A., Johanson K. A., Dostine P. (2019). Why are So Many Species Based on a Single Specimen? Zoosymposia 14 (1), 32–38. doi: 10.11646/zoosymposia.14.1.5
Wetzer R. (2015). Collecting and Preserving Marine and Freshwater Isopoda (Crustacea: Peracarida). Biodivers. Data J. 3, e4912. doi: 10.3897/BDJ.3.e4912
Wheeler Q. (2018). Blank Canvas: The Case for Descriptive Taxonomy. Integr. Comp. Biol. 58, 1118–1121. doi: 10.1093/icb/icy067
Will K. W., Mishler B. D., Wheeler Q. D. (2005). The Perils of DNA Barcoding and the Need for Integrative Taxonomy. Syst. Biol. 54, 844–851. doi: 10.1080/10635150500354878
Wilson G. D. F. (2003). A New Genus of Tainisopidae Fam. Nov. (Crustacea: Isopoda) From the Pilbara, Western Australia. Zootaxa 245, 1–20. doi: 10.11646/zootaxa.245.1.1
Wilson G. D. F. (2017). Macrofauna Abundance, Species Diversity and Turnover at Three Sites in the Clipperton-Clarion Fracture Zone. Mar. Biodivers. 47, 323–347. doi: 10.1007/s12526-016-0609-8
Wilson G. D. F., Ahyong S. T. (2015). Lifestyles of the Species-Rich and Fabulous: The Deep-Sea Crustaceans. in The Natural History of the Crustacea: Lifestyles and feeding Biology. Eds.Thiel M. F., Watling L. (Oxforf University Press), 279–298.
Wilson G. D. F., Humphrey C. L. (2020). The Eophreatoicus Nicholls 1926 Species Flock From Kakadu and Arnhem Land, With a Description of a New Genus of Amphisopidae (Crustacea: Isopoda: Phreatoicidea). Zootaxa 4854, 1–303. doi: 10.11646/zootaxa.4854
Wilson K., Thorndyke M., Nilsen F., Rogers A., Martinez P. (2005). Marine Systems: Moving Into the Genomics Era. Mar. Ecol. 26 (1), 3–16. doi: 10.1111/j.1439-0485.2005.00041.x
Wirkner C. S., Richter S. (2004). Improvement of Microanatomical Research by Combining Corrosion Casts With MicroCT and 3D Reconstruction, Exemplified in the Circulatory Organs of the Woodlouse. Microsc. Res. Tech. 64, 250–254. doi: 10.1002/jemt.20076
Wittmann K. J., Ariani A. P., Lagardère J. P. (2014). “Orders Lophogastrida Boas 1883, Stygiomysida Tchindonova 1981, and Mysida Boas 1883 (Also Known Collectively as Mysidacea),” in Treatise on Zoology – Anatomy, Taxonomy, Biology. The Crustacea, vol. vol 4 Part B . Eds. von Vaupel Klein J. C., Charmantier-Daures M., Schram F. R. (Leiden: Koninklijke Brill NV), pp 189–pp 396.
Wittmann K. J., Ariani A. P., Daneliya M. (2016). The Mysidae (Crustacea: Peracarida: Mysida) in Fresh and Oligohaline Waters of the Mediterranean. Taxonomy, Biogeography, and Bioinvasion. Zootaxa 4142, 1–70. doi: 10.11646/zootaxa.4142.1.1
Wolff C. (2009). The Embryonic Development of the Malacostracan Crustacean Porcellio Scaber (Isopoda, Oniscidea). Dev. Genes Evol. 219, 545–564. doi: 10.1007/s00427-010-0316-6
WoRMS Editorial Board (2021) World Register of Marine Species. Available at: https://www.marinespecies.org.
Yeom J., Park N., Jeong R., Lee W. (2021). Integrative Description of Cryptic Tigriopus Species From Korea Using MALDI-TOF MS and DNA Barcoding. Front. Mar. Sci. 8, 495. doi: 10.3389/fmars.2021.648197
Zeidler W. (1990). Pelagic Amphipods, Infraorder Physosomata (Crustacea: Amphipoda. Hyperiidea) From the CSK International Zooplankton Collection (Western North Pacific) With the Description of Four New Species of Scina. Publ. Seto Mar. Biol. 34 (4/6), 167–200.
Zeidler W. (2009). A Review of the Hyperiidean Amphipod Superfamily Lanceoloidea Bowman and Gruner 1973 (Crustacea: Amphipoda: Hyperiidea). Zootaxa 2000, 1–117. doi: 10.11646/zootaxa.2000.1.1
Zhang Z. Q. (2008). Zoological Taxonomy at 250: Showcasing Species Descriptions in the Cyber Era. Zootaxa 1671 (1), 1–2. doi: 10.11646/zootaxa.1671.1.1
Zhang R., Zhou Y., Lu B., Wang C. (2017). A New Species in the Genus Styracaster (Echinodermata: Asteroidea: Porcellanasteridae) From Hadal Depth of the Yap Trench in the Western Pacific. Zootaxa 4338, 153–162. doi: 10.11646/zootaxa.4338.1.8
Keywords: integrative taxonomy, morphology, microscopy, imaging, suprabenthos, Isopoda, Tanaidacea, Amphipoda
Citation: Frutos I, Kaiser S, Pułaski Ł, Studzian M and Błażewicz M (2022) Challenges and Advances in the Taxonomy of Deep-Sea Peracarida: From Traditional to Modern Methods. Front. Mar. Sci. 9:799191. doi: 10.3389/fmars.2022.799191
Received: 21 October 2021; Accepted: 05 May 2022;
Published: 30 June 2022.
Edited by:
Ana Colaço, Marine Research Institute (IMAR), PortugalReviewed by:
Oliver Simon Ashford, OceanMind, United KingdomCopyright © 2022 Frutos, Kaiser, Pułaski, Studzian and Błażewicz. This is an open-access article distributed under the terms of the Creative Commons Attribution License (CC BY). The use, distribution or reproduction in other forums is permitted, provided the original author(s) and the copyright owner(s) are credited and that the original publication in this journal is cited, in accordance with accepted academic practice. No use, distribution or reproduction is permitted which does not comply with these terms.
*Correspondence: Inmaculada Frutos, aW5tYWN1bGFkYS5mcnV0b3NAYmlvbC51bmkubG9kei5wbA==
†Present address: Stefanie Kaiser, INES Integrated Environmental Solutions UG, c/o DZMB, Wilhelmshaven, Germany
Disclaimer: All claims expressed in this article are solely those of the authors and do not necessarily represent those of their affiliated organizations, or those of the publisher, the editors and the reviewers. Any product that may be evaluated in this article or claim that may be made by its manufacturer is not guaranteed or endorsed by the publisher.
Research integrity at Frontiers
Learn more about the work of our research integrity team to safeguard the quality of each article we publish.