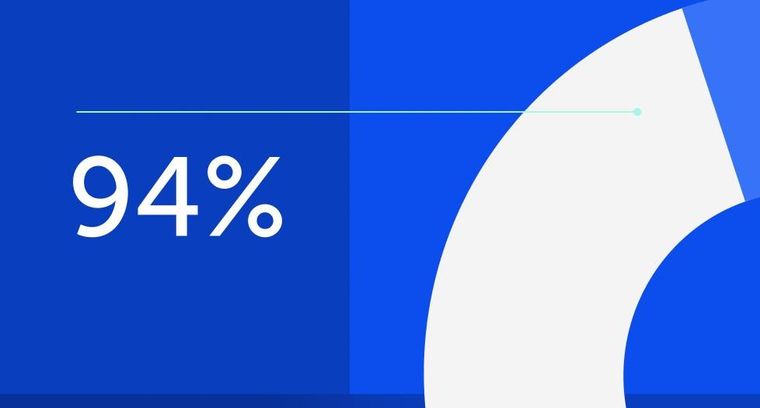
94% of researchers rate our articles as excellent or good
Learn more about the work of our research integrity team to safeguard the quality of each article we publish.
Find out more
ORIGINAL RESEARCH article
Front. Mar. Sci., 17 June 2022
Sec. Marine Megafauna
Volume 9 - 2022 | https://doi.org/10.3389/fmars.2022.779047
This article is part of the Research TopicMovement and Connectivity of Large Pelagic SharksView all 22 articles
Pelagic elasmobranchs are key elements of oceanic ecosystems and must be preserved if marine trophic networks are to be kept in balance. Yet, they face intense fishing pressure that has been threatening their populations worldwide. Ensuring proper conservation management of these taxa depends on a better understanding of the strategies they use to explore the pelagic realm and their contributions to trophic web structuring across the ocean column. This study aimed at examining relationships between vertical habitat use and trophic attributes among six sympatric pelagic elasmobranchs using satellite transmitting tags in the western equatorial South Atlantic Ocean. The vertical movements of 35 elasmobranch individuals were tracked during an overall total of 1911 days. Clear relationships between species’ feeding habits, maximum diving depths, and proportion of time spent either in epipelagic or in surface waters were evidenced by Bayesian generalized linear mixed models and multivariate analysis. Filter-feeders made most use of deep waters from the mesopelagic and bathypelagic and shifted their diving depths in phase with diel vertical migrations of the deep scattering layer, i.e., shallower during the night and deeper during the day. Specialists exhibited distinct diving patterns in epipelagic and mesopelagic waters across the diel period which are potentially indicative of habitat partitioning, whereas generalists were more surface-oriented but also explored deeper waters compared to specialists. The trophic level also seemed to influence elasmobranch maximum diving depths, which tended to become shallower as species’ trophic level increased. These results corroborate previous evidence of widespread vertical habitat partitioning among sympatric pelagic predators and depict a trophic-mediated structuring of the pelagic environment where top-down control may be exerted at different depths by distinct species. Further research is yet required to understand the role of elasmobranch vertical movements in structuring pelagic habitats as well as to guide ecosystem-based fisheries management aimed at reducing species susceptibility to fishing gear and at preserving the structure and functionality of marine trophic networks.
Globally, chondrichthyan fishes face widespread, unsustainable fishing pressure (Davidson et al., 2016; Queiroz et al., 2019) which has resulted in more than one-third of these taxa being currently threatened with extinction (Dulvy et al., 2021). Populations of easily accessible coastal elasmobranchs are known to have already collapsed (Jackson et al., 2001), and modern technology has enabled the exploitation of the oceanic realm by industrial fisheries at alarmingly high rates, so that about half of all oceanic shark species are is now endangered (Pacoureau et al., 2021). Such a reality poses a considerable challenge to researchers and managers because these taxa generally play an important role in balancing and connecting marine ecosystems (Heithaus et al., 2012; Afonso et al., 2017) while being considerably susceptible to overfishing (Dulvy and Forrest, 2010). Hence, serious concerns about the sustainability of elasmobranch fisheries and the ecological consequences of their removal from the marine environment have been raised (Ferretti et al., 2010; Trindade-Santos et al., 2020). Ensuring the health of marine ecosystems may thus depend on the effective conservation of elasmobranch populations. However, essential knowledge about the ecology and behavior of pelagic species is scant due to their remoteness, crypticness, and vagility, ultimately hampering the ability to achieve optimal resource management.
The pelagic oceanic realm comprises the largest ecosystems on the globe (Robison, 2009) and is divided into different depth strata with distinct physicochemical and biological properties. The epipelagic euphotic biome spans from the surface down to 200 m in depth and corresponds to the stratum where sunlight penetration enables primary production by photosynthetic organisms. The mesopelagic dysphotic biome spans between 200 and 1000 m in depth and comprises a major faunal assemblage with one of the greatest biomasses in the biosphere (Irigoien et al., 2014). This assemblage gathers mostly fish and invertebrates within the so-called ‘Deep Scattering Layer’ (DSL) (Costello and Breyer, 2017) and constitutes a regular source of prey for marine megafauna (Hazen, 2010). The bathypelagic biome spans below 1000 m in depth and is characterized by the total absence of sunlight and by a sharp decrease in fish abundance (Sutton et al., 2010). The vertical distribution of oceanic fauna in the pelagic environment is modulated by abiotic factors including temperature, dissolved oxygen, light level, and pressure (Bianchi et al., 2013; Klevjer et al., 2016; Bernal et al., 2017) which vary considerably across the water column (Costello and Breyer, 2017). Notwithstanding, systematic movements across pelagic biomes by several marine taxa are known to occur on a regular basis, particularly between the epipelagic and mesopelagic strata (Sutton, 2013). This is because, at small spatial scales, the vertical gradient of the oceanic realm renders much higher habitat variability than the horizontal one, a feature that DSL organisms and pelagic fauna explore to improve foraging efficiency and feeding success while reducing predation risk. Diel vertical migrations conducted by nektonic and planktonic fauna from the DSL are among the most massive migratory processes known to date, and epipelagic predators are believed to adapt their movements accordingly to feed directly on DSL prey or on lower order predators that feed on DSL prey (Hays, 2003).
Large-bodied elasmobranchs shape the structure of marine ecosystems via trophic-related relationships mediated by direct predation upon lower trophic levels (Hammerschlag, 2019) and intra- or inter-specific competition (Sabando et al., 2020). Competition among predators may lead to fewer feeding opportunities and reduced fitness (Smith et al., 2017; Jorgensen et al., 2019), hence resource partitioning between sympatric elasmobranchs has often evolved (Tillett et al., 2014; Espinoza et al., 2019; Mulas et al., 2019). In the pelagic realm, elasmobranch resource partitioning could be more feasibly achieved on the vertical scale, and there is growing evidence that co-occurring predatory species use different compartments of the depth gradient to forage (Le Croizier et al., 2020b; Besnard et al., 2021; Madigan et al., 2021). An intrinsic relationship between species diving behavior and its trophic attributes might thus be expected among the pelagic elasmobranch community. In accordance, previous simulation-based research highlighted that the vertical distribution of prey could have a greater influence on the diving behavior of pelagic predators than the abiotic gradient by itself (Dagorn et al., 2000). Pelagic habitat partitioning has been identified among other high-level predators such as tuna and seabirds, and prey abundance showed to improve distribution models for these species (Receveur et al., 2021). It is known that anthropogenic disturbances to marine ecosystems such as the ones produced by fisheries tend to propagate across the complexity of food webs (Ferretti et al., 2010), eventually developing into unforeseen ecological damage. Therefore, understanding the vertical structure of pelagic trophic networks and the venues of energy flow within and between oceanic habitats is utterly required to guide fisheries management toward a sustainable use of marine resources.
This study seeks to explore potential relationships between the diving behavior and intrinsic trophic attributes in sympatric, large-bodied pelagic elasmobranchs using biologging data collected in a poorly known region, i.e., the South Atlantic Ocean. With this approach, we aim at finding preliminary evidence of a trophic-mediated, vertical compartmentalization of the pelagic realm shaped by the behaviors of pelagic predators and their prey, as well as at depicting possible pathways for energy transference between different pelagic biomes.
Elasmobranch tagging was conducted with the approval of the Ethics Committee on Research with Animals of the Universidade Federal Rural de Pernambuco (licenses no. #23082.009679/2009, #23082.025519/2014, and #23082.025800/2015).
This study was conducted in the western equatorial Atlantic Ocean off Northeast Brazil (Figure 1). We focused our elasmobranch tagging efforts on a region spanning between latitudes 1°N and 8°S to preclude potentially confounding effects derived from regional-specific environmental factors. Tagging locations were off the Saint Peter and Saint Paul Archipelago (0.9°N, 29.3°W), off the Fernando de Noronha Archipelago (3.8°S, 32.4°W), and off Recife (8.1°S, 34.5°W).
Figure 1 Map of the study area in the western equatorial and tropical South Atlantic Ocean depicting tag deployment locations (triangles) and pop-up locations (inverted triangles). Colors represent different elasmobranch species.
Elasmobranchs were tagged with pop-up satellite archival tags (MK-10 and miniPAT models; Wildlife Computers, USA), hereafter referred to as PSATs. These tags record a time-series of depth, seawater temperature, and luminosity readings which depict both vertical and horizontal movements performed by free-ranging tagged individuals during a user-programmable deployment span. Then, the tags pop up to the ocean surface and inform their position with high (< 1.5 km) accuracy while transmitting summarized depth (± 4 m) and temperature (± 0.05°C) data through the satellites with a 1- to 24-hour temporal resolution. These summaries include the depth range (i.e., minimum and maximum depths) of PSAT movements along with a vertical profile of seawater temperature (PDT), and histograms of the relative time spent at different depth strata (TAD) within a temporal unit. Luminosity data are also relayed to reconstruct horizontal movements based on the timing of crepuscular events. PSATs can withstand pressure levels as high as 200 atm, thus they are able to track bathypelagic dives up to ~2000 m in depth.
The tagged species included three carcharhinids (tiger shark Galeocerdo cuvier, silky shark Carcharhinus falciformis, and blue shark Prionace glauca), one sphyrnid (scalloped hammerhead shark Sphyrna lewini), one rhincodontid (whale shark Rhincodon typus), and one mobulid (sicklefin devil ray Mobula tarapacana). In general, sharks were caught with longline fishing gear and either brought onboard or restrained underwater alongside the boat. Sharks were identified, sexed, and measured for total length (TL) to the nearest centimeter. A PSAT was then fitted to the first dorsal fin or, alternatively, to the dorsal musculature beneath the first dorsal fin so that it would be towed near the shark’s body. Mobulids and whale sharks were tagged while swimming near the surface by an experienced diver, who made use of a 150 cm pole to fit PSATs into the posterior region of the dorsal musculature. Whale shark total length and devil ray disk width (DW) were visually estimated during the tagging procedure. More detailed information about the tagging procedure for the different species can be found in Afonso (2013); Carvalho et al. (2015); Macena (2016); Afonso et al. (2017); Bezerra et al. (2019), and Mendonça et al. (2018).
Satellite-relayed data were decoded using the proprietary manufacturer’s DAP® software, and the most likely movements between tag deployment and pop-up locations were estimated using GPE3® software in the Wildlife Computers Data Portal. Elasmobranch maximum diving depths (maxDepth, in meters) were filtered from PDT data and ascribed with a diel stage (i.e., day or night), which was defined by applying the sunriset function in the maptools R package (Bivand and Lewin-Koh, 2021) to the date, time, and most likely location of each maxDepth. Because PSAT data were pooled from different individual studies and presented heterogeneous temporal resolution (i.e., data were summarized in 3-, 4-, 6-, or 12-hour intervals), the maximum depth reading during a single diel stage was used. Moreover, the proportion of time spent by elasmobranchs in epipelagic waters above the 200-m isobath (T200) and in surface waters above the 10-m isobath (T10) were retrieved from TAD data. These depth strata were selected in order to measure the combined utilization of meso- and bathypelagic waters by these epipelagic species, and also to examine their association with the uppermost layer of the sea column where potential air-breathing prey could be the most available. A diel stage was also ascribed to T200 and T10 data as previously described.
Since individual trophic levels were not assessed in this study, shark trophic levels at the species level were obtained from Cortés (1999), whereas the trophic level of the sicklefin devil ray was obtained from FishBase (Froese and Pauly, 2021). Also, species were grouped according to their feeding habits into three categories, namely filter-feeders, generalists, and specialists. Categorizing elasmobranch species as dietary generalists or specialists may be rudimental since these categories often fail to capture the continuum nature of trophic specialization (Compagno, 1990). In fact, elasmobranchs tend to be more or less specialized, but they rarely match with the whole definition of specialist or generalist (Munroe et al., 2014). Insufficient data on elasmobranch diet and prey composition prevent comprehensive assessments of dietary specialization, though. As such, the relative categorization of elasmobranch trophic habits could still provide helpful information about ecosystem structure and species vulnerabilities. Here, we opted for conducting a relative categorization of generalist and specialist species based on the cumulative proportions of prey categories in sharks’ diets, as reported by Cortés (1999). For each species, we assessed the number of prey categories (in decreasing order of relevance) required to accumulate at least 90% of the diet composition. Species with fewer or more prey categories at the 90% threshold were categorized as specialists or generalists, respectively. Accordingly, P. glauca, C. falciformis, and S. lewini were all classified as specialists because they required only two to three prey categories to attain the 90% threshold (93%-100% of their diets were composed of teleosts, cephalopods, and crustaceans). On the other hand, G. cuvier was classified as a generalist because it required six prey categories to comprise 94% of its diet composition (Table S1). Filter-feeders were identified by their widely described feeding strategy.
All analyses were conducted in R version 4.1.1 (R Core Team, 2021). Because the available data were not balanced across feeding habits, a random filter was applied to the overrepresented generalist dataset using the sample R function with no replacements so that the number of both maxDepth and TAD samples would amount to 500 in generalists. Vertical profiles of seawater temperature were generated with PDT data to inspect for potential thermal variability between species. Distribution density histograms of maxDepth per feeding habit category and per diel stage were generated to visualize patterns in diving behavior across these factors, with maxDepth being also discriminated across species. In turn, the relationship between species trophic level and maxDepth was visually explored with boxplots, with significant differences between trophic levels being tested with Kruskal-Wallis variance analysis followed by a post-hoc multiple comparison Dunn test, which was run with the kruskal.test and the dunnTest functions in the FSA R package (Ogle et al., 2021). Even though the trophic level is an intrinsically continuous measure, the properties of our dataset precluded an efficient use of parametric regression analysis. Therefore, we opted for interpreting trophic level as an ordered categorical variable to identify any potentially consistent trend in maxDepth variation across the trophic level gradient. Additionally, a multivariate cluster analysis was conducted to assess similarities between species in relation to the frequency distribution of maxDepth and to determine the most adequate species grouping with no prior assumptions. The NbClust R package (Charrad et al., 2014) was used to ascertain the most adequate number of clusters. The function NbClust calculates 23 different types of indexes and proposes the best clustering scheme based on the most common number of clusters given by the indexes (i.e., majority rule). The data was scaled prior to cluster analysis, and the dissimilarity matrix was computed using Euclidean distance with the ‘complete’ method of cluster analysis. Then, a dendrogram was plotted to examine hierarchical relationships among species and compare the resulting clusters with species trophic attributes. On the other hand, distribution density histograms of T200 and T10 per feeding habit and per diel phase were generated to examine trends in the utilization of epipelagic (≤ 200 m in depth) and surface (≤ 10 m in depth) waters by species groups. TAD data for blue sharks were unavailable, hence this species was not included in the analysis.
Bayesian generalized linear mixed models were used to identify statistically significant covariates that could affect the variability in elasmobranch diving behavior and vertical distribution. Three different models were fitted using maxDepth (continuous variable ≥ 0), T200, and T10 (both proportions ranging between 0 and 1) as response variables. Preliminary inspection of the distribution of maxDepth indicated that these data were highly left-skewed. Hence, we applied a transformation of Tukey’s Ladder of Powers to reduce the skewness and produce a more normal distribution of the response variable (lambda = 0.075; W = 0.9846; p < 0.001) using the rcompanion R package (Mangiafico, 2015). Therefore, after this transformation, we assumed that the response variable maxDepth was a random variable with a normal distribution. T200 and T10 proportion data were modeled via the beta probability distribution, where the mean of proportions enters the model through the logit link function. The beta distribution is defined for any real number between 0 and 1 and therefore it is appropriate for proportional data with asymmetric shapes (Gupta and Nadarajah, 2004). This approach has been successfully applied to regression modeling and brings several advantages over other methods addressing binomial data (Ferrari and Cribari-Neto, 2004; Paradinas et al., 2018). Candidate predictor variables included feeding habit (i.e., generalist, specialist, and filter-feeding), diel phase (i.e., day and night), and the interaction among these factors. Including the interaction of feeding habit with diel phase was deemed necessary because descriptive plots suggested different species might respond differently to the diel cycle, but the independent effect of the diel phase was not explored. Further, due to the nature of the data which comprised multiple observations over time for the same fish, we included each individual elasmobranch as a random factor, thus implying the data are independent, identically, and normally distributed (i.i.d.), with mean 0 and variance σ2.
Bayesian inference and parameter estimates in the form of marginal posterior distributions were obtained through the Integrated Nested Laplace Approximation (INLA) approach, which is currently implemented in the R environment by the R-INLA package (http://www.r-inla.org). As recommended by Held et al. (2010), we used default priors for all fixed-effect parameters, which were defined by a vague zero-mean Gaussian prior distribution with a variance of 100 (except for the variance of the intercept which has the default value of zero). The selection of predictors and the decision on their entry or exclusion was based on the forward stepwise approach considering the Deviance Information Criterion (DIC). The best model was chosen based on the lowest DIC score and on the visual inspection of the residual distributions following the methodology of standard graphical checks proposed by Ortiz and Arocha (2004). In order to prioritize parsimony over model complexity, simpler models with less predictors were chosen when rounded DIC scores were tied.
Between June 2009 and June 2014, a total of 35 elasmobranchs belonging to six species were tagged with PSAT tags in the western equatorial South Atlantic Ocean between latitudes 0.92°N and 8.36°S (Figure 1). These comprised 16 tiger sharks, two blue sharks, three silky sharks, six scalloped hammerhead sharks, three whale sharks, and five sicklefin devil rays (Table S2). The size of tagged individuals ranged from 128 to 310 cm TL with a mean ± standard deviation of 195 (± 54) in tiger sharks, 130 to 180 cm TL (mean = 156 ± 25) in silky sharks, 205 to 260 cm TL (mean = 224 ± 25) in scalloped hammerhead sharks, 600 to 900 cm TL (mean = 800 ± 173) in whale sharks, and 245 to 270 cm DW (mean = 255 ± 13; N = 3) in sicklefin devil rays (Table S2). No measurements for blue sharks were available. Female-to-male sex ratios equaled 2.2 in tiger sharks, 1.0 in blue sharks and whale sharks, and 4.0 in scalloped hammerhead sharks and sicklefin devil rays, with no female silky sharks being tagged. Species classification per feeding habit (and trophic level) resulted in a filter-feeding group encompassing whale sharks (3.6) and sicklefin devil rays (3.8); a specialist group encompassing silky sharks (4.2), blue sharks (4.1), and scalloped hammerheads (4.1); and a generalist group represented by tiger sharks (4.1).
PSAT deployment duration ranged between 3 and 122 days (mean = 54.6 ± 43.8), rendering a total of 1911 tracking days (Table S2). In general, the distance between the tagging and pop-up locations was relatively small and all movements were circumscribed to the western equatorial and tropical South Atlantic (Figure 1). Overall, a total of 1783 PDT samples and 2497 TAD samples were collected, with the number of samples per species ranging from 85 to 1052 (mean = 297 ± 373) for PDT data and from 191 to 1361 (mean = 499 ± 485) for TAD data. After applying a random filter to the overrepresented tiger shark, the number of samples across functional groups was reasonably balanced for both PDT (Nfilter-feeder = 311, Ngeneralist = 489, Nspecialist = 420) and TAD (Nfilter-feeder = 544, Ngeneralist = 500, Nspecialist = 592) data.
The maximum diving depth of species grouped by feeding habit (i.e., filter-feeding, generalist, and specialist) exhibited striking differences. Filter-feeders moved mostly within the upper 500 m of the water column and exhibited a bimodal maximum diving depth frequency distribution peaking evenly at about the 100- and 300-m isobaths during the night, contrasting with a unimodal distribution peaking at the ~300-m isobath during the day (Figure 2). Notwithstanding, filter-feeders also performed some deeper dives across the mesopelagic and bathypelagic zones up to ~2000 m in depth. Generalists exhibited comparatively shallower diving depths, with most dives occurring around the 50-m isobath and becoming increasingly less frequent with increasing depth (up to ~1100 m) regardless of the diel phase (Figure 2). Specialists showed a bimodal diving depth frequency distribution during the day, with the main peak at the ~100 m isobath and a secondary peak at the ~300 m isobath. Yet, a single peak at ~150 m was noticed at night, albeit deeper mesopelagic dives > 500 m in depth were more frequent during this diel stage. Also, specialists performed dives up to ~750 m in depth, which is shallower than the > 1000 m deep dives performed by generalists and filter-feeders.
Figure 2 Density plot of the smoothed distribution of pelagic elasmobranch maximum diving depths (maxDepth, in meters) grouped by species feeding habit for each diel phase. The upper and lower horizontal dashed lines depict the 200- and 1000-m isobaths, respectively.
Additionally, specialist species showed to be highly segregated by depth in terms of their diving behavior. C. falciformis consistently performed diurnal shallow dives within the epipelagic at a strikingly preferred depth of about 80 m, but during the night it dove more frequently into deeper waters around the 150-m isobath (Figure S1). Likewise, S. lewini showed a diurnal preference for epipelagic diving centered around the 100-m isobath, albeit performing frequent dives into mesopelagic waters up to ~400 m in depth (Figure S1). However, the frequency of maximum diving depths of this species changed to a relatively uniform distribution across the water column down to the 750-m isobath during nocturnal periods. In turn, P. glauca dove to maximum depths of ~600 m but it exhibited a preference for waters from the upper mesopelagic centered around the 300-m isobath during the daytime, and around the 250-m isobath during the night (Figure S1). In both diel stages, a secondary peak in diving depth frequency in epipelagic waters between the 50- and 100-m isobaths was observed.
In comparison, filter-feeding species showed more coinciding diving depth distributions, although R. typus performed deeper bathypelagic dives than M. tarapacana (Figure S1). The former species showed a unimodal, mesopelagic-oriented diving pattern centered around the 300-m isobath during the day, but during nocturnal periods it tended to perform more shallow dives into the lower epipelagic (~130 m in depth). In turn, M. tarapacana showed a bimodal distribution in maximum diving depth frequency which resembled the distribution of P. glauca, with a deeper peak around the 350-m isobath most prominent during the daytime and a shallower peak around the 100-m isobath most prominent during the night (Figure S1). The seawater temperature experienced by tagged elasmobranchs ranged between 4 and 31°C, with species’ vertical movements being associated with similar thermal profiles of the water column (Figure S2).
Elasmobranch maximum diving depths also seemed to correlate negatively with species trophic level, as exploratory boxplots indicated medians and interquartile ranges of diving depth distributions to consistently decrease with increasing trophic level (Figure 3). Average maximum diving depths decreased monotonously from 448 m in the lowest trophic level (3.5), to 93 m in the highest trophic level (4.2). A Kruskal-Wallis rank sum test showed that these differences in diving depth across trophic levels were statistically significant (χ2 = 112.37, d.f. = 3, p < 0.001), and a post-hoc multiple comparison Dunn test rendered significant differences to all pairwise combinations of trophic level (p ≤ 0.006; Table S3). Due to collinearity issues with feeding habitat, we refrained from including trophic levels in the INLA modeling.
Figure 3 Boxplots of the distribution of maximum diving depths in relation to trophic level in pelagic elasmobranch species. The bold horizontal line depicts the median, the solid horizontal lines in the box depict the interquartile range, the vertical solid lines represent the distribution range, and the solid circles depict potential outliers. The larger, solid, blue circles depict the mean maximum diving depths for each trophic level. Note the decreasing trend in maximum diving depths with increasing trophic levels.
The stepwise INLA model selection procedure for the response variable maxDepth selected feeding habit and its interaction with the diel cycle as the most relevant predictors of elasmobranch diving depth (Table S4). Overall, generalists and specialists performed significantly shallower dives than filter-feeders (Figure 4; Table S5). Further, filter-feeders tended to dive shallower during the night, contrasting with deeper diving behavior during the night by specialists (Figure 4; Table S5). Maximum diving depths in generalists proved to be unaffected by the diel stage. The diagnosis of model performance revealed that it conformed reasonably with its assumptions (Figure S3).
Figure 4 Marginal posterior distributions of model parameters provided by a generalized linear model with integrated nested Laplace approximation developed to assess the effects of feeding habit (filter-feeding, specialist, and generalist) and the interaction of feeding habit with the diel cycle (day vs. night) on pelagic elasmobranch maximum diving depths (maxDepth). The vertical dashed line depicts null effects. Note that 2.5% of the distribution in each tail was discarded to provide a confidence level of 95%.
Multivariate analysis of maxDepth was conducted with three clusters because the output of the NbClust R package indicated this number of clusters to have the greatest statistical support (Figure S4). Tiger sharks formed a single-species group with the highest dissimilarity from the remainder of the species, while whale sharks formed another single-species group (Figure 5). The remainder of the species formed a third group, but sicklefin devil rays were hierarchically closer to whale sharks. The blue, silky, and scalloped hammerhead sharks were all grouped together, although the latter exhibited higher dissimilarity among these three species (Figure 5). The observed clusters and hierarchical arrangement strongly matched species grouping by feeding habits, although filter-feeders turned out to be separated into different clusters.
Figure 5 Hierarchical cluster dendrogram depicting three groups of pelagic elasmobranch species (dashed rectangles) ordered by their similarities in relation to maximum diving depths. The shaded, colored rectangles represent groups of species which share the same feeding habit (i.e., filter-feeding, specialist, and generalist).
An epipelagic-oriented distribution in the taxa analyzed was clearly evidenced by TAD data, with all functional groups exhibiting a tendency to spend most of their time above the 200-m isobath (Figure 6). However, differences in deep (> 200 m) water use were observed across feeding habit categories and across the diel phase. Generalists distinguished from the remaining categories by exhibiting a striking preference for epipelagic waters and spending a nearly negligible proportion of time in mesopelagic or deeper waters during both day and night (Figure 6). Specialists tended to make short-lived (< 25% of a time-unit) incursions below the epipelagic, but occasionally they spent the whole time-unit in mesopelagic waters (Figure 6). In comparison, filter-feeders showed a higher use of deep (> 200 m) waters but they rarely moved exclusively at such depths during a time-unit. A propensity to spend more time at depths below 200 m during the daytime was observed in this group (Figure 6). However, a beta-regression INLA modeling of the T200 response variable informed that a single-predictor model based on feeding habits would provide the best fit for the data (Table S4). This implies that the diel cycle did not significantly interact with the time spent by these species below the epipelagic zone. As for feeding habits, both specialists and generalists spent significantly more time in epipelagic waters than filter-feeders, with generalists exhibiting the most striking difference (Table 1).
Figure 6 Density plot of the smooth distribution of the proportion of time spent in epipelagic waters (≤ 200 m in depth; T200) by pelagic elasmobranchs grouped by their feeding habits (filter-feeding, generalist, specialist) and across the diel cycle (night and day).
Table 1 Means and credibility intervals of 95% (Inferior and Superior) for marginal posterior distributions estimated by a beta-regression INLA model to assess the effect of feeding habit (FeedHab; as generalist, specialist, and filter-feeding) on the proportion of time spent in epipelagic (≤ 200 m in depth) waters by pelagic elasmobranchs.
Regarding surface behavior, differences in the proportion of time spent in the uppermost 10-m layer of the water column were best explained by the effects of feeding habits and the interaction of feeding habits with the diel cycle (Table S4). Specialists exhibited the lowest association to surface waters among all species groups, but they seemed to respond to the diel cycle by increasing the proportion of time spent above the 10-m isobath during the night (Figure 7). Generalists showed a more extensive reliance on surface waters which was most striking also during the night, while filter-feeders exhibited the opposite trend by tending to spend more time in surface waters during the day (Figure 7). The INLA modeling of the T10 response variable revealed that specialists spent significantly less time in surface waters than filter-feeders, but generalists were statistically similar to filter-feeders (Table 2). Furthermore, sea surface (≤ 10 m in depth) use was significantly higher during the night for both specialists and generalists, whereas no significant diel differences were detected in filter-feeders.
Figure 7 Density plot of the smooth distribution of the proportion of time spent in surface waters (≤ 10 m in depth; T10) by pelagic elasmobranchs grouped by their feeding habits (filter-feeding, generalist, specialist) and across the diel cycle (night and day).
Table 2 Means and credibility intervals of 95% (Inferior and Superior) for marginal posterior distributions estimated by a beta-regression INLA model to assess the effect of feeding habit (FeedHab; as generalist, specialist, and filter-feeding) and the interaction of FeedHab with the diel period (DielPer) (i.e., day and night) on the proportion of time spent in surface (≤ 10 m in depth) waters by pelagic elasmobranchs.
This study explores a novel approach to addressing marine habitat structuring by different functional groups within the pelagic elasmobranch assemblage. The dataset used in this study was restricted to sympatric elasmobranchs tagged in the same equatorial region to avoid introducing any potentially-confounding geographical effects into the analysis. This resulted in a small number of species being represented in the dataset, raising the possibility that the observed trends may not generalize to the whole pelagic elasmobranch community. Nonetheless, the results herein reported are promising in that they denote a most relevant relationship between species vertical movements and their respective trophic attributes, besides being built upon a sample size that is aligned with recommendations for an exploratory approach such as ours (Sequeira et al., 2019). We thus encourage future research efforts aimed at examining the occurrence of trophic-mediated structuring of pelagic habitats by elasmobranchs with more diversified datasets and in other regions of the globe. The role of deep-water movements in epipelagic fish is likely related to relevant bioecological functions including foraging, thermoregulation, predator avoidance, parasite eviction, and navigation (Carey and Scharold, 1990; Braun et al., 2022). Physiological traits are traditionally regarded as the main modulators of vertical distribution in marine fishes because different species have distinct capacities to cope with depth-related environmental gradients (Horodysky et al., 2016). However, previous research suggests that the preyscape in the pelagic realm may be equally relevant in determining the dynamics in predator vertical movements (Dagorn et al., 2000; Howey et al., 2016). Even though it is widely accepted that foraging plays a central role in shaping deep-diving behavior by pelagic predators, empirical evidence of such a linkage is generally lacking (Braun et al., 2022). On that account, the multivariate approach undertaken in this study suggests that trophic behavior should be closely related to diving behavior in pelagic elasmobranchs since species ended up being clustered according to their feeding habits. Such an outcome sustains the suitability of the feeding habit classification herein used and corroborates the hypothesis that trophic traits modulate depth use in epipelagic elasmobranchs.
Marine predators play a significant role in structuring and connecting pelagic habitats through complex trophic interactions, and their continued removal by fisheries produces cascading alterations to marine food webs, inclusively in the open ocean (Pauly et al., 1998; Scheffer et al., 2005; Polovina and Woodworth-Jefcoats, 2013). Increasing the knowledge about the trophic structure of pelagic environments is essential to understand the extent of ecological damage produced by fisheries and derive ecosystem-focused management strategies. This study depicts trophic-related trends in diving behavior and depth use within the pelagic elasmobranch assemblage which may contribute to clarifying some of the processes regulating the vertical compartmentalization and connectivity of the pelagic environment by sympatric predators. Vertical movement patterns in large epipelagic predators have been distinguished between single dives, oscillatory swimming, and diel vertical movements, with the latter being the most common pattern detected over a 24-hour period (Andrzejaczek et al., 2019). The temporal resolution provided by our sampling process (i.e., hours) was therefore adequate to capture diel shifts in vertical habitat use, which should more directly depict interspecific differences in trophic behavior compared to other diving patterns of finer (i.e., < 1 hour) resolution.
The INLA modeling showed that depth use was significantly influenced by species’ feeding habits, and this relationship was sustained by complementary multivariate analysis. Since functional groups experienced similar rates of temperature decrease while diving, the observed differences should not derive from variability in the thermal properties of the ocean column at different tagging sites. Filter-feeding whale sharks and sicklefin devil rays made most use of deep waters from the mesopelagic and bathypelagic regarding both maximum diving depths and amount of time spent below the 200-m isobath. Both these species are known to be deep divers and evidence suggesting they feed extensively on demersal and deep-water zooplankton has been reported (Couturier et al., 2013; Rohner et al., 2013; Thorrold et al., 2014; Tyminski et al., 2015), although they are often observed while feeding at or near the sea surface (Motta et al., 2010; Mendonça et al., 2018). In the Atlantic Ocean, a relatively high biomass of meso- and macroplanktonic organisms including copepods, chaetognaths, and decapods have been detected across mesopelagic and bathypelagic waters around equatorial latitudes (Vereschchaka et al., 2017), inclusively in Brazilian waters (Cavalcanti & Larrazábal, 2004). Deep-water incursions by epipelagic filter-feeders may thus relate to a trophic strategy relying on feeding grounds located at the epipelagic zone and at greater depths, e.g., in the DSL (Braun et al., 2022). Such a hypothesis is sustained by the diel pattern of depth use observed in this group. Even though the T200 model did not incorporate the diel cycle as a predictor, likely because all species exhibited a strong affinity for epipelagic waters during the whole diel cycle, significantly shallower diving depths and a greater proportion of time spent in epipelagic waters by filter-feeders during nocturnal periods match the diel vertical migration pattern exhibited by many organisms from the DSL, which ascent during the night and descent during the day (Robinson et al., 2010). Further, since the lower mesopelagic fauna may not undertake significant diel vertical movements (Sutton et al., 2013; Olivar et al., 2017), a substantial source of deep-water prey would still be available during the night, which could explain the maintenance of nocturnal deep-diving behaviors by filter-feeders. During the daytime, filter-feeders tended to dive deeper and spend more time in the mesopelagic, which could relate to significantly greater diurnal utilization of surface (≤ 10 m in depth) waters, e.g., for thermoregulation purposes.
Specialists also showed a response to the diel cycle regarding the utilization of mesopelagic waters. Interspecific variability in vertical habitat use among specialist species was evidenced in both diel stages, with silky sharks assuming a more epipelagic behavior while blue and hammerhead sharks made differential use of mesopelagic waters across the diel cycle. The two latter species are known to make extensive use of deep waters (Bezerra et al., 2019; Vedor et al., 2021) and to feed on mesopelagic and vertical migrant fish and cephalopods from the DSL in the tropical South Atlantic Ocean and elsewhere (Vaske Júnior et al., 2009a; Besnard et al., 2021), but they seem to explore the depth gradient in different ways. Deep-water fishes, particularly gonostomatids and myctophids, are frequently found around this region, with the Cyclothone genus being the most abundant of such taxa off equatorial Brazil (Olivar et al., 2017). These species could potentially provide a reliable prey source to pelagic piscivorous sharks. In turn, silky sharks are generally associated with the surface mixed layer (Curnick et al., 2020; Madigan et al., 2021) and they exhibit highly selective diving depths well above the mesopelagic zone, which likely reflects predatory behavior on epipelagic prey including fish, cephalopods, and crustaceans (Filmalter et al., 2017). Altogether, the three species categorized as specialists showed to follow distinct strategies of depth use which may be indicative of habitat partitioning. The generalist tiger shark did not change its diving behavior across the diel cycle, but it tended to make broader use of the water column than specialist species by exhibiting more surface-oriented behavior while exploring greater depths from the lower mesopelagic and upper bathypelagic zones. Resource partitioning across spatial and temporal gradients has been identified among sympatric coastal sharks (Papastamatiou et al., 2006; Lear et al., 2021) and within pelagic elasmobranch assemblages, where it should be most associated with the vertical dimension of the oceanic realm as different species tend to forage at different depths (Besnard et al., 2021). In fact, the vertical distribution of pelagic oceanic fauna assessed with echosounders revealed a high density of organisms in the epipelagic zone above the 200-m isobath and in the mesopelagic zone, where a primary DSL extending vertically over > 200 m and centered at a mean depth of 525 m precedes a secondary, narrower DSL centered around the 825-m isobath (Proud et al., 2017). Such a prey landscape endows sympatric pelagic elasmobranchs with diverse feeding opportunities across a range of depths, which could favor the emergence of vertical habitat partitioning as a strategy to avoid interspecific competition resulting from a reportedly high degree of trophic overlap among these species (Li et al., 2016; Bornatowski et al., 2017). As for the generalist tiger shark, its diet comprises a multitude of taxa including air-breathing prey (Lowe et al., 1996; Dicken et al., 2017), which could explain the comparatively high association with the top 10 m of the water column. However, it should be acknowledged that the horizontal component of tiger shark movements may have partially amplified this result because this species is also able to explore shallow, coastal habitats.
Regarding the effect of the trophic level, a negative influence on elasmobranch diving depths seems to be present at least to some extent. Although such an inverse relationship was partially shaped by the effect of deep-diving filter-feeders associated with low trophic levels, it still prevailed for the remainder of the species in this study. Hence, generalist and specialist predation upon high-order epipelagic mesopredators should also be contributing to the observed pattern. Tiger sharks are apex predators known to feed on a wide variety of high-level predators including cetaceans, elasmobranchs, and seabirds (Dicken et al., 2017), but since their typical diet also comprises low-order consumers (e.g., chelonians, crustaceans; Lowe et al., 1996) the resulting trophic level turns out to be similar to other more specialist sharks which tend to feed mostly on mesopredator teleosts and cephalopods such as the blue (Kubodera et al., 2007) and scalloped hammerhead (Vaske Júnior et al., 2009b) sharks. If the trophic level ascribed to tiger sharks were to reflect their position as apex predators within the elasmobranch assemblage herein considered, a stronger relationship between trophic level and diving depths might have been found. Albeit a parametric approach was attempted to assess the effect of trophic level on diving depths, it proved ineffective mainly due to a low representativity and coarse resolution of the trophic level variable. Indeed, conspecifics may exhibit a range of different trophic levels which could not be determined because individual trophic levels were not empirically measured, thus all conspecifics were necessarily ascribed with a unique trophic level. Additional variability in a species’ trophic level across spatial and temporal scales might be expected since it depends on the prey consumed by individuals, which could change geographically and seasonally. In this study, we opted to use the most cited literature reporting diet-based trophic levels for shark species (i.e., Cortés, 1999), but other authors report different trophic level values and even different feeding habits for the same species. For instance, the silky shark has been ascribed with a generalist feeding habit and a trophic level of 4.4 (Páez-Rosas et al., 2018), although these estimates were built upon stable isotopes rather than stomach content analysis. Further research controlling for potential bias in trophic level assessments and including more pelagic elasmobranch species is required to clarify whether vertical habitat use could be predicted based on species’ trophic level. Notwithstanding, a linkage between diving behavior and trophic level may be present in pelagic elasmobranchs and could reflect the vertical distribution of species’ preferred prey, assuming that prey trophic levels tend to decrease with increasing depth and that the foraging strategies of these predators rely on a differential consumption of prey, as observed by Páez-Rosas et al. (2018).
Marine habitat partitioning has been reported for several taxonomic groups including batoids (Humphries et al., 2016), teleosts (Fairclough et al., 2008; Young et al., 2010), mammals, and seabirds (Pinela et al., 2010; Receveur et al., 2021), and may as well be a common feature within pelagic elasmobranch assemblages. Different from coastal elasmobranchs, for which a multiplicity of distinct food webs is available in the much-diversified neritic environment, oceanic elasmobranchs tend to rely mostly on latitudinally-restricted phytoplankton-based food webs (Bird et al., 2018). This could translate into a high interspecific competition in the comparatively homogeneous pelagic environment, which would potentially promote habitat partitioning by sympatric predators. Because the variability in preyscape is much higher across the vertical scale of the oceanic realm than along the horizontal plane, a resource partitioning following the depth gradient might be expectable. In accordance, previous research revealed differential mercury uptake among sympatric epipelagic shark species likely indicative of distinct foraging depths (Le Bourg et al., 2019; Besnard et al., 2021), since mercury content in oceanic predators tends to increase with an increased depth distribution of pelagic prey (Blum et al., 2013; Choy et al., 2015; Madigan et al., 2018). Mounting evidence of interspecific variation in vertical habitat use among sympatric pelagic top predators (e.g., Musyl et al., 2011; Madigan et al., 2021) further corroborates the hypothesis of pelagic resource partitioning and highlights the necessity of considering species-specific feeding depths when developing trophodynamic models of the pelagic ecosystem. Several other pelagic elasmobranchs such as the great white, Carcharodon carcharias; the shortfin mako, Isurus oxyrinchus; the oceanic whitetip, Carcharhinus longimanus; and the pelagic thresher, Alopias pelagicus sharks display mesopelagic diving patterns suggestive of their reliance on this biome (Howey et al., 2016; Arostegui et al., 2020; Le Croizier et al., 2020a; Santos et al., 2021). Moreover, a comprehensive review on vertical movements by large epipelagic elasmobranchs and teleosts identified diel patterns in depth use in nearly all species examined (Andrzejaczek et al., 2019). Other extrinsic and intrinsic factors not addressed by this study will expectedly modulate elasmobranch depth use in further detail (e.g., ontogenetic stage; Afonso and Hazin, 2015). Since trophic-mediated vertical movements by epipelagic and mesopelagic fauna promote great interconnectivity and interdependence between ocean depth strata (Sutton, 2013), understanding how the depth gradient is compartmentalized by species movements and how such a compartmentalization influences energy flow within the oceanic community is essential to gain a thorough perspective on ecosystem resilience and vulnerabilities. Given the high, three-dimensional complexity of pelagic ecosystems, such a goal may be only achievable through dedicated, global research integrating the multitude of bioecological and environmental processes which regulate trophodynamics in the open ocean.
Pelagic elasmobranchs are unarguably keystone elements in oceanic ecosystems because they regulate the abundance of other marine taxa including high-order predators (Bornatowski et al., 2018) while providing a swift pathway to energy and nutrient transference between vertical pelagic habitats by making use of a wide portion of the ocean column. Therefore, ascertaining depth-explicit trophic linkages in this group could be most useful to build accurate scenarios for the potential effects of overfishing and climate change on marine ecosystems. The fact that the populations of several pelagic shark species from the South Atlantic have low resilience to fishing pressure and have been declining (e.g., the silky shark declined 12.7%·y-1 according to Santander-Neto et al., 2021; see also Barreto et al., 2016) adds to the growing concerns about a severe, downgrading damage to marine trophic networks potentially produced by ongoing climate change (Nagelkerken et al., 2020), which will expectedly be exacerbated in equatorial latitudes (Chaudhary et al., 2016). Further research on vertical habitat partitioning by pelagic marine predators worldwide is utterly warranted to identify species’ essential habitats along the oceanic vertical gradient and to bridge gaps in knowledge about trophic-mediated structuring processes of pelagic food webs where top-down control is exerted at different depths by distinct species. All this information may prove essential toward the effectiveness of pelagic ecosystem-based fisheries management aimed at reducing species susceptibility to fishing gear and at preserving the structure and functionality of marine trophic webs.
The original contributions presented in the study are included in the article/Supplementary Material. Further inquiries can be directed to the corresponding author.
BCLM and AA conceived and designed the study. BCLM, AA and NB conducted elasmobranch tagging and data sampling. BM and BCLM performed data analysis with contributions from AA and FHVH. AA wrote the manuscript with contributions from all authors. FH supervised the execution of the project. All authors contributed to the article and approved the submitted version.
Funding provided by the TRIATLAS project through the European Union’s Horizon 2020 Research and Innovation Programme (grant No. 817578), the Conselho Nacional de Desenvolvimento Científico e Tecnológico - CNPq (478070/2008-0, 482557/2011-7), the Fundação Grupo Boticário (0760/2007.2), the Save Our Seas Foundation (66/2008), and the Fundação para a Ciência e Tecnologia – FCT (UIDB/04292/2020; UIDP/04292/2020), is deeply acknowledged. Scholarships granted by the FACEPE foundation to NPAB and by CNPq to BCLM (140567/2012-7) are also acknowledged.
The authors declare that the research was conducted in the absence of any commercial or financial relationships that could be construed as a potential conflict of interest.
All claims expressed in this article are solely those of the authors and do not necessarily represent those of their affiliated organizations, or those of the publisher, the editors and the reviewers. Any product that may be evaluated in this article, or claim that may be made by its manufacturer, is not guaranteed or endorsed by the publisher.
We are thankful to all colleagues and interns from Universidade Federal Rural de Pernambuco and to all fishermen who assisted with field work. We acknowledge the Instituto Chico Mendes de Conservação da Biodiversidade (ICMBio), the Brazilian Navy (SECIRM) and the Conselho Nacional de Desenvolvimento Científico e Tecnológico (CNPq) through the Pró-Arquipélago Program (557183/2009-0; 562062/20102-6; 405460/2012-0; 442884/2015-0) for providing precious logistic support.
The Supplementary Material for this article can be found online at: https://www.frontiersin.org/articles/10.3389/fmars.2022.779047/full#supplementary-material
Afonso A. S. (2013). Bioecology and Movement Patterns of Sharks Off Recife, Brazil: Applications in the Mitigation of Shark Attack Hazard (Portugal: Universidade do Algarve). Available at: http://hdl.handle.net/10400.1/2872.
Afonso A. S., Garla R., Hazin F. H. V. (2017). Tiger Sharks can Connect Equatorial Habitats and Fisheries Across the Atlantic Ocean Basin. PLoS One 12, 1–15. doi: 10.1371/journal.pone.0184763
Afonso A. S., Hazin F. H. V. (2015). Vertical Movement Patterns and Ontogenetic Niche Expansion in the Tiger Shark. PLoS One 10, e0116720. doi: 10.1371/journal.pone.0116720
Andrzejaczek S., Gleiss A. C., Pattiaratchi C. B., Meekan M. G. (2019). Patterns and Drivers of Vertical Movements of the Large Fishes of the Epipelagic. Rev. Fish. Biol. Fish. 29, 335–354. doi: 10.1007/s11160-019-09555-1
Arostegui M. C., Gaube P., Berumen M. L., DiGiulian A., Jones B. H., Røstad A., et al. (2020). Vertical Movements of a Pelagic Thresher Shark (Alopias Pelagicus): Insights Into the Species’ Physiological Limitations and Trophic Ecology in the Red Sea. Endanger. Species Res. 43, 387–394. doi: 10.3354/ESR01079
Barreto R., Ferretti F., Flemming J. M., Amorim A., Andrade H., Worm B., et al. (2016). Trends in the Exploitation of South Atlantic Shark Populations. Conserv. Biol. 30, 792–804. doi: 10.1111/cobi.12663
Bernal D., Brill R. W., Dickson K. A., Shiels H. A. (2017). Sharing the Water Column: Physiological Mechanisms Underlying Species-Specific Habitat Use in Tunas. Rev. Fish. Biol. Fish. 27, 843–880. doi: 10.1007/s11160-017-9497-7
Besnard L., Le Croizier G., Galván-Magaña F., Point D., Kraffe E., Ketchum J., et al. (2021). Foraging Depth Depicts Resource Partitioning and Contamination Level in a Pelagic Shark Assemblage: Insights From Mercury Stable Isotopes. Environ. Pollut. 283, 117066. doi: 10.1016/j.envpol.2021.117066
Bezerra N. P. A., Macena B. C. L., Travassos P., Afonso P., Hazin F. H. V. (2019). Evidence of Site Fidelity and Deep Diving Behaviour of Scalloped Hammerhead Shark (Sphyrna Lewini) Around the Saint Peter and Saint Paul Archipelago, in the Equatorial Mid-Atlantic Ridge. Mar. Freshw. Res 71, 708–718. doi: 10.1071/MF19029
Bianchi D., Galbraith E. D., Carozza D. A., Mislan K. A. S., Stock C. A. (2013). Intensification of Open-Ocean Oxygen Depletion by Vertically Migrating Animals. Nat. Geosci. 6, 545–548. doi: 10.1038/ngeo1837
Bird C. S., Veríssimo A., Magozzi S., Abrantes K. G., Aguilar A., Al-Reasi H., et al. (2018). A Global Perspective on the Trophic Geography of Sharks. Nat. Ecol. Evol. 2, 299–305. doi: 10.1038/s41559-017-0432-z
Bivand R., Lewin-Koh N.. (2021). Maptools: Tools for Handling Spatial Objects. R package version 1.1-2. Available at: https://CRAN.R-project.org/package=maptools.
Blum J. D., Popp B. N., Drazen J. C., Choy A. C., Johnson M. W. (2013). Methylmercury Production Below the Mixed Layer in the North Pacific Ocean. Nat. Geosci. 6, 879–884. doi: 10.1038/ngeo1918
Bornatowski H., Angelini R., Coll M., Barreto R. R. P., Amorim A. F. (2018). Ecological Role and Historical Trends of Large Pelagic Predators in a Subtropical Marine Ecosystem of the South Atlantic. Rev. Fish. Biol. Fish. 28, 241–259. doi: 10.1007/s11160-017-9492-z
Bornatowski H., Barreto R., Navia A. F., de Amorim A. F. (2017). Topological Redundancy and ‘Small-World’ Patterns in a Food Web in a Subtropical Ecosystem of Brazil. Mar Ecol. 38, e12407. doi: 10.1111/maec.12407
Braun C. D., Arostegui M. C., Thorrold S. R., Papastamatiou Y. P., Gaube P., Fontes J., et al. (2022). The Functional and Ecological Significance of Deep Diving by Large Marine Predators. Ann. Rev. Mar. Sci. 14, 7.1–7.31. doi: 10.1146/annurev-marine-032521-103517
Carey F. G., Scharold J. V. (1990). Movements of Blue Sharks (Prionace Glauca) in Depth and Course. Mar. Biol. 106, 329–342. doi: 10.1007/BF01344309
Carvalho F., Ahrens R., Murie D., Bigelow K., Aires-Da-Silva A., Maunder M. N., et al. (2015). Using Pop-Up Satellite Archival Tags to Inform Selectivity in Fisheries Stock Assessment Models: A Case Study for the Blue Shark in the South Atlantic Ocean. ICES J. Mar. Sci. 72, 1715–1730. doi: 10.1093/icesjms/fsv026
Cavalcanti E. A. H., Larrazábal M. E. L. (2004). Macrozooplâncton Da Zona Econômica Exclusiva do Nordeste do Brasil (Segunda Expedição Oceanográfica – REVIEZEE/NE II) Com Ênfase Em Copepoda (Crustacea). Ver. Bras. Zool. 21, 467–475. doi: 10.1590/S0101-81752004000300008
Charrad M., Ghazzali N., Boiteau V., Niknafs A. (2014). Nbclust: An R Package for Determining the Relevant Number of Clusters in a Data Set. J. Stat. Software 61, 1–36. doi: 10.18637/jss.v061.i06
Chaudhary C., Saeedi H., Costello M. J. (2016). Bimodality of Latitudinal Gradients in Marine Species Richness. Trends Ecol. Evol. 31, 670–676. doi: 10.1016/j.tree.2016.06.001
Choy C. A., Popp B. N., Hannides C. C. S., Drazen J. C. (2015). Trophic Structure and Food Resources of Epipelagic and Mesopelagic Fishes in the North Pacific Gyre Ecosystem Inferred From Nitrogen Isotopic Compositions. Limnol. Oceanogr. 60, 1156–1171. doi: 10.1002/lno.10085
Compagno L. V. (1990). Alternative Life-History Styles of Cartilaginous Fishes in Time and Space. Environ. Biol. Fish. 28, 33–75. doi: 10.1007/bf00751027
Cortés E. (1999). Standardized Diet Compositions and Trophic Levels of Sharks. ICES J. Mar. Sci. 56, 707–717. doi: 10.1006/jmsc.1999.0489
Costello M. J., Breyer S. (2017). Ocean Depths: The Mesopelagic and Implications for Global Warming. Curr. Biol. 27, R36–R38. doi: 10.1016/j.cub.2016.11.042
Couturier L. I. E., Rohner C. A., Richardson A. J., Marshall A. D., Jaine F. R. A., Bennett M. B., et al. (2013). Stable Isotope and Signature Fatty Acid Analyses Suggest Reef Manta Rays Feed on Demersal Zooplankton. PLoS One 8(10), e77152. doi: 10.1371/journal.pone.0077152
Curnick D. J., Andrzejaczek S., Jacoby D. M. P., Coffey D. M., Carlisle A. B., Chapple T. K., et al. (2020). Behavior and Ecology of Silky Sharks Around the Chagos Archipelago and Evidence of Indian Ocean Wide Movement. Front. Mar. Sci. 7. doi: 10.3389/fmars.2020.596619
Dagorn L., Menczer F., Bach P., Olson R. J. (2000). Co-Evolution of Movement Behaviours by Tropical Pelagic Predatory Fishes in Response to Prey Environment: A Simulation Model. Ecol. Modell. 134, 325–341. doi: 10.1016/S0304-3800(00)00374-4
Davidson L. N. K., Krawchuk M. A., Dulvy N. K. (2016). Why Have Global Shark and Ray Landings Declined: Improved Management or Overfishing? Fish. Fish. 17, 438–458. doi: 10.1111/faf.12119
Dicken M. L., Hussey N. E., Christiansen H. M., Smale M. J., Nkabi N., Cliff G., et al. (2017). Diet and Trophic Ecology of the Tiger Shark (Galeocerdo Cuvier) From South African Waters. PLoS One 12, e0177897. doi: 10.1371/journal.pone.0177897
Dulvy N. K., Forrest R. E. (2010). “Life Histories, Population Dynamics, and Extinction Risks in Chondrichthyans.,” in Sharks and Their Relatives II: Biodiversity, Adaptive Physiology, and Conservation. Eds. Carrier J., Musick J., Heithaus M. (Florida, USA: CRC Press), 655–696.
Dulvy N. K., Pacoureau N., Rigby C. L., Pollom R. A., Jabado R. W., Ebert D. A., et al. (2021). Overfishing Drives Over One-Third of All Sharks and Rays Toward a Global Extinction Crisis. Curr. Biol. 31, 1–15. doi: 10.1016/j.cub.2021.08.062
Espinoza M., Matley J., Heupel M. R., Tobin A. J., Fisk A. T., Simpfendorfer C. A. (2019). Multi-Tissue Stable Isotope Analysis Reveals Resource Partitioning and Trophi(2019). Multi-Tissue Stable Isotope Analysis Reveals Resource Partitioning and Trophic Relationships of Large Reef-Associated Predators. Mar. Ecol. Prog. Ser. 615, 159–176. doi: 10.3354/meps12915
Fairclough D. V., Clarke K. R., Valesini F. J., Potter I. C. (2008). Habitat Partitioning by Five Congeneric and Abundant Choerodon Species (Labridae) in a Large Subtropical Marine Embayment. Estuar. Coast. Shelf Sci. 77, 446–456. doi: 10.1016/j.ecss.2007.10.004
Ferrari S. L. P., Cribari-Neto F. (2004). Beta Regression for Modelling Rates and Proportions. J. Appl. Stat. 31, 799–815. doi: 10.1080/0266476042000214501
Ferretti F., Worm B., Britten G. L., Britten G. L., Lotze H. K. (2010). Patterns and Ecosystem Consequences of Shark Declines in the Ocean. K. Ecol. Lett. 13, 1055–1071. doi: 10.1111/j.1461-0248.2010.01489.x
Filmalter J. D., Cowley P. D., Potier M., Ménard F., Smale M. J., Cherel Y., et al. (2017). Feeding Ecology of Silky Sharks Carcharhinus Falciformis Associated With Floating Objects in the Western Indian Ocean. J. Fish. Biol. 90, 1321–1337. doi: 10.1111/jfb.13241
Froese R., Pauly D. (2021) Fishbase (World Wide Web electronic publication). Available at: http://www.fishbase.org (Accessed February 6, 2021).
Gupta A. K., Nadarajah S. (2004). Handbook of Beta Distribution and Its Applications. 1st Edn (Boca Raton: CRC Press).
Hammerschlag N. (2019). Quantifying Shark Predation Effects on Prey: Dietary Data Limitations and Study Approaches. Endanger. Species Res. 38, 147–151. doi: 10.3354/ESR00950
Hays G. C. (2003). A Review of the Adaptive Significance and Ecosystem Consequences of Zooplankton Diel Vertical Migrations. Hydrobiologia 503, 163–170. doi: 10.1023/B:HYDR.0000008476.23617.b0
Hazen E. L., Johnson D. W. (2010). Meridional Patterns in the Deep Scattering Layers and Top Predator Distribution in the Central Equatorial Pacific. W. Fish. Oceanogr. 19, 427–433. doi: 10.1111/j.1365-2419.2010.00561.x
Heithaus M. R., Wirsing A. J., Dill L. M. (2012). The Ecological Importance of Intact Top-Predator Populations: A Synthesis of 15 Years of Research in a Seagrass Ecosystem. Mar. Freshw. Res. 63, 1039–1050. doi: 10.1071/MF12024
Held L., Schrödle B., Rue H. (2010). “Posterior and Cross-Validatory Predictive Checks: A Comparison on MCMC and INLA,” in Statiscial Modelling and Regression Structures. Eds. Kneib T., Tutz G. (Berlin: Physica-Verlag), 111–131.
Horodysky A. Z., Cooke S. J., Graves J. E., Brill R. W. (2016). Fisheries Conservation on the High Seas: Linking Conservation Physiology and Fisheries Ecology for the Management of Large Pelagic Fishes. Conserv. Physiol. 4, 1–18. doi: 10.1093/conphys/cov059
Howey L. A., Tolentino E. R., Papastamatiou Y. P., Brooks E. J., Abercrombie D. L., Watanabe Y. Y., et al. (2016). Into the Deep: The Functionality of Mesopelagic Excursions by an Oceanic Apex Predator. Ecol. Evol. 6, 5290–5304. doi: 10.1002/ece3.2260
Humphries N. E., Simpson S. J., Wearmouth V. J., Sims D. W. (2016). Two’s Company, Three’s a Crowd: Fine-Scale Habitat Partitioning by Depth Among Sympatric Species of Marine Mesopredator. Mar. Ecol. Prog. Ser. 561, 173–187. doi: 10.3354/meps11937
Irigoien X., Klevjer T. A., Røstad A., Martinez U., Boyra G., Acuña J. L., et al. (2014). Large Mesopelagic Fishes Biomass and Trophic Efficiency in the Open Ocean. Nat. Commun. 5, 3271. doi: 10.1038/ncomms4271
Jackson J. B. C., Kirby M. X., Berger W. H., Bjorndal K. A., Botsford L. W., Bourque B. J., et al. (2001). Historical Overfishing and the Recent Collapse of Coastal Ecosystems. Science 293, 629–638. doi: 10.1126/science.1059199
Jorgensen S. J., Anderson S., Ferretti F., Tietz J. R., Chapple T., Kanive P., et al. (2019). Killer Whales Redistribute White Shark Foraging Pressure on Seals. Sci. Rep. 9 (6153), 1–9. doi: 10.1038/s41598-019-39356-2
Klevjer T. A., Irigoien X., Røstad A., Fraile-Nuez E., Benítez-Barrios V. M., Kaartvedt S. (2016). Large Scale Patterns in Vertical Distribution and Behaviour of Mesopelagic Scattering Layers. Sci. Rep. 6, 19873. doi: 10.1038/srep19873
Kubodera T., Watanabe H., Ichii T. (2007). Feeding Habits of the Blue Shark, Prionace Glauca, and Salmon Shark, Lamna Ditropis, in the Transition Region of the Western North Pacific. Rev. Fish. Biol. Fish. 17, 111–124. doi: 10.1007/s11160-006-9020-z
Lear K. O., Whitney N. M., Morris J. J., Gleiss A. C. (2021). Temporal Niche Partitioning as a Novel Mechanism Promoting Co-Existence of Sympatric Predators in Marine Systems. Proc. R. Soc B. 288, 20210816. doi: 10.1098/rspb.2021.0816
Le Bourg B., Kiszka J. J., Bustamante P., Heithaus M. R., Jaquemet S., Humber F. (2019). Effect of Body Length, Trophic Position and Habitat Use on Mercury Concentrations of Sharks From Contrasted Ecosystems in the Southwestern Indian Ocean. Environ. Res. 169, 387–395. doi: 10.1016/j.envres.2018.11.024
Le Croizier G., Lorrain A., Sonke J. E., Hoyos-Padilla E. M., Galván-Magaña F., Santana-Morales O., et al. (2020a). The Twilight Zone as a Major Foraging Habitat and Mercury Source for the Great White Shark. Environ. Sci. Technol. 54, 15872–15882. doi: 10.1021/acs.est.0c05621
Le Croizier G., Lorrain A., Sonke J. E., Jaquemet S., Schaal G., Renedo M., et al. (2020b). Mercury Isotopes as Tracers of Ecology and Metabolism in Two Sympatric Shark Species. Environ. Pollut. 265, 114931. doi: 10.1016/j.envpol.2020.114931
Li Y., Zhang Y., Dai X. (2016). Trophic Interactions Among Pelagic Sharks and Large Predatory Teleosts in the Northeast Central Pacific. J. Exp. Mar. Bio. Ecol. 483, 97–103. doi: 10.1016/j.jembe.2016.04.013
Lowe C. G., Wetherbee B. M., Crow G. L., Tester a. L. (1996). Ontogenetic Dietary Shifts and Feeding Behavior of the Tiger Shark, Galeocerdo Cuvier, in Hawaiian Waters. L. Environ. Biol. Fishes 47, 203–211. doi: 10.1007/BF00005044
Macena B. C. L. (2016). Habitats Adequados E Aspectos Ecológicos do Tubarão-Baleia (Rhincodon, Typus Smith 1828) No Oceano Atlântico Sudoeste E Equatorial (Brazil: Universidade Federal de Pernambuco). Available at: https://repositorio.ufpe.br/bitstream/123456789/18654/1/Tese_B_Macena_Full_Tubaleia_oficial_FINAL_BIB.pdf.
Madigan D. J., Li M., Yin R., Baumann H., Snodgrass O. E., Dewar H., et al. (2018). Mercury Stable Isotopes Reveal Influence of Foraging Depth on Mercury Concentrations and Growth in Pacific Bluefin Tuna. Environ. Sci. Technol. 52, 6256–6264. doi: 10.1021/acs.est.7b06429
Madigan D. J., Richardson A. J., Carlisle A. B., Weber S. B., Brown J., Hussey N. E. (2021). Water Column Structure Defines Vertical Habitat of Twelve Pelagic Predators in the South Atlantic. ICES J. Mar. Sci. 78, 867–883. doi: 10.1093/icesjms/fsaa222
Mangiafico S. S. (2015). An R Companion for the Handbook of Biological Statistics, version 1.3.2. (New Brunswick (NJ, USA): Rutgers Cooperative Extension). Available at: rcompanion.org/rcompanion/.
Mendonça S. A., Macena B. C. L., Afonso A. S., Hazin F. H. V. (2018). Seasonal Aggregation and Diel Activity by the Sicklefin Devil Ray Mobula Tarapacana Off a Small, Equatorial Outcrop of the Mid-Atlantic Ridge. J. Fish. Biol. 93, 1121–1129. doi: 10.1111/jfb.13829
Motta P. J., Maslanka M., Hueter R. E., Davis R. L., de la Parra R., Mulvany S. L., et al. (2010). Feeding Anatomy, Filter-Feeding Rate, and Diet of Whale Sharks Rhincodon Typus During Surface Ram Filter Feeding Off the Yucatan Peninsula, Mexico. Zoology 113, 199–212. doi: 10.1016/j.zool.2009.12.001
Mulas A., Bellodi A., Cannas R., Carbonara P., Cau A., Marongiu M. F., et al. (2019). Resource Partitioning Among Sympatric Elasmobranchs in the Central-Western Mediterranean Continental Shelf. Mar. Biol. 166, 153. doi: 10.1007/s00227-019-3607-0
Munroe S. R. M., Simpfendorfer C. A., Heupel M. R. (2014). Defining Shark Ecological Specialisation: Concepts, Context, and Examples. Rev. Fish. Biol. Fish. 24, 317–331. doi: 10.1007/s11160-013-9333-7
Musyl M. K., Brill R. W., Curran D. S., Fragoso N. M., McNaughton L. M., Nielsen A., et al. (2011). Postrelease Survival, Vertical and Horizontal Movements, and Thermal Habitats of Five Species of Pelagic Sharks in the Central Pacific Ocean. Fish. Bull. 109, 341–368. https://spo.nmfs.noaa.gov/sites/default/files/pdf-content/2011/1094/1094musyl.pdf
Nagelkerken I., Goldenberg S. U., Ferreira C. M., Ullah H., Connell S. D. (2020). Trophic Pyramids Reorganize When Food Webs Architecture Fails to Adjust to Ocean Change. Science 369, 829–832. doi: 10.1126/science.aax0621
Ogle D. H., Doll J. C., Wheeler P., Dinno A. (2021) R Package. Available at: https://github.com/droglenc/FSA.
Olivar M. P., Hulley P. A., Castellón A., Emelianov M., López C., Tuset V. M., et al. (2017). Mesopelagic Fishes Across the Tropical and Equatorial Atlantic: Biogeographical and Vertical Patterns. Prog. Oceanography 151, 116–137. doi: 10.1016/j.pocean.2016.12.001
Ortiz M., Arocha F. (2004). Alternative Error Distribution Models for Standardization of Catch Rates of Non-Target Species From a Pelagic Longline Fishery: Billfish Species in the Venezuelan Tuna Longline Fishery. Fish. Res. 70, 275–297. doi: 10.1016/j.fishres.2004.08.028
Pacoureau N., Rigby C. L., Kyne P. M., Sherley R. B., Winker H., Carlson J. K., et al. (2021). Half a Century of Global Decline in Oceanic Sharks and Rays. Nature 589, 567–571. doi: 10.1038/s41586-020-03173-9
Páez-Rosas D., Insuasti-Zarate P., Riofrío-Lazo M., Galván-Magaña F. (2018). Feeding Behavior and Trophic Interaction of Three Shark Species in the Galapagos Marine Reserve. PeerJ 6, e4818. doi: 10.7717/peerj.4818
Papastamatiou Y. P., Wetherbee B. M., Lowe C. G., Crow G. L. (2006). Distribution and Diet of Four Species of Carcharhinid Shark in the Hawaiian Islands: Evidence for Resource Partitioning and Competitive Exclusion. Mar. Ecol. Prog. Ser. 320, 239–251. doi: 10.3354/meps320239
Paradinas I., Pennino M. G., López-Quílez A., Marín M., Bellido J. M., Conesa D. (2018). Modelling Spatially Sampled Proportion Processes. REVSTAT Stat. J. 16, 71–86. doi: 10.57805/revstat.v16i1.233
Pauly D., Christensen V., Dalsgaard J., Froese R., Torres (1998). Fishing Down Marine Food Webs. Science 279, 860–863. doi: 10.1126/science.279.5352.860
Pinela A. M., Borrell A., Cardona L., Aguilar A. (2010). Stable Isotope Analysis Reveals Habitat Partitioning Among Marine Mammals Off the NW African Coast and Unique Trophic Niches for Two Globally Threatened Species. Mar. Ecol. Prog. Ser. 416, 295–306. doi: 10.3354/meps08790
Polovina J. J., Woodworth-Jefcoats P. A. (2013). Fishery-Induced Changes in the Subtropical Pacific Pelagic Ecosystem Size Structure: Observations and Theory. PLoS One 8, e62341. doi: 10.1371/journal.pone.0062341
Proud R., Cox M. J., Brierley A. S. (2017). Biogeography of the Global Ocean’s Mesopelagic Zone. Curr. Biol. 27, 113–119. doi: 10.1016/j.cub.2016.11.003
Queiroz N., Humphries N. E., Couto A., Vedor M., da Costa I., Sequeira A. M. M., et al. (2019). Global Spatial Risk Assessment of Sharks Under the Footprint of Fisheries. Nature 572, 461–466. doi: 10.1038/s41586-019-1444-4
R Core Team (2021). “R: A Language and Environment for Statistical Computing, (Vienna, Austria: R Foundation for Statistical Computing).
Receveur A., Allain V., Menard F., Dhaussy A. L., Laran S., Ravache A., et al. (2021). Modelling Marine Predator Habitat Using the Abundance of its Pelagic Prey in the Tropical South-Western Pacific. Ecosystems 1–23. doi: 10.1007/s10021-021-00685-x
Robinson C., Steinberg D. K., Anderson T. R., Arístegui J., Carlson C. A., Frost J. R., et al. (2010). Mesopelagic Zone Ecology and Biogeochemistry - A Synthesis. Deep. Res. Part II Top. Stud. Oceanogr. 57, 1504–1518. doi: 10.1016/j.dsr2.2010.02.018
Robison B. H. (2009). Conservation of Deep Pelagic Biodiversity. Conserv. Biol. 23, 847–858. doi: 10.1111/j.1523-1739.2009.01219.x
Rohner C. A., Couturier L. I. E., Richardson A. J., Pierce S. J., Prebble C. E. M., Gibbons M. J., et al. (2013). Diet of Whale Sharks Rhincodon Typus Inferred From Stomach Content and Signature Fatty Acid Analyses. Mar. Ecol. Prog. Ser. 493, 219–235. doi: 10.3354/meps10500
Sabando M. A., Rieucau G., Bradley D., Caselle J. E., Papastamatiou Y. P. (2020). Habitat-Specific Inter and Intraspecific Behavioral Interactions Among Reef Sharks. Oecologia 193, 371–376. doi: 10.1007/s00442-020-04676-y
Santos C. C., Domingo A., Carlson J., Natanson L. J., Travassos P., Macías D., et al. (2021). Movements, Habitat Use, and Diving Behavior of Shortfin Mako in the Atlantic Ocean. Front. Mar. Sci. 8. doi: 10.3389/fmars.2021.686343
Santander-Neto J., Barreto R., Santana F. M., Lessa R. P.T. (2021). Age, Growth and Demography of the Silky Shark Carcharhinus Falciformis From the Southwestern Atlantic. Endang. Species Res. 45, 237–49. doi: 10.3354/esr01131
Scheffer M., Carpenter S., de Young B. (2005). Cascading Effects of Overfishing Marine Systems. Trends Ecol. Evol. 20, 579–581. doi: 10.1016/j.tree.2005.08.018
Sequeira A. M.M., Heupel M. R., Lea M. A., Eguíluz V. M., Duarte C. M., Meekan M. G., et al. (2019). The Importance of Sample Size in Marine Megafauna Studies. Ecol. Appl. 29, e01947. doi: 10.1002/eap.1947
Smith A. D., Houde A. L. S., Neff B., Peres-Neto P. R. (2017). Effects of Competition on Fitness-Related Traits. Oecologia 183, 701–713. doi: 10.1007/s00442-017-3816-5
Sutton T. T. (2013). Vertical Ecology of the Pelagic Ocean: Classical Patterns and New Perspectives. J. Fish. Biol. 83, 1508–1527. doi: 10.1111/jfb.12263
Sutton T. T., Wiebe P. H., Madin L., Bucklin A. (2010). ). Diversity and Community Structure of Pelagic Fishes to 5000m Depth in the Sargasso Sea. Deep. Res. Part II Top. Stud. Oceanogr. 57, 2220–2233. doi: 10.1016/j.dsr2.2010.09.024
Thorrold S. R., Afonso P., Fontes J., Braun C. D., Santos R. S., Skomal G. B., et al. (2014). Extreme Diving Behaviour in Devil Rays Links Surface Waters and the Deep Ocean. Nat. Commun. 5 (4274), 1–7. doi: 10.1038/ncomms5274
Tillett B. J., Meekan M. G., Field I. C. (2014). Dietary Overlap and Partitioning Among Three Sympatric Carcharhinid Sharks. Endanger. Species Res. 25, 283–293. doi: 10.3354/esr00615
Trindade-Santos I., Moyes F., Magurran A. E. (2020). Global Change in the Functional Diversity of Marine Fisheries Exploitation Over the Past 65 Years. Proc. R. Soc B. Biol. Sci. 287, 20200889. doi: 10.1098/rspb.2020.0889
Tyminski J. P., de la Parra-Venegas R., Cano J. G., Hueter R. E. (2015). Vertical Movements and Patterns in Diving Behavior of Whale Sharks as Revealed by Pop-Up Satellite Tags in the Eastern Gulf of Mexico. PLoS One 10, e0142156. doi: 10.1371/journal.pone.0142156
Vaske Júnior T., Lessa R. P., Gadig O. B. F. (2009a). Feeding Habits of the Blue Shark (Prionace Glauca) Off the Coast of Brazil. Biota Neotrop. 9, 55–60. doi: 10.1590/S1676-06032009000300004
Vaske Júnior T., Vooren C. M., Lessa R. P. (2009b). Feeding Strategy of the Night Shark (Carcharhinus Signatus) and Scalloped Hammerhead Shark (Sphyrna Lewini) Near Seamounts Off Northeastern Brazil. Braz. J. Oceanogr. 57, 97–104. doi: 10.1590/s1679-87592009000200002
Vedor M., Mucientes G., Hernández-Chan S., Rosa R., Humphries N., Sims D. W., et al. (2021). Oceanic Diel Vertical Movement Patterns of Blue Sharks Vary With Water Temperature and Productivity to Change Vulnerability to Fishing. Front. Mar. Sci. 8. doi: 10.3389/fmars.2021.688076
Vereshchaka A., Abyzova G., Lutina A., Musaeva E. (2017). The Deep-Sea Zooplankton of the North, Central, and South Atlantic: Biomass, Abundance, Diversity. Deep-Sea Res. II 137, 89–101. doi: 10.1016/j.dsr2.2016.06.017
Keywords: diving behavior, foraging strategy, telemetry, marine predators, mesopelagic, shark, ray
Citation: Afonso AS, Macena BCL, Mourato B, Bezerra NPA, Mendonça S, de Queiroz JDGR and Hazin FHV (2022) Trophic-Mediated Pelagic Habitat Structuring and Partitioning by Sympatric Elasmobranchs. Front. Mar. Sci. 9:779047. doi: 10.3389/fmars.2022.779047
Received: 17 September 2021; Accepted: 16 May 2022;
Published: 17 June 2022.
Edited by:
Clive N. Trueman, University of Southampton, United KingdomReviewed by:
Víctor Hugo Cruz-Escalona, Instituto Politécnico Nacional (IPN), MexicoCopyright © 2022 Afonso, Macena, Mourato, Bezerra, Mendonça, de Queiroz and Hazin. This is an open-access article distributed under the terms of the Creative Commons Attribution License (CC BY). The use, distribution or reproduction in other forums is permitted, provided the original author(s) and the copyright owner(s) are credited and that the original publication in this journal is cited, in accordance with accepted academic practice. No use, distribution or reproduction is permitted which does not comply with these terms.
*Correspondence: Bruno C. L. Macena, YnJ1bm9tYWNlbmFAZ21haWwuY29t
†These authors have contributed equally to this work
‡Deceased
Disclaimer: All claims expressed in this article are solely those of the authors and do not necessarily represent those of their affiliated organizations, or those of the publisher, the editors and the reviewers. Any product that may be evaluated in this article or claim that may be made by its manufacturer is not guaranteed or endorsed by the publisher.
Research integrity at Frontiers
Learn more about the work of our research integrity team to safeguard the quality of each article we publish.